DOI:
10.1039/D4QI00171K
(Research Article)
Inorg. Chem. Front., 2024,
11, 2626-2633
Synthesis and characterization of a CsPbCl3 perovskite doped with Nd3+: structural, optical, and energy transfer properties
Received
18th January 2024
, Accepted 18th March 2024
First published on 2nd April 2024
Abstract
The purpose of this paper is to synthesize a micrometric inorganic perovskite CsPbCl3:Nd3+ and investigate the impact of doping with rare-earth ions on structural and optical properties, as well as energy transfer pathways between the host and dopant. Herein, we report the solid-state reaction synthesis of a concentration series of CsPbCl3:x%Nd3+ annealed under a nitrogen atmosphere. Additional doping of a material that already exhibits luminescence with an optically active ion increases its application potential. Structural features were determined using X-ray powder diffraction and Raman spectroscopy. Morphology studies performed with scanning electron microscopy revealed micrometric, well-separated cubic-like crystallites with a good distribution of individual elements. Surprisingly, a photoluminescence (PL) study showed that only blue emission appears when the material is excited with a diode operating in the UV range. Apparently, the emission of Nd3+ ions can only be obtained with direct excitation of the lanthanide. The photoluminescence excitation (PLE) spectrum monitored for Nd3+ emission confirmed the lack of energy transfer between the host and dopant. Possible explanations for this behavior have been put forth and substantiated by the first-principles electronic structure calculations in the framework of hybrid density functional theory.
Introduction
Perovskite halides (chlorides, iodides, and bromides) are popular choices for nanocrystalline optoelectronic materials due to their simple synthesis route and efficient tunable luminescence. The research on perovskite halides as luminescent materials has been conducted extensively due to the broadband host emission believed to originate from the coupling of the excited carriers with distorted lead halide units.1 This origin of the luminescence of halide perovskites is responsible for high quantum yield and provides the possibility of material optimization by doping with either optically active2 or inactive ions,3 by means of bandgap engineering. Cesium lead chloride with the chemical formula CsPbCl3 is a member of the all-inorganic halide perovskite family and has attracted significant attention in the scientific community due to its high efficiency and stability compared to its organic–inorganic counterparts. The choice of the synthesis method can affect the quality, size, and morphology of the resulting samples, influencing their optoelectronic properties and potential applications. The development of efficient and reproducible synthesis methods is crucial for the widespread use of the CsPbCl3 perovskite in various optoelectronic applications.
Recent research on perovskite nanocrystals and thin films has focused on improving the stability and efficiency of CsPbCl3 perovskite solar cells.4,5 Moreover, the CsPbCl3 perovskite has shown potential in other optoelectronic applications, such as light-emitting diodes (LEDs)6 or as an element of a phosphor–glass composite.7 Overall, the CsPbCl3 perovskite has demonstrated great potential as a candidate for high-performance and stable optoelectronic devices. It has also recently been studied extensively as a nanocrystal host for rare earth and transition metal dopants. Since the 1990s, it has also been known for exhibiting free exciton emission in the UV region under high-intensity excitation.8,9 This exciton state has been used as a sensitizer to enhance the dopant's emission.10 It can be noted that the literature considering nanometric inorganic halide perovskites is quite rich, but studies involving larger analogs are still very limited. CsPbCl3 quantum dots have been reported to exhibit efficient blue-violet emission under UV excitation.11 A recent study investigated the structural and spectroscopic properties of micrometric CsPbCl3 perovskite powders doped with Yb3+ ions, with varying dopant concentrations (1–20%) prepared via a simpler solid-state reaction route. Compared to quantum dots, micrometric CsPbCl3 can be prepared via a solid-state reaction route and exhibit blue emission.12 The study illustrates that similar to other lead halides, the CsPbCl3 host is expected to transfer energy to the RE dopant.2 Our current study demonstrates this assumption to be inaccurate for some RE dopants.
Nd3+ has a characteristic emission spectrum that includes sharp lines in the near-infrared region, with emission peaks at around 880 nm, 1060 nm, and 1330 nm. The emission intensity and lifetime of Nd3+ luminescence are highly dependent on the local crystal field environment, which can be tailored by doping the ion into different host materials. Nd3+ exhibits a fluorescence lifetime, which can range from microseconds to milliseconds, making it ideal for applications such as solid-state lasers,13 where the neodymium ions act as an active medium, producing high-power laser emissions. These materials also find applications in optical amplifiers, fiber optic communication,14 and biological imaging.15 Due to the fact that the ionic radius of Nd3+ ions is smaller (99.5 pm) than that of Pb2+ ions (119 pm),16 they can be doped in higher concentrations without changing the structure of CsPbCl3.
