The critical role of light in moderating microbial stress due to mixtures of engineered nanomaterials†
Received
12th June 2017
, Accepted 10th November 2017
First published on 10th November 2017
Abstract
Exposure to light is a key parameter that influences the chemical interactions and microbial stress of engineered nanomaterials (ENMs). Yet, the photochemistry and phototoxic stress responses of many ENMs and ENM mixtures have not been adequately detailed. We exposed E. coli bacteria to binary combinations of n-TiO2 (a stable metal oxide) with different plasmonic metal ENMs (n-Ag, n-Au, or n-Pt) in a natural aqueous medium under light and dark conditions. Using ATP level and cell membrane integrity probes, we measure the toxic stress due to these ENM mixtures. We previously found that under dark conditions, n-TiO2 attenuates the toxic stress due to low concentrations of n-Ag (<20 μg L−1) via adsorption of Ag+. However, mixtures containing n-Au or n-Pt produce no difference in toxic stress responses with and without n-TiO2 in the dark. In contrast, under simulated solar irradiation, we observed that n-Ag, n-Au, and n-Pt each with n-TiO2 cause synergistic toxic stress. The n-Ag/n-TiO2 combination causes the greatest phototoxicity, followed by n-Au/n-TiO2 and then n-Pt/n-TiO2. Measurements of photocatalytic production of reactive oxygen species reveal that irradiation of n-Ag or n-Au with n-TiO2 yields synergistic production of superoxide anion and hydrogen peroxide. The photochemical interactions of these ENMs, governed by metal ENM solubility and localized surface plasmon resonance, provide mechanistic insight into the amplified photoactivity of mixtures.
Environmental significance
Toxic stresses due to mixtures of engineered nanomaterials (ENMs) can differ greatly from those of individual ENMs due to chemical interactions, particularly under light. Yet, the photochemistry and phototoxicity of many ENMs and ENM mixtures have not been adequately detailed. We observe varying levels of synergistic toxic stress to E. coli from mixtures of metal nanoparticles (n-Ag, n-Au, and n-Pt) with nanotitania (n-TiO2) under light, dependent on metal nanoparticle solubility and photoactivation. This report is environmentally significant because we probe the chemical and biological interactions of ENMs at low concentrations and as part of mixtures. We provide more evidence that mixtures of ENMs act as emergent systems with chemistry and toxicity not predicted by that of the individual ENMs.
|
Introduction
The increased industrial and commercial use of engineered nanomaterials (ENMs) leads to their unintended release into environmental systems and the subsequent creation of complex mixtures. However, the chemical interactions between various ENMs and resulting effects on microorganisms have scarcely been investigated.1 Furthermore, the influence of light on these chemical and biological interactions has largely been neglected. In previous work we found that ENM mixtures often produce drastically different stress responses in exposed microorganisms than individual ENMs.2,3 These responses can be additive, attenuated, antagonistic, or synergistic depending on environmental conditions, particularly the presence of light.
We previously investigated the chemical interactions and combined toxic stress due to n-TiO2 and n-Ag, two highly used ENMs with different photo-chemical properties. n-TiO2 is a stable photoactive semiconductor while n-Ag, which is both a plasmonic and soluble ENM, is used primarily for its antimicrobial properties.4 Under dark conditions, we observed that n-TiO2 can control the toxicity of the mixture to Escherichia coli.3 The adsorption of highly toxic Ag+ ions onto n-TiO2 mitigated the stress effects of n-Ag, but only at low concentrations of n-Ag, below 20 μg L −1.3 In contrast, under simulated solar irradiation, measurements of bacterial ATP levels and cell membrane integrity revealed that the mixture causes synergistic toxic stress relative to the individual ENMs.5
We proposed that the dissolution of n-Ag and photoreduction of Ag+ on the n-TiO2 surface creates a self-assembled n-Ag/n-TiO2 composite with enhanced photoactivity and production of reactive oxygen species (ROS). Nanoscale Ag clusters are plasmonic and can be excited by visible light. As part of a composite with n-TiO2, they can contribute to enhanced photoactivity by acting as a photosensitizer to extend the photoresponse,6,7 producing ROS by activation of surface plasmons,8 and hindering recombination.9 We showed evidence of Ag clusters associated with n-TiO2 by scanning transmission electron microscopy (STEM), and suggested that these nanocomposites explain the enhanced toxic stress and correlated increase in hydrogen peroxide, arising from sensitization.5 In contrast to our results under dark conditions, this work revealed the role of light in controlling the chemical interactions between different types of ENMs and their resulting toxic effects on microorganisms. In this paper, we investigate whether the enhancement we observed with n-Ag and n-TiO2 extends to other commonly used plasmonic metal ENMs, including n-Au and n-Pt that vary in their solubility and photoactivity.
