Effect of raw and purified carbon nanotubes and iron oxide nanoparticles on the growth of wheatgrass prepared from the cotyledons of common wheat (triticum aestivum)†
Received
27th July 2017
, Accepted 8th November 2017
First published on 9th November 2017
Abstract
The increase in global production of nanomaterials has raised concern as to their possible effects on plants that could ultimately affect the human population. The effects on the hydroponic growth of wheatgrass of four types of nanomaterials were studied: raw-single walled carbon nanotubes (SWCNTs), purified-SWCNTs, multi walled carbon nanotubes (MWCNTs), and iron oxide nanoparticles (n-FeOx) as a model of the catalyst residue typically present in CNTs. The germination rate (GR), mean germination time (MGT), shoot length, and a visual score of the plants' growth were determined for wheatgrass over the course of two weeks as a function of exposure to the nanomaterials dispersed in either water or THF (as well as appropriate controls). Raw-SWCNTs, MWCNTs, and n-FeOx show little impact suggesting that the catalyst residue (iron oxide) present in CNTs has little effect. Exposure to purified-SWCNTs dispersed in water shows increased GR (and shoot length), while wheatgrass exposed to purified-SWCNT dispersed in THF had retarded GR, suggesting that SWCNTs act as a carrier for adsorbed organic solvents whose effects are detrimental. A similar but lesser effect was observed for MWCNTs. Interestingly raw-SWCNTs showed no solvent effect, suggesting that the reduction of hydrophobicity of the SWCNTs through functionalisation enables the adsorption and subsequent release of harmful organic solvents. The positive effect of purified SWCNTs when dispersed in water is likely a function of their highly hydrophobic nature facilitating enhanced uptake of water.
Environmental significance
Increased production of carbon nanotubes (CNTs) will inevitably lead to greater environmental contamination. The lack of any natural degradation pathway and unique properties raises concern that once released they become a ubiquitous contaminant. The potential for being introduced into agricultural land raises the question as to the impact on the food chain in particular the impact of CNTs and their associated contaminants on plant growth. In this paper, the effect of raw and purified single walled carbon nanotubes (SWCNTs), multi walled carbon nanotubes, and iron oxide NPs (representing the residual catalyst material present in the majority of CNTs) on the germination and growth of wheatgrass were investigated. No significant alteration was observed, except surprisingly for purified SWCNTs where greater germination rate and growth, consistent with the hydrophobic nature providing a water reservoir for the seeds. In contrast, significant inhibition of growth is observed for purified SWCNTs in the presence of THF, suggesting that they act as a carrier for organic solvents that would otherwise be naturally diluted. This study raises a new concern for the environmental impact of carbon-based materials.
|
Introduction
Particles with sizes ranging between 10 nm to 1000 nm that are engineered using nanotechnology (the synthetic manipulation of matter at the nanometric scale), released inadvertently through human-made processes such as internal combustion,1 or produced through random natural processes or events such as wildfires, lightning, weathering or erosion, and microbial activity can be termed as nanoparticles (NPs). According to the National Nanotechnology Initiative, since the year 2000, inventions, discoveries, and markets for NPs has increased at an annual rate of 25%, with applications in drugs, cosmetics and fabrics, water filters, and in military weapons.2 In 2010 the value of nanotechnology-enabled products was $254 billion and it is estimated to increase to $3 trillion by 2020.3 Carbon-based nanomaterials are one of the major types of engineered materials, are currently being produced on an ever-expanding industrial scale (thousands of tonnes of CNTs are being produced annually since 2001 and such large-scale production has raised concerns related to their release into the environment in large quantities.4
While regulations pertaining to the safe usage and disposal of nano materials are still being defined,5 there is an increasing body of work on the biological and environmental impact of NPs.6–9 Of the myriad classes of NPs, carbon nanomaterials (both CNTs and fullerenes) have received special attention due to their unique physical and chemical properties.10 Reports covering cellular uptake, microorganism toxicity, transdermal transport, and pulmonary toxicity have been published;11–15 however, the potential impact on the plant growth has received relatively less attention. This is surprising since the resistance to natural degradation by CNTs coupled with their ready dispersion via winds,16 could result in their interaction with soil surfaces, where environmental transport studies have shown that accumulation in soil, rather than dispersion (due to weathering processes), is the likely fate.17 Aslani et al. have reviewed the effects of nanomaterials on plant growth,18 and the importance of understanding the relationships between nanomaterials and edible plants has been reviewed by Gardea-Torresdey and co-workers.19 The impact of multi walled carbon nanotubes (MWCNTs) on selected plant species was reported by Begum et al. They propose that the presence of nanomaterials can modify the surface chemistry of the roots.20,21 Additional studies have shown toxicity on rice cells, effects on root elongation of select crop species, and the ability of CNTs to penetrate seed coat and affect germination.22–25
Given the limited information on the phytotoxicity of carbon nanomaterials on plants we have undertaken the current study. While the effects of MWCNTs have been studied, we are interested in the comparison of MWCNTs with single walled carbon nanotubes (SWCNTs) due to the greater hydrophobic nature of the latter and their known adsorption of organic species.26
Environmental exposure of SWCNTs could be either in the raw form (as prepared in industry or a laboratory) or after some form of purification for subsequent processing. The difference between these two categories is important since the former contains significant residual quantities of the catalyst material (typically based on transition metals such as iron, cobalt, and molybdenum) used in their growth.27,28 This catalyst residue (e.g., iron oxide in the case of iron catalysed SWCNT growth) is known to adversely impact cell viability,29–31 and also inhibition of a number of chemical processes.32,33 Purification strategies typically help remove a considerable amount of catalyst residues, but depending on the procedure employed, could likely merely morph the metal oxides from one oxidation state to the other (e.g. Fe0 to Fe2+ to Fe3+).34–36 In order to account for the catalyst residue iron oxide nanoparticles (n-FeOx) were also investigated at comparable concentrations as present in the SWCNTs. The use of oleic acid surface stabilisation is analogous to adsorption of natural organic matter, which occurs in the environment.37
In addition to a reduction in catalytic impurities, purification of SWCNTs removes amorphous carbon residue. While it is conventional to view SWCNTs as idealized in graphical representations, in reality, oxygen functional groups such as hydroxides, carboxylic acids and epoxides are also present.38,39 Purification can either reduce or increase these species, which clearly can dramatically alter the chemical nature of the surface of the SWNT.38 We have recently reported that a combination of microwave irradiation and Cl2 treatment lowers both the metal content and side wall functionality.40
The inherent hydrophobic nature and high surface area of CNTs make them likely to interact with both organic and inorganic materials.41 As a consequence previous reports of carbon nanomaterials incorrectly ascribed observed toxicity to the carbon nanomaterial itself,42–44 when it was subsequently shown that the toxicity was due to the presence of solvent and the nano-material was acting as a concentrator and carrier.45 In this regard, it has been reported that MWCNTs and C60 impact the accumulation of pesticides in plants.41 In the present study THF was selected because of its ability to disperse CNTs,46–55 at least long enough as to enable transfer of the nanomaterials via pipetting to seed germination substrates immediately after sonication in the solvent. THF has been previously used in processing CNTs, for applications including the fabrication of sensors and devices.56,57 In addition, THF is known to contribute to inhibited bacterial growth.58 Potential health issues of THF/carbon nanomaterials have been suggested given the tumorigen and mutagen effects of THF.59 Oberdörster has shown that juvenile largemouth bass exposed to C60 dissolved in THF showed elevated lipid peroxidation products in the brain and a reduction in the total glutathione pool of the gills.60 Thus, THF can also potentially model the possible impact other chemical pollutants can have, in conjunction with nanomaterials, to provide useful related insights.
