DOI:
10.1039/C7SC05087A
(Edge Article)
Chem. Sci., 2018,
9, 2552-2558
A chemiluminescent probe for cellular peroxynitrite using a self-immolative oxidative decarbonylation reaction†
Received
28th November 2017
, Accepted 31st January 2018
First published on 31st January 2018
Abstract
Peroxynitrite (ONOO−) is a highly reactive oxygen species which has been recognized as an endogenous mediator of physiological activities like the immune response as well as a damaging agent of oxidative stress under pathological conditions. While its biological importance is becoming clearer, many of the details of its production and mechanism of action remain elusive due to the lack of available selective and sensitive detection methods. Herein, we report the development, characterization, and biological applications of a reaction-based chemiluminescent probe for ONOO− detection, termed as PNCL. PNCL reacts with ONOO−via an isatin moiety through an oxidative decarbonylation reaction to initiate light emission that can be observed instantly with high selectivity against other reactive sulphur, oxygen, and nitrogen species. Detailed studies were performed to study the reaction between isatin and ONOO−, which confirm selectivity for ONOO− over NO2˙. PNCL has been applied for ONOO− detection in aqueous solution and live cells. Moreover, PNCL can be employed to detect cellular ONOO− generated in macrophages stimulated to mount an immune response with lipopolysaccharide (LPS). The sensitivity granted by chemiluminescent detection together with the specificity of the oxidative decarbonylation reaction provides a useful tool to explore ONOO− chemistry and biology.
Introduction
Peroxynitrite (ONOO−) is a highly reactive oxygen species that can be formed biologically from a diffusion-controlled reaction between superoxide (O2˙−) and nitric oxide (NO˙).1 In biological systems, ONOO− has long been known as a deleterious species due to its oxidative damage to lipids, proteins, and nucleic acids.2 Abnormal regulation of ONOO− in living systems is associated with diseases such as ischemia-reperfusion,3 diabetes,4 cardiac dysfunction,5 inflammatory conditions,6 autoimmune, and neurodegenerative diseases.7,8 However, ONOO− has been proposed to act as a physiological mediator in certain contexts, for example in immune system function9 and ischemic preconditioning.10 Macrophages produce nitric oxide and superoxide to form ONOO− to attack invading pathogens.11 Macrophage cells recognize lipopolysaccharide (LPS), a component of the bacterial cell wall, which induces high expression of inducible nitric oxide synthase (iNOS).12 The effect of LPS is amplified by release of IFN-β, providing a mechanism for robust local production of NO˙ and ONOO−. Although important biological functions of ONOO− are still emerging, there remains a lack of efficient and selective methods to monitor its production in real time to study its effects in living systems. Therefore, the development of precise approaches for detecting ONOO− is crucial to provide a more detailed understanding of its complex biological effects.
Much of the difficulty of studying the biological roles of ONOO− stems from its complex chemistry. ONOO− coexists with its protonated form ONOOH (pKa 6.8) under physiological pH, and can directly undergo two-electron reactions with thiols,13 CO2,14 and carbonyls.15 The H+ and CO2 adducts, ONOOH and ONOOCO2−, rapidly decompose via homolytic cleavage reactions to form secondary radicals such as hydroxyl radical (HO˙), carbonate radical (CO3˙−), and nitrogen dioxide (NO2˙), which mediate potent one-electron chemistry, including tyrosine nitration.16 ONOO− can also form from reaction between O2 and NO− produced by photolysis of Angeli's salt under alkaline conditions.17 Under physiological conditions, Angeli's salt has been widely used as a nitroxyl (HNO) donor for biological studies. Reaction of HNO with O2 also proceeds at neutral pH to form a potent oxidative species and represents an important decomposition pathway of HNO.18,19 While there are similarities and differences between ONOO− and the autooxidation product of HNO formed at neutral pH, the details of this chemistry and the exact species eliciting the oxidative effect of Angeli's salt is still under debate.