The effects of metal ENMs on microorganisms are predominantly investigated under dark conditions, except for studies using algae,10–12 even though many have photoactivity arising from surface plasmons, i.e., delocalized electrons undergoing oscillations as a result of an excitation by electromagnetic radiation.13,14 The localized surface plasmon resonance (LSPR) wavelength, where these particles exhibit the strongest light absorption, is a function of the dielectric constant of the medium containing the ENMs and the size and shape of the nanoparticles.15 For spherical nanoparticles, the optical maximum absorption due to LSPR of n-Au, n-Ag, and n-Pt are located in the visible region,16,17 near UV,16,18 and middle UV,19 respectively. Under irradiation at or below the LSPR wavelength, conduction electrons on the surface of the metal nanoparticles are excited; these “hot electrons” can take part in chemical reactions and have been shown to contribute to the production of reactive oxygen species (ROS).8 For example, n-Au was shown to produce superoxide anion under ultraviolet irradiation,20 and Choi, et al., observed the production of hydroxyl radical by n-Ag under sunlight.21
In contrast to metal ENMs, n-TiO2’s photoactivity stems from ultra bandgap excitation under ultraviolet light of wavelengths typically less than 385 nm, where an electron from the valence band is promoted to the conduction band, creating an electron–hole pair.22 These electrons and holes can react with di-oxygen or water to form a suite of ROS including singlet oxygen (1O2), superoxide anion (O2˙−), hydrogen peroxide (H2O2), and hydroxyl radical (˙OH).23 n-TiO2's photoactivity is primarily responsible for its toxic effects to microorganisms,24,25 specifically its formation of ROS that can oxidize organic compounds including components of the bacterial cell.26 In the field of materials design, strategies to enhance the photoactivity of n-TiO2 have included doping the lattice with metal ions27,28 or adding surficial metallic clusters to n-TiO2.29,30 As depicted in Fig. 1, metal clusters can modify the photoactivity of n-TiO2 by (A) sensitization that extends the photoresponse into the visible region31 and/or (B) serving as electron sinks to prevent recombination of electron–hole pairs by the storage of electrons on metal clusters.32 The interactions between metal ENMs and n-TiO2 are likely governed by their photoactivity and their solubility, which may determine the likelihood of forming a nanocomposite with n-TiO2. While n-Ag is soluble in oxygenated solutions,33 n-Au and n-Pt are generally stable against dissolution except in acidic media.34,35
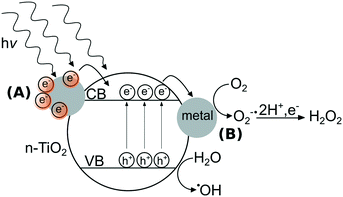 |
| Fig. 1 Schematic of enhancements to photoactivity of n-TiO2 by metallic surface clusters through (A) sensitization that extends the photoresponse into the visible light region and (B) separation of electron–hole pairs by storage of electrons on metal clusters. | |
In this work, we probe the photochemistry and bacterial stress caused by mixtures of plasmonic metal ENMs and n-TiO2. We propose that light controls the chemistry of ENM mixtures, and that the chemical interactions produce enhanced photoactivity and toxic effects to microorganisms. Using binary systems of metal ENMs (n-Ag, n-Au, and n-Pt) with n-TiO2, we determine the effects of exposure on bacterial ATP levels and cell membrane integrity and survey their production of ROS to probe how chemical interactions and photochemistry change with respect to individual ENMs.
Experimental
ENM characterization
Citrate-stabilized n-Ag was synthesized as discussed in our previous work.3 Briefly, n-Ag was synthesized by chemical reduction and stored under dark conditions inside an anaerobic chamber (Coy Labs) to prevent oxidative dissolution. The diameter of n-Ag was found to be 7.9 ± 1.5 nm. Detailed characterization of n-Ag is also presented in our previous work.3 Citrate-stabilized n-Au and citrate-stabilized n-Pt were purchased from nanoComposix (San Diego) and stored in the dark at 4 °C. n-Au and n-Pt nanoparticles were characterized by scanning transmission electron microscopy (STEM), UV–vis spectrophotometry, and X-ray diffraction (XRD) (Fig. S1 in ESI†). STEM images of n-Au and n-Pt captured using a Hitachi HD2300A are presented in Fig. S1A and B.† The diameters of the nanoparticles were about 7.9 ± 0.8 nm for n-Au and 30.8 ± 2.1 nm for n-Pt, based on particle-size analysis using ImageJ software36 for at least 100 particles from several micrographs for each ENM (Fig. S1C and D†). UV–vis extinction spectra (Shimadzu UV-2450) show the characteristic LSPR peak for n-Au at 520 nm, while the n-Pt spectrum shows an absorbance maximum near 240 nm (Fig. S1E and F†). Grazing-incidence X-ray diffraction (Rigaku ATXG) of these materials show that they both have face-centered cubic crystalline structures (Fig. S1G and H†).