Wheatgrass was chosen as the experimental plant because of its widespread human consumption, the prior observation that Cu NPs show reduced root and seedling growth,61 and the increased interest in the phytotoxicity of NPs.62,63 In this study, the type and dosage of NPs on seed germination and plant growth in terms of initial seed mass gain, germination rate (GR), mean germination time (MGT), seedling height, and a visual score denoting seed and plant health.
Experimental methods
Chemicals and materials
Raw HiPCO single-walled carbon nanotubes (SWCNTs) were obtained from the Carbon Nanotube Laboratory at Rice University (Batches #195.7 and #188.4). Purification was carried out using a combination of microwave irradiation and chlorination treatments as described previously.40,64 Multi-walled carbon nanotubes (MWCNTs) were prepared using a table top horizontal tube reactor (Nanotech Innovations SSP-354) as previously reported.65 Iron oxide NPs (n-FeOx) were prepared using the thermal degradation method.66,67 THF was obtained from Sigma Aldrich and used without further purification. Distilled water (DI-water) was obtained in-house from existing laboratory facilities and used without further purification. Organic wheatgrass seeds were purchased from PowerGrow Systems.
NP characterisation
The nanomaterials used in the present study have been characterized using optical microscopy, scanning electron microscopy (SEM) and associated energy dispersive spectroscopy (EDS), transmission electron microscopy (TEM), thermogravimetric analysis/differential scanning calorimetry (TGA/DSC), Raman spectroscopy, small angle X-ray scattering (SAXS), and contact angle measurements. The data is consistent with that obtained previously for the same materials prepared by identical routes.40,64–66
Optical images were taken using an AmScope ME520TA Episcopic and diascopic trinocular metallurgical microscope equipped with an AmScope MA1000 10MP digital microscope camera. SEM was conducted using a FEI Quanta 400 equipped with an EDS detector. Images were acquired with a typical operating voltage of 30 kV or 15 kV, with a working distance of 10 mm, and spot size of 3. EDS spectra were performed and analysed using EDAX TEAM™ software. Samples were mounted with carbon tape onto aluminium microscopy specimen mounts (Electron Microscopy Sciences). TEM characterization was performed on either a JEOL 2100 field emission gun TEM at 100 kV or a JEOL 1230 high contrast TEM at 120 kV, both equipped with a CCD camera. Samples were prepared by drop drying a sample suspended in EtOH onto a 300 mesh gold grid with a lacy carbon film (Agar-Scientific, Ltd.). Simultaneous TGA/DSC experiments were performed on a TA instruments Q-600 using Ar or air as the carrier gas (Matheson Tri-Gas). For SWCNTs iron percentage and extent of functionalization was measured using ca. 10 mg of sample placed in a platinum pan and heated under ambient conditions up to 800 °C in dry air with a ramp rate was 5 °C min−1, and sampling interval of 3 s.33,67 Contact angle measurements using a Kruss DSA 25 EasyDrop instrument. Raman spectra of solid samples were measured in a Renishaw Raman microscope equipped with a 514 nm excitation laser. To maximize the Raman signal a continuous scan was carried out of the G peak (∼1600 cm−1), while the focus of the beam was altered to maximize the G peak intensity. When the maximal intensity was found, data was acquired using an integration of 10 accumulations at a power of 10%, with cosmic ray background removal applied. Each sample was probed a number of times at a variety of locations, to acquire data that represented the entirety of the sample. Raman data was acquired for a range of wavenumbers ranging from 100–3300 cm−1. Size determination of the FeOx NPs was achieved by SAXS using a Rigaku SmartLab X-Ray diffractometer using a Cu-Kα radiation source as previously reported.67 Samples were prepared by sealing a concentrated nanoparticle solution in hexanes into a 1 mm “Glass Number 50 Capillary” tube (Hampton Research Inc.) and the data was resolved using Rigaku's NANO-solver. Additional selected data are provided in the ESI.†
Plant growth
12 medium-sized (4′′ diameter) petri dishes were filled with cotton wool. Wheatgrass seeds were grown in the petri dishes pre-treated with solutions shown in Table 1, consistent with protocols defined by Yang and Watts.68 NPs were either dispersed in THF or water (10 mL). After dispersing the NPs in the appropriate solvent using an ultrasonic bath for 30 min, an aliquot (0.5 mL) of the each solution was placed directly onto 20 locations (20 replicates per solution type) on top of its respective petri dish. It should be noted that in the case of SWCNTs and MWCNTs that dispersion in both water and THF is temporary; however, the suspension is sufficiently stable to allow the delivery of an aliquot onto the cotton wool. The NPs were placed in a way such that they were approximately 1 cm apart from each other. The cotton in petri dishes exposed to solutions that were dispersed in THF was placed in a vacuum desiccator in order to evaporate the THF, while those with water solutions were allowed to air dry. After drying, wheatgrass seeds were placed directly on top of the treated spots in the cotton beds. Water (1 mL) was placed on each seed, and this was repeated each day for 8 days.
Table 1 Summary of samples composition
Sample |
Solvent (10 mL) |
Raw-SWCNTs (mg mL−1) |
Pure-SWCNTs (mg mL−1) |
MWCNTs (mg mL−1) |
n-FeOx (mg mL−1) |
1 |
H2O |
|
|
|
|
2 |
THF |
|
|
|
|
3 |
H2O |
0.5 |
|
|
|
4 |
THF |
0.5 |
|
|
|
5 |
H2O |
|
0.5 |
|
|
6 |
THF |
|
0.5 |
|
|
7 |
H2O |
|
|
0.5 |
|
8 |
THF |
|
|
0.5 |
|
9 |
H2O |
|
|
|
0.125 |
10 |
THF |
|
|
|
0.125 |
11 |
H2O |
|
|
|
0.5 |
12 |
THF |
|
|
|
0.5 |
Evaluation of seed germination and plant growth
The general evaluation protocols follow many of the approaches used by other researchers.69 Several metrics were used to determine the health of the seeds and plants germinated from the seeds in relation to the various treatment conditions studied. In each case these followed prior documented approaches. These were: germination rate,70–72 mean germination time (MGT),73–75 mass gain of the seeds over 4 days,23 seedling height after 8 days,76,77 and a semi-quantitative visual score.
