DOI:
10.1039/D3QM01000G
(Review Article)
Mater. Chem. Front., 2024,
8, 732-768
Recent advances in polyoxometalate-based materials and their derivatives for electrocatalysis and energy storage
Received
11th September 2023
, Accepted 6th November 2023
First published on 8th November 2023
Abstract
The development and implementation of sustainable clean energy have attracted more attention in response to the urgent requirements of environmental pollution and the energy crisis caused by fossil fuels. Consequently, there is a pressing demand for the advancement and implementation of diverse energy conversion and storage technologies to facilitate the generation and utilization of clean energy sources. With the characteristics of reversible redox properties, high thermodynamic and chemical stability, controllable size, and precise structure, polyoxometalates (POMs) have broad application prospects in electrocatalysis and energy storage. Herein, the significant roles of POMs in electrocatalysis and energy storage have been highlighted. Then, the recent advances of POM-based materials and their derivatives in water-splitting, CO2 reduction reactions (CO2RR), the N2 reduction reaction (NRR), supercapacitors (SCs), and rechargeable batteries are systematically summarized. Furthermore, this review discusses the relationship between the catalyst structure and catalytic reaction activity by comparing different electrocatalytic or energy storage systems. Finally, the conclusions and outlooks of POM-based materials and their derivatives in electrocatalysis and energy storage are discussed to promote new progress.
1. Introduction
The excessive consumption of fossil fuels has led to a series of problems such as environmental pollution, climate change, and the global energy crisis, making the exploration of environmentally renewable green energy and the development of clean new energy the main goals of the energy strategy.1 While renewable energy sources such as solar, wind, nuclear, and hydrogen have been extensively explored, their geographical limitations and intermittent nature pose significant obstacles to their application and sustainable development.2,3 If these intermittent renewable energy sources could be converted and stored, this would greatly facilitate their widespread use and enable sustainable energy utilization.
Within this context, researchers have proposed a strategy to achieve sustainable energy utilization through the mutual conversion of electrical energy and chemical energy. Electrical energy can be converted into chemical energy through energy conversion technologies, such as water-splitting to hydrogen,4 CO2 reduction reactions (CO2RRs) to chemical fuels,5 N2 reduction reactions (NRR) to ammonia fuel,6 and so on. However, energy storage systems can realize energy conversion from stored chemical energy to electrical energy, such as supercapacitors (SCs),7 lithium-ion batteries (LIBs),8 metal–air batteries,9 other rechargeable batteries,10etc. In both energy conversion technologies and energy storage systems, a reliable means to improve the efficiency of energy conversion is to develop high-activity and low-cost electrocatalysts or electrode materials that reduce the energy barrier.11
Polyoxometalates (POMs) are a type of metal–oxygen cluster compound, which contain abundant metal sources (Mo, W, V, etc.).12 The basic building unit of POMs is MOx (x = 4, 5, 6), in which M is commonly a high-valent transition metal element such as W, Mo, V, Nb, Ta, etc.13 These transition metal elements can be substituted by metal ions from almost all regions of the periodic table, including the d-, f-, ds-, and p-block. Additionally, the central atoms in POM anions can be substituted by various heteroatoms, including a wide range of metal and non-metal elements, such as Si, P, B, Ge, S, Se, Ga, etc. Therefore, the elemental composition, skeleton structure, valence state, and atomic number of POMs can be fine-tuned and designed,14 which can be ideal candidates for non-precious metal-based electrocatalysts and electrode materials.
Recently, researchers have reviewed the prospects of POM-based materials and their derivatives in electrocatalytic and energy storage applications. Lan et al.15 summarized advances in POM-based materials for water-splitting, CO2 reduction, rechargeable batteries, supercapacitors, and dye-sensitized solar cells. Afterward, Lan et al.16 outlined the progress of POM-based photo/electrocatalysts for application in the HER, OER, and CO2RR. Wei et al.17 summarized a comprehensive overview of POM-contained and POM-derived electrocatalysts for the HER. Song et al.18 reviewed the synthesis, structure modulation, and HER/OER performance of POM/MOF composites and their derivatives. Li et al.19 reviewed POM-based electrocatalysts for electrocatalytic CO2RRs and highlighted the advantages of POMs in CO2RRs. Zang et al.20 summarized POM-based catalysts in electro/photocatalytic CO2RRs and focused on the relationship between the structure and catalytic activity. Liu and Streb21 highlighted the application of POM-SAC in energy-relevant applications, including water-splitting, COx, and N2 activation. Song et al.22 discussed the prepared methods of POM/nanocarbon composite materials and applied them as electrocatalysts or electrodes for LIBs, SCs, and sensors. Pang et al.23 highlighted progress on POM-based materials in fuel cells, Li- and Na-ion batteries, and redox flow batteries. Han et al.24 summarized recent research on POM derivatives for various rechargeable batteries. Zhao et al.25 reviewed the advances of POM composite and POM-based composite materials in the fields of electrochemical detection, electrocatalysis, and energy. Dubal et al.26 provided a review of POM-generated materials for applications in energy devices (SCs, batteries, etc.) and energy catalysts. Fabre et al.27 reviewed the application of POM-functionalized (photo)electrodes in the HER, OER, and CO2RR since the mid-2010s. It should be pointed out that POM-based materials have only been discussed in terms of electrocatalytic or energy storage. Some articles provide a comprehensive overview of POM-based materials, but a detailed overview has not received special attention in the above review. In recent years, POM-based materials and their derivatives have made significant advances in innovative preparation methods and applications. Therefore, it is essential to summarize the recent progress of POMs and their derivatives in electrocatalysis and energy storage.
In this review, the recent research progress on POM-based materials and their derivatives in electrocatalysis and energy storage applications are provided. The focus is primarily on the application of POMs in various electrocatalytic processes such as water splitting, the CO2RR, the NRR, and energy storage including SCs and rechargeable batteries. The advantages of POMs in factors affecting catalytic performance are discussed. Finally, the challenges and outlooks of POM-based materials in electrocatalysis and energy storage are briefly discussed, and we look forward to the development of efficient and controllable POM-based electrocatalytic and electrode materials.
2. Advantages of POMs in electrocatalytic and energy-related devices
In 1826, Berzelius’ research group first discovered hetero-polyacid compound (NH4)3[PMo12O40]·nH2O.28 However, the structure of POMs could not be determined for a long time, leading to stagnation in the development of POM chemistry. It was not until 1934 that Keggin determined the crystallographic structure of H3[PW12O40]·29H2O using X-ray diffraction methods.29 With the advancement of instrument technology, especially single-crystal X-ray crystallography, researchers have discovered hundreds of new POM structures.
POMs have excellent physical and chemical properties including solubility, thermal/chemical stability, acidity, reversible redox ability, high negative charges, electron/proton storage, transport capacities, etc.16,30 Most importantly, these corresponding properties can be tuned by changing the structural dimensions, composition elements, and organic bridging components.16 Thus, the unique properties of POMs lead them to be used in applications of catalysis,31,32 energy,15,33 material,34–36 sensors,37,38 electronic,39 biomedicine,40 radionuclide capture,41 and so on. In addition, significant progress in the innovation of synthesis methods on POM-based materials and POM-derived materials has been achieved, such as an ion-exchanged method,31 oxidative polymerization method,42 supramolecule-confinement pyrolysis (SCP) strategy,43 soft-template approach,44 direct solid reaction,45 encapsulation strategy,46 sol–gel method,47 magnesiothermic reduction approach,48 electrochemical etching technology,49 charge-directed self-assembly method,50 solid-phase hot-pressing method,51 inkjet printing technology,52 interfacial engineering strategy,53 single-molecule confinement strategy,54 and so on.
2.1. Advantages of POM molecules in electrocatalytic and energy-related devices
The main characteristics of POMs are as follows (Scheme 1): (i) clear composition and diverse structures. The composition and structure of POMs are clear, which is helpful to identify the catalytic active sites and reveal the reaction mechanism.55 A single POM molecule can contain 6 to 368 metal ions, and most elements from the periodic table can be assembled into polyoxoanion frameworks. More than 70 different elements can serve as heteroatoms, including many electrocatalytic active elements, which are conducive to regulating the electrocatalytic performance of POM-based electrocatalysts.56 (ii) Adjustable molecular volume. The molecular volume of POMs ranges from a few nanometers to over ten nanometers, which is beneficial for combining POMs with other porous materials, such as MOFs, COFs, etc. The construction of POMOFs improves the stability of POMs in electrocatalytic and energy-related devices. Meanwhile, POMOFs can combine the advantages of POMs and MOFs, which is a promising platform for constructing electrode materials with high capacitance activity and multifunctional ion channels. (iii) Reversible redox properties. POMs can maintain high structural stability while rapidly gaining or losing electrons, which is conducive to improving the efficiency of multi-electron catalytic reactions.57,58 (iv) Adjustable valence state. The same polyoxoanion can exist in both oxidized and reduced states, and undergo color changes during electron gain or loss, which is favorable to energy storage devices like lithium-ion batteries and pseudocapacitors where energy storage and release are based on chemical redox reactions.23,59 (v) The modifiability of the active site. POMs can be applied as flexible scaffolds to assemble POM-based materials through host–guest, hydrogen bonding, electrostatic interaction, or organic modification. Thus, the active sites of POM materials can be regulated according to the characteristics of electrocatalytic and energy-related devices.16 (vi) Easy derivatization. POMs have oxygen-rich surfaces that are easily activated and modified to construct organic–inorganic hybrid and organic derivative materials, making POMs a promising precursor for the development of non-noble metal-based electrocatalytic materials, supercapacitors, and rechargeable battery electrode materials. The infinite molecular structures of POMs give them unparalleled physical and chemical properties, which have been extensively explored for their potential applications in catalysis, water electrolysis for hydrogen production, the CO2RR, NRR, supercapacitors, rechargeable batteries, and other fields.
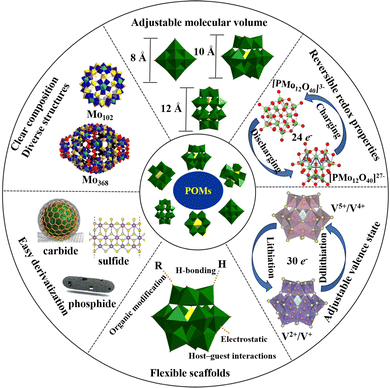 |
| Scheme 1 The main characteristics of POMs. | |
2.2. Advantages of POMs as precursors for preparing electrocatalysts
The synthesis of electrocatalysts using POMs molecules as precursors has the following advantages: (i) the composition of POMs contains a variety of electrocatalytic active metal elements (such as Mo, W, V, etc.), which can be used as a metal source to improve the redox properties of electrode materials;17,60 (ii) heteroatoms in POMs can be doped as heterogeneous elements to improve catalytic performance;61 (iii) POMs have a clear composition and crystal structure, which are easily regulated at the molecular or atomic level.62
3. The research process of POMs for electrocatalytic applications
3.1. Water splitting
As a “zero-carbon” green energy carrier, hydrogen energy has the advantages of high energy density, clean and pollution-free, which is considered to be one of the most promising energy sources to replace traditional fossil energy.63–65 Electrolyzed water hydrogen production technology with the characteristics of being clean and efficient has become the most promising large-scale hydrogen production method.66 Water splitting includes two half-reactions, i.e., the cathodic hydrogen evolution reaction (HER) and anodic oxygen evolution reaction (OER).67 At present, the high performance of HER and OER catalysts are commercial Pt/C and IrO2/RuO2, respectively, but their large-scale application is severely limited due to their low earth abundance, high cost, and poor stability.68 While the efficiency of non-noble metal-based electrocatalysts is lower, which leads to the high cost of hydrogen production from electrolytic water. Therefore, the core task of developing water electrolysis technology is to develop efficient and low-cost electrocatalysts.
3.1.1. POM-based materials for water splitting.
In the past few decades, POM molecular materials have attracted much attention as electrocatalysts for water splitting. Due to the superior redox ability of POMs, one or multiple electrons can be reversibly provided or accepted during the electrochemical process.69 Besides, POMs have many advantages as catalysts for electrocatalysis, as shown in Section 2.1. Thus, POM-based materials are ideal candidates for non-noble metal-based HERs and OERs or bifunctional electrocatalysts.
POM-based HER electrocatalysts.
Keita et al.70 studied the HER activity of POM-modified electrodes in acidic electrolytes by using different POM molecular catalysts ([H7P8W48O184]33−, [Co6(H2O)30{Co9Cl2(OH)3(H2O)9(β-SiW8O31)3}]5− and [{Co3(B-β-SiW9O33(OH))(B-β-SiW8O29OH)2}2]22−). The storage behavior of POMs on protons and electrons can affect the microenvironment of POM-modified electrodes, which significantly affects its HER performance. Afterward, the Cronin group71 made a significant breakthrough in the POM-based water electrolysis system. It can be observed from Fig. 1 that a water separation system was developed using the electron-coupled proton buffer (ECPB) concept. During the process of water oxidation, phosphomolybdic acid (H3PMo12O40) in this system acts as a redox mediator to absorb electrons and protons generated, which results in the production of H2 and O2 occurring in distinct times and spaces. This method effectively avoids the problems of traditional water electrolysis processes, such as product mixing and electrolytic cell degradation. Under the action of the reduction mediator silicotungstic acid (H4[SiW12O40]), the pure hydrogen produced by the platinum catalytic system at the equivalent platinum loading is more than 30 times faster than that of the proton exchange membrane electrolyzer.
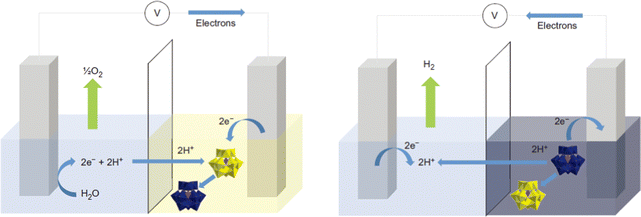 |
| Fig. 1 Schematic of the ECPB-based approach to water splitting.71 Copyright 2013, Springer Nature. | |
Although reduced POMs have been proven to be good HER electrocatalysts, their catalytic performance will be limited due to their small specific surface area and poor conductivity.72 To increase the specific surface area and conductivity of POMs, researchers have combined POMs with larger specific surface area materials including metal–organic framework (MOFs),73 graphene,74 carbon nanotubes (CNTs),75 covalent organic frameworks (COFs),76etc. Nohra et al.77 first reported a POM-based MOF compound (POMOF) for the HER (Fig. 2a) with a turnover frequency (TOF) of 6.7 s−1 at an overpotential of 200 mV. Lan's group78 synthesized NENU-500 and NENU-501, which showed excellent HER performance in acidic electrolytes, as plotted in Fig. 2b. Liu et al.79 prepared a new type of P8W48/rGO nanocomposites by anchoring POM [H7P8W48O184]33− (P8W48) on the 3D structure of reduced graphene oxide (rGO) nanosheets using an electroreduction method (Fig. 2c), which showed excellent HER performance and nearly 100% Faraday efficiency (FE) under acidic conditions. Kortz's group80 prepared nanocomposites of POMs (P2W18, P5W30, and P8W48) and rGO through one-step electroreduction synthesis, and studied the relationship between POMs size, shape, composition, charge, and HER activity. Wei's group81 co-deposited Cu and Anderson-type POM (NiMo6O24) on TiO2 arrays to prepare NiMo6O24@Cu/TNA, and investigated its structure and valence state. It was proven that the POM can regulate and modify the surface morphology of Cu dendrites. In acidic HER, NiMo6O24 as a Brønsted acid, can improve H+ transfer, further adsorb H atoms, make up for the weak H atom adsorption capacity of Cu dendrites, and accelerate the HER rate by combining the strong electron transfer effect of POM molecules.
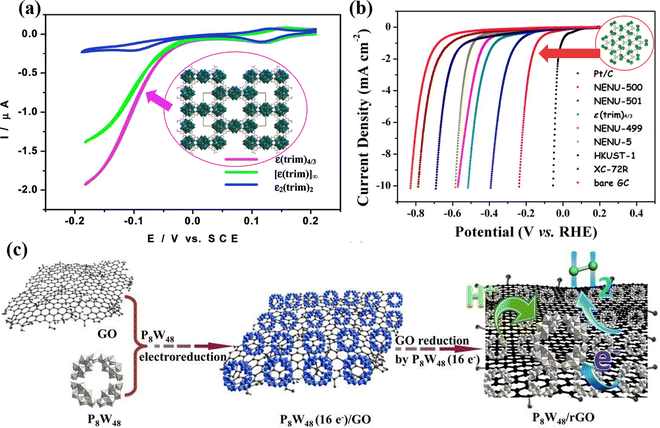 |
| Fig. 2 (a) Comparison of the HER activity of ε(trim)4/3, ε2(trim)2, and [ε(trim)]∞.77 Copyright 2011, American Chemical Society (ACS). (b) Comparison of the HER activity of the NENU-500, NENU-501, and other POMOF catalysts.78 Copyright 2015, American Chemical Society (ACS). (c) Scheme of the synthesis of the P8W48/rGO nanocomposite.79 Copyright 2016, Royal Society of Chemistry (RSC). | |
POM-based OER electrocatalysts.
POM-based OER electrocatalysts also face the problem of poor stability and conductivity of POMs. Thus, the preparation of high-efficient and stable OER electrocatalysts by combining POMs with conductive substrates (such as graphene, CNT, nickel foam, conductive polymers, etc.) has been widely studied.82,83 The effective electron transfer between POMs and conductive substrates is crucial for the OER process of multi-electron transfer. POMs modified by highly redox-active metals (Ru and Co) are the most promising catalysts for OER electrocatalysis.84
Howells et al.85 reported the first POM molecular electrocatalyst Na14[RuIII2Zn2(H2O)2(ZnW9O34)2] for the OER. Toma et al.86 assembled Ru-containing POM clusters (Ru4POM: M10[Ru4(H2O)4(μ-O)4(μ-OH)2(γ-SiW10O36)2], M = Cs, Li) and multi-walled carbon nanotubes (MWCNT) into an efficient and stable OER catalyst (POM/MWCNT). As shown in Fig. 3a, the interaction between the MWCNT carrier and POMs increased the specific surface area and accelerated the electron transfer rate to the electrode, which is beneficial for the improvement of OER performance. Song's group87 deposited POM ([Co6.8Ni1.2W12O42(OH)4(H2O)8]) molecular catalyst microcrystalline on the nickel foam electrode to prepare a NiCo-POM/Ni electrode (Fig. 3b), which has high OER performance and near 100% FE. The NiCo-POM/Ni electrode can work for a long time under alkaline conditions, and the crystal structure of POM remains unchanged (Fig. 3b). Blasco-Ahicart et al.88 reported two water-insoluble cobalt-based POM catalysts: Ba[Co-POM]/CP (Ba8[Co9(H2O)6(OH)3(HPO4)2(PW9O34)3]·55H2O) and Cs[Co-POM]/CP (Cs15K[Co9(H2O)6(OH)3(HPO4)2(PW9O34)3]·28H2O), which have better OER performance and good stability (Fig. 3c), indicating that this type of non-noble metal POMs is expected to replace precious metal catalysts. Cronin's group89 synthesized Co4MoxWy with a sandwich structure via Mo-doped into [Co4(H2O)2(PW9O34)2]10− (Co4W18). As illustrated in Fig. 3c, water can be oxidized at a lower initial potential by adjusting the amount of Mo doping in POMs and controlling the doping sites of Mo.