The classical approach for studying biological ONOO− relies on immunostaining of 3-nitrotyrosine residues,20 which lacks temporal precision and is an indirect method tracking the “footprint” of ONOO−. A large number of reaction-based fluorescent probes have been developed in an attempt to solve this problem. These small molecule tools can react with chemical species as they are produced, enabling detection within living intact systems. Several families of reaction-based fluorescent probes for ONOO− detection have been reported and applied in biological studies, such as trifluorocarbonyl-based,21N-dearylation-based,22 and boronic ester-based23 probes. Organoselenium-based,24 and organotellurium-based25 probes are advantageous because the fluorescence signal is reversible, but depend upon reaction with cellular reductants to reverse the signal.26 This limited reversibility, as well as other intrinsic drawbacks of fluorescent detection methods such as photobleaching and autofluorescence highlights the need for new methods of ONOO− detection. Chemiluminescence provides an attractive solution for studying biological molecules and processes due to the low background, high signal-to-noise ratio, and reversible signal of this technique.27 Nanoparticle-based methods have recently been reported for chemiluminescent ONOO− detection.28,29 These probes rely on an interesting radical-pair mechanism to generate signal from the ONOO− decomposition products HO˙ and O2˙−. Although these systems do display good sensitivity, complementary approaches that operate via direct reaction with ONOO− are desirable. Chemiluminescent probes based on sterically hindered 1,2-dioxetanes offer opportunities to incorporate specific reaction-based triggers and have already been applied for detection of analytes in cellular systems and for live animal imaging.30–34 One fruitful design strategy uses Schaap's adamantylidene-dioxetane attached to an analyte-specific reactive handle.35 A striking substituent effect for Schaap's 1,2-dioxetanes has been reported, where incorporation of an electron withdrawing group such as an acrylonitrile at the ortho position of the phenol results in a significant increase in the overall chemiluminescent quantum yield of 1,2-dioxetanes.36 As a result, chemiluminescent emission can be observed in aqueous conditions without the addition of any polymeric enhancer solutions. We herein describe the development and application of a selective chemiluminescent probe for ONOO− detection, PNCL, with both in vitro and live cell applications.
Results and discussion
Design and synthesis of PNCL
As part of a program to develop chemical probes for imaging reactive sulphur,29,37 oxygen,30,38,39 and nitrogen species, our laboratory has previously reported that isatin reacts with ONOO−via an oxidative decarbonylation reaction to generate anthranilic acid.4019F NMR probes, 5-fluoroisatin and 6-fluorisatin, have been developed for selective detection of ONOO− that utilize this newly discovered transformation. In order to expand this reaction-based detection strategy for luminogenic detection, we adopted a self-immolative 1,6-elimination strategy for chemiluminescent ONOO− detection that relies on tethering an isatin moiety to a 1,2-dioxetane chemiluminescent scaffold via an ether linkage (Scheme 1). The isatin serves as the reaction handle, and 1,6-elimination can be triggered after reacting with ONOO− to form the anthranilic acid with the amino group positioned para to the benzylic ether. Luminescent emission is then initiated upon the decomposition of the 1,2-dioxetane through a chemically initiated electron exchange luminescence (CIEEL) mechanism. Synthesis of a peroxynitrite chemiluminescent probe (PNCL) proceeded from commercially available 5-iodoisatin 1 by protection of the ketone group as the dimethylacetal 2 (Scheme 2). The protected 5-iodoisatin was subjected to palladium catalyzed reductive formylation with N-formyl saccharin as the CO source to form the aldehyde 3,41 followed by reduction of the aldehyde with NaBH4 and deprotection to afford benzyl alcohol 4. The alcohol 4 was linked to the phenol 5 (ref. 36) through a Mitsunobu reaction to deliver the enol ether precursor 6. This precursor underwent [2 + 2] cycloaddition with photogenerated singlet oxygen using Rose bengal as a sensitizer to provide the final 1,2-dioxetane PNCL.
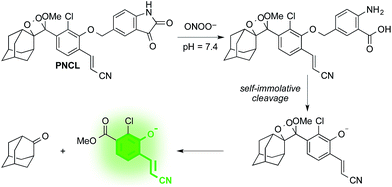 |
| Scheme 1 Spiroadamantane 1,2-dioxetane-based probe for chemiluminescent ONOO− detection. | |
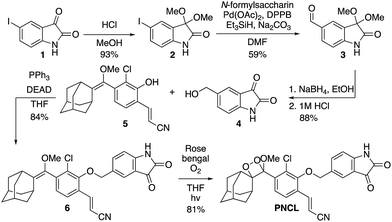 |
| Scheme 2 Synthesis of PNCL. | |
Spectral response of the probes to ONOO−
After obtaining PNCL, its photophysical properties and reactivity towards ONOO− were characterized. Luminescence emission spectra were collected using an F-7000 Hitachi spectrophotometer by treating 20 μM PNCL with 0–200 μM ONOO− (Fig. 1). An emission peak centered at 525 nm was observed in the presence of ONOO− with ∼28-fold increase in peak luminescence intensity compared to a blank control (Fig. 1A). The reaction between PNCL and ONOO− was analyzed by GC-MS, confirming the generation of 2-adamantanone and 2-chloro-4-acrylonitrile-3-(methoxycarbonyl)phenol (Fig. S1†). The luminescence emission slowly decayed over a course of 20 min (Fig. 1B), consistent with fast reaction kinetics of ONOO− with the isatin moiety of PNCL,40 followed by a slower 1,6-elimination. Interestingly, ONOO− can be added to a solution of PNCL over several cycles with good recovery of chemiluminescent signal (Fig. S2†). This shows that the reversible chemiluminescent emission has good reproducibility and can be used monitor ONOO− fluxes over time. The dose dependence of the peak chemiluminescent emission at 525 nm measured 10 seconds after ONOO− addition showed excellent linearity, demonstrating that ONOO− can be quantified using PNCL (Fig. 1C). Calibration of the response of PNCL with lower concentrations of ONOO− revealed a detection limit of 6 nM (3σ) bolus ONOO− (Fig. S3†), comparing favorably to previous fluorescent and chemiluminescent methods (Table S1†). The reactivity of PNCL towards SIN-1 was also evaluated (Fig. 1D), showing that PNCL can monitor the slow release of ONOO− over time, as would be observed in biological systems.