The germination tests (with the exception of the semi-quantitative visual score) were performed according to International Seed Testing Association protocols (www.seedtest.org). The seedlings were considered as germinated if the radicle was at least 2 mm in length. Mean germination time and germination rate was calculated based on previous reports.78 Germination rate (GR) was determined using eqn (1), where a,b,c…n indicates the number of germinated seeds after 1,2,3…N days of planting. Mean germination time (MGT) was calculated using eqn (2), where X = number of days since planting the seeds and F = number of seeds newly germinated at X day.
| GR = (a/1) + (b − a/2) + (c − b/3) + … + (n−(n − 1))/N | (1) |
A maximum of 4 days were chosen for plant weight measurement as the seeds had to be individually lifted from their growth beds and weighed. Beyond 4 days, roots had formed and were embedding themselves into the cotton beds and this made it difficult to remove the seedlings without damaging them. The seedlings were monitored over a total duration of 8 days since planting, and seedling/plant heath was measured at the end of day 8 and tabulated for analysis. A semi-quantitative scale was developed and applied to record observations in plant growth that included scores for visual evaluation of root and shoot growth (Table 2). Such a scale would likely help capture any trends that are not easily observable through the quantitative metrics. This approach could especially be useful and convenient in scenarios where only bulk phenomena can be observed, and the number of individual phenomena related to specific biochemical and biological processes may be too many or too complex to be isolated and evaluated easily.
Table 2 Qualitative visual growth rating system
1 |
No change |
2 |
Very little to almost no root |
3 |
Short root but no shoot |
4 |
Medium length root but no shoot |
5 |
Shoot (<1 cm) |
6 |
Shoot (<3 cm) |
7 |
Shoot (<5 cm) |
8 |
Shoot (<7 cm) |
9 |
Shoot (<9 cm) |
10 |
Shoot (<11 cm) |
Results
Characterization of nanomaterials
The length and diameter of the raw HiPco SWCNTs is determined to be ca. 2–3.5 μm and 1–2 nm, respectively, as measured by SEM and TEM analysis (Fig. 1).79 The length and diameter are not altered upon purification. The Raman ID
:
IG ratio of raw and purified SWNTs is 0.08 and 0.03, respectively.33,64 As determined from TGA, the amount of residual catalyst is reduced from 30% wt% to about 1.8% wt% after purification, suggesting a reduction in the presence of non nanotube impurities in the latter.33,40,80 This is confirmed by EDX analysis which show a reduction in Fe At% from 4.4% to 1.2% after purification (Table S1, see ESI†).
 |
| Fig. 1 SEM (a) and TEM (b) images of raw HiPco SWCNTs. Scale bar = 10 μm and 50 nm, respectively. | |
The MWCNTs are 150–200 nm in diameter, are >20 μm in length as determined by SEM (Fig. 2). TGA shows ca. 10% catalyst residue, while the Raman ID
:
IG ratio is 0.50.65
 |
| Fig. 2 SEM image of as produced MWCNTs. Scale bar = 10 μm. | |
Iron oxide NPs (n-FeOx) have oleic acid functionality to provide stability and solubility. The size distribution is within the range of 5–20 nm, as determined by SAXS,66,67 which is consistent with the TEM measurements, and comparable to the range observed for catalyst residue in raw HiPco SWCNTs (3–13 nm).39 Thus, the n-FeOx are representative of the catalyst residue in the raw SWCNTs. Although the n-FeOx were 5–20 nm particles, when deposited from solution they form aggregates of 0.5–3 μm in diameter (Fig. 3).
 |
| Fig. 3 SEM image of n-FeOx aggregates formed by drying an aqueous dispersion. Scale bar = 10 μm. | |
Seed germination and plant growth
Samples of each NP were dispersed in either water or THF at a constant concentration (0.5 mg mL−1), except for one set of the iron oxide NPs (n-FeOx) samples, which used a concentration similar to that typically present in raw-SWCNTs (0.125 mg mL−1). Two “reference samples” were also chosen: water and evaporated THF. The former was to ensure that any densification (matting) or change in texture of the cotton wool upon addition of the NP dispersions could be taken into account. The THF sample was allowed to evaporate under vacuum in order to differentiate between any effects that were due to adsorption of THF onto the NP and onto cotton wool. The germination of the seeds and growth of the wheatgrass was recorded over 8 days and selected images are shown in Fig. 4 for samples after 1, 4 and 8 days.
 |
| Fig. 4 Photographic images of wheatgrass seeds and subsequent plants after 1, 4 and 8 days growth. The sample numbers correspond to those in Table 1. | |
The figures show germination of the seeds and growth of the seedlings under various conditions. Germination rate, MGT, seedling height, and numbers based on visual scores were easy to correlate with the visual data. On day 0, there is obviously no visible difference between the seeds, since they needed time to absorb water and initiate the germination and growth process. The main difference at this stage was the appearance of the nanomaterial deposits on the cotton beds on which the seeds were placed. On day 1, a few seeds in each dish began to exhibit the emergence of roots through the visible growth of appendages on one end. Variations within the same dish were likely due to the stochastic nature of the experiment wherein some seeds tend to germinate faster than the others, while others germinate later, and tend to “catch up” in terms of growth over several days. Day 2 showed the emergence of more appendages to the seeds, and the seeds that exhibited this phenomenon on day 1 showed a little more growth, eventually leading to the growth of the root. Emergence of the root leads to the subsequent emergence and growth of the shoot, or the upper portion of the plant. On day 4 (Fig. 4), one can see the emergence of plant shoots from the germinated seeds in most of the dishes. It is also evident that shoot growth was more in the case of some of the dishes than in the case of some of the other dishes. The trends seen on day 4 continued onto day 6, and the seedlings exhibited even further growth of shoots and roots. As can be seen from Fig. 4, there were tall shoots (4–11 cm) tall in all cases at day 8, and the lag exhibited previously continued to be exhibited on this day as well.
Initial mass gain in seeds leading up to germination
Fig. 5 shows average percentage mass gain of seeds exposed to the various treatment conditions in the dishes over 4 days. A comparison of the reference samples (water and evaporated THF) showed that there was a small apparent difference, where water-treated seeds gained more mass, although statistically the results were within standard deviation. This suggest that any significant differences were likely not due to adsorption of THF on the cotton wool. Seeds exposed to raw-SWCNTs dispersed in water showed a stagnation of the mass change at day 4 (Fig. 5a); however, this is not represented by the subsequent growth, see below. There appeared to be little or no change in mass increase (within standard deviation) beyond day 1 and up to day 4 for the n-FeOx samples irrespective of the solvent or concentration of n-FeOx used. MWCNTs exhibited comparable mass gains over the 4 day incubation period regardless of whether water or THF is being used as the solvent. In contrast, the results for purified SWCNTs were dramatically and statistically significantly different between water and THF suspensions. Seeds exposed to a THF suspension of pure-SWCNTs showed the lowest growth in mass compared to all the samples, but the analogous sample based on water as the solvent showed the highest mass increase.