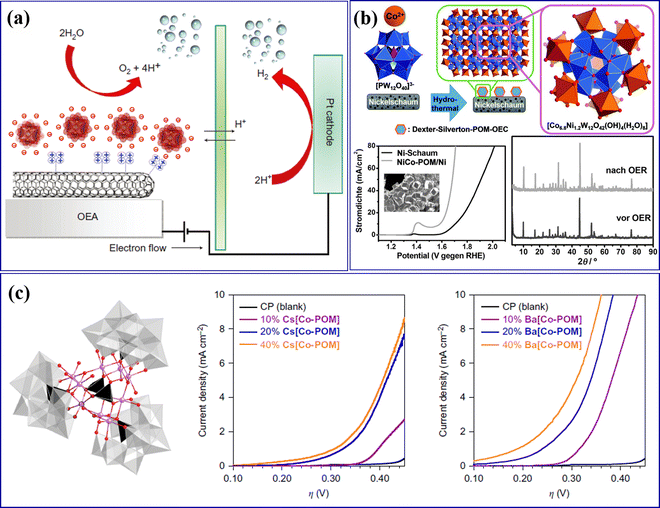 |
| Fig. 3 (a) Scheme for a water-splitting electrocatalytic cell with the integrated nanostructured oxygen-evolving anode of POM/MWCNT.86 Copyright 2010, Macmillan Publishers. (b) Deposition of Dexter–Silverton polyoxometalate microcrystals on nickel foam electrodes with their OER activity.87 Copyright 2017, Wiley-VCH. (c) Molecular structure of the Co-POM cluster and electrochemical behavior of Co-POM/CP electrodes.88 Copyright 2017, Springer Nature. | |
POM-based HER and OER bifunctional electrocatalysts.
Wei's group90 anchored POM molecules (PW12) on NF-based zinc–cobalt sulfide nanowires to prepare a bifunctional self-supporting electrode (POM@ZnCoS/NF), which displayed high HER and OER activity (Fig. 4a and b). The anchored POM nanoparticles increased the number of electrochemically active sites and electrochemically active surface area. Their work showed that the incorporation of POMs has obvious advantages in the design of bifunctional catalysts with low cost and high performance. In addition, Talib et al.91 investigated the effect of single transition metal (M1) loaded on phosphomolybdic acid (PMA) clusters on the HER and OER electrocatalytic performance by density functional theory (DFT) calculation. The volcano diagram calculation showed that V1/PMA, Ti1/PMA, Ru1/PMA, and Pt1/PMA catalysts have good HER performance (Fig. 4c), while Co1/PMA and Pt1/PMA can be used as highly active OER catalysts (Fig. 4d). This study showed that PMA clusters are a promising single-atom support for the HER and OER, providing high-efficiency electrocatalytic activity and low cost.
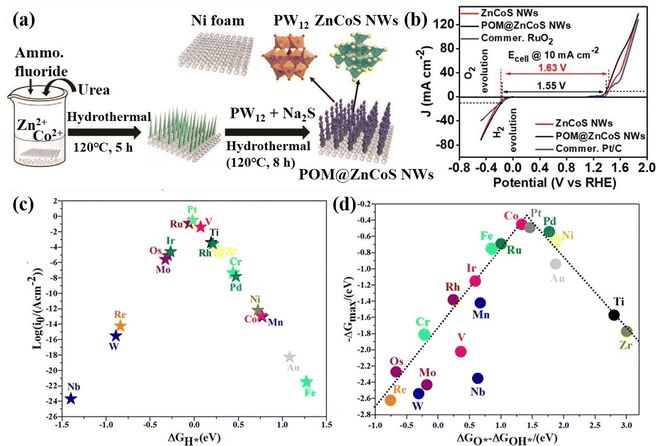 |
| Fig. 4 (a) The synthesis diagram of POM@ZnCoS NWs. (b) Half-cell LSV curves for the OER and HER of the as-prepared electrodes at a scan rate 10 mV s−1.90 Copyright 2021, Wiley-VCH. (c) HER volcano plot and (d) OER volcano plot of the M1/PMA cluster.91 Copyright 2021, American Chemical Society (ACS). | |
3.1.2. POM-derived materials for water splitting.
Although POMs molecular catalysts have made some progress in the field of HER and OER, the application of POMs electrocatalysis is still limited by the solubility and poor stability of POMs in the reaction medium.16 In recent years, the preparation of POM-derived materials via thermal conversion technology using POM molecules as precursors has attracted wide attention. Pyrolysis of POMs (such as carbonization, nitridation and phosphating) in an inert atmosphere is an effective method to enhance the activity and stability of POM-based electrocatalysts.15 Heteroatom-doped and carbon-coated composites can be prepared via annealing the mixture of heteroatom-doped POMs and carbon sources. During the pyrolysis treatment process, the carbon source is converted into a graphitized carbon layer wrapped on the surface of the catalyst, which can improve the surface area and conductivity of the catalyst. Carbon-coating can protect the metal species from corrosion in a harsh electrolyte environment.92 Additionally, doping heteroatoms (such as N, P, S, etc.) can adjust the surface properties of the carbon layer and increase the electron density of the carbon layer.93 Zou's group94 used soluble small-sized POMs (ammonium molybdate) as the Mo source and dicyandiamide (DCA) as the small-molecule organic carbon source. After mixing and calcining them under an Ar atmosphere, ultra-small molybdenum carbide nanoparticles (Mo2C@NC) with N-doped and C-coated were prepared. The nanoparticles have high HER activity in all-pH electrolytes. Similarly, Li's group prepared nano-sized HER electrocatalysts P-W2C@NC,95 MoP/Mo2C@C,96 CoMoP@C,97 TM-Mo2C@C,98 and Co2P/WC@NC99 by mixing Mo-based or W-based POMs with DCA and carbonization, as well as preparing POM-derived HER electrocatalysts by pyrolysis of POMs with other carbon materials, such as MoCx@C,100 Ni/WC@NC,101 P-Mo2C@NC,102 and MoPS/NC.103
In addition, Lan's group used phosphomolybdic acid and phosphotungstic acid as POM sources assembling with polypyrrole (PPy) and rGO into nanocomposites, namely PMo12-PPy/rGO and PW12-PPy/rGO. Then, further calcined PMo12-PPy/rGO and PW12-PPy/rGO under a N2 atmosphere to synthesize N, P co-doped and C-coated carbide catalysts: Mo2C@NPC/NPRGO104 (Fig. 5a) and NC@WxC/NRGO105 (Fig. 5c). It can be seen from Fig. 5b and d that both of these materials exhibit a HER performance comparable to 20% Pt/C. Wei's group106 designed and synthesized the N-doped molybdenum phosphide and molybdenum carbide composite catalyst N@MoPCx using benzene imine-derivatized POMs (Mo4O(ArPO3)2(NAr)4(μ2-NAr)5, Ar = phenyl, Mo4-CNP) as the precursor under the high-temperature pyrolysis in inert gas (Fig. 5e). Benzenimine and phenyl phosphonic acid ligands as N, C, and P sources can be doped, carbonized and phosphatized at the same time. The organic ligand can in situ generate substrate carbon during the pyrolysis process, which was beneficial to enhance the conductivity and stability of the catalyst. The composite material exhibits excellent HER activity and stability. Afterward, the N-implanted Mo2C (N@Mo2C-n/CFP) was prepared by pyrolysis organoimido-derivatized POM that can directly adjust the amounts of N atoms in N@Mo2C-n/CFP107 (Fig. 5f). This design of the molecular structure of POMs to achieve in situ doping is another effective method for preparing high-efficiency POM-based composite catalysts. Wang's group108 through a high-temperature calcination process synthesized the Co/WC@NC electrocatalyst using bimetallic POMs ([Co9(OH)3(H2O)6(HPO4)2(PW9O34)3]16−) as soft templates and polyethyleneimine (PEI) as a carbon source. The Co/WC@NC displayed high HER activity and stability in both acidic and alkaline electrolytes mainly attributed to the synergistic effect of Co nanoparticles with WC, and the protective effect of the graphitized carbon layer.
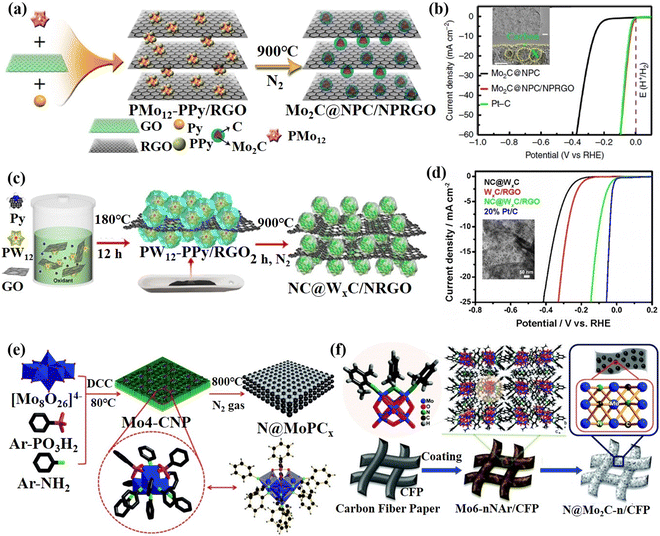 |
| Fig. 5 (a) The synthetic process and (b) HER activity of Mo2C@NPC/NPRGO.104 Copyright 2016, Nature. (c) The preparation process and (d) HER activity of NC@WxC/NRGO.105 Copyright 2017, Wiley-VCH. (e) The synthetic procedure for the preparation of N@MoPCx.106 Copyright 2017, Wiley-VCH. (f) A schematic illustration of the synthesis of N@Mo2C-n/CFP.107 Copyright 2017, Wiley-VCH. | |
Furthermore, POM-derived catalysts can also be prepared by pyrolysis of POM precursors in a reducing atmosphere. Fu's group109 prepared a P-doped WN/rGO composite catalyst by further phosphating the composite of phosphotungstic acid and graphene after annealing in an NH3 atmosphere (Fig. 6a and b). Owing to the P doping and the interaction between rGO and WN, the catalyst has high HER performance, as plotted in Fig. 6c and d. In addition, Tan's group110 adopted a molecular-to-cluster strategy to calcine the mixture of POM molecules ([{Co4(OH)3PO4}4(SiW9O34)4]32−, [{Fe2Co2(OH)3PO4}4(SiW9O34)4]24−, and [{FeCo3(OH)3PO4}4(SiW9O34)4]28−) and EDA-C60 under a H2/Ar atmosphere to prepare ultra-small transition metal clusters (TMC: 1-CoW, 2-CoFeW, and 3-CoFeW) with controllable chemical composition (Fig. 6e–g). The catalytic performance of TMC was fine-tuned by surface doping, which can solve the adsorption performance of the reaction intermediate on the surface of the transition metal clusters (Fig. 6h). As shown in Fig. 6i, the prepared 3-CoFeW exhibited high OER performance and stability in alkaline media.
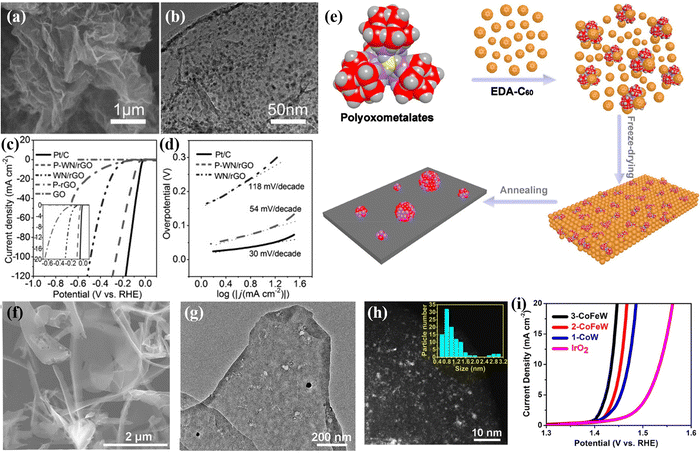 |
| Fig. 6 (a) SEM image and (b) TEM image of P-WN/rGO; (c) LSV curves and (d) Tafel plots for the as-prepared electrodes.109 Copyright 2015, Wiley-VCH. (e) Schematic illustration of synthesizing the TMCs; (f) SEM image, (g) TEM image, and (h) HAADF-STEM image of 1-CoW; (i) OER curves of catalysts loaded on carbon cloth.110 Copyright 2018, American Chemical Society (ACS). | |
Although the above methods can effectively improve the activity of POM-derived electrocatalysts, there still exist some problems. For example, POM molecules, especially transition metal-substituted POMs molecules, tend to form multiphase mixed structures after annealing treatment, which may lead to unclear active sites. In addition, high-temperature pyrolysis treatment requires large energy consumption, which is contrary to the concept of green sustainability. At present, the preparation of POM-derived materials using a mild hydrothermal synthesis method can take advantage of the solubility of most POMs and meanwhile can save energy. In addition, researchers have further improved the performance of POM-derived materials through various regulation methods, such as a surface reconstruction strategy, interface engineering strategy, defect construction strategy, and atom doping strategy, single-atom catalysts (SACs) strategy, etc.
Surface reconstruction strategy.
During the alkaline electrocatalytic process, the oxides, sulfides, nitrides, and phosphides of the later transition metal (Fe, Co, Ni, etc.) are prone to surface reconstruction, and the corresponding hydroxides or hydroxyl oxides are derived and participate in the catalytic reaction as true active species.111 While the existence of high valence states former transition metals (Mo, W, etc.) can accelerate the occurrence of surface reconstruction. Hence, exploring the surface reconstruction of electrocatalysts has important significance for understanding the structure–activity relationship of catalysts and developing high-performance catalysts. Lan's group112 reported a P-NiMo4N5@Ni self-supporting electrode with controllable morphology through hydrothermal and phosphating treatment (Fig. 7a). It is worth noting that PMo12 with water solubility and acidity can moderately dissolve a small amount of Ni on the NF surface, which was conducive to the uniform growth of a nano-array structure on NF. Meanwhile, heteroatom P in PMo12 was introduced into the electrode material. After the OER stability test, the surface of the P-NiMo4N5@Ni electrode oxidized to OER active species such as metal hydroxides or hydroxyl oxides. As displayed in Fig. 7b, the P-NiMo4N5@Ni showed high HER, OER, and water-splitting performance. Zhang et al.49 prepared a CoMoxO4@CP self-supporting electrode by depositing PMo12 and Co salt on carbon paper (CP). During the electrochemical activation process (Fig. 7c), the electrode surface undergoes continuous structural evolution due to the etching effect of MoO42−, resulting in a significant increase in OER activity (Fig. 7a and e). Kou et al.113 synthesized a Co–Mo2C material as an OER pre-catalyst by anion exchange reaction of Co in Co-MOF with MoO42− in POMs. During the OER process, the metal Co phase rapidly reconstructed into γ-CoOOH enriched with Mo, which significantly improved the catalytic activity (Fig. 7f). Through various in situ characterization analyses, the process of electron interaction was summarized as shown in Fig. 7g.
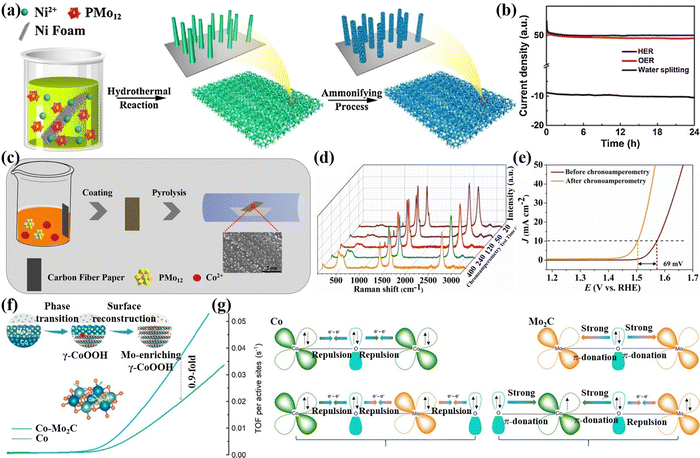 |
| Fig. 7 (a) The synthetic process of P-NiMo4N5@Ni composite. (b) Chronoamperometric curves of P-NiMo4N5@Ni-1 for the HER, OER, and water splitting.112 Copyright 2018, Elsevier B.V. (c) The synthetic process of CoMoxO4@CP (x = 0.3, 1 or 3). (d) In situ Raman spectra with the increase of the chronoamperometry test time. (e) LSV measured before and after chronoamperometry test.49 Copyright 2019, Elsevier B.V. (f) The process of phase transition of Co–Mo2C to Mo-enriching γ-CoOOH. (g) Schematic representation of the electronic coupling between Co and Mo in the post-OER products of Co, Mo2C, and Co–Mo2C heterostructure.113 Copyright 2020, American Chemical Society (ACS). | |
Interface engineering strategy.
Interface engineering has important significance for improving the performance of heterostructure electrocatalysts, especially for electrocatalytic reactions involving various reaction intermediates such as the alkaline HER and OER.114 Heterostructure electrocatalysts with rich interfaces exhibit obvious kinetic acceleration behavior for alkaline HER or OER owing to the synergistic effect of different components, then balance the adsorption or desorption behavior of intermediates at the interface.115,116 Interface engineering can effectively adjust the electronic structure of active sites by means of electronic interaction,117 interface bonding,118 and lattice strain,119 thus optimizing the binding energy of target intermediates. Furthermore, the confinement effect of the composite interface to maintain the high density of active sites of the catalyst is essential for improving stability.120
Heteropolyoxometalates are composed of fixed-proportion heteroatoms and coordination atoms. Thus, applying POMs as precursors is beneficial to the construction of multi-interface catalysts. Sun's group121 successively prepared bimetallic sulfide and carbon cloth composite electrocatalysts (oxygenated–CoS2–MoS2) using the hydrothermal method to sulfurize Anderson-type POMs ((NH4)4[CoIIMo6O24H6]·6H2O). Owing to the exposure of active heterointerfaces, and the facilitated charge transport, the overall water-splitting performance of oxygenated–CoS2–MoS2 arrays delivered 10 mA cm−2 at 1.6 V (Fig. 8a). Lu et al.122 constructed multiple interface-regulated CoS2–MoS2 heterojunction catalysts (Co2Mo10Sx/CC) at the atomic level using Evans–Showell-type POM (Co3[Co2Mo10O38H4]) as a molecular pre-assembly platform using a hydrothermal method. Due to the electronic interaction between the active component CoS2 and MoS2, the active center reconstructed into the CoMoS site through the interface effect (Fig. 8b). Compared with the single synthesized Co2Mo10Sx/CC, the Co2Mo10Sx/CC electrode derived from POMs showed higher over water-splitting activity (Fig. 8c). Experiments combined with theoretical calculations showed that its excellent electrocatalytic activity was attributed to the improved intrinsic catalytic activity of CoMoS sites, the synergistic effect of heterostructures, and the heterogeneous interface with defect-rich sites. Hou et al.123 used a POM-based functional material ([(1,2,4-triazole)3Co2·6H2O][H3GeMo12O40]·9H2O) as a bimetallic precursor for hydrothermal sulfidation, and finally generated a CoS2@MoS2 nanoarray catalyst with a multiple interface structure. Due to the high crystallinity of POMs and the fixed-proportion of bimetallic sources, the synthesized CoS2@MoS2@CC-30 h electrode material displayed high electrocatalytic HER activity in a wide pH range (Fig. 8d and e). Based on the advantages of POMs and multi-interface component catalysts, Zhang et al.53 used an Anderson-type POM (NH4)4[FeMo6O24H6]·6H2O (FeMo6) as a bimetallic precursor to prepare the Fe–Mo–S/Ni3S2@NF electrode with a multi-interface using a one-step hydrothermal method (Fig. 8f). DFT calculations showed that HER activity was mainly provided by the Fe3S4/Ni3S2(003) and Ni3S2(003)/Mo3S13 interfaces (Fig. 8g), while the Fe3S4/Ni3S2(110) interface provided a high OER performance (Fig. 8h). Thereby, the Fe–Mo–S/Ni3S2@NF electrode showed high HER, OER, and overall water-splitting performance, as shown in Fig. 8i.