Recently Xiong et al. investigated CsPbCl3:Nd3+,17 where the dopant was used not as a luminescent center but rather as an enhancement agent for exciton emission. Another study reported the spectroscopic properties of Mn2+- and Nd3+-co-doped CsPbCl3.18 To the best of our knowledge, no luminescence of Nd3+ ions in CsPbCl3 upon direct excitation of the dopant was reported. In this paper, we report the structure, morphology, and luminescence properties of a concentration series of CsPbCl3:Nd3+ microcrystals synthesized by a solid-state reaction method. Additionally, we provide an explanation for the absence of energy transfer between the host and the dopant, supported by first-principles calculations using a hybrid density functional model.
Results and discussion
Structural and thermal properties
Fig. 1a shows the XRD patterns of a concentration series of CsPbCl3 perovskite powders doped with Nd3+ ions. Analysis of the diffractograms confirmed that the studied host can accommodate a maximum of 20% dopant without structural disruption. A further increase in the concentration of Nd3+ ions caused the appearance of foreign peaks, inconsistent with pattern #243734. Therefore, the CsPbCl3:25%Nd3+ sample was not considered in further investigations. The pure phase samples exhibited an orthorhombic structure belonging to the Pnma space group. Fig. 1b presents a simplified diagram of a CsPbCl3 unit cell containing only lead atoms. The studied material reveals a distance between Pb ions, partially substituted by Nd,19 reaching more than 5 Å, which ensures a low concentration quenching rate.
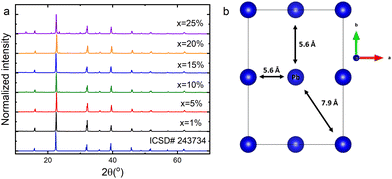 |
| Fig. 1 X-ray diffraction patterns of the CsPbCl3:x%Nd3+ perovskite powders (a) and a simplified unit cell scheme of an undoped sample (b). | |
To validate the thermal stability of the studied perovskites, we performed thermogravimetric analysis for powders containing 1 and 20% of Nd3+ ions (Fig. 2). The decomposition of the sample with a lower concentration begins at around 840 K. Increasing the dopant ion concentration to 20% lowers the degradation threshold to 825 K. In both cases, degradation ends at around 1200 K. The differences in the weight loss suggest that metallic neodymium is the final form. Fig. 3 shows the scanning electron microscopy (SEM) images of the morphology of CsPbCl3 perovskite powders doped with Nd3+. It can be observed that the investigated host shows well-developed crystallites with a polyhedron shape, sharp boundaries and different sizes with a dominant micrometric fraction. It is worth noting that even a 20% dopant ion content does not affect the quality of the obtained crystallites. The situation is different in comparison with CsPbCl3 doped with Yb3+ ions synthesized using the same technique.20 In the latter case, the strongly doped samples (more than 10%) had blurred grain boundaries and their aggregation was very advanced, which significantly affected their optical properties.
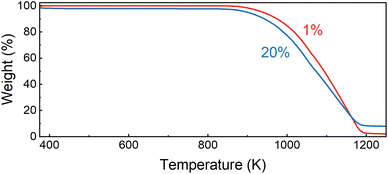 |
| Fig. 2 TGA curves for CsPbCl3 powders composed of 1 and 20% of Nd3+ ions. | |
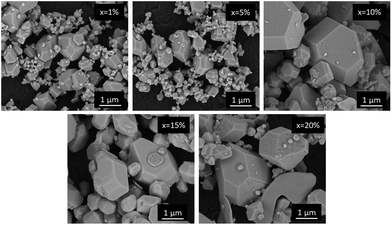 |
| Fig. 3 SEM images of the CsPbCl3 perovskite powders containing different concentrations of Nd3+ ions. | |
In order to check the distribution of individual elements, EDS mapping for a CsPbCl3:10%Nd3+ ion was performed and is presented in Fig. 4. It is proven that the elements are evenly distributed throughout the crystallite volume and the incorporation of Nd3+ was successful.