 |
| Fig. 5 Plots of seed mass gain as a function of time (days) for (a) samples dispersed in water and (b) samples dispersed in THF. Error bars represent one standard deviation. | |
Germination rate and germination time (MGT)
The germination rate (GR) with standard deviations for the various treatment conditions is shown in Fig. 6. The GR for the water blank (0.37 day−1) and the THF reference (0.34 day−1) were similar, showing no residual effect of the THF after evaporation. There appeared to be little effect of the addition of the NPs in water; however, the solvent used to disperse the pure-SWCNTs had a significant effect with a 43% decrease in GR for the THF dispersed samples. The THF dispersed pure-SWCNTs shows the lowest average GR for all the samples. A similar trend is observed for the MWCNTs albeit at a lower level (26% decrease). A comparison between raw and pure SWCNT dispersed in water indicated an approximately 35% decrease in GR in the case of the former in relation to the latter, suggesting that in impurities in nanomaterials could adversely impact GR in a water-mediated interaction. However, pure SWCNT + THF caused a lower GR (∼28% lower) compared to raw SWCNT + THF, although within the bounds of standard deviation for both, hinting at a possible closer association between the solvent and pure SWCNT that could have likely caused extended interactions between the seeds and THF thereby yielding a lower GR. It should be noted that the presence and concentration of n-FeOx appeared to have no effect on GR, regardless of whether water or THF was being used as the solvent.
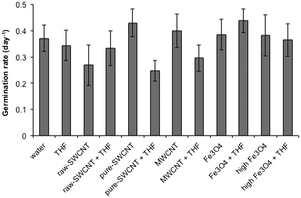 |
| Fig. 6 Plot of mean germination rate (GR) for samples studied. Error bars represent one standard deviation. | |
Data related to average mean germination time (MGT) for the various treatment conditions is as shown in Fig. 7; data for individual seeds are given in ESI.† A lot of variation was seen within each treatment condition (Fig. 7) likely due to the stochastic nature of seed germination rather than interactions with the NPs; however, it is worth noting that as with GR, the largest difference between samples dispersed in water and THF was for the pure-SWCNTs, suggesting that THF associated with pure SWCNTs likely delayed the germination process. GR and MGT are inversely related to each other.
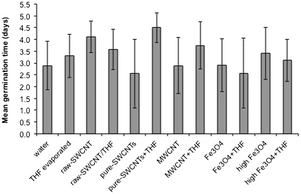 |
| Fig. 7 Plot of mean germination time (MGT) for samples studied. Error bars represent one standard deviation. | |
Seedling height
Fig. 8 shows the average plant shoot height data after 8 days for the various treatment conditions (data for individual seeds are given in ESI†). In the case of individual seed data, outliers were identified in terms of visibly significant difference from the mean, and from the observations we noted that the number of outliers in all petri dishes were similar regardless of whether they contained THF or not. This suggests that there is a certain amount of natural variation in the way the plants grew, and averaged data provides a more effective picture of the effects of NP and solvents on the growth of the seedlings.
 |
| Fig. 8 Plot of average plant height after 8 days for samples studied. Error bars represent one standard deviation. | |
The average data for seedling height over time shows trends similar to those observed in the case of germination rate. The addition of raw-SWCNTs, MWCNTs, or n-FeOx did not significantly impact the plant growth after 8 days. However, as with GR and MGR there was a significant disparity between results associated with water and THF dispersions for purified SWCNTs, representing a 45% decrease in plant height due to the presence of THF. It should also be noted that there appeared to be a 13% increase for pure-SWCNTs in water versus the water blank.
Visual growth scores
Fig. 9 shows the progression of visual growth scores of the seeds/seedlings over time for each treatment condition. The progression of growth for the two blanks (water and evaporated THF shown in Fig. 9a and b, respectively) shows only slight trends within experimental variation. The comparison of water versus THF for raw-SWCNTs (Fig. 9c and d), MWCNTs (Fig. 9g and h), and n-FeOx (Fig. 9i–l) show a similar lack of dependence on the solvent used. As with the other results this is not true for the purified SWCNTs (Fig. 9e and f). The presence of THF resulted in an inhibition in further growth after 2 days that was not observed in any of the other samples. This is clearly seen from the photographs of the petri dishes in Fig. 4.
 |
| Fig. 9 Plots ((a)–(l)) of composite visual growth scores (CVS) for samples studied. Error bars represent one standard deviation. | |
It is worth noting that after 8 days there was no significant effect of the presence of n-FeOx, MWCNTs, or raw-SWCNTs as compared to the water blank; however, there was a significant increase in growth for the pure-SWCNT sample. This was also observable in Fig. 4, where the pure-SWCNT/water sample had distinctly more growth than any other sample.
Discussion
This study evaluated the relative effects of various types/concentrations/purities of NPs, the solvents they were dispersed in (water or THF), and duration of their exposure to wheatgrass seeds. Based on a consideration of the numerous observations and data points throughout the study, a few trends became apparent.
Effect of carrier solvent: water versus THF
No residual effects outside of statistical variations were observed among most of the various samples. This suggests that any negative impacts of THF on seeds and seedlings were likely mitigated through evaporation and dilution through repeated addition of water to the growth petri dishes on a daily basis. Importantly, this also indicates that the cotton wool is not a factor in any observed solvent effects.
The most significant exception was the case involving pure SWCNTs dispersed in THF, wherein significant decreases in the various seed/seedling parameters were observed when compared to trends from pure SWCNTs dispersed in water. The trends observed in the case of this exception suggest that the any effects of THF (or potentially other organic solvents) are likely due to adsorption of the solvent on NP. The combined effect of THF and purified SWCNTs was mitigated over time suggesting that subsequent water addition to the cotton beds bearing the growing seedlings ensured that the THF dosage was likely eventually diluted, probably due to a weak association between solvent and the nanotubes.
It has been suggested that the extent of interaction between polar solvents and nanotube surfaces could be dependent on solvent molecular weight.81 Further research needs to be conducted in this regard extending to adsorptive effects of polar and non-polar solvents and selected organic species (e.g., humic acids) typically found in nature, of various molecular weights and surface functionalities, on the nanotube surfaces, and their related effects on seed germination and plant growth. The solvent (THF or water), and growth substrate (cotton) used in the current study was chosen such that the various seed germination and subsequent growth processes could be evaluated in a “clean” system wherein individual effects of nano materials and/or solvents can be more easily identified and analysed. Future studies would likely need to incorporate more complex seed/plant growth environments to more closely mimic environmental conditions.