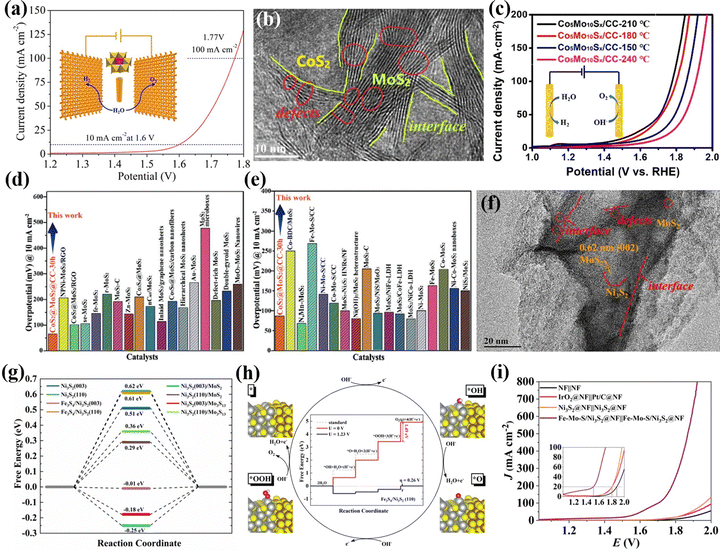 |
| Fig. 8 (a) Overall water splitting performance through the use of O–CoMoS array as a perspective anode and cathode.121 Copyright 2018, American Chemical Society (ACS). (b) HRTEM images of Co5Mo10Sx@CC. (c) Overall water-splitting performance of Co5Mo10Sx@CC.122 Copyright 2020, Elsevier B.V. A comparison of overpotentials in CoS2@MoS2@CC-30 h and the reported MoS2-based electrocatalysts at 10 mA cm−2 in (d) 0.5 M H2SO4 and (e) 1.0 M KOH electrolyte.123 Copyright 2020, Wiley-VCH. (f) HRTEM images with different magnifications of Fe–Mo–S/Ni3S2@NF. (g) HER free energy changes on reaction surfaces. (h) Free energy diagrams for the OER process at the Fe3S4/Ni3S2(110) interface. (i) Overall water-splitting performance of Fe–Mo–S/Ni3S2@NF.53 Copyright 2020, Elsevier B.V. | |
Defect construction strategy.
Defects can affect the electronic structure, surface and interface properties, thereby changing the original distribution to adjust the adsorption energy of the intermediate.124 In addition, some defects can act as electrocatalytic active sites, such as vacancies, unsaturated coordination sites, and lattice defects to enhance the catalytic activity of the materials.125 Thus, the reasonable construction of defects is helpful to adjust and optimize the electrocatalytic performance of POM-based materials. Xie's group126 synthesized MoS2 ultrathin nanosheets with defect-rich structures by using ammonium molybdate as a Mo source with excessive thiourea under hydrothermal conditions (Fig. 9a). The MoS2 nanosheets with a rich defect structure could expose more edge active sites, which exhibited excellent HER performance, as plotted in Fig. 9b. Lan's group127 combined PMo12 that has good water solubility and strong redox properties with GO to prepare MoS2/N-RGO composite with defects after the sulfurization process (Fig. 9c and d). Due to electrostatic repulsion, PMo12 particles can be uniformly dispersed between GO layers. Meanwhile, GO can be reduced to RGO owing to the strong reducibility of PMo12. The MoS2/N-RGO composite displayed excellent HER performance under acidic conditions. Tang et al.128in situ prepared a defective CoMoS/KB nanocluster catalyst (Fig. 9e) by using small and uniform sizes of reduced POMs ((NH4)3PMo12O40) as the template and anchor sites of metal salts, which was beneficial to expose more edge active sites. Therefore, the CoMoS/KB catalyst has excellent activity and stability for the HER. In summary, the construction of surface defects provides a solution to further improve the catalytic activity.
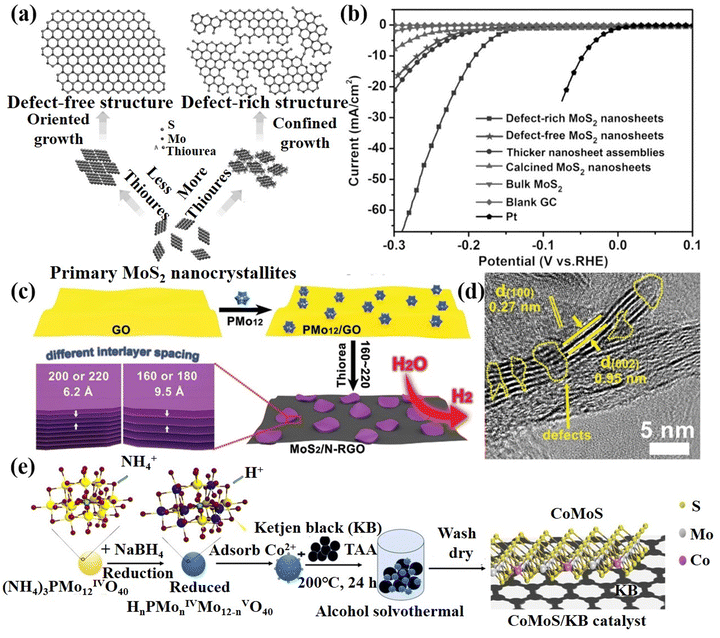 |
| Fig. 9 (a) Structural models and synthetic path diagrams of defect-free and defect-rich structures; (b) LSV curves of various samples as indicated.126 Copyright 2013, Wiley-VCH. (c) The schematic preparation process of MoS2/N-RGO-T nanocomposite; (d) HRTEM of MoS2/N-RGO-180.127 Copyright 2016, Wiley-VCH. (e) Schematic illustration of the synthesis of the CoMoS/KB catalyst.128 Copyright 2021, American Chemical Society (ACS). | |
Doping control strategy.
Heteroatom doping can evidently increase the intrinsic conductivity of the catalyst, regulate the electronic structure, and optimize the adsorption and desorption of intermediates on the catalyst surface, which play a key role in improving the electrocatalytic performance.129 Heteroatom doping can be roughly divided into non-metal atom doping (such as N, P, S, O, etc.) and metal atom doping (such as Fe, Co, Ni, Zn, etc.). Among them, non-metallic atom doping can enhance the electronic structure at the catalytic active sites to effectively adjust the work function and increase the adsorption of the reactants at a specific position, thereby improving their electrocatalytic activity.130 Metal doping can accelerate the electron transfer in different sites through the synergistic effect between metal atoms and other atoms, then reduce the energy barrier of the reaction intermediate.131
Heteropolyoxometalates have inherent heteroatoms, which can provide various doped heteroatoms (such as P, W, Mo, Ni, Co, Fe, etc.) for the final electrocatalyst. These heteroatoms can enhance the electrochemical performance through empty orbits or lone pair electrons.132 Cronin's group89 synthesized Co4MoxWy containing a Mo and W mixed sandwich structure through doping Mo into Co4W18, as shown in Fig. 10a. Adjusting the amount of Mo doping in POMs could control the doping sites of Mo, which oxidized water at a lower onset potential without degradation of the catalyst. The OER overpotential of 3b[Cs] was 188 mV lower than that of the pure tungsten skeleton (Fig. 10b). Their work provided a strategy for preparing molecular heterogeneous catalysts by using Mo-doped POM clusters to improve water oxidation performance, and also opens up new ideas for the development of POM-based electrolyzed water catalysts via similar design principles. Zhang et al.61 synthesized a Fe–S–NiMoO4/MoO3@NF self-supporting electrode using FeMo6 as a precursor through a one-step solvothermal method, as illustrated in Fig. 10c. The FeMo6 precursor could accurately control the doping ratio of Fe in Fe–S–NiMoO4/MoO3@NF. The in situ Raman experiments (Fig. 10d–f) on the optimal Fe–S–NiMoO4/MoO3@NF sample in the electrolyte temperature range of 25–80 °C showed that the improvement of OER activity was due to the formation of more OER active phases, and finally achieved a quasi-industrial OER performance (Fig. 10g).
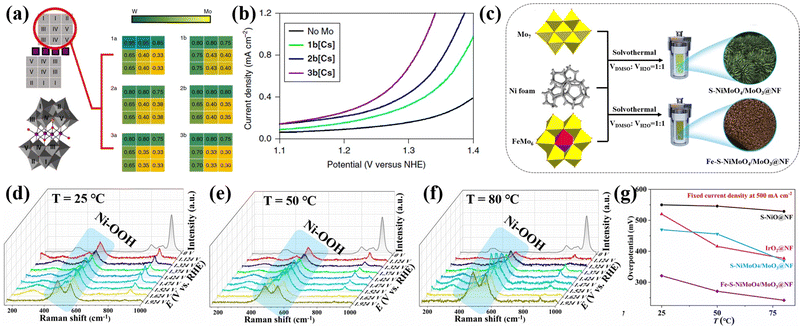 |
| Fig. 10 (a) Crystallographic data for the six compounds of Co4MoxWy; (b) water oxidation activity of 1b[Cs]–3b[Cs] compounds.89 Copyright 2018, Springer Nature. (c) Schematic map of the synthesis of Fe–S–NiMoO4/MoO3@NF; In situ Raman spectra of Fe–S–NiMoO4/MoO3@NF during the OER process at different electrolysis temperatures: (d) 25 °C, (e) 50 °C, and (f) 80 °C; (g) Comparison of the requirement overpotential of the prepared electrodes at 25–80 °C at 500 mA cm−2.61 Copyright 2021, Elsevier B.V. | |
In addition to single-atom doping, multi-atom doping is also an effective way to enhance catalytic performance. Wei's group133 prepared 1T-MoS2 co-doped with different TMs and O atoms, namely NiO@1T-MoS2, CoO@1T-MoS2, and FeO@1T-MoS2 using Anderson-type POMs that contain different heteroatoms as precursors for electrochemical HERs, as shown in Fig. 11a. It can be observed from the HAADF-STEM image in Fig. 11b that NiO@1T-MoS2 showed a hexagonal intensity change, which was consistent with the single-layer of 1T-MoS2 reported by Eda, G. et al.134 While the strength of Ni centers and Mo atoms in all regions of the sample have changed (Fig. 11c), indicating that the hexagonal units of NiMo6 were well retained in the final NiO@1T-MoS2 nanosheets, which avoided the agglomeration problem that may be caused by direct doping of metal atoms. Experimental and theoretical studies have shown that Ni and O atoms were simultaneously doped into ultrathin 1T-MoS2 nanosheets, which could effectively regulate the electronic structure, and also provided more catalytic active sites, reducing the energy barrier required for hydrolysis. Zhang et al.135 prepared P and Mo co-doped Ni3S2 (P–Mo–Ni3S2@NF) using PMo12 as the dopants through a theory-guided strategy (Fig. 11d). As presented in Fig. 11e, the P–Mo–Ni3S2@NF electrode showed a decent performance of urea assisted electrolytic water.
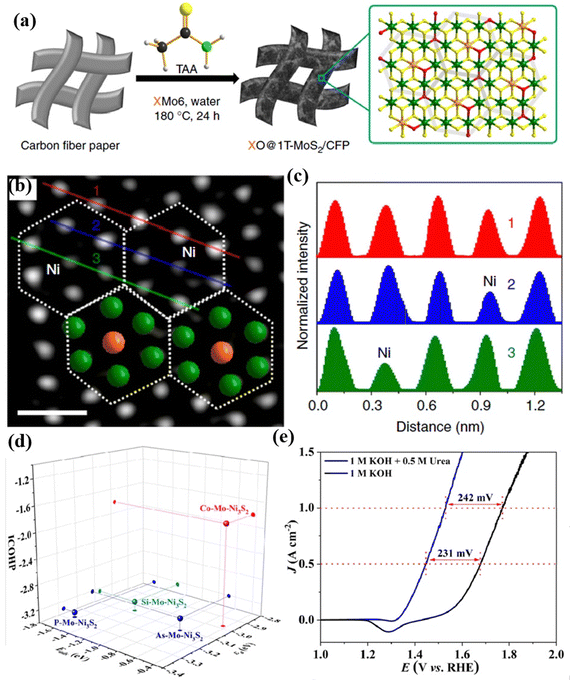 |
| Fig. 11 (a) The preparation process of XO@1T-MoS2/CFP; (b) aberration-corrected atomic resolution HAADF-STEM image (scale bar: 0.5 nm); (c) intensity profiles along the lines indicated in (b).133 Copyright 2019, Springer Nature. (d) A comparison of Eads(urea), εd, and ICOHP values. (e) A comparison of the UOR and OER activity of P–Mo–Ni3S2@NF.135 Copyright 2023, Royal Society of Chemistry (RSC). | |
Single-atom catalyst (SAC) strategy.
A single-atom catalyst can be used to stabilize a single independent atom on the carrier, which has the advantage of clear active sites of homogeneous catalysts and recyclable heterogeneous catalysts.136 POMs are ideal molecular systems for exploring SAC anchoring,21 which can greatly help to avoid the agglomeration of metal parts during the synthesis process.17 Li's group137 used POMs (PtW6, PtMo6, PtV9 and PtSiW11) as Pt-based SAC models, and the prepared {PtW6}/C has excellent electrocatalytic HER activity. This work clearly explains the structure–activity relationship of the electrocatalytic HER of SAC Pt for the first-time using POMs as a model. Specifically, the catalytic activity of Pt SACs electrocatalysts is affected by both the metal–oxide support and the coordination environment of Pt single atom active sites (Fig. 12a). The coordination environments of Pt single atoms were similar in the models of {PtW6}, {PtMo6} and {PtV9}, and the electron acquisition of the Pt single atom was affected due to the different electron affinity of the metal–oxide support carrier, which led to the different effective potential and catalytic activity of the Pt center. Whereas in the POM molecular models of {PtSiW11} and {PtW6}, the Pt single atoms exhibited similar metal–oxide supports, the different coordination environment of single atom Pt in the two POM models resulted in different catalytic reaction pathways, thus affecting the final HER activity (Fig. 12b). Zhang's group138 fabricated Pt1–Mo2C–C catalyst derived Pt-POMs (Fig. 12c), which exhibited high activity and stability in the HER. Talib et al.139 investigated the HER, OER, and ORR performance of M1/PW12O40 single-atom electrocatalysts via DFT calculation. The above studies demonstrated that POM-based SACs display the advantages of performance improvement in electrocatalysis. The following points can be summarised. (i) Due to the high stability and electron-rich properties of POM clusters, the POM-based SACs can effectively adjust the electronic properties of SAC active sites, promote electron transfer between SAC active sites and existing support, which can be the key factor in improving the catalytic efficiency. (ii) The overall catalytic activity of the POM-based SACs can be attributed to the synergy between all the metal sites in the framework. (iii) The interaction between SACs sites and POM clusters is usually stabilized by coordination bonds or covalent coordination bonds, which will prevent the aggregation and leaching of SAC active sites in the catalytic reaction process, thus enhancing the stability. The HER and OER performances of the POM-based materials and POM-derived materials in this review are shown in Table 1 and Table 2, respectively.