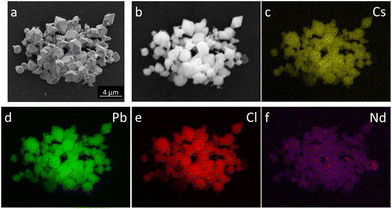 |
| Fig. 4 SEM image (a) and EDS elemental mapping (b–f) of the CsPbCl3:10%Nd3+ perovskite powder. | |
Raman spectra
Fig. 5 illustrates the Raman spectra of all examined powders. All spectra exhibit four bands with maxima at about 24, 36, 114, and 199 cm−1, as well as two shoulders at roughly 57 and 104 cm−1 at ambient temperature. The recorded spectra match well the data reported in the literature for the orthorhombic phase of CsPbCl3 and demonstrate that the structure is maintained over the whole concentration range.21,22 Raman bands become narrow and well-spaced at 80 K, with peaks at 30, 35, 41, 51, 71, 88, 109, 120, and 201 cm−1 for x = 1%. At both temperatures, an increased dopant ion concentration induces band broadening and an intensity decrease, which are connected to the increased concentration of defects generated by substitution. This impact is most prominent under ambient conditions for bands in the 100–120 cm−1 range, with a strong contribution from Cs+ ion movements.21,22 The relative change in the intensity of this band decreases exponentially as the dopant ion concentration increases, demonstrating successful substitution with Nd3+ ions in the crystal structure. The concentration-related broadening of bands associated with longer phonon lifetimes becomes more apparent after cooling. Band shifts caused by various masses and radii of Pb2+ and Nd3+ ions are noticeable in addition to the observed widening. The strongest of these, ranging up to around 2.3 cm−1, are connected with the vibrations of PbCl6 octahedra in the 70–90 cm−1 range.21,22
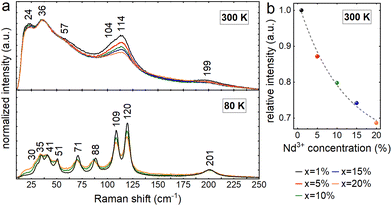 |
| Fig. 5 Raman spectra of the CsPbCl3 perovskite powders containing different concentrations of Nd3+ ions at 300 and 80 K (a) together with the relative intensity change of the band at 114 cm−1 at 300 K (b). | |
Optical properties
The diffuse reflectance spectra of a concentration series of CsPbCl3 perovskite powders doped with Nd3+ ions cover the range of the UV-VIS-NIR region as presented in Fig. 6a. It exhibits a strong band in the range of 200–400 nm corresponding to the absorption of the host.23 An exciton band at the absorption edge flattens out as the concentration of Nd3+ ions increases (Fig. 6b). In the visible and infrared spectral ranges, less intense absorption bands originating from Nd3+ f–f transitions can be observed.24 Diffuse reflectance spectra were used to determine the size of the energy band gap (Eg) in CsPbCl3:x%Nd3+ perovskite powders by the Kubelka–Munk method: | 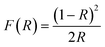 | (1) |
where R is the reflectance. The band gap energies were extrapolated from the intercept of the fitted straight line at F(R) = 0 in the F(hν)1/2versus the energy (hν) plot. The calculation results can be found in Fig. 6c. It was found that the energy gap is of the order of 2.9 eV and reaches the highest value for the material containing the smallest concentration of the dopant. Our first-principles calculations confirmed a gap of 2.93 eV between the host's valence band (VB) and the conduction band (CB), as illustrated in Fig. 9, providing further support for this experimental determination.
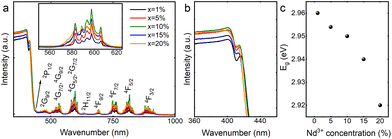 |
| Fig. 6 Diffuse reflectance spectra of the CsPbCl3:x%Nd3+ perovskite powders (a) together with an enlarged spectrum showing the exciton band (b) and energy gap values as a function of dopant concentration (c). | |
The excitation spectrum of CsPbCl3:20%Nd3+ was recorded and is shown in Fig. 7a. It can be seen that by monitoring the wavelength of 1061 nm, conventional f–f transitions of Nd3+ ions were observed, identical to those in the visible to NIR range of the absorption spectrum (Fig. 6); however, in the ultraviolet range (300–400 nm), no band originating from the host was detected, indicating a lack of energy transfer between the host and the dopant. It is worth mentioning that there are not many reports in the literature on CsPbCl3 doped with Nd3+ ions. It is probably due to the lack of energy transfer, which greatly limits its potential applications and makes this material unattractive. Recently, a paper reporting emission from Nd3+ ions in CsPbCl3 under 365 nm excitation was published.18 However, in that case, Mn2+ ions were introduced into the crystal structure of the host, which provided a bridge for energy transfer between the matrix and the Nd3+ ions.