Effect of catalyst residue
Several mechanisms for nanoparticle-induced cell and tissue injury have been proposed. The most developed hypothesis involves reactive oxygen species (ROS) generation.82 In the case of iron oxide NPs, ROS (in particular hydroxyl radicals) may be generated by acting as catalysts in Fenton-type reactions.83,84 Iron oxide NPs at the two chosen concentrations had the same effect as water in terms of the various parameters studied. The lower chosen concentration of n-FeOx was based on concentrations of iron catalyst particles typically observed in raw CNTs, and the related data in either water or THF as solvent suggest that there was likely no significant adverse affect of the n-FeOx on seed/seedling growth.85 It is interesting to note that Cu NPs have been reported to reduce root and seedling growth of wheat at a similar concentration (<0.2 mg mL−1).61
There was no adverse interaction observed between THF and n-FeOx that could impact the various parameters. However, this does not mean that iron catalyst particles cannot synergistically associate with CNTs to collectively have an impact on seed/seedling health. As can be seen in the data pertaining to germination rate, seeds exposed to raw SWCNTs expressed an approximately 35% lower GR value compared with their counterparts treated with purified SWCNTs (both in water media). Similar trends were seen in the case of data pertaining to seedling height on day 8, and visual scores. The effects were also inversely correlated in the case of the MGT data. The observed adverse effects of raw SWCNTs could be due to one or more of several mechanisms likely involving biomolecules pertaining to seed germination and plant growth. Further research is required to explore the various possible biochemical pathways through which nanomaterials (or mixtures thereof) can affect seed or plant health.34 Iron catalyst residues present in CNT samples are often encapsulated with carbon, but it has been shown that their bioavailability is equivalent to exposed analogues.86
Effect of CNTs
The typical length of the CNTs suggests that cellular uptake and intracellular transformations are unlikely to play a major role in their environmental fate. This was evidenced by results from a previous study where MWCNTs were not observed to fully enter or become encapsulated within wheat cells, possibly due to the relatively large size of the MWCNTs.87
MWCNTs showed seed mass gain as a function of time that was slightly greater than water. This trend was also observed with THF. Hence there was minimal or no effect of THF mediated by MWCNTS suggesting that THF was not adsorbed significantly onto the NPs. The lack of negative effect for the MWCNTs is consistent with the observation that MWCNT in aqueous suspension do not generate ROS.88 Conversely, it is observed that, when in contact with an external source of hydroxyl or superoxide radicals, MWCNT exhibit a remarkable radical scavenging capacity.
These results are in contrast with the work of Lin et al.89 that reported that the MWCNT suspended in natural organic matter (NOM) inhibited rice plant reproduction by delaying flowering by at least 1 month. The concentration used in this study (0.4 mg mL−1) was comparable to that used herein (0.5 mg mL−1), however, the presence of a solubilizing agent (in this case NOM) provides a key distinction.
The presence of MWCNTs in wheat and rapeseed leaves has been reported by Larue et al.90 In the present case, while optical microscopy of the root shows the association of MWCNTs (Fig. 10), no evidence was observed for uptake into the leaves. The lack of significant uptake in our system is unsurprising since it was previously found that uptake occurred only when the MWCNTs were dispersed using gum Arabic or humic acids.90 No uptake was observed when the MWCNTs were not solubilized.
 |
| Fig. 10 Optical microscopy image (100×) of sample 7 (Table 1) wheatgrass root after 8 days growth showing the presence of MWCNTs. | |
Although, De La Torre-Roche, et al. reported that MWCNTs impact the accumulation of weathered pesticides,41 it appears that THF (as an example of a small organic molecule) has minimal effect, suggesting limited adsorption to the surface of MWCNTs. As a result, the data suggests that any hydrophobic effects of MWCNTs were likely at best confined to the early growth stage.
Raw-SWCNTs showed a slightly lower mean germination rate (GR) and higher mean germination time (MGT) than the reference; however, the average plant height after 8 days was similar, suggesting that the effects were small and mainly during initial growth, after which they were negligible. Furthermore there is no effect of dispersion in THF. It is interesting to note that despite similar water dosage, the plants grown in the presence of raw-SWCNTs and purified-SWCNTs (in the absence of THF) were distinctly darker green in colour suggesting a healthier plant and is undoubtedly a symptom of the increased water delivery.
Purified SWNTs (without THF) encouraged the best germination rates and times, growth of seedlings, and overall visual scores in relation to the various parameters measured, when compared with nanomaterial-free controls with and without THF. As with iron oxide NPs, CNTs have been associated with the generation of ROS. However, studies have shown that unfunctionalized SWCNTs exhibit no measureable ROS production,91,92 while functionalized SWCNTs (e.g., carboxylated single-walled carbon nanotubes, SWNT-COOH) generate reactive species.91,93 Based upon this precedent there would not be expected any detrimental effects; however, the improved growth is unusual.
Contact angle measurements of both raw and purified SWCNTs show them to be hydrophobic (130 ± 2° and 62 ± 1°), which could cause the water to bead up and contain the water on the cotton wool. In contrast, iron oxide particle samples are hydrophilic (contact angle = 18 ± 3°) and thus are expected to result in the wetting of the n-FeOx and hence the cotton wool. Given the highly hydrophobic nature of the SWCNTs this effect is likely due to the creation of a contained source of water around the seed (rather than allowing for dispersion through the cotton wool). Thus, the SWCNTs may act as a “concentrator” for water delivery.
When THF was used as a dispersing solvent the results are dramatically different. It is likely that the THF adsorbed onto the hydrophilic regions of the purified SWNTs, causing localized high concentration areas that adversely impacted the growth of the seedlings despite the precautions taken initially to evaporate as much of the solvent as possible under vacuum. It is interesting that this detrimental effect diminishes with time; presumably as successive aliquots of water are added causing dilution of the THF.
Parameters chosen for the study
Parameters such as MGT and germination rate were chosen for evaluation in this study based on the guidelines of the International Seed Testing Association. In addition, scores based on visual observation such as seed mass gain, height of seedling on day 8, and a pre-defined visual score (based on the criteria laid out in Table 2) were also considered, especially in the context of the wheatgrass plant species used in this study. Based on an understanding of the various data points, visual scores were quick and easy to quantify, provided a composite overview of seed germination and plant growth, and correlated well with the other parameters. In particular, visual scores were found to be proportional to germination rate, and inversely proportional to mean germination time. Based on the authors' experience, visual scores and the way they are defined can be useful and convenient for field researchers especially when quantifying the consolidated impact of a chemical or nanomaterial on plant life. Such impacts can be so complex that it may be difficult to quickly or economically isolate certain phenomena. In such a case, composite scores can be used to represent the various changes/observations, and may provide a direct or indirect correlation with certain hard-to-measure parameters. The choice of the various parameters can be varied based on the type of study and its objectives. Sophisticated statistical tools can be used to further isolate relationships between various factors and observed phenomena, in addition to being able to develop well-defined mathematical regression models of experimental data. For example, depending on the type of plant species studied, future studies can potentially address phenomena such as the chronological expression of a biomarker or a biomolecule produced by the plant under various treatment conditions, the number of days taken for the first leaf to emerge from a shoot, or the number of leaves as a function of the treatment condition of time.