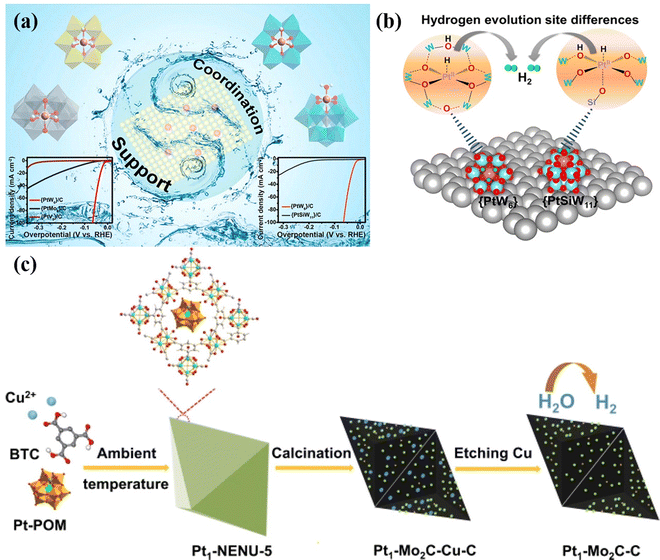 |
| Fig. 12 (a) Schematic illustration of Pt-based SAC models and HER performance. (b) Different hydrogen evolution sites responsible for the {PtW6} and {PtSiW11}.137 Copyright 2021, American Chemical Society (ACS). (c) Schematic illustration of the formation of Pt1–Mo2C–C.138 Copyright 2021, ELSEVIER B.V. | |
Table 1 Comparison of the POM-based and POM-derived materials in this review for HER performance
Electrocatalysts |
Electrolyte |
j (mA cm−2) |
η (mV) |
Tafel slope (mV dec−1) |
Ref. |
NENU-500 |
0.5 M H2SO4 |
10 |
237 |
96 |
78
|
NENU-501 |
0.5 M H2SO4 |
10 |
392 |
137 |
78
|
P8W48/rGO |
0.5 M H2SO4 |
10 |
28 |
38 |
79
|
P2W18@rGF_ox |
0.5 M H2SO4 |
10 |
35 |
37 |
80
|
P5W30@rGF_ox |
0.5 M H2SO4 |
10 |
33 |
33 |
80
|
P8W48@rGF_ox |
0.5 M H2SO4 |
10 |
44 |
41 |
80
|
NiMo6O24@Cu/TNA |
0.5 M H2SO4 |
10 |
215 |
89.2 |
81
|
POM@ZnCoS/NF |
1 M KOH |
10 |
170 |
53.5 |
90
|
Mo2C@NC |
0.5 M H2SO4 |
10 |
124 |
60 |
94
|
P-W2C@NC |
0.5 M H2SO4 |
10 |
89 |
53 |
95
|
MoP/Mo2C@C |
0.5 M H2SO4 |
10 |
89 |
45 |
96
|
CoMoP@C |
0.5 M H2SO4 |
10 |
41 |
50 |
97
|
Ni–Mo2C@C |
0.5 M H2SO4 |
10 |
72 |
65.8 |
98
|
Co2P/WC@NC |
0.5 M H2SO4 |
10 |
91 |
40 |
99
|
MoCx@C |
0.5 M H2SO4 |
10 |
79 |
56 |
100
|
Ni/WC@NC |
0.5 M H2SO4 |
10 |
53 |
43.5 |
101
|
P-Mo2C@NC |
0.5 M H2SO4 |
10 |
109 |
76 |
102
|
MoPS/NC |
0.5 M H2SO4 |
10 |
120 |
52 |
103
|
Mo2C@NPC/NPRGO |
0.5 M H2SO4 |
10 |
34 |
33.6 |
104
|
WxC@NC/NRGO |
0.5 M H2SO4 |
10 |
100 |
58.4 |
105
|
N@MoPCx-800 |
0.5 M H2SO4 |
10 |
108 |
69.4 |
106
|
N@Mo2C-3/CFP |
0.5 M H2SO4 |
10 |
56 |
51 |
107
|
Co/WC@NC |
0.5 M H2SO4 |
10 |
129 |
93 |
108
|
Co/WC@NC |
1 M KOH |
10 |
142 |
91 |
108
|
P-WN/rGO |
0.5 M H2SO4 |
10 |
85 |
54 |
109
|
O-CoMoS |
1 M KOH |
10 |
97 |
70 |
121
|
CoS2@MoS2@CC-30 h |
0.5 M H2SO4 |
10 |
65 |
122 |
123
|
Fe–Mo–S/Ni3S2@NF |
1 M KOH |
10 |
141 |
123 |
53
|
defect-rich MoS2 |
0.5 M H2SO4 |
13 |
200 |
50 |
126
|
MoS2/N-RGO-180 |
0.5 M H2SO4 |
10 |
56 |
41.3 |
127
|
CoMoS/KB |
1 M KOH |
10 |
81 |
64 |
128
|
NiO@1T-MoS2 |
1 M KOH |
10 |
46 |
52 |
133
|
{PtW6}/C |
0.5 M H2SO4 |
10 |
22 |
29.8 |
137
|
Pt1–Mo2C–C |
1 M KOH |
10 |
155 |
64 |
138
|
Table 2 Comparison of the POM-based and POM-derived materials in this review for OER performance
Electrocatalysts |
Electrolyte |
j (mA cm−2) |
η (mV) |
Tafel slope (mV dec−1) |
Ref. |
NiCo-POM/Ni |
0.1 M KOH |
10 |
360 |
126 |
87
|
Cs[Co-POM] |
1 M H2SO4 |
10 |
466 |
98 |
88
|
Ba[Co-POM] |
1 M H2SO4 |
10 |
361 |
97 |
88
|
POM@ZnCoS/NF |
0.5 M H2SO4 |
10 |
200 |
67.7 |
90
|
3-CoFeW |
1 M KOH |
10 |
192 |
36 |
110
|
P-NiMo4N5@Ni-1 |
1 M KOH |
100 |
297 |
33.6 |
112
|
Mo1–CoOOH@CP |
1 M KOH |
10 |
274 |
66 |
49
|
Co–Mo2C |
1 M KOH |
10 |
190 |
94.3 |
113
|
O-CoMoS |
1 M KOH |
10 |
272 |
45 |
121
|
Co5Mo10Sx/CC |
1 M KOH |
10 |
153 |
57.8 |
122
|
Fe–Mo–S/Ni3S2@NF |
1 M KOH |
100 |
300 |
98 |
53
|
Fe–S–NiMoO4/MoO3@NF |
1 M KOH |
10 |
212 |
41 |
61
|
3.2 CO2 reduction reaction
Recently, the excessive consumption of fossil fuels has led to a large release of CO2 into the atmosphere, exacerbating the greenhouse effect and disrupting the natural carbon balance.140 In this context, there is an urgent demand to explore effective technologies for reducing the concentration of CO2 in the atmosphere. Considering that CO2 is an essential C1 resource for various industrial processes and plant growth, the rational conversion of CO2 into high-value chemicals has emerged as a clean carbon-neutral strategy.141 This approach not only addresses environmental issues caused by CO2 but also offers a prospective means to meet future sustainable energy development. Structurally, CO2 molecules are linear and centrally symmetric, characterized by high stability, a large energy gap, and low electron affinity,142,143 making the activation of CO2 a challenging process that requires significant energy input and stringent reaction conditions. Due to the environmental compatibility and mild reaction conditions, CO2 reduction reactions (CO2RRs) have gained widespread attention, demonstrating the enormous potential for future industrial applications.5,144 Nevertheless, the unregulated interaction between electrons and protons during the CO2RR has resulted in the emergence of multiple coupling processes, leading to a diverse range of reaction pathways. Consequently, the CO2RR often yields numerous distinct products, ultimately resulting in low FE and selectivity.145 To date, various types of catalyst materials have been developed and applied in the CO2RR.146–148 Although these catalysts have shown good CO2RR activity and selectivity, the sources of catalyst activity and the mechanisms for controlling selectivity are still unclear. A deeper understanding of the reaction mechanism and process of the CO2RR requires further research. Therefore, constructing well-defined heterogeneous CO2RR model catalysts is an important approach to elucidating the reaction mechanism of the CO2RR and designing efficient heterogeneous catalysts for the CO2RR.
3.2.1. POM-based molecular materials for the CO2RR.
POMs have unique redox properties, excellent electron/proton storage and transport capabilities, and show significant potential in the photocatalytic and electrocatalytic reduction of CO2.19 In addition, POMs possess abundant surface oxygen atoms, which exhibit Lewis basicity and can serve as potential active centers for CO2 molecule activation and coupling.149 Xu's group150 demonstrated that metal atom-substituted heteropolyanions ((C3H5N2)3(C3H4N2)[PMo11CoO38(CO2)]·4H2O and (C3H5N2)4[SiMo11CoO38(CO2)]·4H2O) can undergo covalent interactions with CO2 molecules through X-ray single-crystal diffraction. The bond angle of the covalently bonded CO2 molecule showed a slight deviation, bending from 180° to 158.7°. This indicates that the metal atoms in the POMs clusters can immobilize and activate CO2 molecules. Accordingly, POMs possess a well-defined structure and the potential to adsorb and activate CO2, making them suitable as a structural model for comprehending the crucial stages involved in the molecular-scale CO2RR reaction process.
The initial investigation into the catalytic activity of POMs in the CO2RR was studied by Kozik et al.151 As illustrated in Fig. 13a–d, a series of spectroscopic experiments were applied to demonstrate the formation of POM–CO2 complexes. Kozik et al.152 prepared the first POMs catalyst, α-[SiW11O39Co]6− (SiW11Co), capable of electrochemically catalyzing the CO2RR. Compared to the CV curve of SiW11Co in dichloromethane (CH2Cl2) electrolyte bubbled without CO2, the CV curve of SiW11Co in CH2Cl2 electrolyte with CO2 exhibited a higher reduction in current signal, suggesting that SiW11Co possesses high CO2RR activity. The CV curve of α-[P2W18O62]6− (P2W18) without TM substitution remained largely unchanged in the CH2Cl2 electrolyte before and after CO2 bubbling, indicating that TM-substituted POMs play a key role in the CO2RR (Fig. 13f). Therefore, the future direction for constructing efficient and highly selective CO2RR catalysts based on POMs in aqueous electrolytes lies in the creation of stable, multiphase CO2RR active sites. This work provides design principles and guidance for the development of novel heterogeneous CO2RR electrocatalysts.
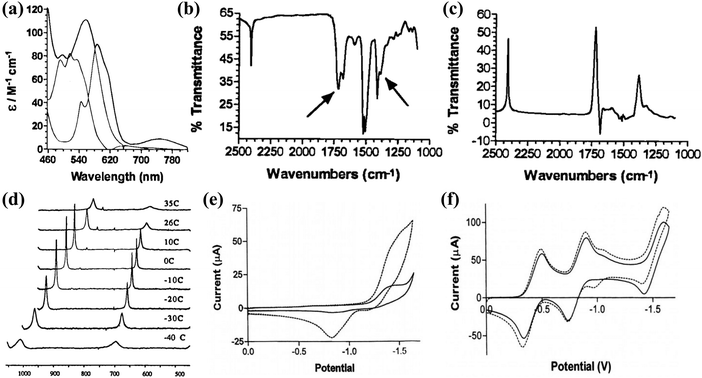 |
| Fig. 13 (a) Visible spectra of α-[SiW11O39Co(OH2)]6−, α-[SiW11O39Co(_)]6−, and α-[SiW11O39Co(CO2)]6− in toluene. (b) Infrared spectrum of α-[SiW11O39Co(CO2)]6− in CCl4. (c) Difference spectrum between α-[SiW11O39Co(CO2)]6− and α-[SiW11O39Co(_)]6−. (d) 13C NMR spectra of α-[SiW11O39Co(CO2)]6− in toluene at different temperatures.151 Copyright 1998, American Chemical Society (ACS). (e) CV curves of SiW11Co in CH2Cl2 before and after bubbling with CO2 gas. (f) CV curves of P2W18 in CH2Cl2 before and after bubbling with CO2 gas.152 Copyright 2002, Springer. | |
3.2.2. POM-involved materials for the CO2RR.
Designing POM-based heterogeneous electrocatalysts has emerged as a challenging approach for designing CO2RR catalysts owing to the solubility of various POMs in aqueous solutions. Constructing POM-involved inorganic–organic hybrid materials can significantly reduce the solubility of POMs, and integrate organic molecular catalysts with CO2RR activity into POMs. Therefore, designing POM-involved inorganic–organic hybrid materials becomes an ideal strategy for constructing novel CO2RR electrocatalysts.16 Du et al.145 prepared a series of POM-carbonyl manganese compounds (abbreviated as POM–MnL) and used them as heterogeneous electrocatalysts for the CO2RR (Fig. 14a). Among them, SiW12–MnL displayed the most significant electrocatalytic activity, selectively reducing CO2 to CO. Various characterizations combined with DFT results indicated that the electron transfer process between the POMs and MnL units can suppress the competing HER, enabling efficient CO2 reduction to CO (Fig. 14b). Furthermore, by changing the type of POMs in the POM–MnL composite catalyst, the electron transfer pathway can be regulated (Fig. 14c), allowing for control of the FE in the CO2RR. Wang's group153 developed a method to incorporate PMo12 into Zn–CoTAPc double molecular layer sandwich nanosheets (MLSs). Various characterizations confirmed that the nanosheet had a thickness of ∼1.2 nm and consisted of two reticular layers of Zn–CoTAPc with interlayered PMo12 anions (Fig. 14d). This unique structure provided highly exposed Co active sites and structural stability against stacking and deactivation. The MLSs showed exceptional catalytic performance in the CO2RR, and the performance of MLSs was further enhanced with external light irradiation (Fig. 14e and f).
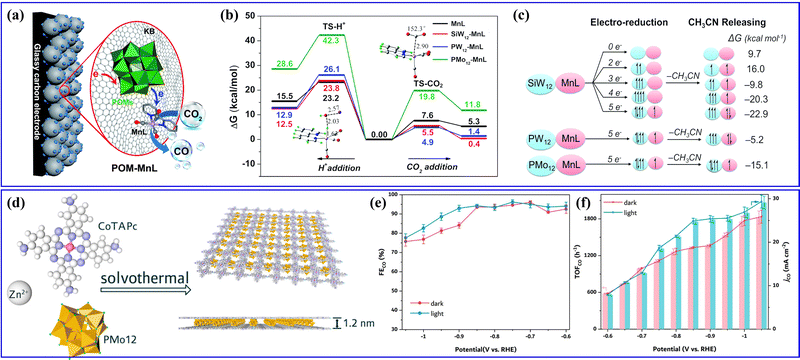 |
| Fig. 14 (a) The CO2RR reaction mechanism of POM–MnL. (b) Potential energy surfaces for H+ and CO2 addition to the respective active CO2 and H adducts. (c) The electronic configurations for the different reduction degree states.145 Copyright 2020, Royal Society of Chemistry (RSC). (d) The synthetic procedure toward Zn–CoTAPc/PMo12 MLSs. (e) FECO-potential plots and (f) jCO and TOFCO plots of Zn–CoTAPc/PMo12 MLSs.153 Copyright 2021, American Chemical Society (ACS). | |
Furthermore, Lan's group has previously reported various series of POMOF materials as heterogeneous catalysts for electrocatalytic CO2RRs.154–156 They conducted a hydrothermal method to prepare a series of stable POM-metalloporphyrin organic framework (PMOF) materials for electrocatalytic CO2RRs (Fig. 15a).154 The research findings revealed that the TM in PMOFs plays a crucial role in the electrocatalytic CO2RR. Among them, Co-modified PMOFs exhibited the highest CO2RR activity (Fig. 15b). Although Co-PMOF exhibited higher CO2RR activity than Co-TCPP, the electrocatalytic CO2RR activity of PMOFs was still derived from the M-TCPP component, and the regulation of different types of POMs in this composite system needs to be further studied. Afterward, they synthesized a series of mixed-valence POM-based MOF composite catalysts (POM@PCN-222(Co), POM = [CoIIICoII(H2O)W11O39]7−, M = Co, Fe, Mn, and Ni) using a post-modification method155 (Fig. 15c). The POM@PCN-222(Co) electrocatalyst combines the advantages of POMs and metalloporphyrin single metal sites, exhibiting highly efficient activity for electrocatalytic CO2 reduction to CO. DFT calculations indicated that POMs could facilitate the directed transfer of electrons from the electrode to the single metal sites, enriching the electron density of the Co center (Fig. 15d), which reduced the reaction energy barrier for the key rate-determining steps in the CO2RR and enhanced the activity of electrocatalytic CO2RR. In addition, metalloporphyrins (M-TCPPs) were used to construct a series of POM-based MOF (M-POMOFs: [PMoV8MoVI4O35(OH)5ZnII4]2[FeIII-TCPP-Cl] Guest; [PMoV8MoVI4O35(OH)5ZnII4]2[MII-TCPP][H2O] Guest; M = Zn, Ni, Cu, Co, and Mn) for photo- and electrocatalytic CO2RR (PCR and ECR) by Lan's group156 (Fig. 15e). This study aimed to uncover the role of single metal sites and clusters in catalysis. In the ECR (Fig. 15f), the Fe-POMOF catalyst exhibited a superior FE in converting CO2 to CO. Their research contributed to understanding the relationships between single metal sites and cluster catalysis.
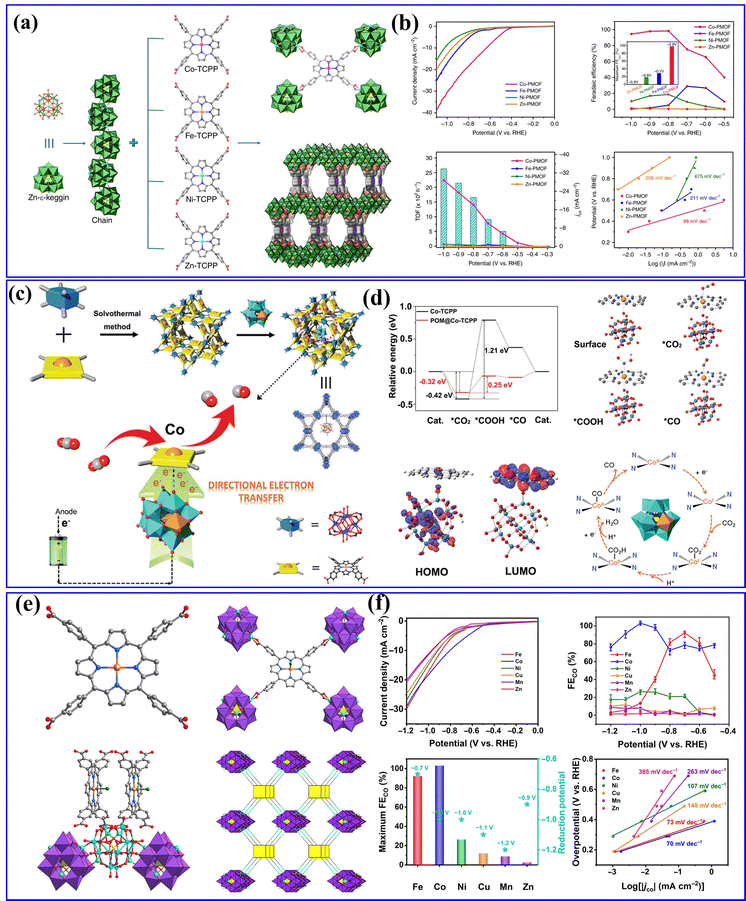 |
| Fig. 15 (a) Schematic illustration of the structures of M-PMOFs. (b) Electrocatalytic performances of M-PMOFs.154 Copyright 2018, Springer Nature. (c) A schematic diagram of the synthesis steps and reaction pathways of POM@PCN-222(Co). (d) DFT calculations of the CO2RR in H-POM@PCN-222(Co).155 Copyright 2021, Wiley-VCH. (e) The crystal structures of M-POMOFs. (f) Electrocatalytic performances of M-POMOFs.156 Copyright 2022, Science Publishing Group. | |
In addition, POMs can also be used as reducing agents and stabilizers, which are widely applied in the synthesis of nanomaterials. Guo et al. synthesized a series of silver nanocluster catalysts reduced by electrodeposition with PMo12 (Ag-PMo)157 or bovine serum albumin (BSA), subsequently modified by SiW12 (AgNC@BSA–SiW).158 The incorporation of POMs as electron transfer mediators resulted in significantly improved efficiency of the silver nanocluster catalysts in the reduction of CO2 to CO, surpassing the performance of bulk Ag. Wei's group159 utilized Mo8-type POM-modified copper nanocubes to obtain nano-catalysts with abundant Cu–O–Mo interfaces for electrocatalytic CO2 reduction to produce acetic acid. The Mo8-modified Cu nano-catalyst can efficiently convert CO2 to acetic acid with a high FE. DFT calculations revealed that the POMs modification facilitates the formation of abundant Cu–O–Mo active sites, promoting the generation of *CH3 intermediates and facilitating their coupling with CO2 molecules, ultimately leading to the production of acetic acid. This work demonstrated the control of the Cu nano-catalyst interface via POM modification, enabling deep reduction of CO2 and providing guidance for the regulation of CO2 reduction depth using POMs. The summary of POM-involved materials for electrocatalytic CO2RR in this review is shown in Table 3.
Table 3 The summary of the POM-involved materials for electrocatalytic CO2RR in this review
Electrocatalyst |
Electrolyte |
Potential |
Principal product |
FE (%) |
Ref. |
SiW12–MnL/KB |
0.5 M KHCO3 |
−0.72 V vs. RHE |
CO |
95 |
145
|
PW12–MnL/KB |
0.5 M KHCO3 |
−0.72 V vs. RHE |
CO |
80 |
145
|
PMo12–MnL/KB |
0.5 M KHCO3 |
−0.52 V vs. RHE |
CO |
65 |
145
|
MnL/KB |
0.5 M KHCO3 |
−0.72 V vs. RHE |
CO |
65 |
145
|
Zn–CoTAPc/PMo12 MLSs (dark) |
0.5 M KHCO3 |
−0.7 V vs. RHE |
CO |
96.1 |
153
|
Zn–CoTAPc/PMo12 MLSs (light) |
0.5 M KHCO3 |
−0.75 V vs. RHE |
CO |
96.2 |
153
|
Co-PMOF |
0.5 M KHCO3 |
−0.8 V vs. RHE |
CO |
98.7 |
154
|
Fe-PMOF |
0.5 M KHCO3 |
−0.7 V vs. RHE |
CO |
28.8 |
154
|
Ni-PMOF |
0.5 M KHCO3 |
−0.8 V vs. RHE |
CO |
18.5 |
154
|
Zn-PMOF |
0.5 M KHCO3 |
−0.9 V vs. RHE |
CO |
0.95 |
154
|
H-POM@PCN-222(Co) |
0.5 M KHCO3 |
−0.8 V vs. RHE |
CO |
96.2 |
155
|
M-POM@PCN-222(Co) |
0.5 M KHCO3 |
−0.8 V vs. RHE |
CO |
91.2 |
155
|
L-POM@PCN-222(Co) |
0.5 M KHCO3 |
−0.8 V vs. RHE |
CO |
71.2 |
155
|
Fe-POMOF |
0.5 M KHCO3 |
−0.7 V vs. RHE |
CO |
92.1 |
156
|
Mo8@Cu/TNA |
NaHCO3 |
−1.13 V vs. RHE |
CH3COOH |
48.68 |
159
|
The above research demonstrates the application of a series of POM-involved catalysts in electrocatalytic CO2RRs. These results indicate that when POMs are combined with organic molecular catalysts possessing CO2RR activity through electrostatic, covalent, or coordination interactions, the solubility of POM catalysts in the electrolyte can be significantly reduced, enabling the construction of heterogeneous POMs catalysts. These findings provide an explanation for the origin of activity in multi-component, multi-center composite electrocatalysts, thereby offering a deeper understanding of the design of heterogeneous CO2RR electrocatalysis.