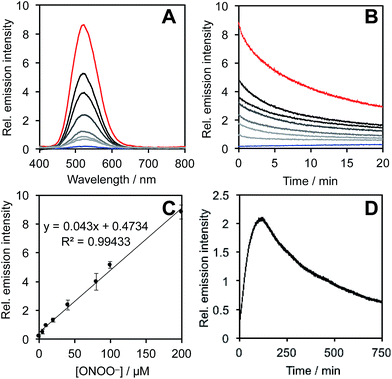 |
| Fig. 1 Response of PNCL to ONOO−. (A) Chemiluminescent emission spectra of 20 μM PNCL and 0 (blue trace), 5, 10, 20, 40, 80, 100, 200 μM (red trace) ONOO−. (B) Time scans of the chemiluminescent emission at 525 nm of 20 μM PNCL and 0 (blue trace), 5, 10, 20, 40, 80, 100, 200 μM (red trace) ONOO−. (C) Peak emission intensity at 525 nm of 20 μM PNCL after adding 0–200 μM ONOO−. (D) Time scan of the chemiluminescent emission at 525 nm of 20 μM PNCL and 200 μM SIN-1. All experiments were performed in 20 mM HEPES (pH 7.4), containing <1% DMSO. | |
The selectivity of PNCL for ONOO− was tested against a panel of biologically relevant reactive sulphur, oxygen, and nitrogen species and metal cations. The response of PNCL to 200 μM ONOO− was compared against other analytes in HEPES buffer (20 mM, pH 7.4), by adding 5 mM reduced glutathione (GSH), 1 mM L-cysteine, or 200 μM other reactive species. Most of the species tested displayed no significant increase in luminescence intensity over the blank control within 20 min (Fig. 2). Given the destructive reactivity of HO˙, we examined the interference of this species by treating PNCL with ONOO− in the presence of the HO˙-generating Fenton system (Fig. S4†), where only a minimal reduction in signal was observed. A small luminescence increase was observed when treating PNCL with Angeli's salt, commonly used as a nitroxyl (HNO) donor. This was consistent with previous observations using HPLC to track the reactivity of 5-fluoroisatin with Angeli's salt.37
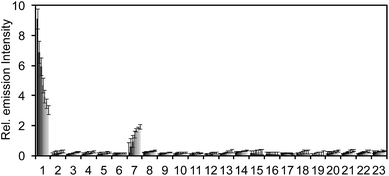 |
| Fig. 2 Selectivity of PNCLversus cations and reactive sulphur, oxygen, and nitrogen species. Chemiluminescent emission at 525 nm of 20 μM PNCL and 200 μM biologically relevant analytes in 20 mM HEPES (pH 7.4). Bars represent chemiluminescent emission at 525 nm at 4, 8, 12, 16, 20 min after addition of reactive species. Legend: (1) ONOO−, (2) Cys, (3) DEA NONOate, (4) GSH (5 mM), (5) GSNO, (6) H2O2, (7) Angeli's salt, (8) KO2, (9) Na2S, (10) Na2S2O3, (11) Na2SO4, (12) NaNO2, (13) HO˙, (14) OCl−, (15) tBuOOH, (16) 1O2, (17) Na+, (18) Mg2+, (19) K+, (10) Ca2+, (21) Zn2+, (21) Fe2+, (23) blank. Error bars are ± S.D. All experiments were performed in 20 mM HEPES or 20 mM PBS (pH 7.4), containing <1% DMSO. | |
In light of studies exploring the generation of ONOO− and other nitrogen oxide species from the autooxidation of NO− and HNO,17–19 we performed a series of competition experiments with HCO3− and GSH to better understand the luminescence increase resulting from reaction of PNCL with Angeli's salt. ONOO− reacts rapidly with CO2, but slowly with GSH. HNO, on the other hand, reacts rapidly with GSH.42 We treated PNCL with ONOO− (Fig. 3A and B) or Angeli's salt (Fig. 3C and D) in the presence of various amount of HCO3− (1 mM, 2 mM, 3 mM, 4 mM, and 5 mM). HCO3− decreased the chemiluminescence emission intensity generated by both ONOO− and Angeli's salt, providing evidence that Angeli's salt can generate ONOO−. A similar experiment was performed by reacting PNCL (20 μM) with ONOO− (Fig. 4A and B) or Angeli's salt (Fig. 4C and D) and various amounts of GSH (50 μM, 100 μM, 200 μM, and 1 mM). However, the GSH only quenched the luminescence intensity from Angeli's salt (Fig. 4B) and no significant luminescence decrease was observed from ONOO− (Fig. 4A). Interestingly, substoichometric quantities of GSH would not only reduce the total