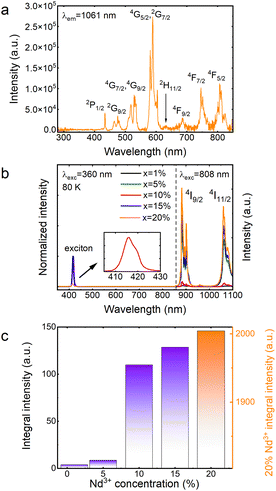 |
| Fig. 7 Excitation spectra of CsPbCl3:20%Nd3+ at 300 K (a). Emission spectra of the CsPbCl3:x%Nd3+ perovskite powders recorded at 80 K together with an inset showing an enlarged exciton spectrum of the CsPbCl3:10%Nd3+ sample (b), and integral intensities of Nd3+ emission (c). For clarity in (c), the sample containing 20% of the dopant is shown on a larger scale. | |
The emission spectra of a concentration series of CsPbCl3 perovskite powders doped with Nd3+ ions recorded at liquid nitrogen temperature are depicted in Fig. 7b. Experiments at 80 K were required because the luminescence of the exciton was completely quenched at 300 K. It is also worth noting that the spectra in the visible range were recorded upon 360 nm excitation, while the spectra in the infrared range were collected under 808 nm excitation. The measurement system was designed to expose the sample to two different beams at the same time, while the detector recorded the signal continuously in the range of 350–1100 nm. This arrangement made it possible to merge both spectra. The use of two excitation sources was necessary because it turned out that no energy transfer to Nd3+ ions was observed when the sample was excited into the host's conduction band (Fig. 7a). Regardless of the concentration of dopant ions, the analyzed materials revealed exciton bands in the visible range, the maxima of which are located at about 416 nm, while in the infrared range, two bands of Nd3+ ions were visible, whose emission maxima are located at 882 nm and 1060 nm, assigned to 4F3/2 → 4I9/2 and 4F3/2 → 4I11/2 transitions, respectively.25 In order to indirectly compare the emission intensities of Nd3+ ions, the spectra shown in Fig. 7b were normalized relative to the exciton intensity and their relative intensities are plotted in Fig. 7c. It can be clearly seen that as the dopant concentration increases, the Nd3+ emission intensity also increases, and the concentration quenching,26 typical of this lanthanide usually occurring by a cross-relaxation process, is not observed. In fact, the opposite situation happens. The intensity of the CsPbCl3:20%Nd3+ sample is so high that showing it in the same figure as the other samples required an additional scale. The lack of concentration quenching is probably related to the large Nd3+–Nd3+ distances (partially substituted lead positions due to its similar size and smaller charge mismatch compared to cesium27) in the unit cell presented in Fig. 1b. A minimal shift of the maximum of the exciton's absorption bands toward higher energies is observed. This is related to a slight reduction in the unit cell volume of CsPbCl3:Nd3+ as a consequence of the substitution of the larger (Pb2+) ion with a smaller one (Nd3+), which is consistent with the quantum confinement effect.28,29 A similar behavior was reported earlier in the literature.17
Fig. 8 shows the luminescence kinetics of the investigated compounds measured under 360 nm and 808 nm excitation and monitoring of the emission maximum of the exciton (Fig. 8a) and Nd3+ ions (Fig. 8b), respectively. It is worth noting that depending on the monitored band, measurements at different temperatures were made due to hardware limits. Unfortunately, the Nd3+ emission intensity of the sample containing 1% dopant was below the detection capabilities of the measurement system; therefore, recording its profile was impossible. Nevertheless, it can be observed that all of the measured curves show a bi-exponential nature. In the case of an exciton, this can be attributed to the presence of free and bound excitons (the asymmetrical shape of the band shown in the inset of Fig. 7b
20). However, in the case of dopant ions, some non-radiative processes may be responsible for such behavior. It can be clearly seen that as the Nd3+ concentration increases, the deviation from the exponential characteristics of the profiles becomes more pronounced. The luminescence profiles of CsPbCl3:x%Nd3+ were well matched using a double exponential function:
| 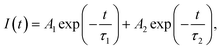 | (2) |
where
I(
t) means the emission intensity,
A1 and
A2 are the fitting constants and
τ1 and
τ2 stand for their corresponding lifetimes. The average lifetimes (
tav) were determined using
eqn (3) and are presented in
Table 1. It turned out that the luminescence decays of excitons observed in the investigated material are shorter than those already reported for CsPbCl
3 doped with transition metals or lanthanides obtained in the form of nanocrystalline colloids or films.
30–33 | 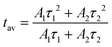 | (3) |
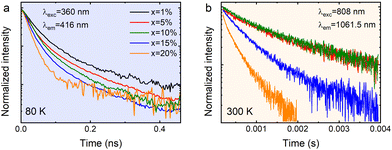 |
| Fig. 8 The luminescence decay profiles of the exciton (a) and Nd3+ (b) luminescence in CsPbCl3:x%Nd3+ perovskite powders. | |
Table 1 The lifetimes of the excitons and Nd3+ ions calculated by fitting the decays with the double-excitation function
Dopant content |
Exciton lifetime (80 K) |
Neodymium lifetime (300 K) |
1% |
53 ps |
|
5% |
47 ps |
2.15 ms |
10% |
46 ps |
1.93 ms |
15% |
31 ps |
0.86 ms |
20% |
28 ps |
0.19 ms |
It seems that the mechanism responsible for the generation of exciton luminescence in CsPbCl3:Nd3+ is similar to that presented by us earlier for the same host but doped with Yb3+ ions.20 In this case, as in the previous one, there is also an asymmetric emission band in the visible range upon excitation with a 360 nm line (inset in Fig. 7b), which indicates the emission of a free exciton (FE) and bound exciton (BE) on the defect simultaneously after the prior population of the host conduction band. However, in the analog containing Nd3+, there was no energy transfer between the host and dopant ions, as they appear relatively isolated from each other. The reduction of the Nd3+ emission lifetime is observed in samples with a dopant concentration above 10%. Given the fact that the possibility of concentration quenching was excluded by the increasing intensity as a function of concentration, such a reduction in lifetimes can be related to the appearance of surface defects due to heavy doping with lanthanide ions.