Conclusions
Overall, the chosen NPs and dosages, in addition to the solvents used to disperse them did not appear to adversely affect the wheatgrass seeds in terms of either germination or subsequent growth. The one main exception was the case of pure SWCNTs dispersed in THF wherein significant reductions in seed mass gain, and seedling germination/growth were observed. Although this effect is short lived upon continued water exposure, it still raises significant concerns over the environmental exposure of SWCNTs. While pure they may not represent a significant risk (and appear to have potential benefit) the combination of SWCNTs in the environment with typical organic pollutants and degradation products suggest that they may be concentrated and immobilised by the SWCNTs and thus made available more readily to plants. These results must be interpreted bearing in mind that the environment is a complex interplay between various types of biosystems (e.g., plants, animals, microbes, and associated cells and tissues) that interact with each other in a hard-to-predict manner. More research is needed in this regard. Further, caution and common sense need to be exercised when working with nanomaterials keeping in mind that we need to distinguish between nanomaterials that are biodegradable and not degradable. Certainly, the potential beneficial effects of CNTs to plant growth is an area of research in which only a few studies are reported.94–96 The enhanced growth observed in the presence of purified SWCNTs (in the absence of organic contaminants) suggests that similar hydrophobic substrates should be explored for high efficiency water channelling to seeds.97 This may be specifically important in regions where water supplies are limited and where percolation versus evaporation is an issue. In addition, future studies that involve evaluating the effect of varying dosages of nanomaterials, and controlled mixtures based on them, on germination and growth of various plant species in diverse conditions (e.g. soil-based cultivation in addition to hydroponic growth, with or without added nutrients/model chemical contaminants), can go a long way in attempting to understand how these human-made materials can affect the environment.
Conflicts of interest
There are no conflicts to declare.
Acknowledgements
Financial support was provided by the Welsh Government Sêr Cymru Programme and the Robert A. Welch Foundation (C-0002). The assistance Prof. K. Whitmire and Desmond Schipper are acknowledged for the use of optical microscopy. Purified SWCNTs were provided by David Pham, Kevin Zhang, Olawale Lawal, Dr. Robert Hauge, and Dr. Wade Adams. Dr. Kourtney D. Wright, Dr. Cathren Gowenlock, Dr. Shirin Alexander, Dr. Varun Shenoy Gangoli, and Dr. Bruce Brinson are acknowledged for their assistance with contact angle, Raman, and TEM measurements.
References
- J. Kolosnjaj-Tabi, J. Just, K. B. Hartman, Y. Laoudi, S. Boudjemaa, D. Alloyeau, H. Szwarc, L. J. Wilson and F. Moussa, EBioMedicine, 2015, 2, 1697–1704 CrossRef PubMed.
- J. Panyam and V. Labhasetwar, Adv. Drug Delivery Rev., 2003, 55, 329–347 CrossRef CAS PubMed.
-
M. C. Roco, C. A. Mirkin and M. C. Hersam, Nanotechnology Research Directions for Societal Needs in 2020: Retrospective and Outlook, Springer, 2010 Search PubMed.
- M. R. Wiesner, G. V. Lowry, P. Alvarez, D. Dionysiou and P. Biswas, Environ. Sci. Technol., 2006, 40, 4336–4345 CrossRef CAS PubMed.
-
P. M. V. Raja, G. Lacroix, J.-A. Sergent, F. Bois, A. R. Barron, E. Monbelli and D. Elgrabli, Nanotoxicology: Role of Physical and Chemical Characterization and Related In Vitro, In Vivo, and In Silico Methods, in Metrology and Standardization of Nanotechnology: Protocols and Industrial Innovations, ed. E. Mansfield, D. L. Kaiser, D. Fujita and M. Van de Voorde, Wiley-VCH Verlag GmbH & Co. KGaA, Weinheim, 2017 Search PubMed.
- K. L. Dreher, Toxicol. Sci., 2004, 77, 3–5 CrossRef CAS PubMed.
- A. Nel, T. Xia, L. Mädler and N. Li, Science, 2006, 311, 622–627 CrossRef CAS PubMed.
- C. Marambio-Jones and E. M. V. Hoek, J. Nanopart. Res., 2010, 12, 1531–1551 CrossRef CAS.
- P. M. Raja, J. Connolley, G. P. Ganesan, L. Ci, P. M. Ajayan, O. Nalamasu and D. M. Thompson, Toxicol. Lett., 2007, 169, 51–63 CrossRef CAS PubMed.
-
R. B. Mathur, B. P. Singh and S. Pande, Carbon Nanomaterials: Synthesis, Structure, Properties and Applications, Taylor & Francis, 2017 Search PubMed.
- C.-W. Lam, Toxicol. Sci., 2003, 77, 126–134 CrossRef PubMed.
- Z. Li, A. L. Branco de Barros, D. C. F. Soares, S. N. Moss and L. Alisaraiw, Int. J. Pharm., 2017, 524, 41–54 CrossRef CAS PubMed.
- K. Hegde, S. K. Brar, M. Verma and R. Y. Surampalli, Nanotechnol. Environ. Eng., 2016, 1, 5, DOI:10.1007/s41204-016-0005-4.
- J. G. Rouse, J. P. Ryman-Rasmussen, J. Yang, A. R. Barron and N. A. Monteiro-Riviere, Nano Lett., 2007, 7, 155–160 CrossRef CAS PubMed.
- M. Ema, H. Takehara, M. Naya, H. Kataura, K. Fujita and K. Honda, J. Toxicol. Sci., 2017, 42, 367–378 CrossRef PubMed.
- L. McJilton, C. Horton, C. Kittrell, D. Ogrin, H. Peng, F. Liang, W. E. Billups, H. K. Schmidt, R. H. Hauge, R. E. Smalley and A. R. Barron, Carbon, 2009, 47, 2528–2530 CrossRef CAS.
- B. Espinasse, E. M. Hotze and M. R. Wiesner, Environ. Sci. Technol., 2007, 41, 7396–7402 CrossRef CAS PubMed.
- F. Aslani, S. Bagheri, N. M. Julkapli, A. S. Juraimi, F. S. G. Hashemi and A. Baghdadi, Sci. World J., 2014, 2014, 641759, DOI:10.1155/2014/641759.
- C. M. Rico, S. Majumdar, M. Duarte-Gardea, J. R. Peralta-Videa and J. L. Gardea-Torresdey, J. Agric. Food Chem., 2011, 59, 3485–3498 CrossRef CAS PubMed.
- P. Begum, R. Ikhtiari, B. Fugetsu, M. Matsuoka, T. Akasaka and F. Watari, Appl. Surf. Sci., 2012, 262, 120–124 CrossRef CAS.
- P. Begum, R. Ikhtiari and B. Fugetsu, Nanomaterials, 2014, 4, 203–221 CrossRef PubMed.
- X.-M. Tan, C. Lin and B. Fugetsu, Carbon, 2009, 47, 3479–3487 CrossRef CAS.
- M. Khodakovskaya, E. Dervishi, M. Mahmood, Y. Xu, Z. Li, F. Watanabe and A. S. Biris, ACS Nano, 2009, 3, 3221–3227 CrossRef CAS PubMed.
- J. E. Cañas, M. Long, S. Nations, R. Vadan, L. Dai, M. Luo, R. Ambikapathi, E. H. Lee and D. Olszyk, Environ. Toxicol. Chem., 2008, 27, 1922–1931 CrossRef.