3.3. N2 reduction reaction
Ammonia (NH3), as a hydrogen-rich carrier with great potential, is an important next-generation green energy source for fuel cells.160 However, industrial ammonia synthesis still relies on the traditional Haber–Bosch method, which requires high temperature (400–600 °C) and high pressure (20–40 MPa), accounting for 1–2% of global energy consumption, as well as large CO2 emissions.161,162 Therefore, exploring the green environmental protection ammonia synthesis technology with normal temperature and pressure is essential for converting intermittent energy sources (such as solar energy and electricity) into continuous ammonia fuel that can be stored and transported anytime and anywhere.
Electrocatalytic nitrogen reduction reaction (NRR) has low energy consumption that can be carried out at room temperature and pressure, which is the most likely method to replace the traditional Hubble method for the production of NH3.163–165 However, the Faraday efficiency of the NRR is still relatively low due to the limitation of thermodynamics and kinetics.166 In terms of thermodynamics, N2 molecules are extremely chemically stable under natural conditions and are difficult to activate.167 From a kinetic point of view, the main challenge of the NRR is the high dissociation energy of nitrogen and the serious side reaction of hydrogen evolution.163,168 Thus, it is necessary to demand the development of highly active electrocatalysts that can effectively reduce the activation energy of N2 reduction and inhibit the side reaction of hydrogen evolution.
3.3.1. POM-based materials for the NRR.
In the NRR process, POMs can be defined as an electron reservoir to provide electrons directly to N2 molecules, which is beneficial to the activation of N2 molecules.57,58 Besides, POMs have been proved by DFT calculations to be an electron source pointing to the active site in the NRR process.57 Hence, POM-based materials can be used as an ideal candidate catalyst for the NRR in both experimental and theoretical studies.
Recently, Liao et al.169 synthesized Mo-PTA@CNT catalyst by immobilizing Mo-PTA (PTA = H3PW12O40) on multi-walled CNT (Fig. 16a), in which Mo-PTA could efficiently adsorb and activate N2 molecules, and CNT could enrich the N2 concentration and accelerate NRR electron transfer. Thus, the as-prepared Mo-PTA@CNT exhibited high NRR activity with an NH3 yield rate and FE value (Fig. 16b). Yang et al.57 integrated POMs into stable MOFs to construct POMOFs (FexCoyMOF-P2W18) for the NRR (Fig. 16c). In the FexCoyMOF-P2W18 catalyst, P2W18 provided electron-rich centers and increased the electron transfer capacity, whereas the FexCoyMOF skeletons increased the stability of the reduced electron-rich POMs. Benefiting from the synergistic effect between POMs and MOFs, the FexCoyMOF-P2W18 catalyst showed excellent electrocatalytic NRR activity. It can be observed from Fig. 16d that the FeCoMOF-P2W18 catalyst exhibited the highest NH3 yield rate (47.04 μg h−1) and FE (31.76%) as compared to other FexCoyMOF-P2W18. Feng et al.170 fabricated an Ag/PW12/Zr–mTiO2 composite with a mesoporous structure through one-pot synthesis (Fig. 16e), which showed superior activity in photo- and electro-driven NRR. In the Ag/PW12/Zr–mTiO2 composite, Ag NPs and PW12 facilitated charge transfer efficiency due to interface engineering, whereas doping Zr in mTiO2 significantly promoted chemisorption of N2, together with enhanced active sites and the production rate of NH3/NH4+. As a result, the Ag/PW12/Zr–mTiO2 composite could reach an NH3/NH4+ production rate of 324.2 μmol gcat−1 h−1 in a photo-driven NRR process and 55 μg gcat−1 h−1 in an electro-driven NRR process.
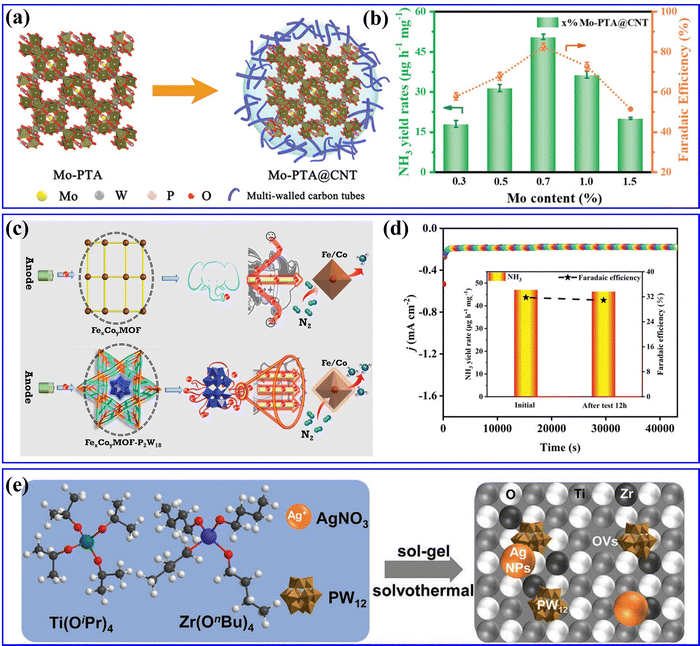 |
| Fig. 16 (a) Schematic diagrams of Mo-PTA and Mo-PTA@CNT. (b) NH3 yield rates and faradaic efficiencies of Mo-PTA@CNT samples with different Mo loadings.169 Copyright 2021, Wiley-VCH. (c) Proposed electron-transfer channel of FexCoyMOF and FexCoyMOF-P2W18 for e-NRR. (d) j–t curve for 12 h of a continuous e-NRR process (inset: the NH3 yields and FEs of before and after electrolysis for 12 h).57 Copyright 2023, Wiley-VCH. (e) Schematic representation of synthesizing Ag/PW12/Zr–mTiO2.170 Copyright 2021, Wiley-VCH. | |
In addition, some researchers also proved the superiority of POM-based materials as NRR catalysts by DFT calculations. Gao et al.171 calculated phosphotungstic acid (PTA)-supported single metal and proved that the M-PTA showed high NRR activity (Fig. 17a and b). Lin et al.172 developed a series of POM (SiMA, SiTA, PMA, and PTA) supported Ru single atoms as NRR electrocatalysts (Fig. 17c). Among them, Ru-PMA and Ru-PTA displayed excellent NRR activity in the entire reactions. Talib et al.173 proved that the NRR mechanisms of Mo1/PMA SAC as an NRR catalyst for activating N2 to convert NH3 were the enzymatic pathway via DFT calculations. The Mo1/PMA catalyst could impede the competitive HER process, thus enhancing the selectivity of NRR (Fig. 17d). Wang et al.174 anchored Ta atoms on a Ta-substituted POM support to form dual-atom catalysts (DACs) for the NRR. The DFT calculations suggested that the interaction between Ta and Ta-POM was the bonding interaction, and the POM carrier surface structure was sensitive to the Ta substituted effects (Fig. 17e). Lin et al.175 manifested that the central heteroatoms (X = Fe, Co, Ni, Cu, Zn, Ga, Ge, As) of POMs could adjust the coordination environment of a single Mo atom (Fig. 17f), and Mo atoms supported the POM catalyst with the central heteroatoms of Co and As has higher NRR activity and a lower limiting potential.
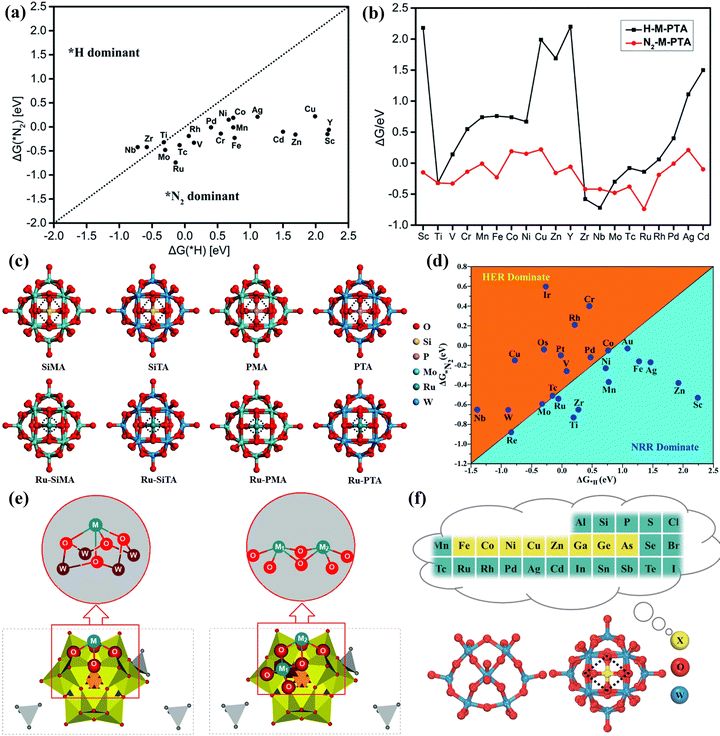 |
| Fig. 17 (a) The calculated ΔG(*N2) and ΔG(*H) on M-PTA. (b) The Gibbs free energy of *N2 and *H adsorbed on M-PTA.171 Copyright 2019, Royal Society of Chemistry (RSC). (c) Optimized structures of POM and Ru-POM.172 Copyright 2020, Royal Society of Chemistry (RSC). (d) Calculated Gibbs free energies of N2 and H2 adsorbed on the M1/PMA cluster.173 Copyright 2022, Royal Society of Chemistry (RSC). (e) The structure of [N(CH3)4+]3[TaII2/POM] (POM = [PW11O39]7−).174 Copyright 2022, Royal Society of Chemistry (RSC). (f) Optimized structures of POM.175 Copyright 2023, Springer, Tsinghua University Press 2022. | |
3.3.2 POM-derived materials for the NRR.
According to previous studies, Mo-based and W-based electrocatalysts exhibit highly active NRR catalysts.176,177 POMs contain the highest oxidation state of early transition metals (Mo, W, V, etc.), which are considered as excellent platforms to construct Mo- and W-derived electrocatalysts.12,61 Wang et al.178 fabricated Fe1.89Mo4.11O7/FeS2@C and FeMoO4/FeS2@C using PMo12@MIL-100(Fe)@PVP as the precursor (Fig. 18a). As presented in Fig. 18b, the Fe1.89Mo4.11O7/FeS2@C showed a high NH3 yield rate and FE in acidic solution, higher than most reported NRR catalysts. Wang et al.179 prepared a POM-derived CoS2/MoS2 electrocatalyst with POMs (H3PMo12O40) as the precursor for the NRR, as shown in Fig. 18c. Compared with individual CoS2 or MoS2, the CoS2/MoS2 catalyst displayed an enhanced NRR activity (Fig. 18d), which was due to the bimetals synergistic effect improving the activation of nitrogen molecules and speeding up the charge transfer at the interface. Yang et al.180 synthesized ternary oxide materials (MoFeO@X, X = 650, 750, 850) utilizing POMs (FeMo6) as the precursor. The FeMo6 precursor was conducive to the formation of controllable multi-interfaces, and could also control the uniform dispersion of ternary oxide composite at the molecular level. The NH3 yield rate and FE of the synthesized MoFeO@750 reached 16.57 μg h−1 mgcat.−1 and 12.33% at −0.4 V vs. RHE in 0.1 mol·L−1 Na2SO4 solution. Yin et al.177 fabricated Pt–W/Mo@rGO-6 with PW6Mo6 as the W/Mo platform (Fig. 18e), in which the Mo and W species exhibited a synergetic effect playing a critical role in the activation and dissociation of N2. As a result, the NH3 yield of the Pt–W/Mo@rGO-6 electrocatalyst was reaching 79.2 μg h−1 mgcat.−1 at −0.3 V vs. RHE. A summary of the POM-based materials and POM-derived materials for electrocatalytic NRR in this review is shown in Table 4.
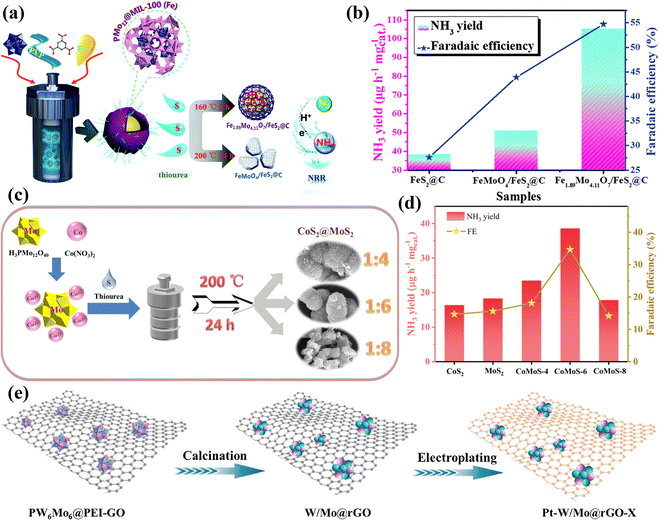 |
| Fig. 18 (a) An illustration of the preparation of Fe1.89Mo4.11O7/FeS2@C and FeMoO4/FeS2@C composites. (b) Comparison of the NH3 yield rate and FE of the prepared electrodes.178 Copyright 2020, Royal Society of Chemistry (RSC). (c) Schematic illustration for the one-step hydrothermal synthesis of CoS2/MoS2 catalyst. (d) Comparison of NH3 yield rate and FE of CoS2, MoS2, CoMoS-4, CoMoS-6, and CoMoS-8.179 Copyright 2021, Elsevier Inc. (e) Schematic synthesis of Pt–W/Mo@rGO-X (X means the electroplating time).177 Copyright 2023, Elsevier B.V. | |
Table 4 A summary of POM-based and POM-derived materials for electrocatalytic NRR in this review
Electrocatalyst |
Electrolyte |
Potential |
NH3 yield rate (μg h−1 mgcat.−1) |
FE (%) |
Ref. |
Mo-PTA@CNT |
0.1 M K2SO4 |
−0.1 V vs. RHE |
51 ± 1 |
81 ± 1 |
169
|
FeCoMOF-P2W18 |
0.1 M HCl |
−0.4 V vs. RHE |
47.04 |
31.56 |
57
|
Ag/PW12/Zr–mTiO2 |
0.05 M H2SO4 |
−0.6 V vs. RHE |
55 |
2.2 |
170
|
Fe1.89Mo4.11O7/FeS2@C |
0.5 M H2SO4 |
−0.4 V vs. RHE |
105.3 |
54.7 |
178
|
Fe1.89Mo4.11O7/FeS2@C |
0.1 M KOH |
−0.4 V vs. RHE |
86.3 |
53.6 |
178
|
CoS2/MoS2 |
1 M K2SO4 |
−0.25 V vs. RHE |
38.61 |
34.66 |
179
|
MoFeO@750 |
0.1 M Na2SO4 |
−0.4 V vs. RHE |
16.57 |
12.33 |
180
|
Pt–W/Mo@rGO-6 |
0.05 M H2SO4 |
−0.3 V vs. RHE |
79.2 |
20 |
177
|
4. The research process of POM-based materials for energy storage devices
4.1. Supercapacitors
Supercapacitors (SCs) have the advantages of large power, long cycles, and simple operation, which have become an ideal choice for electrochemical energy storage.181–183 SCs contain two main types, i.e., an electrochemical double-layer capacitor that stores charge by reversibly adsorbing ions in the electrolyte and pseudocapacitors that work by relying on Faraday reactions.184 POMs can achieve fast and reversible electron transfer, making them very suitable for use as pseudocapacitors.185 However, the inherent low conductivity and high solubility of POMs in many solvents hinder their direct application as electrode materials of SCs.186 Therefore, researchers immobilized POMs on chemically stable, high-surface-area carbon carriers to form mixed or nanocomposite electrochemical supercapacitor electrodes.22 The carbon carrier can improve the conductivity, and simultaneously provide an electrochemical double-layer capacitor to achieve a complementary effect with the Faraday charge storage of POM molecules.187–189
At present, researchers have prepared a variety of POM-based electrode materials, such as POM-CNT, POM-activated carbon (POM-AC), POM-porous carbon (POM-PC), POM-graphene (POM-GO), and POM-conductive polymer (POM-COP), etc. for SCs. Chen et al.190 reported a novel nanocomposite material of SWCNT-TBA-PV2Mo10 for the first time, which exhibited improved power density and energy density due to the redox activity of TBA-PV2Mo10 in the material and the high conductivity and double-layer capacitance of SWCNTs. Nevertheless, the electrostatic bonding approach, while effective in introducing non-conductive molecules, often leads to undesired electron transfer and compromises the structural stability of POMs, thereby hindering their practical use in electrochemistry. To address the issue of leaching, Wang et al.191 fabricated a 3D hybrid hydrogel (PMo12/PPy/CNT) employing an electrostatic co-assembly strategy. As illustrated in Fig. 19a, the PMo12/PPy/CNT consisted of a highly uniform and tightly distributed arrangement of individual PMo12 molecules on PPy hydrogel-functionalized CNT. Compared with conventional composite systems, the PPy hydrogel linker, resembling a “fishnet” serving as the dual purpose of precisely confining the PMo12 clusters in a supramolecular manner and ensuring efficient electrical contact between PMo12 and CNTs (Fig. 19b and c). As a result, the PMo12/PPy/CNT hybrid hydrogel exhibited enhanced supercapacitor performance compared to PMo12/PPy/CNT composite systems. Furthermore, the specific capacitance of the solid device remained stable even when subjected to various bending or twisting conditions (Fig. 19d). Yang et al.192 developed a coaxial cable-like hybrid material (CNT/PMo/PANI) composed of CNTs, polyoxometalate (PMo), and polyaniline (PANI) (Fig. 19e and f). The PMo clusters and PANI played crucial roles as active centers for rapid and reversible redox reactions, contributing to pseudocapacitance.