amount of luminescence, but displayed a lag time before producing a chemiluminescent signal (Fig. 4C). Angeli's salt generates HNO with a half-life of 2.3 minutes at 37 °C. The observed lag time of the chemiluminescent response is consistent with GSH scavenging the newly formed HNO more rapidly than the autooxidation reaction of HNO. Once the GSH is consumed, HNO can be oxidized to ONOO− or another nitrogen oxide species capable of two-electron chemistry and reaction with PNCL proceeds to produce a luminescent signal.
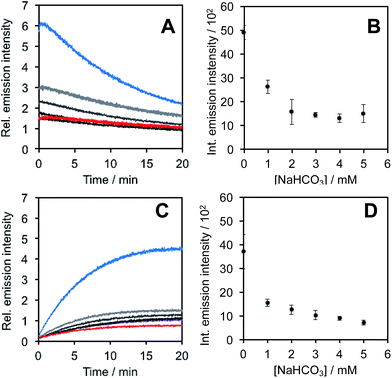 |
| Fig. 3 Inhibition of response by NaHCO3. (A) Time scans and (B) integrated intensity of the chemiluminescent emission of 20 μM PNCL, 200 μM ONOO− and 0 (blue trace), 1 mM, 2 mM, 3 mM, 4 mM, and 5 mM (red trace) NaHCO3. (C) Time scans and (D) integrated intensity of the chemiluminescent emission of 20 μM PNCL, 200 μM Angeli's salt and 0 (blue trace), 1 mM, 2 mM, 3 mM, 4 mM, and 5 mM (red trace) NaHCO3. All experiments were performed in 20 mM HEPES (pH 7.4), containing <1% DMSO. | |
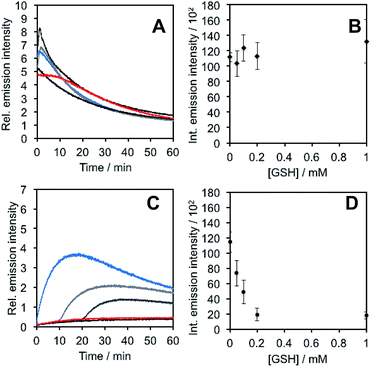 |
| Fig. 4 Inhibition of response by glutathione. (A) Time scans and (B) integrated intensity of the chemiluminescent emission of 20 μM PNCL, 200 μM ONOO− and 0 (blue trace), 50 μM, 100 μM, 200 μM, and 1 mM (red trace) glutathione. (C) Time scans and (D) integrated intensity of the chemiluminescent emission of 20 μM PNCL, 200 μM Angeli's salt and 0 (blue trace), 50 μM, 100 μM, 200 μM, and 1 mM (red trace) glutathione. All experiments were performed in 20 mM HEPES (pH 7.4), containing <1% DMSO. | |
In order to confirm this selectivity and that HCO3−/CO2 does not scavenge HNO, we prepared a fluorescent nitroxyl (HNO) probe, herein called XF1 (Fig. 5A). HNO reacts with the diphenylphosphinobenzoyl group to form an aza-ylide that will nucleophilically attack the ester to trigger the release of the fluorophore.43,44 A time-dependent fluorescence turn-on was observed when treating 10 μM XF1 with 200 μM Angeli's salt (Fig. S5A and B†), and selectivity tests demonstrated that XF1 can distinguish HNO from other reactive sulfur, oxygen, and nitrogen species (Fig. S5C†). Inhibition experiments were conducted by treating XF1 with various amounts of HCO3− or GSH together with Angeli's salt. While no obvious inhibition of the fluorescence response was observed with the addition of HCO3−, the fluorescence was completely quenched with 200 μM GSH (Fig. 5B and C). These results confirm that GSH scavenges HNO generated from Angeli's salt but HCO3−/CO2 does not scavenge HNO. Taken together, the results from Fig. 3–5 are consistent with the luminescence increase from Angeli's salt being due to the generation of ONOO−. We do note that alternative oxidative species formed from HNO have been proposed.18 Although we cannot completely rule this out, the results here are consistent with the formation of ONOO− from HNO and support the high specificity of PNCL for detecting ONOO−. As a final mechanistic investigation, we examined the selectivity of ONOO−versus NO2˙, HO˙, and CO3˙− (the decomposition products of ONOO−) by treating isatin with ONOO− in the presence of HCO3−/CO2 or TEMPOL, a free radical scavenger (Fig. S6†). While HCO3−/CO2 inhibits the reaction, TEMPOL has no effect. This suggests that isatin reacts directly with ONOO− and not with free radical species like NO2˙, HO˙, or CO3˙− that are formed via spontaneous ONOO− decomposition.