- D. Lin and B. Xing, Environ. Pollut., 2007, 150, 243–250 CrossRef CAS PubMed.
- B. Pan and B. Xing, Environ. Sci. Technol., 2008, 42, 9005–9013 CrossRef CAS PubMed.
- T. Kashiwagi, E. Grulke, J. Hilding, K. Groth, R. Harris, K. Butler, J. Shields, S. Kharchenko and J. Douglas, Polymer, 2004, 45, 4227–4239 CrossRef CAS.
- D. Bom, R. Andrews, D. Jacques, J. Anthony, B. Chen, M. S. Meier and J. P. Selegue, Nano Lett., 2002, 2, 615–619 CrossRef CAS.
- J. Kayat, V. Gajbhiye, R. K. Tekade and N. K. Jain, Nanomedicine, 2011, 7, 40–49 CrossRef CAS PubMed.
- V. E. Kagan, Y. Y. Tyurina, V. A. Tyurin, N. V. Konduru, A. I. Potapovich, A. N. Osipov, E. R. Kisin, D. Schwegler-Berry, R. Mercer, V. Castranova and A. A. Shvedova, Toxicol. Lett., 2006, 165, 88–100 CrossRef CAS PubMed.
- B. Manshian, G. J. S. Jenkins, P. M. Williams, C. Wright, A. R. Barron, A. P. Brown, N. Hondow, P. R. Dunstan, R. Rickman, K. Brady and S. H. Doak, NANO, 2013, 7, 144–156 CAS.
- K. Wright and A. R. Barron, C, 2017, 3, 17, DOI:10.3390/c3020017.
- D. Pham, K. S. Zhang, S. Ghosh, V. S. Gangoli, O. Lawal, B. Kellogg, T. Ainscough, R. H. Hauge, W. Adams and A. R. Barron, C, 2017, 3, 19, DOI:10.3390/c3020019.
- L. Siegert, D. K. Kampouris, J. Kruusma, V. Sammelselg and C. E. Banks, Electroanalysis, 2009, 21, 48–51 CrossRef CAS.
- T. Suzuki, K. Suhama, X. Zhao, S. Inoue and Y. Ando, Diamond Relat. Mater., 2007, 16, 1116–1120 CrossRef CAS.
- E. F. Antunes, V. G. de Resende, U. A. Mengui, J. B. M. Cunha, E. J. Corat and M. Massi, Appl. Surf. Sci., 2011, 257, 8038–8043 CrossRef CAS.
- M. Baalousha, Sci. Total Environ., 2009, 407, 2093–2101 CrossRef CAS PubMed.
- K. J. Ziegler, Z. Gu, H. Peng, E. L. Flor, R. H. Hauge and R. E. Smalley, J. Am. Chem. Soc., 2005, 127, 1541–1547 CrossRef CAS PubMed.
- D. Ogrin, J. Chattopadhyay, A. K. Sadana, W. E. Billups and A. R. Barron, J. Am. Chem. Soc., 2006, 128, 11322–11323 CrossRef CAS PubMed.
- V. Gomez, S. Irusta, O. B. Lawal, W. Adams, R. H. Hauge, C. W. Dunnill and A. R. Barron, RSC Adv., 2016, 6, 11895–11902 RSC.
- R. De La Torre-Roche, J. Hawthorne, Y. Deng, B. Xing, W. Cai, L. A. Newman, Q. Wang, X. Ma, H. Hamdi and J. C. White, Environ. Sci. Technol., 2013, 47, 12539–12547 CrossRef CAS PubMed.
- E. Oberdörster, Environ. Health Perspect., 2004, 112, 1058–1062 CrossRef.
- C. M. Sayes, A. M. Gobin, K. D. Ausman, J. Mendez, J. L. West and V. L. Colvin, Biomaterials, 2005, 26, 7587–7595 CrossRef CAS PubMed.
- C. M. Sayes, J. D. Fortner, G. Wenh, D. Lyon, A. M. Boyd, K. D. Ausman, Y. Tao, B. Sitharaman, L. J. Wilson, J. B. Hughes, J. L. West and V. L. Colvin, Nano Lett., 2004, 4, 1882–1887 CrossRef.
- T. B. Henry, E. J. Petersen and R. N. Compton, Curr. Opin. Biotechnol., 2011, 22, 533–537 CrossRef CAS PubMed.
- J. Cambedouzou, S. Rols, N. Bendiab, R. Almairac, J.-L. Sauvajol, P. Petit, C. Mathis, I. Mirebeau and M. Johnson, Phys. Rev. B: Condens. Matter Mater. Phys., 2005, 72, 041404 CrossRef.
- W. Ding, A. Eitan, F. Fisher, X. Chen, D. Dikin, R. Andrews, L. Brinson, L. S. Schadler and R. S. Ruoff, Nano Lett., 2003, 3, 1593–1597 CrossRef CAS.
- N. A. Koratkar, J. Suhr, A. Joshi, R. S. Kane, L. S. Schadler, P. M. Ajayan and S. Bartolucci, Appl. Phys. Lett., 2005, 87, 063102 CrossRef.
- W. Xu, J. Zhao, L. Qian, X. Han, L. Wu, W. Wu, M. Song, L. Zhou, W. Su, C. Wang, S. Nie and Z. Cui, Nanoscale, 2014, 6, 1589–1595 RSC.
- G. Girishkumar, K. Vinodgopal and P. V. Kamat, J. Phys. Chem. B, 2004, 108, 19960–19966 CrossRef CAS.
- G. Girishkumar, M. Rettker, R. Underhile, D. Binz, K. Vinodgopal, P. McGinn and P. Kamat, Langmuir, 2005, 21, 8487–8494 CrossRef CAS PubMed.
- S. Barazzouk, S. Hotchandani, K. Vinodgopal and P. Kamat, J. Phys. Chem. B, 2004, 108, 17015–17018 CrossRef CAS.
- P. Kamat, K. Thomas, S. Barazzouk, G. Girishkumar, K. Vinodgopal and D. Meisel, J. Am. Chem. Soc., 2004, 126, 10757–10762 CrossRef CAS PubMed.
- M. S. Shaffer, X. Fan and A. H. Windle, Carbon, 1998, 36, 1603–1612 CrossRef CAS.
- H. Hu, B. Zhao, M. A. Hamon, K. Kamaras, M. E. Itkis and R. C. Haddon, J. Am. Chem. Soc., 2003, 125, 14893–14900 CrossRef CAS PubMed.
- F. Wang, H. Gu and T. M. Swager, J. Am. Chem. Soc., 2008, 130, 5392–5393 CrossRef CAS PubMed.
- C. Wei, L. Dai, A. Roy and T. B. Tolle, J. Am. Chem. Soc., 2006, 128, 1412–1413 CrossRef CAS PubMed.
- B. Zhang, M. Cho, J. D. Fortner, J. Lee, C. H. Huang, J. B. Hughes and J. H. Kim, Environ. Sci. Technol., 2009, 43, 108–113 CrossRef CAS PubMed.