Degussa P25 n-TiO2 was donated by Evonik Industries (Germany). P25 is a mixed phase n-TiO2 composed of 84% anatase and 16% rutile.2 The nominal size of P25 is approximately 20 nm and particles are spherical. Detailed characterization of P25 n-TiO2 is given in previous reports.25,37 Prior to use in experiments, a ∼1 g L−1 suspension of P25 was sonicated in an ultrasonic bath for 20 min (Health-Sonics, 110 W, 42 kHz) for preparation of ENM mixtures.
Bacteria preparation
Bacteria preparation was performed as described previously.3E. coli ATCC 25922 (American Type Culture Collection) was cultured in low-salt lysogeny broth (Alfa Aesar) until the optical density at 600 nm reached a value of ∼0.4. The cells were washed twice with the exposure medium, Lake Michigan water (LMW), before dilution to an optical density of ∼0.07. This OD corresponds to 107 cells per mL as measured by plate counting. LMW was used because of its environmental relevance as a complex medium that is representative of oligotrophic surface waters. LMW was collected at the Evanston Drinking Water Treatment plant. Prior to use, LMW was stored at 4 °C and was filtered to remove small particles and bacteria using a 0.2 μm pore size filter (Nalgene, PES membrane). Chemical characterization of LMW is presented in Table S1 of the ESI.†
Bacterial ATP response
Changes in bacterial ATP levels in response to ENM exposure were measured using the BacTiter-Glo assay (Promega). ENM mixtures were prepared immediately prior to the exposure and 10 μL of ENMs and 90 μL of bacteria suspension were added to a 96-well microplate. The concentrations of ENMs in these tests were 0–30 μg L−1 metal ENMs with 0, 1, or 2 mg L−1 P25. Under dark conditions, exposures were 1 hour long, so that these results can be compared to those previously attained for n-Ag with n-TiO2.3 Each test (n = 3) was done in triplicate and a representative plot is shown. For light exposure tests, simulated solar irradiation (SSI) was provided by a Xe arc lamp operating at 999 W (Newport Oriel) with the lamp output attenuated by a water-filled chamber to reduce heating due to infrared light. The spectrum of the lamp output is presented in Fig. S2 in the ESI.† Under SSI, the exposure time was set at 15 minutes to allow observation of the effects at these ENM concentrations. After the exposure, the BacTiter-Glo reagent was added to the microplate, mixed, and incubated for 5 min. Luminescence resulting from the assay was measured using a microplate reader (BioTek Synergy MX). For both light and dark exposure tests, microplates were incubated on an orbital shaker rotating at 200 rpm. A minimum of 7 tests from at least 3 replicate plates were averaged to generate means with their associated error bars (standard deviations) and p-values were calculated using the Student's t-test. All data processing and the calculation of p-values were performed in Microsoft Excel. The potential interference of the assay with ENMs was tested and no interference was found (Fig. S3A in the ESI†).
Cell membrane integrity
Cell membrane integrity was determined using the BacLight Live/Dead assay (ThermoFisher). Again, ENM mixtures were prepared immediately prior to exposure, and here 20 μL of ENMs and 180 μL of bacteria suspension were added to the microplate. The concentrations of ENMs, agitation on orbital shaker, and SSI conditions were the same as those of the ATP assay, and exposures under irradiation were 45 minutes, again, to allow observation of differences between experimental conditions. After the exposure, the BacLight reagent was added to the microplate, mixed, and incubated for 15 minutes before reading the green fluorescence due to Syto 9 (ex/em = 470/510) that binds to cell membranes and red due to propidium iodide (ex/em = 520/635) that binds to DNA. Green/red fluorescence ratios were normalized to a control (no ENM exposure) to allow for comparison of all conditions. Replicates and statistics were performed for the BacLight assay in the same manner as for the ATP assay. The potential interference of the assay with ENMs was tested and no interference was found (Fig. S3B in the ESI†).