Fig. 9 shows the calculated density of states of the CsPbCl3:Nd3+ system. For the pristine host, the top of the valence bands is dominated by the Pb-s and Cl-p orbitals, and the bottom of the conduction bands is dominated by the Pb-p orbitals. With the doping of Nd3+ ions, the top of the valence bands is hardly influenced, while the bottom of the conduction bands is greatly hybridized by the Nd-4f orbitals. Our first-principles calculations demonstrate that the ground state 4I9/2 of Nd3+ ions in CsPbCl3 lies 3.88 eV below the top of the host's VB, as illustrated in Fig. 9. The energy positions of Nd3+ dopants are greatly influenced by the properties of hosts, as the variation of the vacuum-referred binding energy of the 4f level is no more than 0.4 eV for inorganic compounds.34 Here, the halide anions exhibit less Pauling electronegativity than the oxygen anion, and the top of the valence bands was greatly hybridized by the Pd-s orbitals, which makes the vacuum-referred binding energy of the valence bands higher than those of the oxides. As a result, the excited energy levels of Nd3+ ions, considering the multiplets up to 4D3/2 obtained from Dieke's diagram, are entirely buried within the VB and are unable to accept carriers from the host. Moreover, the energy mismatch between the exciton energy and the energy separation between Nd3+ multiplets exacerbates the hindrance of energy transfer. These factors, as depicted in Fig. 10, effectively block any energy transfer from the host to Nd3+ dopants. Therefore, the only way to obtain luminescence from Nd3+ ions in the investigated host is to excite them directly using, for example, an 808 nm line.
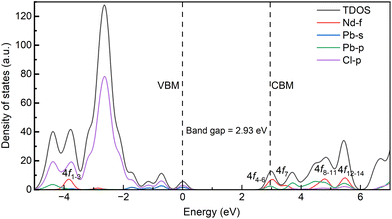 |
| Fig. 9 Density of state diagrams calculated for CsPbCl3:Nd3+. The labels VBM and CBM represent the maximum of the valence band and the minimum of the conduction band, respectively. The positions of the fourteen 4f dopant energy states are denoted by 4fn (n = 1–14). | |
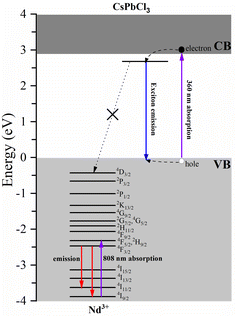 |
| Fig. 10 Electronic energy level scheme determined for the CsPbCl3:Nd3+ perovskite powder. | |
Conclusions
Systematic structural and optical studies considering a micrometric CsPbCl3 perovskite containing Nd3+ ions with concentrations in the range of 1–25% have been reported. Powdered samples were prepared using the classical solid state reaction method. XRD diffractograms and Raman spectra proved that the investigated materials exhibit an orthorhombic Pnma symmetry and do not show additional phases originating from impurities when the dopant concentration does not exceed 20%. TGA analysis revealed that the investigated materials are highly thermally stable. SEM images confirmed the micrometric size of the CsPbCl3:Nd3+ crystallites and elemental mapping showed that the dopant is homogeneously distributed throughout the sample volume. Diffuse reflectance spectra indicated strong absorption in the UV range and typical Nd3+ ion transitions in CsPbCl3. It turned out that the magnitude of the energy gap is of the order of 2.96 eV and gently decreases with increasing dopant concentration. Studies of optical properties revealed that by excitation of the host conduction band, only exciton luminescence can be obtained. In order to produce emission from Nd3+ ions, direct excitation is required. The reliability of the measured host band gap was confirmed through our first-principles calculations. The calculations further demonstrate that the electronic energy levels of the excitons remain isolated from those of Nd3+ ions, thereby indicating the absence of energy transfer between them.
Experimental
Chemicals
The concentration series of CsPb1−xNdxCl3 (x = 1, 5, 10, 15, 20, and 25 mol%) perovskite powder was obtained via the solid-state reaction route. Stoichiometric amounts of the following precursors: CsCl (Sigma-Aldrich, 99.9%), PbCl2 (Sigma-Aldrich, 98%) and NdCl3·6H2O (Sigma-Aldrich, 99.9%) were weighed and mixed vigorously in an agate mortar in the presence of CH3OH. Alcohol was added solely to provide a better environment for mixing the reactants, which is crucial in solid-state synthesis to achieve an even distribution of components. After thorough mixing and evaporation of CH3OH, the obtained powder was moved to a quartz boat and placed in a tube furnace. The heating process was carried out according to the same regime as reported previously12,20 under a nitrogen flow. The dopant content was determined based on theoretical calculations.