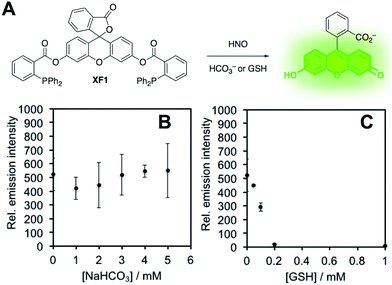 |
| Fig. 5 HNO scavenging by NaHCO3 and GSH. (A) Design of XF1, a fluorescent probe for HNO. (B) Peak emission intensity of 10 μM XF1, 200 μM Angeli's salt and 0–5 mM NaHCO3. (C) Peak emission intensity of 10 μM XF1, 200 μM Angeli's salt and 0–1 mM glutathione. All experiments were performed in 20 mM HEPES (pH 7.4), containing <1% DMSO with λex = 488 nm. | |
Evaluation of PNCL for ONOO− detection in living cells
After demonstrating that PNCL reacts with ONOO− with high sensitivity and selectivity, we then proceeded to investigate the ability of PNCL for ONOO− detection in living cells using a BioTek Cytation 5 plate reader. Toxicity was determined by the MTT assay, which indicated low toxicity after 24 hour treatment with PNCL at concentrations less than 100 μM and more than 50% viability after treating with high concentrations of 1 mM PNCL for 24 hours (Fig. S7†). Cellular internalization was verified by imaging the intrinsic fluorescence emission of PNCL (Fig. S8†). In order to evaluate the ability of PNCL to detect cellular delivery of ONOO− from donor compounds, RAW 264.7 macrophages were incubated with PNCL for 30 minutes, washed, and then treated with 0, 200 μM, 400 μM, 800 μM, 1 mM, or 2 mM SIN-1, a commonly used ONOO− donor that operates by simultaneously generating NO˙ and O2˙−. A time course of the chemiluminescent emission intensity increased to reach a maximum at 40 minutes (Fig. 6A). Both the peak and integrated luminescence intensities increased with increasing concentrations of SIN-1 (Fig. 6A and B), and a 5-fold luminescence increase was obtained with 2 mM SIN-1 compared with a blank control. We also performed the same experiment in A549 cells (Fig. S9†). Similar results were observed and excellent linearity was achieved in living cells between 0 and 400 μM SIN-1 (Fig. S9C†). This increase in chemiluminescent emission from SIN-1 and PNCL was attenuated by the ONOO− scavenger Mn(III)TMPyP (Fig. S10†), confirming that the response is due to ONOO−.