Reactive oxygen species (ROS) detection
Hydrogen peroxide (H2O2) production was quantified using the ROS-Glo assay (Promega) in mixtures of 20 μg L−1 metal ENMs with or without 2 mg L−1 P25. 10 μL of ENM mixtures were incubated with 90 μL LMW under SSI for 15 min. After the exposure, the ROS-Glo H2O2 substrate was added to the microplate, mixed, and then incubated for 20 minutes. Next, the ROS-Glo detection reagent was mixed with the wells and incubated for another 15 minutes before reading the luminescence, which correlates to the formation of H2O2. Superoxide anion (O2˙−) production was measured using 2,3-bis(2-methoxy-4-nitro-5-sulfophenyl)-2H-tetrazolium-5-carboxanilide sodium salt (XTT sodium salt, Sigma-Aldrich) for mixtures at the same concentrations as the H2O2 measurements. 20 μL of 1 mM XTT sodium salt was mixed with 20 μL ENM mixtures and 160 μL LMW and exposed to SSI for 30 minutes. XTT reacts with O2˙− to form XTT formazan, an orange-colored product with absorbance at 410 nm. The absorbance was measured using the microplate reader. Hydroxyl radical (˙OH) was measured hydroxyphenyl fluorescein (HPF, Cayman chemical). 10 μL of ENM mixtures, 10 μL of 10 μM HPF were mixed with 80 μL of LMW and exposed to SSI for 30 min. A different ratio of ENMs (100 μg L−1 metal ENMs with 1 mg L−1 P25) was used for ˙OH because no significant differences between conditions were observed at the concentrations used for other ROS detection experiments.
Results and discussion
We first studied the response of E. coli to mixtures of plasmonic metal ENMs with P25 n-TiO2 under dark conditions. In previous studies, we found that ATP levels are a sensitive measure of stress in bacteria.2,3 As shown in Fig. 2, n-Au and n-Pt alone in the 0–30 μg L−1 concentration range cause little change in cellular ATP. Dose response curves for n-Au and n-Pt are given in the ESI† (Fig. S4) and show no decrease in ATP levels for exposures at less than 5 mg L−1 of either ENM. This contrasts starkly with n-Ag, for which we observed reduction of ATP starting at 10 μg L−1 and attenuation of the stress by n-TiO2 adsorption of Ag+ ions for concentrations up to 20 μg L−1 n-Ag.3 For n-Au and n-Pt, no significant change in bacterial levels occurs with the addition of 1 or 2 mg L−1 n-TiO2. These results are not surprising given the stability of n-Pt and n-Au at circumneutral pH34,35 and that other studies have reported toxic effects only at relatively high concentrations of n-Au and n-Pt, greater than 5 mg L−1.38,39
 |
| Fig. 2 Luminescence correlating to ATP levels of E. coli bacteria after exposure under dark conditions to mixtures of n-TiO2 with (A) n-Au or (B) n-Pt relative to control (no nanoparticle exposure). Each bar represents the average plus or minus the standard deviation for n = 3 wells. Statistical significance was calculated for all conditions relative to control and relative to same concentration of metal ENM, and no statistically significant differences were found (p < 0.05). | |
As depicted in Fig. 3, under SSI n-Au and n-Pt alone at low concentrations do not reduce bacterial ATP levels. However, as part of a mixture with n-TiO2, we observe a significant enhancement in the toxic stress relative to the individual ENMs. This enhancement is statistically significant compared to 1 and 2 mg L−1 P25 alone for 10–30 μg L−1 for n-Au and at 30 μg L−1 for n-Pt, although, we also observed significant enhanced stress at 2 mg L−1 TiO2 and 10–20 μg L−1 n-Pt. The bacterial response to n-Au and n-Pt with n-TiO2 parallels what we observed with n-Ag: that under irradiation, exposure to mixtures of photoactive ENMs in an environmental medium can produce synergistic toxic effects.