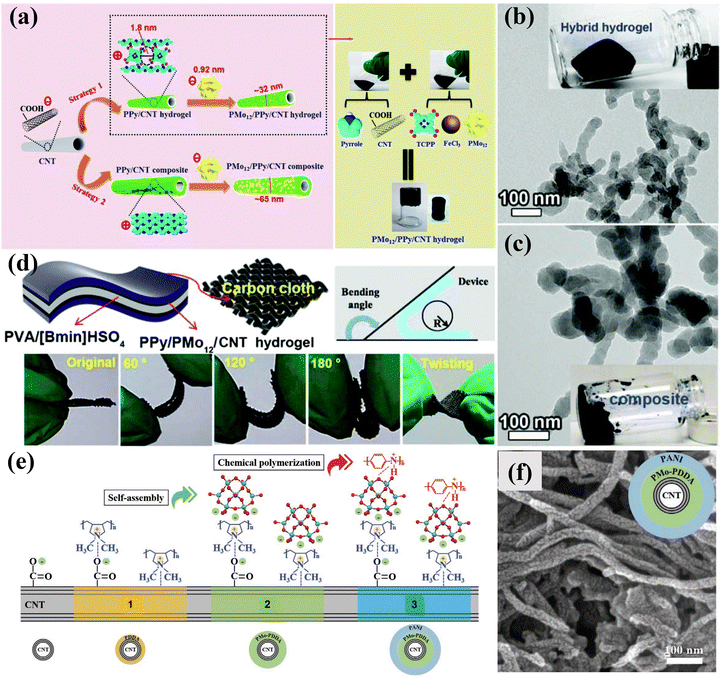 |
| Fig. 19 (a) Fabrication process of PMo12/PPy/CNT; TEM images of (b) PMo12/PPy/CNT hybrid hydrogel and (c) PMo12/PPy/CNT composite. (d) Schematic drawing of the bent SSC and photographs of the flexible SSC under various flexible conditions.191 Copyright 2020, Royal Society of Chemistry (RSC). (e) Schematic diagram of the synthesis mechanism for 1D coaxial cable-like CNT/PMo/PANI. (f) SEM image of CNT/PMo/PANI.192 Copyright 2020, Wiley-VCH. | |
The term “carbon material” specifically pertains to well-defined nano-structures of sp2-hybridized carbon. It has been observed that there exists a significant chemisorption interaction between POMs and carbon materials,22,193 which is beneficial to the stability of the composite SCs electrode. Guevara et al.194 applied PW12 adsorbed onto AC as electrode materials (AC-PW12) (Fig. 20a), resulting in an expanded voltage window of 1.6 V in acidic liquid electrolyte and a high capacitance of 254 F g−1 (Fig. 20b). However, the full potential of the pore structure in commercial AC has not been utilized, and the distribution of supported POMs remains uneven. Subsequently, Palomino et al.195 made efforts to modify the pore size of AC to enhance the capacitance of PMo12-AC (Fig. 20c). While this study successfully adjusted the pore size to enhance PMo12 dispersion, a significant issue remains with the occurrence of leaching or aggregation of PMo12 due to the presence of numerous mesopores (Fig. 20d). Consequently, precise control over the substrate hole size is crucial in addressing this challenge. Hu et al.196 reported an AC@PMo12 composite material in which PMo12 was grown in microporous carbon for SCs. As shown in Fig. 20e, the AC@PMo12 composite material exhibited a uniform distribution of PMo12 within the carbon pores. When AC@PMo12 was assembled into an asymmetric supercapacitor and subjected to constant current charging/discharging cycles in the P-IL electrolyte for 10
000 cycles, the capacitance of the device showed almost no decrease (Fig. 20f).
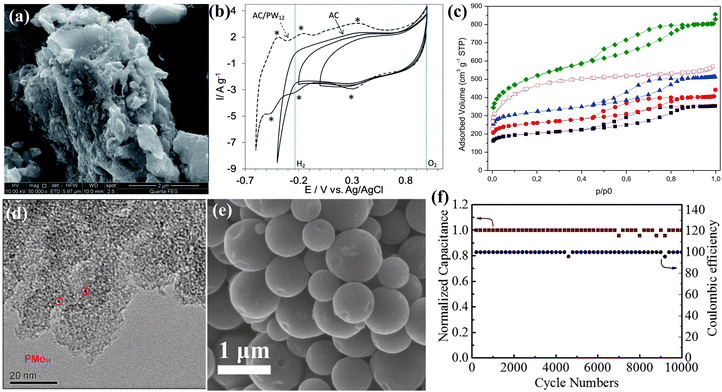 |
| Fig. 20 (a) SEM image of the AC–PW12 hybrid material. (b) CV curves for AC and AC–PW12 at 10 mV s−1.194 Copyright 2014, Royal Society of Chemistry (RSC). (c) Adsorption–desorption isotherms of the carbon support with different activation levels. (d) TEM image of C250-1-PMo12-5.195 Copyright 2016, Elsevier Ltd. (e) SEM image of AC@PMo12. (f) Long-term cycling performance of the asymmetric supercapacitor (AC@PMo12//AC1) over 10 000 cycles at a current density of 10 A g−1.196 Copyright 2016, Elsevier B.V. | |
Researchers have discovered that POMs are more prone to adsorb within the pore size range of 1–2 nm in carbon-based substrates, enabling the achievement of exceptionally high POM loading.197 Genovese et al.185 used pine cones as a biotemplate for synthesizing a PC-PMo12 hybrid with a high specific surface area. Yang et al.54 designed a unique type of porous carbon (referred to as micro-PC) with a monodisperse super-micropore size of ∼1.09 nm. Yang et al.198 designed PMo12-coupled RGO through a simple anion exchange process (Fig. 21a), which showed a high density of POMs distributed individually on the surface of PIL-functionalized RGOs. As shown in Fig. 21b, PMo12-RGO displayed a high SCs performance. Hou et al.199 reported two cases of POMs ([SiMo12O40]4−/[SiW12O40]4−) combined with metal cup aromatic compounds ([Ag5(C2H2N3)6][H5⊂SiMo12O40] and [Ag5(C2H2N3)6][H5⊂SiW12O40], [C2H2N3 = 1H-1,2,4-triazole]) (Fig. 21c). They proposed that the oxidation ability of Keggin ions was the main influencing factor on the electrochemical performance of these POMs combined with metal cup aromatic compounds. Due to the synergistic effect of GO and POMs, the composite electrode achieved a maximum specific capacitance of 230.2 F g−1 (Fig. 21d), which is higher than most reported POM electrode materials. Pakulski et al.200 prepared a novel hybrid material of Mo132-DTAB-EEG with a porous 3D superstructure (Fig. 21e). Combining the redox activity of Mo132 and the high conductivity of EEG, the Mo132-DTAB-EEG enabled a specific capacitance as high as 65 F g−1, as presented in Fig. 21f.
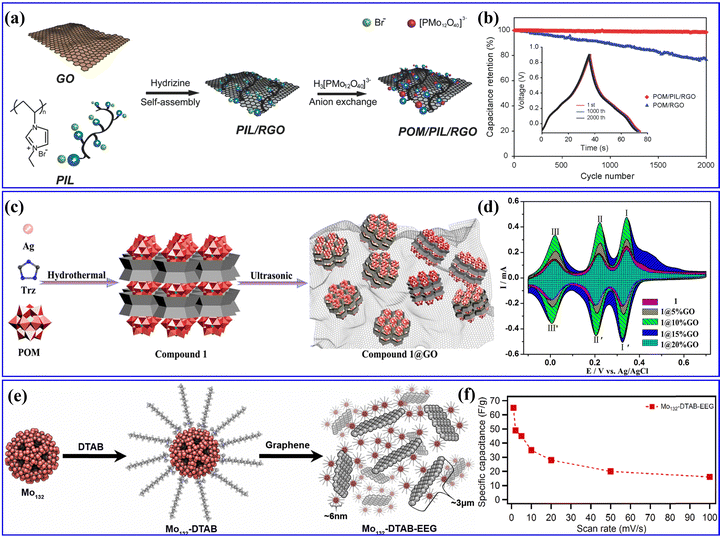 |
| Fig. 21 (a) The synthetic process for the POM/PIL/RGO nanohybrids. (b) Capacitance retention of the POM/PIL/RGO and POM/RGO electrodes evaluated.198 Copyright 2014, Wiley-VCH. (c) The synthetic route of compound 1@GO composite materials. (d) Comparison of CVs for 1- and 1@nGO-based electrodes.199 Copyright 2019, American Chemical Society (ACS). (e) The formation process of Mo132-DTAB-EEG hybrid material. (f) Comparison of specific capacitance vs. scan rate for Mo132-DTAB-EEG.200 Copyright 2018, Elsevier B.V. | |
The polymer exhibits electrical conductivity primarily via the delocalized electrons in the main chain and utilizes the abundant functional groups or positive properties to support POMs by physical cross-linking and electrostatic interaction.201,202 Liu et al.203 prepared PMo12/PPy composite via anchored PMo12 onto a highly interconnected 3D conductive polypyrrole (PPy) hydrogel scaffold (Fig. 22a). In a three-electrode system, the specific capacitance of the PMo12/PPy composite hydrogel was measured to be 776 F g−1, nearly 2.5 times higher than that of traditional PMo12/PPy composite materials (Fig. 22b). The solid-state supercapacitor achieved a maximum specific capacitance of 162.1 F g−1 and an energy density of 50.66 W h kg−1 at a power density of 750 W kg−1, surpassing the electrochemical performance of other POMs or metal oxides (Fig. 22c). The summary of POM-based electrode materials for SCs in this review is displayed in Table 5.
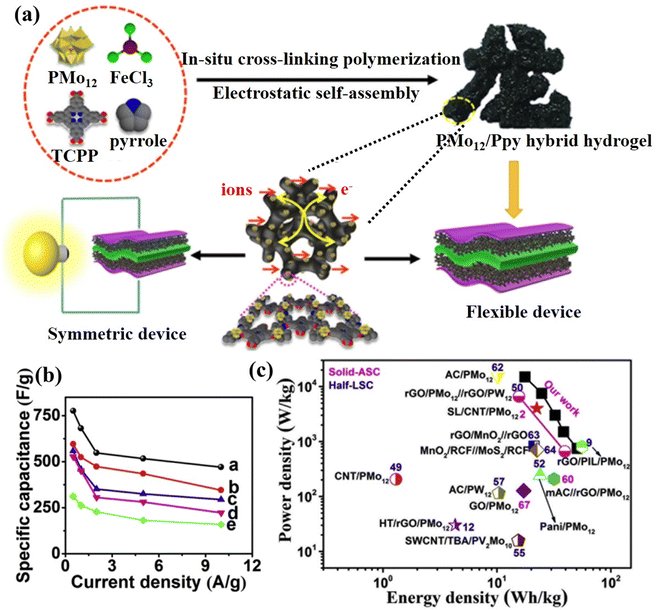 |
| Fig. 22 (a) The preparation process for the PMo12/PPy hybrid hydrogel for SCs. (b) Specific capacitances of the prepared samples at various current densities. (c) Ragone plots of the P2-PPy gel//P2-PPy gel device compared with the values reported for other devices.203 Copyright 2019, Elsevier Ltd. | |
Table 5 The summary of POM-based electrode materials for SCs in this review
Electrode materials |
Electrolyte |
Specific capacitance |
Energy density |
Capacitance retention |
Ref. |
SWCNT-TBA-PV2Mo10 |
1 M H2SO4 |
444 F g−1@2 A g−1 |
15.4 W h kg−1 |
95%@6500 cycles |
190
|
PMo12/PPy/CNT |
0.5 M H2SO4 |
1170 F g−1@1 A g−1 |
67.5 μW h cm−2 |
86%@3000 cycles |
191
|
CNT/PMo/PANI |
1 M H2SO4 |
825 F g−1@5 A g−1 |
18.7 W h kg−1 |
85%@6000 cycles |
192
|
AC–PW12 |
1 M H2SO4 |
254 F g−1 |
2.32 W h cm−3 |
98%@30000 cycles |
194
|
C250-3-PMo12-2 |
1 M H2SO4 |
285 F g−1@6 mA cm−2 |
10.2 W h kg−1 |
— |
195
|
AC@PMo12 |
acidic P-IL |
223 F g−1@1 mV s−1 |
— |
∼100%@10 000 cycles |
196
|
Pinecone AC/PMo12O40 |
1 M H2SO4 |
361 F g−1@10 mV s−1 |
— |
70%@5000 cycles |
185
|
PMo12@micro-PC |
0.5 M H2SO4 |
443 mF cm−2@3 mA cm−2 |
0.12 mW h cm−2 |
81%@7500 cycles |
54
|
POM/PIL/RGO |
0.5 M H2SO4 |
408 F g−1@0.5 A g−1 |
56 W h kg−1 |
98%@2000 cycles |
198
|
Compound 1@15% GO |
0.5 M H2SO4 |
230.2 F g−1@0.5 A g−1 |
— |
93%@1000 cycles |
199
|
Mo132-DTAB-EEG |
1 M H2SO4 |
65 F g −1@1 mV s−1 |
— |
99%@5000 cycles |
200
|
PMo12/PPy |
1 M H2SO4 |
300 F g−1@5 A g−1 |
50.66 W h kg−1 |
80%@1000 cycles |
203
|
4.2. Rechargeable batteries
Rechargeable batteries drive the global energy transition to renewable energy but are faced with resource constraints.204 At present, the common rechargeable batteries include lithium-ion batteries (LIBs), lithium–sulfur batteries (LSBs), metal-ion batteries, metal–air batteries, etc.205 The development of rechargeable batteries with high energy and power density, safety, long cycle life, fast response, and low cost is the goal of researchers.206 Therefore, the design of high-performance electrode materials has important significance for improving the performance of rechargeable batteries.
POM-based electrode materials for LIBs.
The high energy density of LIBs has always been a forefront topic in battery research. The performance of LIBs mainly depends on the active materials used for Li+ storage electrodes.207 The basic requirements for electrode materials include high reversible capacity, good cycling stability, high safety performance, low cost, and environmental friendliness.208,209 It is necessary to use negative electrode materials with larger capacities and a faster Li+ diffusion rate to enhance the energy and power density of LIBs.210 Although different microscale materials such as metal oxides,211 alloys,212 and pyrolytic carbon213 have been developed as negative electrode materials for LIBs, the morphological and structural changes associated with these microscale materials during battery operation pose limitations such as high electronic resistance, slow solid-state Li+ diffusion rate, small surface area, and poor shape flexibility. Therefore, current microscale structured negative electrode materials for LIBs, especially under high current density, still face issues such as poor cycling stability and insufficient charge/discharge capacity, which hinder the further development of current LIBs.
POMs, known for the high stability of thermodynamic and chemical, controllable size, precise structure, and the ability of high-valent metal ions in POMs to undergo multi-electron redox reactions during battery cycling, are considered potential electrode materials for LIBs.23,59 Wang et al.214 conducted a study using X-ray absorption fine structure analysis to study the structure and electronic states of PMo12, which indicated that when PMo12 was applied as a positive electrode material for LIBs, the Mo6+ ions in PMo12 were reduced to Mo4+ during the discharge process, accompanied by the transfer of 24 electrons (Fig. 23a). Their study revealed the behavior characteristics of PMo12 as an “electron sponge” and demonstrated its significant potential as a high-performance electrode material for LIBs. Sonoyama et al.215 utilized Anderson-type POMs Na3[AlMo6O24H6] (NAM) as a novel cathode material for high capacity and excellent stability in LIBs (Fig. 23b). The stability of the [AlMo6O24H6]3− during the charge–discharge process was investigated by in situ polycrystalline X-ray diffraction and Raman spectroscopy. This significant finding provided experimental and theoretical evidence for the application of POMs and POM-based composite materials as electrode materials in LIBs.
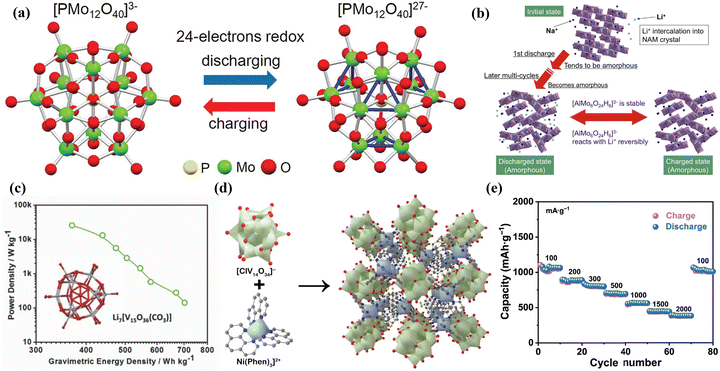 |
| Fig. 23 (a) A schematic diagram of electron gain and loss during charge and discharge of PMo12 cathode materials.214 Copyright 2012, American Chemical Society (ACS). (b) A schematic image of the reaction mechanism for NAM.215 Copyright 2014, Elsevier B.V. (c) Crystal structure of Li7[V15O36(CO3)] and its ratio of power to mass-energy density as the cathode material for LIBs.216 Copyright 2015, Wiley-VCH. (d) View of polyanion, macrocation, and crystal structure of NiV14 along the [001] axis in polyhedron mode. (e) Rate performance at various current densities.217 Copyright 2023, Tsinghua University Press. | |
V (50.96 g mol−1) has a lower relative molecular mass and higher redox capability as compared to Mo (95.96 g mol−1) and W (183.84 g mol−1). Thus, V-based POMs may exhibit a higher mass-energy density and specific capacity when used as battery electrodes. Subsequently, researchers have extensively explored the application of polyoxovanadates (POVs) as electrode materials in LIBs. Cronin's group216 discovered that Li7[V15O36(CO3)] showed promising potential as a positive electrode material for LIBs (Fig. 23c). The VIV and VV in Li7[V15O36(CO3)] could reversibly gain and lose electrons during the charge–discharge process. Therefore, the pouch cell using Li7[V15O36(CO3)] as a positive electrode material exhibited excellent rate performance and cycling stability. Wang et al.217 introduced a novel V-POM ([Ni(Phen)3][ClV14O34]Cl, NiV14) with a large-sized polycation containing multiple charges for studying its application as a negative electrode material in LIBs. The presence of the multi-charged large-sized polycation [Ni(Phen)3]2+ in NiV14 provided more stable open channels (Fig. 23d). The LIBs assembled with NiV14 exhibited a high reversible capacity, high Coulombic efficiency and good stability (Fig. 23e). Electrochemical analysis indicated that capacitance-controlled charge storage processes and fast Li+ ion diffusion kinetics were the main factors contributing to the improved rate performance of the NiV14 electrode.
Although POMs possess precise structures, tunable sizes, and excellent redox performance, their dissolvable nature in the electrolyte and low specific surface area of POMs lead to low capacity, poor stability, and shorter lifespan.218 Additionally, the uneven dispersion and aggregation of POM clusters hinder electron transfer between the material and the electrode, further impacting its cycling and rate performance. These drawbacks still limit the advance of POMs as electrode materials for LIBs. To address these issues, researchers have proposed combining POMs with other materials that have high specific surface areas or porous structures, such as conductive matrices, MOFs, composite materials, etc. By forming composites with these materials, it is possible to achieve uniform loading of POMs while maintaining a high specific surface area and porosity, thereby enhancing the functionality in conjunction with the conductive matrix or MOFs. The POM-based electrode materials for LIBs reported in recent years are summarized in Table 6.