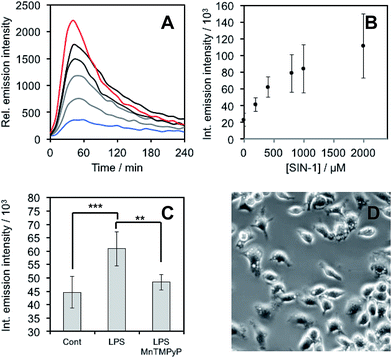 |
| Fig. 6 ONOO− detection in RAW 264.7 macrophages. (A) Time scans and (B) integrated intensity of chemiluminescence emission of RAW 264.7 macrophages incubated with 20 μM PNCL for 30 minutes, washed, and then treated with 0 (blue trace), 200 μM, 400 μM, 800 μM, 1 mM, and 2 mM (red trace) SIN-1. (C) Integrated chemiluminescent emission intensity of RAW 264.7 macrophages stimulated with (Cont) Vehicle control, (LPS) 1000 ng mL−1 LPS, or (LPS, MnTMPyP) 1000 ng mL−1 LPS and 25 μM Mn(III)TMPyP and then treated with 20 μM PNCL. (D) Brightfield images of stimulated RAW 264.7 macrophages. Error bars are S.D. from n = 3–7 wells and 3 biological replicates (n = 10 wells from 6 biological replicates for the control experiment). Statistical significance was assessed using a two-tailed student's t-test. ***p < 0.001, **p < 0.01. | |
Finally, we investigated the ability of PNCL to detect ONOO− generated by macrophages stimulated to mount an immune response. Lipopolysaccharide (LPS) binds to the toll-like receptors of macrophages, activating the transcription factor NF-κβ ultimately leading to the expression of iNOS and generation of reactive oxygen species that can produce ONOO−.12 Macrophages stimulated with LPS produced increased chemiluminescent signal from PNCL (Fig. 6C), showing a 36% increase in integrated luminescence intensity with 1000 ng mL−1 LPS. Treatment with the ONOO− scavenger Mn(III)TMPyP reduces this signal back to control levels and co-incubation with the selective iNOS inhibitor 1400 W also reduces the signal from LPS and PNCL (Fig. S11†). These control experiments validate the ability of PNCL to detect cellular production of ONOO−. Bright field images of the cells after LPS stimulation show the formation of phagosomes (Fig. 6D), a phenotypic response to LPS exposure.
Conclusions
We have designed and synthesized a reaction-based chemiluminescent probe for ONOO−, PNCL. PNCL reacts with ONOO− through an oxidative decarbonylation reaction to initiate light emission that can be observed instantly with high sensitivity and selectivity against other reactive sulphur, oxygen, and nitrogen species. The observed reactivity towards Angeli's salt is attributed to the generation of ONOO−, which is supported by inhibitor experiments with HCO3− and GSH, providing experimental evidence of an autooxidative product of HNO. Several studies have purported that the autooxidation of HNO produces a product unique from ONOO−, as evidenced by data showing no effect of HCO3− on the reaction between Angeli's salt and reactive oxygen species probe dihydrorhodamine 123.18 In our studies, however, we observe a drastic HCO3− dependent reduction in chemiluminescent signal from PNCL and Angeli's salt, consistent with ONOO− formation from HNO under physiological conditions.19PNCL was used to detect ONOO− generated from donor compounds in multiple cell lines, as well as cellular ONOO− generated by macrophages stimulated with LPS. Confirmation of the chemical specificity towards ONOO− over downstream free radical products, coupled with operational compatibility in living cells establishes PNCL a useful and easy-to-use tool for studying ONOO− in chemical and biological systems.
Conflicts of interest
A. R. L. discloses a financial stake in BioLum Science, LLC, a company developing asthma monitoring technology.
Acknowledgements
Research reported in this publication was supported by the National Institute Of General Medical Sciences of the National Institutes of Health under award number R15GM114792. The content is solely the responsibility of the authors and does not necessarily represent the official views of the National Institutes of Health. Additional support was provided by Southern Methodist University (start-up funds to A. R. L and Engaged Learning funds to A. G. R.). We acknowledge Manasa Subbarao for assistance with cell culture and Sara Lange for assistance with preliminary experiments. We thank Maciej Kukula (UT Arlington), Hamilton Lee (UT Dallas), and Prof. Jeremiah Gassensmith (UT Dallas) for assistance with mass spectrometry. Human lung adenocarcinoma epithelial cell (A549) were purchased from ATCC and cultured in F-12K media supplemented with 10% Fetal Bovine Serum (FBS) and 1% antibiotics (penicillin/streptomycin, 100 U mL−1).
Notes and references
- J. S. Beckman, T. W. Beckman, J. Chen, P. A. Marshall and B. A. Freeman, Proc. Natl. Acad. Sci. U. S. A., 1990, 87, 1620 CrossRef CAS.
-
(a) A. J. Gow, D. Duran, S. Malcolm and H. Ischiropoulos, FEBS Lett., 1996, 385, 63 CrossRef CAS PubMed;
(b) G. L. Squadrito and W. A. Pryor, Free Radical Biol. Med., 1998, 25, 392 CrossRef CAS;
(c) C. Szabó, H. Ischiropoulos and R. Radi, Nat. Rev. Drug Discovery, 2007, 6, 662 CrossRef PubMed;
(d) P. Pacher, J. S. Beckman and L. Liaudet, Physiol. Rev., 2007, 87, 315 CrossRef CAS PubMed.
- P. Liu, B. H. Xu, J. Quilley and P. Y. K. Wong, Am. J. Physiol.: Cell Physiol., 2000, 279, 1970 CrossRef PubMed.
- W. Kossenjans, A. Eis, R. Sahay, D. Brockman and L. Myatt, Am. J. Physiol.: Heart Circ. Physiol., 2000, 278, 1311 CrossRef PubMed.