 |
| Fig. 3 ATP levels of E. coli bacteria after exposure under simulated solar irradiation to mixtures of n-TiO2 with (A) n-Au or (B) n-Pt relative to control (no exposure to nanoparticles). Each bar represents the average plus or minus the standard deviation for n ≥ 7 wells distributed over 3 microtiter plates. Statistically significant differences (p < 0.05) between conditions and control are denoted by black asterisks. Statistically significant differences (p < 0.05) between conditions and same concentration of P25 are denoted by red asterisks. | |
Fig. 4 shows the effects of 20 μg L−1 n-Ag, n-Au, and n-Pt individually and as mixtures with n-TiO2 under SSI on cell membrane integrity. Of these plasmonic ENMs, the greatest enhancement in phototoxic stress occurs with n-Ag and n-TiO2, as illustrated by the drastic decrease in the green/red fluorescence ratio. 20 μg L−1 n-Au with n-TiO2 also causes a significant decrease in cell membrane integrity as compared with 2 or 3 mg L−1 P25 alone. The decrease observed for n-Pt with 3 mg L−1 n-TiO2 is statistically significant as compared to P25 alone, however the cell membrane integrity observed for n-Pt with 2 mg L−1 n-TiO2 is not. As cell membrane integrity damage under SSI can be attributed to the production ROS,25 these measurements reveal that the photoactivity enhancements for mixtures with n-TiO2 are greatest with n-Ag, followed by n-Au and then n-Pt. The variation in strength of synergistic enhancements with plasmonic ENMs likely results from differences in their photoactivity and interaction with n-TiO2.
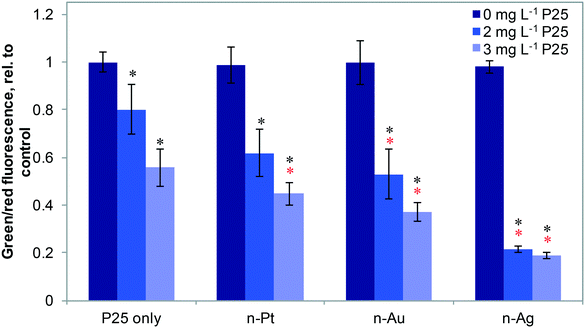 |
| Fig. 4 Cell membrane integrity for E. coli bacteria exposed to ENM mixtures under simulated solar irradiation relative to control (no nanoparticles), for P25 n-TiO2 alone and P25 with 20 μg L−1 n-Pt, n-Au or n-Ag. Each bar represents the average plus or minus the standard deviation for n ≥ 7 wells distributed over 3 microtiter plates. Statistically significant differences (p < 0.05) between conditions and control are denoted by black asterisks. Statistically significant differences (p < 0.05) between conditions and same concentration of P25 are denoted by red asterisks. | |
To probe the photoactivity enhancement occurring in mixtures of metal ENMs and n-TiO2, we measured the production of ROS, illustrated in Fig. 5. For individual metal ENMs, we observed no difference in ROS production as compared to the control. However, for n-Ag and n-Au with n-TiO2, we observed a large increase in luminescence due to H2O2 production, but no increase is observed with n-Pt (Fig. 5A). Similar results were obtained for O2˙− (Fig. 5B), which is a H2O2 precursor. We also measured hydroxyl radical (˙OH) production by ENM mixtures (Fig. S5 in the ESI†). Interestingly, we measured a significant decrease in the ˙OH production for n-Ag with n-TiO2 relative to n-TiO2 alone, indicating that n-Ag may scavenge ˙OH. The results for H2O2 and O2˙− correlate well with our exposure test findings. The metal ENMs alone under SSI do not produce significant toxic stress responses or ROS differing from the control. However, with n-TiO2, n-Ag and n-Au greatly enhance the phototoxic stress triggered by increased ROS production.