Table 6 A summary of the POM-based electrode materials for LIBs reported in recent years
Electrode materials |
Electrode |
Discharge capacities |
Rate performance |
Ref. |
POM/CNT |
Anode |
850 mA h·g−1@0.5 mA cm−2 |
1532 mA h·g−1@0.05 mA cm−2 |
219
|
565 mA h·g−1@1 mA cm−2 |
Py-Anderson-CNT |
Anode |
1899 mA h g−1@0.5 mA cm−2 |
1072 mA h g−1@0.05 mA cm−2 |
220
|
480 mA h g−1@1 mA cm−2 |
CNTs-SiW11 |
Anode |
1189 mA h g−1@0.5 mA cm−2 |
802 mA h g−1@0.05 mA cm−2 |
221
|
584 mA h g−1@1 mA cm−2 |
POM/RGO–MCB |
Cathode |
67 A h kg−1@1.5 V |
— |
222
|
NAM−EDAG |
Anode |
1835 mA h g−1@100 mA g−1 |
1100 mA h g−1@100 mA g−1 |
223
|
400 mA h g−1@10 A g−1 |
Mn3V19-HIL/RGO |
Cathode |
214 mA h g−1@100 mA g−1 |
121 mA h g−1@5 A g−1 |
224
|
73 mA h g−1@2 A g−1 |
Co-SiW@GO |
Anode |
452 mA h g−1@2 A g−1 |
373 mA h g−1@0.1 A g−1 |
225
|
242 mA h g−1@5 A g−1 |
MF POMs/MXenes |
Anode |
297 mA h g−1@1.0 A g−1 |
301 mA h g−1@0.1 A g−1 |
226
|
115 mA h g−1@4 A g−1 |
VM POMs/MXenes |
Anode |
196 W h kg−1 |
627 mA h g−1@0.1 A g−1 |
227
|
244 mA h g−1@4 A g−1 |
POMOF |
Anode |
1525 mA h g−1@0.25C |
1525 mA h g−1@0.25 C |
228
|
1421 mA h g−1@1.25 C |
NNU-11 |
Anode |
1322 mA h g−1@50 mA g−1 |
— |
229
|
NAU3 |
Anode |
1406 mA h g−1@100 mA g−1 |
488 mA h g−1@0.1 A g−1 |
230
|
283 mA h g−1@1 A g−1 |
POMOFs/RGO |
Anode |
2368 mA h g−1@50 mA g−1 |
409 mA h g−1@2 A g−1 |
231
|
365 mA h g−1@3 A g−1 |
PMo10V2-ILs@MIL-100 |
Anode |
1666 mA h g−1@100 mA g−1 |
1033 mA h g−1@0.1 A g−1 |
232
|
348 mA h g−1@3 A g−1 |
POM-based electrode materials for other rechargeable batteries.
POM-based materials are also applied as the electrode material for studies in lithium–sulfur batteries, metal-ion batteries and metal–air batteries. Wei's group233 synthesized rGO–CNT/PW12 as the cathodes in LSBs (Fig. 24a), which displayed 1425 mA h g−1 of specific capacities, and 573 mA h g−1 of rate capability. Their work confirmed the POM catalyst as a key structural element in the charging/discharging process of LSBs (Fig. 24b). In view of the shuttle effect of lithium polysulfides in LSBs, Chen's group234 prepared {Co4W18}/rGO materials (Fig. 24c), which can not only adsorb lithium polysulfides but also improve battery capacity and cycle performance. By using PMo12 as a crystal growth regulator, Ren et al.235 induced the oriented growth of Na3V2(PO4)2F3 crystals along the [110] crystal axis, and constructed a Na3V2(PO4)2F3 cathode material with continuous sodium ion diffusion channels for sodium ion batteries (Fig. 24d). Shen et al.236 used an electron/ion sponge POM (Na2H8[MnV13O38], NMV) as the cathode material for sodium-ion batteries (Fig. 24e). In NMV, it can accommodate 11 electron/ion pairs per mole content, which has a great contribution to the high capacitance of the material. Mandal et al.237 fabricated a PVIM–CoPOM/NCNT composite with a high ORR and OER performance, as shown in Fig. 24f. As a result, the Zn–air batter performance using PVIM–CoPOM/NCNT as the air cathode exhibited a low charge–discharge potential gap and high energy density.
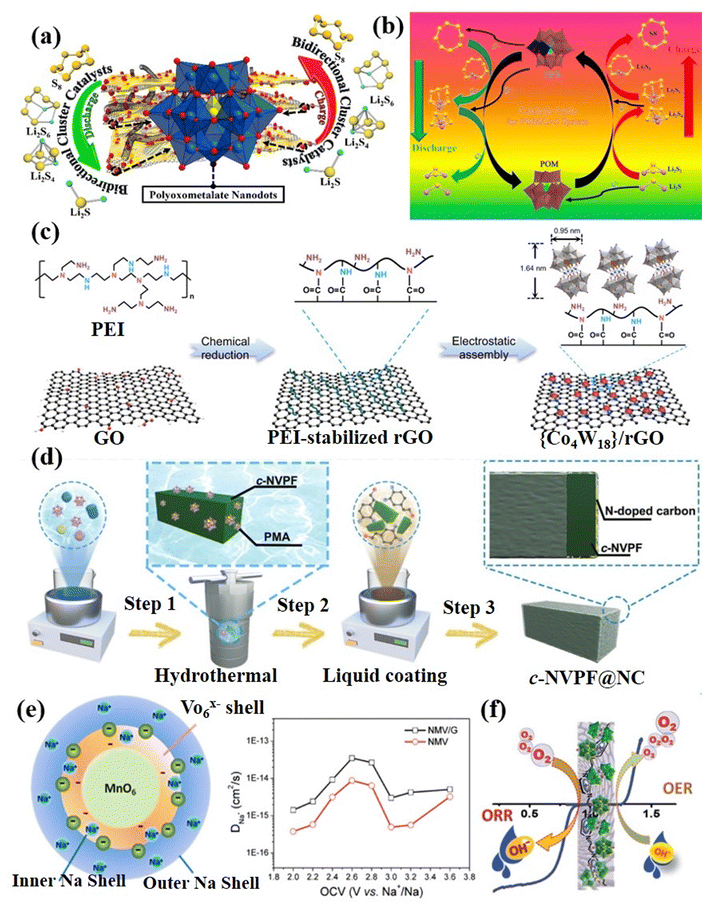 |
| Fig. 24 (a) A schematic diagram of rGO–CNT/PW12 as the cathode in LSBs. (b) The proposed catalytic scheme mediated by a Keggin-type {PW12} catalyst in a LSB system.233 Copyright 2021, American Chemical Society (ACS). (c) A schematic diagram of the synthesis of {Co4W18}/rGO materials.234 Copyright 2022, Springer Nature. (d) NVPF synthesis process.235 Copyright 2023, Wiley-VCH GmbH. (e) Schematic illustration of Na ion storage in a notion of electron/ion sponge and the calculated Na-ion chemical diffusion coefficients.236 Copyright 2017, American Chemical Society (ACS). (f) Scheme representing the bifunctional activity of the PVIM–CoPOM/NCNT composite towards both the OER and ORR.237 Copyright 2021, Royal Society of Chemistry (RSC). | |
5 Conclusions and outlooks
In this review, recently developed POM-based and POM-derived materials in the field of water-splitting, CO2RR, NRR, SCs, and LIBs are systematically summarized. The fundamental roles of POMs in clean energy conversion and storage systems are introduced. The material design principles have been emphasized, ranging from single clusters, assemblies, organic–inorganic hybrids to derivatives. The relationship of the structure and performance of POM-based nanostructures in electrocatalytic applications is also discussed. There still exist challenges and opportunities to meet the requirements of electrocatalytic and energy applications, including but not limited to the following items.
(1) In the context of catalysis and energy-related applications of POMs, one of the key challenges to continue research is anchoring POMs to suitable substrates or constructing POM-based composites. Stability is a crucial factor in the application of POMs as electrode materials. Most POMs are water-soluble and have relatively large molecular sizes. How to control the conditions to make POM particles well dispersed and fixed on the support bracket, or coated POMs with other materials, will effectively improve the stability, which deserves further study.
(2) The unique physical and chemical properties of POMs make them show significant advantages in electrocatalytic applications. To fully understand the changes in fine dynamic structure, the evolution of active sites, and key reaction intermediates during the reaction of POM-based materials, the challenge is developing more accurate in situ electrochemical characterization techniques.
(3) Due to the complexity of POM-based catalysts, exploring the combination of multivariate analysis and machine learning for data analysis to achieve the redesign and optimization of catalysts can accelerate the development of POMs in the field of electrocatalytic and energy.
(4) Although many POM-based materials and their derivatives have been reported as catalysts for electrocatalytic and energy fields, most of the synthesis methods are limited to the laboratory scale due to the complex synthesis process. Therefore, it is very important to develop simple and scalable synthetic routes. At the same time, to achieve industrial applications, it is also necessary to rationally design POM-based materials with high mechanical strength and stability.
Conflicts of interest
The authors declare no conflict of interest in this manuscript.
Acknowledgements
The authors greatly acknowledge the financial support from the National Natural Science Foundation of China (22301257, 21072221, 21875247, 21172252).
References
- T. Muneer, M. Asif and S. Munawwar, Sustainable production of solar electricity with particular reference to the Indian economy, Renewable Sustainable Energy Rev., 2005, 9, 444–473 CrossRef.
- N. Armaroli and V. Balzani, The hydrogen issue, ChemSusChem, 2011, 4, 21–36 CrossRef CAS PubMed.
- X. Tian, C. An and Z. Chen, The role of clean energy in achieving decarbonization of electricity generation, transportation, and heating sectors by 2050: A meta-analysis review, Renewable Sustainable Energy Rev., 2023, 182, 113404 CrossRef.
- S. E. Hosseini and M. A. Wahid, Hydrogen production from renewable and sustainable energy resources: Promising green energy carrier for clean development, Renewable Sustainable Energy Rev., 2016, 57, 850–866 CrossRef CAS.
- W. Zhang, Z. Jin and Z. Chen, Rational-Designed Principles for Electrochemical and Photoelectrochemical Upgrading of CO2 to Value-Added Chemicals, Adv. Sci., 2022, 9, 2105204 CrossRef CAS PubMed.
- R. Shi, X. Zhang, G. I. Waterhouse, Y. Zhao and T. Zhang, The journey toward low temperature, low pressure catalytic nitrogen fixation, Adv. Energy Mater., 2020, 10, 2000659 CrossRef CAS.
- Y. Yang, Y. Han, W. Jiang, Y. Zhang, Y. Xu and A. M. Ahmed, Application of the supercapacitor for energy storage in China: role and strategy, Appl. Sci., 2021, 12, 354 CrossRef.
- K. Turcheniuk, D. Bondarev, G. G. Amatucci and G. Yushin, Battery materials for low-cost electric transportation, Mater. Today, 2021, 42, 57–72 CrossRef CAS.
- A. Kraytsberg and Y. Ein-Eli, The impact of nano-scaled materials on advanced metal–air battery systems, Nano Energy, 2013, 2, 468–480 CrossRef CAS.
- K. Jiao, J. Xuan, Q. Du, Z. Bao, B. Xie, B. Wang, Y. Zhao, L. Fan, H. Wang and Z. Hou, Designing the next generation of proton-exchange membrane fuel cells, Nature, 2021, 595, 361–369 CrossRef CAS PubMed.
- S. Yuan, X. Duan, J. Liu, Y. Ye, F. Lv, T. Liu, Q. Wang and X. Zhang, Recent progress on transition metal oxides as advanced materials for energy conversion and storage, Energy Storage Mater., 2021, 42, 317–369 CrossRef.
- Y. Zhang, M. X. Li, Q. Hao, F. Su, Z. M. Zhu, J. S. Li, X. J. Sang, C. S. Wang and L. C. Zhang, Two new estertin modified tungstosilicates: synthesis, catalytic activity and photoelectrochemical property, Dalton Trans., 2020, 49, 7234–7244 RSC.
- S.-S. Wang and G.-Y. Yang, Recent advances in polyoxometalate-catalyzed reactions, Chem. Rev., 2015, 115, 4893–4962 CrossRef CAS PubMed.
- L. Guo, L. He, Q. Zhuang, B. Li, C. Wang, Y. Lv, J. Chu and Y. F. Song, Recent Advances in Confining Polyoxometalates and the Applications, Small, 2023, 2207315 CrossRef CAS PubMed.
- Y. Zhang, J. Liu, S.-L. Li, Z.-M. Su and Y.-Q. Lan, Polyoxometalate-based materials for sustainable and clean energy conversion and storage, EnergyChem, 2019, 1, 100021 CrossRef.
- N. Li, J. Liu, B. X. Dong and Y. Q. Lan, Polyoxometalate-based compounds for photo-and electrocatalytic applications, Angew. Chem., Int. Ed., 2020, 59, 20779–20793 CrossRef CAS PubMed.
- Z. Zeb, Y. Huang, L. Chen, W. Zhou, M. Liao, Y. Jiang, H. Li, L. Wang, L. Wang, H. Wang, T. Wei, D. Zang, Z. Fan and Y. Wei, Comprehensive overview of polyoxometalates for electrocatalytic hydrogen evolution reaction, Coord. Chem. Rev., 2023, 482, 215058 CrossRef CAS.
- Z.-H. Wang, X.-F. Wang, Z. Tan and X.-Z. Song, Polyoxometalate/metal–organic framework hybrids and their derivatives for hydrogen and oxygen evolution electrocatalysis, Mater. Today Energy, 2021, 19, 100618 CrossRef CAS.
- J. Du, Y.-Y. Ma, H. Tan, Z.-H. Kang and Y. Li, Progress of electrochemical CO2 reduction reactions over polyoxometalate-based materials, Chin. J. Catal., 2021, 42, 920–937 CrossRef CAS.
- D. Zang and H. Wang, Polyoxometalate-based nanostructures for electrocatalytic and photocatalytic CO2 reduction, Polyoxometalates, 2022, 1, 9140006 CrossRef.
- R. Liu and C. Streb, Polyoxometalate-single atom catalysts (POM-SACs) in energy research and catalysis, Adv. Energy Mater., 2021, 11, 2101120 CrossRef CAS.
- Y. Ji, L. Huang, J. Hu, C. Streb and Y.-F. Song, Polyoxometalate-functionalized nanocarbon materials for energy conversion, energy storage and sensor systems, Energy Environ. Sci., 2015, 8, 776–789 RSC.
- Q. Li, L. Zhang, J. Dai, H. Tang, Q. Li, H. Xue and H. Pang, Polyoxometalate-based materials for advanced electrochemical energy conversion and storage, Chem. Eng. J., 2018, 351, 441–461 CrossRef CAS.
- B. Huang, D.-H. Yang and B.-H. Han, Application of polyoxometalate derivatives in rechargeable batteries, J. Mater. Chem. A, 2020, 8, 4593–4628 RSC.
- D. Wang, L. Liu, J. Jiang, L. Chen and J. Zhao, Polyoxometalate-based composite materials in electrochemistry: state-of-the-art progress and future outlook, Nanoscale, 2020, 12, 5705–5718 RSC.
- M. R. Horn, A. Singh, S. Alomari, S. Goberna-Ferrón, R. Benages-Vilau, N. Chodankar, N. Motta, K. K. Ostrikov, J. MacLeod, P. Sonar, P. Gomez-Romero and D. Dubal, Polyoxometalates (POMs): from electroactive clusters to energy materials, Energy Environ. Sci., 2021, 14, 1652–1700 RSC.
- B. Fabre, C. Falaise and E. Cadot, Polyoxometalates-Functionalized Electrodes for (Photo) Electrocatalytic Applications: Recent Advances and Prospects, ACS Catal., 2022, 12, 12055–12091 CrossRef CAS.
- Q. Zhang, F. Li and L. Xu, Application of polyoxometalates in third-generation solar cells, Polyoxometalates, 2023, 2, 9140018 CrossRef.
- J. F. Keggin, Structure of the molecule of 12-phosphotungstic acid, Nature, 1933, 131, 908–909 CrossRef CAS.
- D. Cheng, K. Li, H. Zang and J. Chen, Recent Advances on Polyoxometalate-Based Ion-Conducting Electrolytes for Energy-Related Devices, Energy Environ. Mater., 2023, 6, e12341 CrossRef CAS.
- X. Huang, Z. Wang, T. Wang, W. Wang and P. He, Fe-substituted Polyoxometalate-based Spherical Assemblies as Catalysts for Olefin Epoxidation, Chem. Res. Chin. Univ., 2023, 39, 660–665 CrossRef CAS.
- P. He, B. Xu, X. Xu, L. Song and X. Wang, Surfactant encapsulated palladium-polyoxometalates: controlled assembly and their application as single-atom catalysts, Chem. Sci., 2016, 7, 1011–1015 RSC.
- S. Roy, D. C. Crans and T. N. Parac-Vogt, Polyoxometalates in catalysis, biology, energy and materials science, Front. Chem., 2019, 7, 646 CrossRef CAS PubMed.
- J. Liu, S. Nie, L. Wu and X. Wang, High-entropy Metal Oxide-polyoxometalate-palladium Sub-1 nm Nanowires for Semi-hydrogenation Reaction of Alkynes, Chem. Res. Chin. Univ., 2023, 39, 624–629 CrossRef CAS.
- J. H. Kruse, M. Langer, I. Romanenko, I. Trentin, D. Hernández-Castillo, L. González, F. H. Schacher and C. Streb, Polyoxometalate-Soft Matter Composite Materials: Design Strategies,
Applications, and Future Directions, Adv. Funct. Mater., 2022, 32, 2208428 CrossRef CAS.
- J. Liu, Y. Li, Z. Chen, N. Liu, L. Zheng, W. Shi and X. Wang, Polyoxometalate Cluster-Incorporated High Entropy Oxide Sub-1 nm Nanowires, J. Am. Chem. Soc., 2022, 144, 23191–23197 CrossRef CAS PubMed.
- M. I. S. Veríssimo, D. V. Evtuguin and M. T. S. R. Gomes, Polyoxometalate Functionalized Sensors: A Review, Front. Chem., 2022, 10, 840657 CrossRef PubMed.
- P. Song and T. Wang, Application of Polyoxometalates in Chemiresistive Gas Sensors: A Review, ACS Sens., 2022, 7, 3634–3643 CrossRef CAS PubMed.
- H. Li, S. Pang, S. Wu, X. Feng, K. Müllen and C. Bubeck, Layer-by-Layer Assembly and UV Photoreduction of Graphene–Polyoxometalate Composite Films for Electronics, J. Am. Chem. Soc., 2011, 133, 9423–9429 CrossRef CAS PubMed.
- L. Cheng, C. Liang, W. Liu, Y. Wang, B. Chen, H. Zhang, Y. Wang, Z. Chai and S. Wang, Three-Dimensional Polycatenation of a Uranium-Based Metal–Organic Cage: Structural Complexity and Radiation Detection, J. Am. Chem. Soc., 2020, 142, 16218–16222 CrossRef CAS PubMed.
- H. Zhang, A. Li, K. Li, Z. Wang, X. Xu, Y. Wang, M. V. Sheridan, H.-S. Hu, C. Xu, E. V. Alekseev, Z. Zhang, P. Yan, K. Cao, Z. Chai, T. E. Albrecht-Schönzart and S. Wang, Ultrafiltration separation of Am(VI)-polyoxometalate from lanthanides, Nature, 2023, 616, 482–487 CrossRef CAS PubMed.
- J. Gautam, Y. Liu, J. Gu, Z. Ma, B. Dahal, A. Nabi Chishti, L. Ni, G. Diao and Y. Wei, Three-dimensional nano assembly of nickel cobalt sulphide/polyaniline@polyoxometalate/reduced graphene oxide hybrid with superior lithium storage and electrocatalytic properties for hydrogen evolution reaction, J. Colloid Interface Sci., 2022, 614, 642–654 CrossRef CAS PubMed.