- P. B. Massion, O. Feron, C. Dessy and J. L. Balligand, Circ. Res., 2003, 93, 388 CrossRef CAS PubMed.
- H. Wiseman and B. Halliwell, Biochem. J., 1996, 313, 17 CrossRef CAS PubMed.
- R. Ahmad, Z. Rasheed and H. Ahsan, Immunopharmacol. Immunotoxicol., 2009, 31, 388 CrossRef CAS PubMed.
-
(a) J. S. Beckman, M. Carson, C. D. Smith and W. H. Koppenol, Nature, 1993, 364, 584 CrossRef CAS PubMed;
(b) M. A. Smith, P. L. R. Harris, L. M. Sayre, J. S. Beckman and G. Perry, J. Neurosci., 1997, 17, 2653 CAS;
(c) N. A. Simonian and J. T. Coyle, Annu. Rev. Pharmacol. Toxicol., 1996, 36, 83 CrossRef CAS PubMed.
- C. Brito, M. Naviliat, A. C. Tiscornia, F. Vuillier, G. Gualco, G. Dighiero, R. Radi and A. M. Cayota, J. Immunol., 1999, 162, 3356–3366 CAS.
- S. Altug, A. T. Demiryürek, K. A. Kane and I. Kanzik, Br. J. Pharmacol., 2000, 130, 125 CrossRef CAS PubMed.
- M. N. Alvarez, G. Peluffo, L. Piacenza and R. Radi, J. Biol. Chem., 2011, 286, 6627 CrossRef CAS PubMed.
- A. T. Jacobs and L. J. Ignarro, J. Biol. Chem., 2001, 276, 47950 CrossRef CAS PubMed.
- W. H. Koppenol, J. J. Moreno, W. A. Pryor, H. Ischiropoulos and J. S. Beckman, Chem. Res. Toxicol., 1992, 5, 834 CrossRef CAS PubMed.
- S. V. Lymar and J. K. Hurst, J. Am. Chem. Soc., 1995, 117, 8867 CrossRef CAS.
- G. Merényi, J. Lind and S. Goldstein, J. Am. Chem. Soc., 2002, 124, 40 CrossRef.
- G. Ferrer-Sueta and R. Radi, ACS Chem. Biol., 2009, 4, 161 CrossRef CAS PubMed.
- C. E. Donald, M. N. Hughes, J. M. Thompson and F. T. Bonner, Inorg. Chem., 1986, 25, 2676 CrossRef CAS.
-
(a) K. M. Miranda, A. S. Dutton, L. A. Ridnour, C. A. Foreman, E. Ford, N. Paolocci, T. Katori, C. G. Tocchetti, D. Mancardi, D. D. Thomas, M. G. Espey, K. N. Houk, J. M. Fukuto and D. A. Wink, J. Am. Chem. Soc., 2005, 127, 722 CrossRef CAS PubMed;
(b) J. H. Jorolan, L. A. Buttitta, C. Cheah and K. M. Miranda, Nitric Oxide, 2015, 44, 39 CrossRef CAS PubMed.
- R. Smulik, D. Debski, J. Zielonka, B. Michalowski, J. Adamus, A. Marcinek, B. Kalyanaraman and A. Sikora, J. Biol. Chem., 2014, 289, 35570–35581 CrossRef CAS PubMed.
-
(a) H. Ischiropoulos, Arch. Biochem. Biophys., 1998, 356, 1 CrossRef CAS PubMed;
(b) R. Radi, Proc. Natl. Acad. Sci. U. S. A., 2004, 101, 4003 CrossRef CAS PubMed.
-
(a) D. Yang, H. Wang, Z. Sun, N. Chung and J. Shen, J. Am. Chem. Soc., 2006, 128, 6004 CrossRef CAS PubMed;
(b) Z. Sun, H. Wang, F. Liu, Y. Chen, P. K. H. Tam and D. Yang, Org. Lett., 2009, 11, 1887 CrossRef CAS PubMed.
-
(a) T. Peng, X. Chen, L. Gao, T. Zhang, W. Wang, J. Shen and D. Yang, Chem. Sci., 2016, 7, 5407 RSC;
(b) J. Li, C. S. Lim, G. Kim, H. M. Kim and J. Yoon, Anal. Chem., 2017, 89, 8496 CrossRef CAS PubMed.
- X. Sun, Q. Xu, G. Kim, S. E. Flower, J. P. Lowe, J. Yoon, J. S. Fossey, X. Qian, S. D. Bull and T. D. James, Chem. Sci., 2014, 5, 3368 RSC.