- C. P. Firme III and P. R. Bandaru, Nanomedicine, 2010, 6, 245–256 CrossRef PubMed.
- E. Oberdörster, Environ. Health Perspect., 2004, 112, 1058–1062 CrossRef.
- W. M. Lee, Y. J. An, H. Yoon and H. S. Kweon, Nanomater. Environ., 2008, 27, 1915–1921 CAS.
- D. K. Tripathi, S. Singh, S. Singh, R. Pandey, V. P. Singh, N. C. Sharma, S. M. Prasad, N. K. Dubey and D. K. Chauhan, Plant Physiol. Biochem., 2017, 110, 2–12 CrossRef CAS PubMed.
- J. Yang, W. Cao and Y. Rui, J. Plant Interact., 2017, 12, 158–169 CrossRef CAS; E. Andreoli, R. Suzuki, A. W. Orbaek, M. S. Bhutani, R. H. Hauge, W. Adams, J. B. Fleming and A. R. Barron, J. Mater. Chem., B, 2014, 2, 4740–4747 RSC.
- E. Andreoli, R. Suzuki, A. W. Orbaek, M. S. Bhutani, R. H. Hauge, W. Adams, J. B. Fleming and A. R. Barron, J. Mater. Chem. B, 2014, 2, 4740–4747 RSC.
- A. W. Orbaek, N. Aggarwal and A. R. Barron, J. Mater. Chem. A, 2013, 1, 14122–14132 CAS.
- L. Morrow, D. K. Potter and A. R. Barron, J. Exp. Nanosci., 2015, 10, 1028–1041 CrossRef CAS.
- L. Morrow and A. R. Barron, J. Nanopart., 2015, 2015, 105862, DOI:10.1155/2015/105862.
- L. Yang and D. J. Watts, Toxicol. Lett., 2005, 158, 122–132 CrossRef CAS PubMed.
- M. Hardej and T. Ozimek, Ecol. Eng., 2002, 18, 343–350 CrossRef.
- D. Lin and B. Xing, Environ. Pollut., 2007, 150, 243–250 CrossRef CAS PubMed.
- X. Wang, S. Gao, L. Wang and H. Shuokui, Chemosphere, 2001, 44, 1711–1721 CrossRef CAS PubMed.
- S. J. Scott, R. A. Jones and W. A. Williams, Crop Sci., 1984, 24, 1192–1199 CrossRef.
- M. Khajeh-Hosseini, A. Lomholt and S. Matthews, Seed Sci. Technol., 2009, 37, 446–456 CrossRef.
- S. Matthews and M. Khajeh-Hosseini, Seed Sci. Technol., 2006, 34, 339–347 CrossRef.
- J. B. Edmond and W. J. Drapala, Proc. Am. Soc. Hortic. Sci., 1958, 71, 428–434 Search PubMed.
- H. C. Huang and R. S. Erikson, J. Phytopathol., 2006, 155, 31–37 CrossRef.
- B. Murillo-Amador, R. Lopez-Aguilar, C. Kaya, J. Larrinaga-Mayoral and A. Flores-Hernández, J. Agron. Crop Sci., 2002, 188, 235–247 CrossRef CAS.
- J. D. Maguire, Crop Sci., 1962, 2, 176–177 CrossRef.
- K. L. Strong, D. P. Anderson, K. Lafdi and J. NKuhn, Carbon, 2003, 41, 1477–1488 CrossRef CAS.
- K. D. Wright, C. E. Gowenlock, J. C. Bear and A. R. Barron, ACS Appl. Mater. Interfaces, 2017, 9(32), 27202–27212 CAS.
- H. Honda, C.-M. Yang, H. Kanoh, H. Tanaka, T. Ohba, Y. Hattori, S. Utsumi and K. Kaneko, J. Phys. Chem. C, 2007, 111, 3220–3223 CAS.
- Y. Li, W. Zhang, J. Niu and Y. Chen, ACS Nano, 2012, 6, 5164–5173 CrossRef CAS PubMed.
- A. Nel, T. Xia, L. Madler and N. Li, Science, 2006, 311, 622–627 CrossRef CAS PubMed.
- L. Risom, P. Moller and S. Loft, Mutat. Res., 2005, 592, 119–137 CrossRef CAS PubMed.
- Y. H. Chen and C. C. Lin, Phys. Chem. Miner., 2014, 41, 727–736 CrossRef CAS.
- L. Guo, D. G. Morris, X. Liu, C. Vaslet, R. H. Hurt and A. B. Kane, Chem. Mater., 2007, 19, 3472–3478 CrossRef CAS.
- E. Wild and K. C. Jones, Environ. Sci. Technol., 2009, 43, 5290–5294 CrossRef CAS PubMed.
- I. Fenoglio, M. Tomatis, D. Lison, J. Muller, A. Fonseca, J. B. Nagy and B. Fubini, Free Radical Biol. Med., 2006, 40, 1227–1233 CrossRef CAS PubMed.
- C. Lin, B. Fugetsu, Y. Su and F. Watari, J. Hazard. Mater., 2009, 170, 578–583 CrossRef CAS PubMed.
- C. Larue, M. Pinault, B. Czarny, D. Georgin, D. Jaillard, N. Bendiab, M. Mayne-L'Hermite, F. Taran, V. Dive and M. Carrière, J. Hazard. Mater., 2012, 227–228, 155–163 CrossRef CAS PubMed.
- C.-Y. Chen and C. T. Jafvert, Carbon, 2011, 49, 5099–5106 CrossRef CAS.
- K. Pulskamp, S. Diabaté and H. F. Krug, Toxicol. Lett., 2007, 168, 58–74 CrossRef CAS PubMed.
- C.-Y. Chen and C. T. Jafvert, Environ. Sci. Technol., 2010, 44, 6674–6679 CrossRef CAS PubMed.
- A. Mondal, R. Basu, S. Das and P. Nandy, J. Nanopart. Res., 2011, 13, 4519–4528 CrossRef CAS.
- D. K. Tiwari, N. Dasgupta-Schubert, L. M. Villaseñor Cendejas, J. Villegas, L. Carreto Montoya and S. E. Borjas García, Appl. Nanosci., 2014, 4, 577–591 CrossRef CAS.
- M. V. Khodakovskaya, K. de Silva, A. S. Biris, E. Dervishi and H. Villagarcia, ACS Nano, 2012, 6, 2128–2135 CrossRef CAS PubMed.
- S. Alexander, J. Eastoe, A. M. Lord, F. Guittard and A. R. Barron, ACS Appl. Mater. Interfaces, 2016, 8, 660–666 CAS.
Footnote |
† Electronic supplementary information (ESI) available: Additional photographs of seed trays, individual seed growth data, TEM, SEM, TGA and Raman data. See DOI: 10.1039/c7en00680b |
|
This journal is © The Royal Society of Chemistry 2018 |
Click here to see how this site uses Cookies. View our privacy policy here.