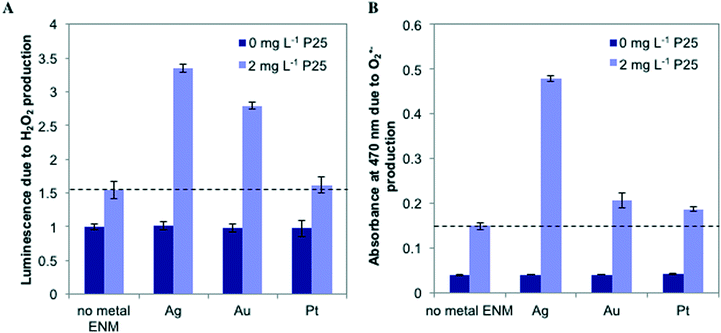 |
| Fig. 5 Measurements of (A) luminescence due to production of H2O2, normalized to control (no nanoparticles) and (B) absorbance at 470 nm due to superoxide anion production for mixtures of 20 μg L−1 metal ENMs (n-Ag, n-Au, and n-Pt) with and without 2 mg L−1 P25. Each bar represents average plus or minus the standard deviation for n = 3 wells. The dashed line shows the effect on the probe molecule from P25 alone. | |
In our work with n-Ag and n-TiO2, we proposed and provided evidence that dissolution of n-Ag releases Ag+ ions that may be photoreduced back to metallic Ag clusters on the surface of n-TiO2,5 forming a nanocomposite that shows unique photochemistry, enhanced ROS production, and amplified bacterial toxic stress. A similar nanostructure may arise from other ENMs interacting with n-TiO2. Photo-generated holes and ROS produced by illuminating n-TiO2 can oxidize metallic Au.40,41 Subsequently, these Au ions can be photoreduced to produce metal clusters on the surface of n-TiO2.30,42 Hence, a photodriven Au redox cycle may promote the self-assembly of an n-Au/n-TiO2 composite that explains the synergistic biological stress we observe. The photoactivity of engineered Au/TiO2 nanocomposites have been attributed both to their ability to act as an electron sink and as a sensitizer. Kamat, et al. observed transfer of electrons from n-TiO2 to n-Au and used stored electrons to reduce other chemical species.43 They also showed that a 3-fold enhancement in photocurrent generation was possible for n-Au adsorbed to a n-TiO2 film.44 Tsukamoto, et al. reported that n-Au on the surface of n-TiO2 acted as a sensitizer enabling the composite to oxidize organic substrates under visible light only.45 Because n-Au is much less soluble than n-Ag, there is likely less n-TiO2 surface coverage with n-Au than would occur under the same conditions with n-Ag. This may explain why we observe lower photoactivity and phototoxicity enhancement for n-Au in a mixture with n-TiO2.
Of the three metal ENMs investigated here, we saw the least enhancement with n-Pt and detected only minor increases in O2˙− and ˙OH production relative to n-TiO2 alone. Unlike n-Au and n-Ag, which have LSPR wavelengths and strong light absorption in the visible region, n-Pt′s LSPR lies in the middle UV, in the same range where n-TiO2 ultra bandgap excitation occurs.46 Thus, n-Pt may not photosensitize n-TiO2 and does not extend its spectral range. Additionally, the chemical interaction of n-TiO2 with n-Pt differs from those of n-Ag and n-Au. Pt is more stable against dissolution and oxidation, making it unlikely that it undergoes redox cycling to form self-assembled nanocomposites with n-TiO2 as we proposed for n-Ag and n-Au. However, the n-Pt and n-TiO2 particles may heterocoagulate to form composites. The phototoxic stress enhancement we observe for n-Pt and n-TiO2 then likely results from n-Pt acting as an electron sink to retard charge recombination. While several groups have reported that n-Pt on the surface of n-TiO2 can prevent recombination;47,48 others have found that n-Pt reacts with H2O2 and superoxide anion, as well as other ROS.49–51 Here, n-Pt′s hindering of charge recombination may outweigh its scavenging of ROS to produce the enhanced photoactivity we observe with n-TiO2 in Fig. 3 and 4.
In this work, we have shown that the synergistic photoactivity and bacterial stress observed for n-Ag and n-TiO2 can be generalized to mixtures with other plasmonic metal ENMs. We demonstrate a strong correlation between the stress levels caused by ENM mixtures under light and their ROS production. Solubility and LSPR wavelength of metal ENMs are key properties that explain the photoactivity and phototoxicity observed as part of the mixture with n-TiO2. Overall, this work provides more evidence that mixtures of metal ENMs with n-TiO2 in a complex environmental medium and under solar irradiation produce emergent systems. These interactions can produce new nanostructures with amplified toxicity greater than the sum of the individual ENMs' effects. Light plays a critical role in moderating both the chemistry and activity of ENMs in complex environmental mixtures and must be included in assessing their environmental fate and impacts.
Conflicts of interest
There are no conflicts to declare.
References
- P. A. Holden, J. L. Gardea-Torresdey, F. Klaessig, R. F. Turco, M. Mortimer, K. Hund-Rinke, E. A. Cohen Hubal, D. Avery, D. Barceló, R. Behra, Y. Cohen, L. Deydier-Stephan, P. L. Ferguson, T. F. Fernandes, B. Herr Harthorn, W. M. Henderson, R. A. Hoke, D. Hristozov, J. M. Johnston, A. B. Kane, L. Kapustka, A. A. Keller, H. S. Lenihan, W. Lovell, C. J. Murphy, R. M. Nisbet, E. J. Petersen, E. R. Salinas, M. Scheringer, M. Sharma, D. E. Speed, Y. Sultan, P. Westerhoff, J. C. White, M. R. Wiesner, E. M. Wong, B. Xing, M. Steele Horan, H. A. Godwin and A. E. Nel, Environ. Sci. Technol., 2016, 50, 6124–6145 CrossRef CAS PubMed.