Measurement methods
The X-ray diffraction (XRD) pattern was measured using an X'Pert PRO powder diffractometer (PANalytical, The Netherlands) equipped with a linear PIXcel detector and using Cu-Kα radiation (λ = 1.54056 Å). A PerkinElmer TGA 4000 was used to perform thermogravimetric analysis (TGA) on samples containing 1 and 20% of Nd3+ ions at temperatures ranging from 300 to 1250 K. The samples weighed 91.88 and 60.63 mg, respectively, and a 10 K min−1 heating rate was used. Nitrogen was used as a purging gas. The chemical composition and general morphology of the samples were checked using a FE-SEM microscope (FEI Nova NanoSEM 230) equipped with an EDS analyzer (EDAX Genesis XM4). Raman spectra at room temperature and 80 K were recorded in the 10–300 cm−1 range using a Renishaw InVia Raman spectrometer equipped with a confocal DM 2500 Leica optical microscope, a thermoelectrically cooled CCD as a detector and a laser operating at 830 nm. The absorption measurements were performed in the back scattering mode using an Agilent Cary 5000 spectrophotometer. The excitation spectrum was recorded using a FLS1000 fluorescence spectrometer from Edinburgh Instruments. Low-temperature emission spectra were recorded with a Hamamatsu photonic multichannel analyzer PMA-12 equipped with a BT-CCD linear image sensor. The laser diode operating under 360 nm and 808 nm was applied as an excitation source. The temperature of the samples during emission measurements was controlled by using a Linkam THMS600 heating/freezing stage. The luminescence decay profiles of the excitons were recorded using a femtosecond laser (Coherent Model Libra). The luminescence decay profiles of Nd3+ emission were recorded using the McPherson spectrometer with a Hamamatsu R955 photomultiplier and a digital oscilloscope Tektronix MDO3052, as well as a Nd:YAG-pumped Ti-sapphire tunable pulse laser.
Computational method
The first-principles calculations were performed utilizing the Vienna ab initio simulation package,35 employing the projector augmented wave method in conjunction with density functional theory (DFT). To accurately capture the electronic properties of Pb-based halide perovskites, the PBE0 hybrid functional with 25% of non-local Fock exchange36 was adopted, along with the consideration of the spin–orbit coupling interaction, following the recommendation of ref. 37. The valence configurations of Nd, Cs, Pb and Cl were determined as 5s25p66s24f4, 5s25p66s1, 5d106s26p2 and 3s23p5, respectively. The dopant effect of Nd3+ ions was modeled in the 2 × 2 × 2 supercell, where one Pb2+ ion was substituted by one Nd3+ ion. For the pure and doped systems, the closed-shell and spin-polarized DFT calculation forms were applied, respectively. The plane-wave basis cutoff energy was set to 500 eV, with the convergence criteria of 10−5 eV for electronic energy minimization and 0.02 eV Å−1 for Hellmann–Feynman forces on each atom. A 6 × 6 × 6 k-point mesh based on the Monkhorst–Pack scheme was employed for the pure CsPbCl3 host, while a single k point at Γ was utilized for all the supercells.
Data availability
The data presented in this study are openly available in Zenodo at DOI: 10.5281/zenodo.8207373.
Conflicts of interest
There are no conflicts to declare.
Acknowledgements
This research was funded by the National Science Center, Poland, grant no. NCN-2021/43/D/ST5/01865. C.G. Ma acknowledges the support from the National Natural Science Foundation of China (Grant No. 52161135110 and 12274048). BL acknowledges the support from the Scientific and Technological Research Program of the Chongqing Municipal Education Commission (Grant No. KJQN202200629). The authors thank Professor Marek Drozd for performing the thermogravimetric measurements.
References
- Y. Zhou, Z. J. Yong, K. C. Zhang, B. M. Liu, Z. W. Wang, J. S. Hou, Y. Z. Fang, Y. Zhou, H. T. Sun and B. Song, Ultrabroad Photoluminescence and Electroluminescence at New Wavelengths from Doped Organometal Halide Perovskites, J. Phys. Chem. Lett., 2016, 7, 2735–2741 CrossRef CAS PubMed
.
- Y. Zhou, J. Chen, O. M. Bakr and H. T. Sun, Metal-Doped Lead Halide Perovskites: Synthesis, Properties, and Optoelectronic Applications, Chem. Mater., 2018, 30, 6589–6613 CrossRef CAS
.
- Z. J. Yong, S. Q. Guo, J. P. Ma, J. Y. Zhang, Z. Y. Li, Y. M. Chen, B. Bin Zhang, Y. Zhou, J. Shu, J. L. Gu, L. R. Zheng, O. M. Bakr and H. T. Sun, Doping-Enhanced Short-Range Order of Perovskite Nanocrystals for Near-Unity Violet Luminescence Quantum Yield, J. Am. Chem. Soc., 2018, 140, 9942–9951 CrossRef CAS PubMed
.
- A. Ishii and T. Miyasaka, Quantum cutting-induced near-infrared luminescence of Yb3+ and Er3+ in a layer structured perovskite film, J. Chem. Phys., 2020, 153, 194704 CrossRef CAS PubMed
.
- J. Jiang, Z. Jin, F. Gao, J. Sun, Q. Wang and S. Liu, CsPbCl3-Driven Low-Trap-Density Perovskite Grain Growth for >20% Solar Cell Efficiency, Adv. Sci., 2018, 5, 1800474 CrossRef PubMed
.