- F. Yu, P. Li, G. Li, G. Zhao, T. Chu and K. Han, J. Am. Chem. Soc., 2011, 133, 11030 CrossRef CAS PubMed.
- F. Yu, P. Li, B. Wang and K. Han, J. Am. Chem. Soc., 2013, 135, 7674 CrossRef CAS PubMed.
- Z. Lou, P. Li and K. Han, Acc. Chem. Res., 2015, 48, 1358–1368 CrossRef CAS PubMed.
- A. R. Lippert, ACS Cent. Sci., 2017, 3, 269 CrossRef CAS PubMed.
- W. Zhou, Y. Cao, D. Sui and C. Lu, Anal. Chem., 2016, 88, 2659–2665 CrossRef CAS PubMed.
- W. Zhou, S. Dong, Y. Lin and C. Lu, Chem. Commun., 2017, 53, 2122–2125 RSC.
- T. S. Bailey and M. D. Pluth, Methods Enzymol., 2015, 554, 81 CAS.
- L. Liu and R. P. Mason, PLoS One, 2010, 5, e12024 Search PubMed.
- J. Cao, R. Lopez, J. M. Thacker, J. Y. Moon, C. Jiang, S. N. S. Morris, J. H. Bauer, P. Tao, R. P. Mason and A. R. Lippert, Chem. Sci., 2015, 6, 1979 RSC.
- J. Cao, J. Campbell, L. Liu, R. P. Mason and A. R. Lippert, Anal. Chem., 2016, 88, 4995 CrossRef CAS PubMed.
-
(a) O. Green, S. Gnaim, R. Blau, A. Eldar-Boock, R. Satchi-Fainaro and D. Shabat, J. Am. Chem. Soc., 2017, 139, 13243 CrossRef CAS PubMed;
(b) N. Hananya, A. E. Boock, C. R. Bauer, R. Satchi-Fainaro and D. Shabat, J. Am. Chem. Soc., 2016, 138, 13438 CrossRef CAS PubMed.
- A. P. Schaap, T. S. Chen, R. S. Handley, R. DeSilva and B. P. Giri, Tetrahedron Lett., 1987, 28, 1155 CrossRef CAS.
- O. Green, T. Eilon, N. Hananya, S. Gutkin, C. R. Bauer and D. Shabat, ACS Cent. Sci., 2017, 3, 349 CrossRef CAS PubMed.
- J. L. Kroll, C. A. Werchan, A. G. Reeves, K. J. Bruemmer, A. R. Lippert and T. Ritz, Physiol. Behav., 2017, 179, 99 CrossRef CAS PubMed.
- A. G. Reeves, M. Subbarao and A. R. Lippert, Anal. Methods, 2017, 9, 3418 RSC.
- M. E. Quimbar, K. M. Krenek and A. R. Lippert, Methods, 2016, 109, 123 CrossRef CAS PubMed.
- K. J. Bruemmer, S. Merrikhihaghi, C. T. Lollar, S. N. S. Morris, J. H. Bauer and A. R. Lippert, Chem. Commun., 2014, 50, 12311 RSC.
- T. Ueda, H. Konishi and K. Manabe, Angew. Chem., Int. Ed., 2013, 52, 8611 CrossRef CAS PubMed.
- J. M. Fukuto, M. D. Bartberger, A. S. Dutton, N. Paolocci, D. A. Wink and K. N. Houk, Chem. Res. Toxicol., 2005, 18, 790 CrossRef CAS PubMed.
-
(a) K. Kawai, N. Ieda, K. Aizawa, T. Suzuki, N. Miyata and H. Nakagawa, J. Am. Chem. Soc., 2013, 135, 12690 CrossRef CAS PubMed;
(b) X. Jing, F. Yu and L. Chen, Chem. Commun., 2014, 50, 14253 RSC;
(c) C. Liu, H. Wu, Z. Wang, C. Shao, B. Zhu and X. Zhang, Chem. Commun., 2014, 50, 6013 RSC;
(d) K. Zheng, W. Lin, D. Cheng, H. Chen, Y. Liu and K. Liu, Chem. Commun., 2015, 51, 5754 RSC;
(e) M. Ren, B. Deng, K. Zhou, J. Wang, X. Kong and W. Lin, J. Mater. Chem. B, 2017, 5, 1954 RSC.
- J. A. Reisz, C. N. Zink and S. B. King, J. Am. Chem. Soc., 2011, 133, 11675 CrossRef CAS PubMed.
Footnote |
† Electronic supplementary information (ESI) available: Additional experimental details, supplementary figures, and scanned spectra. See DOI: 10.1039/c7sc05087a |
|
This journal is © The Royal Society of Chemistry 2018 |