- T. Tong, C. M. Wilke, J. Wu, C. T. T. Binh, J. J. Kelly, J.-F. Gaillard and K. A. Gray, Environ. Sci. Technol., 2015, 49, 8113–8123 CrossRef CAS PubMed.
- C. M. Wilke, T. Tong, J.-F. Gaillard and K. A. Gray, Environ. Sci. Technol., 2016, 50, 11302–11310 CrossRef CAS PubMed.
- C. Gunawan, W. Y. Teoh, C. P. Marquis, J. Lifia and R. Amal, Small, 2009, 5, 341–344 CrossRef CAS PubMed.
- C. M. Wilke, B. Wunderlich, J.-F. Gaillard and K. A. Gray, Environ. Sci. Technol., 2017 Search PubMed , in review.
- H. Zhang, G. Wang, D. Chen, X. Lv and J. Li, Chem. Mater., 2008, 20, 6543–6549 CrossRef CAS.
- K. Awazu, M. Fujimaki, C. Rockstuhl, J. Tominaga, H. Murakami, Y. Ohki, N. Yoshida and T. Watanabe, J. Am. Chem. Soc., 2008, 130, 1676–1680 CrossRef CAS PubMed.
- W. Zhang, Y. Li, J. Niu and Y. Chen, Langmuir, 2013, 29, 4647–4651 CrossRef CAS PubMed.
- A. Takai and P. V. Kamat, ACS Nano, 2011, 5, 7369–7376 CrossRef CAS PubMed.
- S. Leclerc and K. J. Wilkinson, Environ. Sci. Technol., 2014, 48, 358–364 CrossRef CAS PubMed.
- A. D. Burchardt, R. N. Carvalho, A. Valente, P. Nativo, D. Gilliland, C. P. Garcìa, R. Passarella, V. Pedroni, F. Rossi and T. Lettieri, Environ. Sci. Technol., 2012, 46, 11336–11344 CrossRef CAS PubMed.
- V. Aruoja, H.-C. Dubourguier, K. Kasemets and A. Kahru, Sci. Total Environ., 2009, 407, 1461–1468 CrossRef CAS PubMed.
- P. K. Jain, X. Huang, I. H. El-Sayed and M. A. El-Sayed, Plasmonics, 2007, 2, 107–118 CrossRef CAS.
- K. L. Kelly, E. Coronado, L. L. Zhao and G. C. Schatz, J. Phys. Chem. B, 2003, 107, 668–677 CrossRef CAS.
- C. Noguez, J. Phys. Chem. C, 2007, 111, 3806–3819 CAS.
- S. Link, Z. L. Wang and M. A. El-Sayed, J. Phys. Chem. B, 1999, 103, 3529–3533 CrossRef CAS.
- F. X. Zhang, L. Han, L. B. Israel, J. G. Daras, M. M. Maye, N. K. Ly and C.-J. Zhong, Analyst, 2002, 127, 462–465 RSC.
- Y. Sun and Y. Xia, Analyst, 2003, 128, 686–691 RSC.
- R. C. Johnson, J. Li, J. T. Hupp and G. C. Schatz, Chem. Phys. Lett., 2002, 356, 534–540 CrossRef CAS.
- M. Misawa and J. Takahashi, Nanomed. Nanotech. Biol. Med., 2011, 7, 604–614 CrossRef CAS PubMed.
- O. Choi and Z. Hu, Environ. Sci. Technol., 2008, 42, 4583–4588 CrossRef CAS PubMed.
- A. L. Linsebigler, G. Lu and J. T. Yates Jr, Chem. Rev., 1995, 95, 735–758 CrossRef CAS.
- K. Hashimoto, H. Irie and A. Fujishima, Jpn. J. Appl. Phys., 2005, 44, 8269 CrossRef CAS.
- C. T. T. Binh, T. Tong, J.-F. Gaillard, K. A. Gray and J. J. Kelly, Environ. Toxicol. Chem., 2014, 33, 317–327 CrossRef CAS PubMed.
- T. Tong, C. T. T. Binh, J. J. Kelly, J.-F. Gaillard and K. A. Gray, Water Res., 2013, 47, 2352–2362 CrossRef CAS PubMed.
- P.-C. Maness, S. Smolinski, D. M. Blake, Z. Huang, E. J. Wolfrum and W. A. Jacoby, Appl. Environ. Microbiol., 1999, 65, 4094–4098 CAS.