- T. Fan, J.-T. Lü, W.-Q. Deng, J.-X. Mai, J. Liang, T.-T. Deng, W. Fan, Q.-S. Zhou and Q.-F. Lin, Orange LED and cell fluorescence imaging of Mn2+ doped CsPbCl3 water-soluble nanocrystals with improved stability and optimized luminescence, Ceram. Int., 2023, 49, 13066–13073 CrossRef
.
- L.-Y. Cao, S.-C. Si, J.-B. Yu, C.-G. Ma, J.-B. Qiu and J. Wang, A precisely space-separated strategy of donor-acceptor for intense red emitting composite borosilicate glass co-doped with CsPbCl3 quantum dots and Mn2+ ions, Chem. Eng. J., 2021, 417, 129177 CrossRef CAS
.
- I. Baltog, S. Lefrant, C. Dimofte and L. Mihut, Phonon assisted excitonic luminescence in CsPbCl3, Radiat. Eff. Defects Solids, 1995, 135, 285–287 CrossRef CAS
.
- I. Baltog, L. Mihut and S. Lefrant, Excitonic luminescence in CsPbCl3 crystals under intense excitation, J. Lumin., 1996, 68, 271–277 CrossRef CAS
.
- K. Xu and A. Meijerink, Tuning Exciton–Mn2+ Energy Transfer in Mixed Halide Perovskite Nanocrystals, Chem. Mater., 2018, 30, 5346–5352 CrossRef CAS PubMed
.
- Y. Liu, G. Pan, R. Wang, H. Shao, H. Wang, W. Xu, H. Cui and H. Song, Considerably enhanced exciton emission of CsPbCl3 perovskite quantum dots by the introduction of potassium and lanthanide ions, Nanoscale, 2018, 10, 14067–14072 RSC
.
- M. Stefanski, V. Boiko, M. Ptak and W. Strek, Effect of Yb3+ concentration on the optical properties and trap creation in CsPbCl3 perovskite powder, J. Alloys Compd., 2022, 905, 164216 CrossRef CAS
.
- J. Lu, K. I. Ueda, H. Yagi, T. Yanagitani, Y. Akiyama and A. A. Kaminskii, Neodymium doped yttrium aluminum garnet (Y3Al5O12) nanocrystalline ceramics - A new generation of solid state laser and optical materials, J. Alloys Compd., 2002, 341, 220–225 CrossRef CAS
.
- M. Naftaly and A. Jha, Nd3+-doped fluoroaluminate glasses for a 1.3 μm amplifier, J. Appl. Phys., 2000, 87, 2098 CrossRef CAS
.
- Y.-F. Wang, G.-Y. Liu, L.-D. Sun, J.-W. Xiao, J.-C. Zhou and C.-H. Yan, Nd3+-Sensitized Upconversion Nanophosphors: Efficient In Vivo Bioimaging Probes with Minimized Heating Effect, ACS Nano, 2013, 7, 7200–7206 CrossRef CAS PubMed
.
- R. D. Shannon, Revised effective ionic radii in halides and chalcogenides, Acta Crystallogr.,
Sect. A: Cryst. Phys., Diffr., Theor. Gen. Crystallogr., 1976, A32, 751–767 CrossRef CAS
.
- J. Xiong, S. Cao, K. Xing, M. Chen, R. Zeng, B. Zou and J. Zhao, Enhanced photoluminescence efficiencies of CsPbCl3−xBrx nanocrystals by incorporating neodymium ions, J. Lumin., 2022, 243, 118658 CrossRef CAS
.
- M. Zeng, F. Locardi, D. Mara, Z. Hens, R. Van Deun and F. Artizzu, Switching on near-infrared light in lanthanide-doped CsPbCl3 perovskite nanocrystals, Nanoscale, 2021, 13, 8118–8125 RSC
.
- J. P. Ma, Y. M. Chen, L. M. Zhang, S. Q. Guo, J. D. Liu, H. Li, B. J. Ye, Z. Y. Li, Y. Zhou, B. Bin Zhang, O. M. Bakr, J. Y. Zhang and H. T. Sun, Insights into the local structure of dopants, doping efficiency, and luminescence properties of lanthanide-doped CsPbCl3 perovskite nanocrystals, J. Mater. Chem. C, 2019, 7, 3037–3048 RSC
.
- M. Stefanski, M. Ptak, A. Sieradzki and W. Strek, Optical characterization of Yb3+:CsPbCl3 perovskite powder, Chem. Eng. J., 2021, 408, 127347 CrossRef CAS
.
- M. Liao, B. Shan and M. Li, In Situ Raman Spectroscopic Studies of Thermal Stability of All-Inorganic Cesium Lead Halide (CsPbX3, X = Cl, Br, I) Perovskite Nanocrystals, J. Phys. Chem. Lett., 2019, 10, 1217–1225 CrossRef CAS PubMed
.