- S. Kim, S.-J. Hwang and W. Choi, J. Phys. Chem. B, 2005, 109, 24260 CrossRef CAS PubMed.
- C. Sahoo, A. K. Gupta and A. Pal, Dyes Pigm., 2005, 66, 189–196 CrossRef CAS.
- S. C. Chan and M. A. Barteau, Langmuir, 2005, 21, 5588–5595 CrossRef CAS PubMed.
- S. F. Chen, J. P. Li, K. Qian, W. P. Xu, Y. Lu, W. X. Huang and S. H. Yu, Nano Res., 2010, 3, 244–255 CrossRef CAS.
- Y. Tian and T. Tatsuma, J. Am. Chem. Soc., 2005, 127, 7632–7637 CrossRef CAS PubMed.
- F. Xiao, J. Phys. Chem. C, 2012, 116, 16487–16498 CAS.
- J. Liu and R. H. Hurt, Environ. Sci. Technol., 2010, 44, 2169–2175 CrossRef CAS PubMed.
- M.-C. Daniel and D. Astruc, Chem. Rev., 2004, 104, 293–346 CrossRef CAS PubMed.
- L. Tang, B. Han, K. Persson, C. Friesen, T. He, K. Sieradzki and G. Ceder, J. Am. Chem. Soc., 2010, 132, 596–600 CrossRef CAS PubMed.
-
W. S. Rasband, http://rsbweb/.nih.gov/ij/, 2008.
- T. Tong, K. Fang, S. A. Thomas, J. J. Kelly, K. A. Gray and J.-F. Gaillard, Environ. Sci. Technol., 2014, 48, 7924–7932 CrossRef CAS PubMed.
- Y. Zhao, Y. Tian, Y. Cui, W. Liu, W. Ma and X. Jiang, J. Am. Chem. Soc., 2010, 132, 12349–12356 CrossRef CAS PubMed.
- J. Gopal, N. Hasan, M. Manikandan and H.-F. Wu, Sci. Rep., 2013, 3, 1260 CrossRef PubMed.
- V. Subramanian, E. E. Wolf and P. V. Kamat, Langmuir, 2003, 19, 469–474 CrossRef CAS.
- V. Subramanian, E. Wolf and P. V. Kamat, J. Phys. Chem. B, 2001, 105, 11439–11446 CrossRef CAS.
- J. Lee, W. Choi and J. Yoon, Environ. Sci. Technol., 2005, 39, 6800–6807 CrossRef CAS PubMed.
- V. Subramanian, E. E. Wolf and P. V. Kamat, J. Am. Chem. Soc., 2004, 126, 4943–4950 CrossRef CAS PubMed.
- N. Chandrasekharan and P. V. Kamat, J. Phys. Chem. B, 2000, 104, 10851–10857 CrossRef CAS.
- D. Tsukamoto, Y. Shiraishi, Y. Sugano, S. Ichikawa, S. Tanaka and T. Hirai, J. Am. Chem. Soc., 2012, 134, 6309–6315 CrossRef CAS PubMed.
- J. M. Lin, H. Y. Lin, C. L. Cheng and Y. F. Chen, Nanotechnology, 2006, 17, 4391 CrossRef.
- W.-J. An, W.-N. Wang, B. Ramalingam, S. Mukherjee, B. Daubayev, S. Gangopadhyay and P. Biswas, Langmuir, 2012, 28, 7528–7534 CrossRef CAS PubMed.
- Y. Bai, W. Li, C. Liu, Z. Yang, X. Feng, X. Lu and K.-Y. Chan, J. Mater. Chem., 2009, 19, 7055–7061 RSC.
- M. Kajita, K. Hikosaka, M. Iitsuka, A. Kanayama, N. Toshima and Y. Miyamoto, Free Radical Res., 2007, 41, 615–626 CrossRef CAS PubMed.
- T. Hamasaki, T. Kashiwagi, T. Imada, N. Nakamichi, S. Aramaki, K. Toh, S. Morisawa, H. Shimakoshi, Y. Hisaeda and S. Shirahata, Langmuir, 2008, 24, 7354–7364 CrossRef CAS PubMed.
- A. Watanabe, M. Kajita, J. Kim, A. Kanayama, K. Takahashi, T. Mashino and Y. Miyamoto, Nanotechnology, 2009, 20, 455105 CrossRef PubMed.
Footnote |
† Electronic supplementary information (ESI) available. See DOI: 10.1039/c7en00527j |
|
This journal is © The Royal Society of Chemistry 2018 |