- D. M. Calistru, L. Mihut, S. Lefrant and I. Baltog, Identification of the symmetry of phonon modes in CsPbCl3 in phase IV by Raman and resonance-Raman scattering, J. Appl. Phys., 1997, 82, 5391–5395 CrossRef CAS
.
- S. Smółka, M. Mączka, D. Drozdowski, D. Stefańska, A. Gągor, A. Sieradzki, J. K. ZarÈ©ba and M. Ptak, Effect of Dimensionality on Photoluminescence and Dielectric Properties of Imidazolium Lead Bromides, Inorg. Chem., 2022, 61, 15225–15238 CrossRef PubMed
.
- A. Miguel, J. Azkargorta, R. Morea, I. Iparraguirre, J. Gonzalo, J. Fernandez and R. Balda, Spectral study of the stimulated emission of Nd3+ in fluorotellurite bulk glass, Opt. Express, 2013, 21, 9298–9307 CrossRef CAS PubMed
.
- A. R. Molla, A. Tarafder, S. Mukherjee and B. Karmakar, Transparent Nd3+-doped bismuth titanate glass-ceramic nanocomposites: Fabrication and properties, Opt. Mater. Express, 2014, 4, 843–863 CrossRef CAS
.
- T. H. Q. Vu, B. Bondzior, D. Stefańska and P. J. Dereń, Low-temperature optical thermometer based on the luminescence of the double perovskite Ba2MgWO6: Nd3+, J. Lumin., 2023, 257, 119750 CrossRef CAS
.
- G. Pan, X. Bai, D. Yang, X. Chen, P. Jing, S. Qu, L. Zhang, D. Zhou, J. Zhu, W. Xu, B. Dong and H. Song, Doping Lanthanide into Perovskite Nanocrystals: Highly Improved and Expanded Optical Properties, Nano Lett., 2017, 17, 8005–8011 CrossRef CAS PubMed
.
- M. A. Becker, R. Vaxenburg, G. Nedelcu, P. C. Sercel, A. Shabaev, M. J. Mehl, J. G. Michopoulos, S. G. Lambrakos, N. Bernstein, J. L. Lyons, T. Stöferle, R. F. Mahrt, M. V. Kovalenko, D. J. Norris, G. Rainò and A. L. Efros, Bright triplet excitons in caesium lead halide perovskites, Nature, 2018, 553, 189–193 CrossRef CAS PubMed
.
- M. C. Brennan, J. E. Herr, T. S. Nguyen-Beck, J. Zinna, S. Draguta, S. Rouvimov, J. Parkhill and M. Kuno, Origin of the Size-Dependent Stokes Shift in CsPbBr3 Perovskite Nanocrystals, J. Am. Chem. Soc., 2017, 139, 12201–12208 CrossRef CAS PubMed
.
- W. Wu, W. Liu, Q. Wang, Q. Han and Q. Yang, Temperature-dependent photoluminescence of pure and Mn-doped CsPbCl3 nanocrystals, J. Alloys Compd., 2019, 787, 165–172 CrossRef CAS
.
- A. Ishii and T. Miyasaka, Sensitized Yb3+ Luminescence in CsPbCl3 Film for Highly Efficient Near-Infrared Light-Emitting Diodes, Adv. Sci., 2020, 7, 1903142 CrossRef CAS PubMed
.
- G. Pan, X. Bai, D. Yang, X. Chen, P. Jing, S. Qu, L. Zhang, D. Zhou, J. Zhu, W. Xu, B. Dong and H. Song, Doping Lanthanide into Perovskite Nanocrystals: Highly Improved and Expanded Optical Properties, Nano Lett., 2017, 17, 8005–8011 CrossRef CAS PubMed
.
- X. Zhang, Y. Zhang, X. Zhang, W. Yin, Y. Wang, H. Wang, M. Lu, Z. Li, Z. Gu and W. W. Yu, Yb3+ and Yb3+/Er3+ doping for near-infrared emission and improved stability of CsPbCl3 nanocrystals, J. Mater. Chem. C, 2018, 6, 10101–10105 RSC
.
- P. Dorenbos, Lanthanide 4f-electron binding energies and the nephelauxetic effect in wide band gap compounds, J. Lumin., 2013, 136, 122–129 CrossRef CAS
.
- G. Kresse and J. Furthmuller, Efficient iterative schemes for ab initio total-energy calculations using a plane-wave basis set, Phys. Rev. B: Condens. Matter Mater. Phys., 1996, 54, 11169–11186 CrossRef CAS PubMed
.
- C. Adamo and V. Barone, Toward reliable density functional methods without adjustable parameters: The PBE0 model, J. Chem. Phys., 1999, 110, 6158–6170 CrossRef CAS
.
- B. Kang and K. Biswas, Exploring Polaronic, Excitonic Structures and Luminescence in Cs4PbBr6/CsPbBr3, J. Phys. Chem. Lett., 2018, 9, 830–836 CrossRef CAS PubMed
.
|
This journal is © the Partner Organisations 2024 |
Click here to see how this site uses Cookies. View our privacy policy here.