- Y. Lu, C. Yue, Y. Li, W. Bao, X. Guo, W. Yang, Z. Liu, P. Jiang, W. Yan, S. Liu, Y. Pan and Y. Liu, Atomically dispersed Ni on Mo2C embedded in N, P co-doped carbon derived from polyoxometalate supramolecule for high-efficiency hydrogen evolution electrocatalysis, Appl. Catal., B, 2021, 296, 120336 CrossRef CAS.
- Q.-Q. Pan, C.-Y. Xu, X. Li, J.-F. Zhang, X.-L. Hu, Y. Geng and Z.-M. Su, Porous Ni-Mo bimetallic hybrid electrocatalyst by intermolecular forces in precursors for enhanced hydrogen generation, Chem. Eng. J., 2021, 405, 126962 CrossRef CAS.
- Z. Wei, J. Pang, Y. Ji, X. Yang, Y. Liu, L. Ren and Y. Zhang, Ultrafine multi-metallic carbide nanocrystals encased in a carbon matrix as durable electrocatalysts towards effective alkaline hydrogen evolution reaction, Mater. Adv., 2021, 2, 336–344 RSC.
- L. Zhang, S. Li, C. J. Gómez-García, H. Ma, C. Zhang, H. Pang and B. Li, Two Novel Polyoxometalate-Encapsulated Metal–Organic Nanotube Frameworks as Stable and Highly Efficient Electrocatalysts for Hydrogen Evolution Reaction, ACS Appl. Mater. Interfaces, 2018, 10, 31498–31504 CrossRef CAS PubMed.
- C. Wang, L. Li, H. Wang, T. Qu, J. Tian, D. Wang and Z. Kang, Tunable Ni/Fe-Mo carbide catalyst with high activity toward hydrogen evolution reaction, Can. J. Chem. Eng., 2020, 98, 1784–1793 CrossRef CAS.
- C. Huang, X. Miao, C. Pi, B. Gao, X. Zhang, P. Qin, K. Huo, X. Peng and P. K. Chu, Mo2C/VC heterojunction embedded in graphitic carbon network: An advanced electrocatalyst for hydrogen evolution, Nano Energy, 2019, 60, 520–526 CrossRef CAS.
- Y. Zhang, H. Guo, P. Yuan, K. Pang, B. Cao, X. Wu, L. Zheng and R. Song, Structural evolution of CoMoO4 to CoOOH by ion electrochemical etching for boosting oxygen evolution reaction, J. Power Sources, 2019, 442, 227252 CrossRef CAS.
- X. Wang, G. Zhang, W. Yin, S. Zheng, Q. Kong, J. Tian and H. Pang, Metal–organic framework-derived phosphide nanomaterials for electrochemical applications, Carbon Energy, 2022, 4, 246–281 CrossRef CAS.
- Y.-J. Tang, Y. Chen, H.-J. Zhu, A. M. Zhang, X.-L. Wang, L.-Z. Dong, S.-L. Li, Q. Xu and Y.-Q. Lan, Solid-phase hot-pressing synthesis of POMOFs on carbon cloth and derived phosphides for all pH value hydrogen evolution, J. Mater. Chem. A, 2018, 6, 21969–21977 RSC.
- J. Xiao, Z. Zhang, Y. Zhang, Q. Lv, F. Jing, K. Chi and S. Wang, Large-scale printing synthesis of transition metal phosphides encapsulated in N, P co-doped carbon as highly efficient hydrogen evolution cathodes, Nano Energy, 2018, 51, 223–230 CrossRef CAS.
- Y. Zhang, H. Guo, X. Li, J. Du, W. Ren and R. Song, A 3D multi-interface structure of coral-like Fe-Mo-S/Ni3S2@NF using for high-efficiency and stable overall water splitting, Chem. Eng. J., 2021, 404, 126483 CrossRef CAS.
- S. Yang, M. Wang, Y. Zhang, P. He, W. Cong, C. Wang, Q. Yang, X. Liu, T. Wang, X. Zhang and J. Zhou, Single-Molecule Confinement Induced Intrinsic Multi-Electron Redox-Activity to Enhance Supercapacitor Performance, Energy Environ. Mater., 2023, 6, e12396 CrossRef CAS.
- F. Sun, C. Yue, J. Wang, Y. Liu, W. Bao, N. Liu, Y. Tuo and Y. Lu, Lacunary polyoxometalate oriented construction of dispersed Ni3S2 confined in WO3 for electrocatalytic water splitting, J. Colloid Interface Sci., 2023, 645, 188–199 CrossRef CAS PubMed.
- C. Freire, D. M. Fernandes, M. Nunes and M. Araújo, Polyoxometalate-based modified electrodes for electrocatalysis: from molecule sensing to renewable energy-related applications, Adv. Electrode Mater., 2017, 149–212 Search PubMed.
- M. Yang, X. Wang, C. J. Gómez-García, Z. Jin, J. Xin, X. Cao, H. Ma, H. Pang, L. Tan and G. Yang, Efficient Electron Transfer from an Electron-Reservoir Polyoxometalate to Dual-Metal-Site Metal-Organic Frameworks for Highly Efficient Electroreduction of Nitrogen, Adv. Funct. Mater., 2023, 33, 2214495 CrossRef CAS.
- X. Hui, L. Wang, Z. Yao, L. Hao and Z. Sun, Recent progress of photocatalysts based on tungsten and related metals for nitrogen reduction to ammonia, Front. Chem., 2022, 10, 978078 CrossRef CAS PubMed.
- G. M. Bosch, A. Sarapulova and S. Dsoke, Study of Polyoxometalates as Electrode Materials for Lithium-Ion Batteries: Thermal Stability Paves the Way to Improved Cycle Stability, ChemElectroChem, 2020, 8, 656–664 CrossRef.
- Y.-Q. Jiao, H.-J. Yan, C.-G. Tian and H.-G. Fu, Structure engineering and electronic modulation of transition metal interstitial compounds for electrocatalytic water splitting, Acc. Mater. Res., 2022, 4, 42–56 CrossRef.
- Y. Zhang, H. Guo, J. Ren, X. Li, W. Ren and R. Song, MoO3 crystal facets modulation by doping heteroatom Fe from polyoxometalate for quasi-industrial oxygen evolution reaction, Appl. Catal., B, 2021, 298, 120582 CrossRef CAS.
- J. Liu, W. Shi, B. Ni, Y. Yang, S. Li, J. Zhuang and X. Wang, Incorporation of clusters within inorganic materials through their addition during nucleation steps, Nat. Chem., 2019, 11, 839–845 CrossRef CAS PubMed.
- M. Dresselhaus and I. Thomas, Alternative energy technologies, Nature, 2001, 414, 332–337 CrossRef CAS PubMed.
- S. Chu and A. Majumdar, Opportunities and challenges for a sustainable energy future, Nature, 2012, 488, 294–303 CrossRef CAS PubMed.
- G. Zhao, K. Rui, S. X. Dou and W. Sun, Heterostructures for Electrochemical Hydrogen Evolution Reaction: A Review, Adv. Funct. Mater., 2018, 28, 1803291 CrossRef.
- X. Li, L. L. Zhao, J. Y. Yu, X. Y. Liu, X. L. Zhang, H. Liu and W. J. Zhou, Water Splitting: From Electrode to Green Energy System, Nano-Micro Lett., 2020, 12, 131 CrossRef CAS PubMed.
- Z. Zhang, Y. Qin, M. Dou, J. Ji and F. Wang, One-step conversion from Ni/Fe polyphthalocyanine to N-doped carbon supported Ni-Fe nanoparticles for highly efficient water splitting, Nano Energy, 2016, 30, 426–433 CrossRef CAS.
- T. Sun, W. Zang, J. Sun, C. Li, J. Fan, E. Liu and J. Wang, SACs on Non-Carbon Substrates: Can They Outperform for Water Splitting?, Adv. Funct. Mater., 2023, 2301526 CrossRef CAS.
- S. S. Wang and G. Y. Yang, Recent advances in polyoxometalate-catalyzed reactions, Chem. Rev., 2015, 115, 4893–4962 CrossRef CAS PubMed.
- B. Keita, U. Kortz, L. R. B. Holzle, S. Brown and L. Nadjo, Efficient hydrogen-evolving cathodes based on proton and electron reservoir behaviors of the phosphotungstate [H7P8W48O184]33-and the Co (II)-containing silicotungstates [Co6(H2O)30{Co9Cl2(OH)3(H2O)9(β-SiW8O31)3}]5-and [{Co3(B-β-SiW9O33(OH))(B-β-SiW8O29OH)2}2]22-, Langmuir, 2007, 23, 9531–9534 CrossRef CAS PubMed.
- M. D. Symes and L. Cronin, Decoupling hydrogen and oxygen evolution during electrolytic water splitting using an electron-coupled-proton buffer, Nat. Chem., 2013, 5, 403–409 CrossRef CAS PubMed.
- C. Wang, M. Zhou, Y. Ma, H. Tan, Y. Wang and Y. Li, Hybridized Polyoxometalate-Based Metal–Organic Framework with Ketjenblack for the Nonenzymatic Detection of H2O2, Chem.–Asian J., 2018, 13, 2054–2059 CrossRef CAS PubMed.
- Y. Zhang, Y. Liu, D. Wang, J. Liu, J. Zhao and L. Chen, State-of-the-art advances in the syntheses, structures, and applications of polyoxometalate-based metal–organic frameworks, Polyoxometalates, 2023, 2, 9140017 CrossRef.
- M. I. Veríssimo, D. V. Evtuguin and M. Gomes, Polyoxometalate functionalized sensors: a review, Front. Chem., 2022, 10, 840657 CrossRef PubMed.
- N. Kawasaki, H. Wang, R. Nakanishi, S. Hamanaka, R. Kitaura, H. Shinohara, T. Yokoyama, H. Yoshikawa and K. Awaga, Nanohybridization of polyoxometalate clusters and single-wall carbon nanotubes: applications in molecular cluster batteries, Angew. Chem., Int. Ed., 2011, 123, 3533–3536 CrossRef.
- D. Pakulski, A. Gorczyński, D. Brykczyńska, V. Montes-García, W. Czepa, I. Janica, M. Bielejewski, M. Kubicki, V. Patroniak and P. Samori, New Anderson-Based Polyoxometalate Covalent Organic Frameworks as Electrodes for Energy Storage Boosted Through Keto-Enol Tautomerization, Angew. Chem., Int. Ed., 2023, e202305239 CAS.
- B. Nohra, H. El Moll, L. M. Rodriguez Albelo, P. Mialane, J. Marrot, C. Mellot-Draznieks, M. O'Keeffe, R. Ngo Biboum, J. Lemaire, B. Keita, L. Nadjo and A. Dolbecq, Polyoxometalate-based metal organic frameworks (POMOFs): structural trends, energetics, and high electrocatalytic efficiency for
hydrogen evolution reaction, J. Am. Chem. Soc., 2011, 133, 13363–13374 CrossRef CAS PubMed.
- J. S. Qin, D. Y. Du, W. Guan, X. J. Bo, Y. F. Li, L. P. Guo, Z. M. Su, Y. Y. Wang, Y. Q. Lan and H. C. Zhou, Ultrastable Polymolybdate-Based Metal-Organic Frameworks as Highly Active Electrocatalysts for Hydrogen Generation from Water, J. Am. Chem. Soc., 2015, 137, 7169–7177 CrossRef CAS PubMed.
- R. Liu, G. Zhang, H. Cao, S. Zhang, Y. Xie, A. Haider, U. Kortz, B. Chen, N. S. Dalal, Y. Zhao, L. Zhi, C.-X. Wu, L.-K. Yan, Z. Su and B. Keitag, Enhanced proton and electron reservoir abilities of polyoxometalate grafted on graphene for high-performance hydrogen evolution, Energy Environ. Sci., 2016, 9, 1012–1023 RSC.
- D. M. Fernandes, M. P. Araujo, A. Haider, A. S. Mougharbel, A. J. Fernandes, U. Kortz and C. Freire, Polyoxometalate-graphene Electrocatalysts for the Hydrogen Evolution Reaction, ChemElectroChem, 2018, 5, 273–283 CrossRef CAS.
- D. Zang, Y. Huang, Q. Li, Y. Tang and Y. Wei, Cu dendrites induced by the Anderson-type polyoxometalate NiMo6O24 as a promising electrocatalyst for enhanced hydrogen evolution, Appl. Catal., B, 2019, 249, 163–171 CrossRef CAS.
- M. Blasco-Ahicart, J. Soriano-López and J. R. Galán-Mascarós, Conducting organic polymer electrodes with embedded polyoxometalate catalysts for water splitting, ChemElectroChem, 2017, 4, 3296–3301 CrossRef CAS.
- G. Y. Lee, I. Kim, J. Lim, M. Y. Yang, D. S. Choi, Y. Gu, Y. Oh, S. H. Kang, Y. S. Nam and S. O. Kim, Spontaneous linker-free binding of polyoxometalates on nitrogen-doped carbon nanotubes for efficient water oxidation, J. Mater. Chem. A, 2017, 5, 1941–1947 RSC.
- Y. Zhang, J. Liu, S. Li, Z. Su and Y. Lan, Polyoxometalate-based materials for sustainable and clean energy conversion and storage, EnergyChem, 2019, 1, 100021 CrossRef.
- A. R. Howells, A. Sankarraj and C. Shannon, A diruthenium-substituted polyoxometalate as an electrocatalyst for oxygen generation, J. Am. Chem. Soc., 2004, 126, 12258–12259 CrossRef CAS PubMed.
- F. M. Toma, A. Sartorel, M. Iurlo, M. Carraro, P. Parisse, C. Maccato, S. Rapino, B. R. Gonzalez, H. Amenitsch and T. Da Ros, Efficient water oxidation at carbon nanotube–polyoxometalate electrocatalytic interfaces, Nat. Chem., 2010, 2, 826–831 CrossRef CAS PubMed.
- W. Luo, J. Hu, H. Diao, B. Schwarz, C. Streb and Y. Song, Stabile Polyoxometallat-Nickelschaum-Elektroden für elektrochemische Sauerstoffentwicklung im alkalischen Milieu, Angew. Chem., Int. Ed., 2017, 129, 5023–5026 CrossRef.
- M. Blasco-Ahicart, J. Soriano-López, J. J. Carbó, J. M. Poblet and J.-R. Galan-Mascaros, Polyoxometalate electrocatalysts based on earth-abundant metals for efficient water oxidation in acidic media, Nat. Chem., 2018, 10, 24–30 CrossRef CAS PubMed.
- M. Martin-Sabi, J. Soriano-Lopez, R. S. Winter, J. J. Chen, L. Vila-Nadal, D. L. Long, J. R. Galan-Mascaros and L. Cronin, Redox tuning the Weakley-type polyoxometalate archetype for the oxygen evolution reaction, Nat. Catal., 2018, 1, 208–213 CrossRef CAS PubMed.
- J. Gautam, Y. Liu, J. Gu, Z. Y. Ma, J. J. Zha, B. Dahal, L. N. Zhang, A. N. Chishti, L. B. Ni, G. W. Diao and Y. G. Wei, Fabrication of Polyoxometalate Anchored Zinc Cobalt
Sulfide Nanowires as a Remarkable Bifunctional Electrocatalyst for Overall Water Splitting, Adv. Funct. Mater., 2021, 31, 2106147 CrossRef CAS.
- S. H. Talib, Z. Lu, X. Yu, K. Ahmad, B. Bashir, Z. Yang and J. Li, Theoretical Inspection of M1/PMA Single-Atom Electrocatalyst: Ultra-High Performance for Water Splitting (HER/OER) and Oxygen Reduction Reactions (OER), ACS Catal., 2021, 11, 8929–8941 CrossRef CAS.
- J. Wang, F. Xu, H. Jin, Y. Chen and Y. Wang, Non-noble metal-based carbon composites in hydrogen evolution reaction: fundamentals to applications, Adv. Mater., 2017, 29, 1605838 CrossRef PubMed.
- J. Deng, P. Ren, D. Deng and X. Bao, Enhanced electron penetration through an ultrathin graphene layer for highly efficient catalysis of the hydrogen evolution reaction, Angew. Chem. Int. Ed., 2015, 54, 2100–2104 CrossRef CAS PubMed.
- Y. Liu, G. Yu, G. D. Li, Y. Sun, T. Asefa, W. Chen and X. Zou, Coupling Mo2C with nitrogen-rich nanocarbon leads to efficient hydrogen-evolution electrocatalytic sites, Angew. Chem., Int. Ed., 2015, 127, 10902–10907 CrossRef.
- G. Yan, C. Wu, H. Tan, X. Feng, L. Yan, H. Zang and Y. Li, N-Carbon coated P-W2C composite as efficient electrocatalyst for hydrogen evolution reactions over the whole pH range, J. Mater. Chem. A, 2017, 5, 765–772 RSC.
- L. N. Zhang, S. H. Li, H. Q. Tan, S. U. Khan, Y. Y. Ma, H. Y. Zang, Y. H. Wang and Y. G. Li, MoP/Mo2C@C: A New Combination of Electrocatalysts for Highly Efficient Hydrogen Evolution over the Entire pH Range, ACS Appl. Mater. Interfaces, 2017, 9, 16270–16279 CrossRef CAS.
- Y. Ma, C. Wu, X. Feng, H. Tan, L. Yan, Y. Liu, Z. Kang, E. Wang and Y. Li, Highly efficient hydrogen evolution from seawater by a low-cost and stable CoMoP@C electrocatalyst superior to Pt/C, Energy Environ. Sci., 2017, 10, 788–798 RSC.
- F. Y. Yu, Y. Gao, Z. L. Lang, Y. Y. Ma, L. Y. Yin, J. Du, H. Q. Tan, Y. H. Wang and Y. G. Li, Electrocatalytic performance of ultrasmall Mo2C affected by different transition metal dopants in hydrogen evolution reaction, Nanoscale, 2018, 10, 6080–6087 RSC.
- Y. Gao, Z. L. Lang, F. Y. Yu, H. Q. Tan, G. Yan, Y. H. Wang, Y. Y. Ma and Y. G. Li, A Co2P/WC Nano-Heterojunction Covered with N-Doped Carbon as Highly Efficient Electrocatalyst for Hydrogen Evolution Reaction, ChemSusChem, 2018, 11, 1082–1091 CrossRef CAS PubMed.
- X. Yang, X. Feng, H. Tan, H. Zang, X. Wang, Y. Wang, E. Wang and Y. Li, N-Doped graphene-coated molybdenum carbide nanoparticles as highly efficient electrocatalysts for the hydrogen evolution reaction, J. Mater. Chem. A, 2016, 4, 3947–3954 RSC.
- Y.-Y. Ma, Z.-L. Lang, L.-K. Yan, Y.-H. Wang, H.-Q. Tan, K. Feng, Y.-J. Xia, J. Zhong, Y. Liu, Z.-H. Kang and Y.-G. Li, Highly efficient hydrogen evolution triggered by a multi-interfacial Ni/WC hybrid electrocatalyst, Energy Environ. Sci., 2018, 11, 2114–2123 RSC.
- G. Yan, X. J. Feng, S. U. Khan, L. G. Xiao, W. G. Xi, H. Q. Tan, Y. Y. Ma, L. N. Zhang and Y. G. Li, Polyoxometalate and Resin-Derived P-Doped Mo2C@N-Doped Carbon as a Highly Efficient Hydrogen-Evolution Reaction Catalyst at All pH Values, Chem. – Asian J., 2018, 13, 158–163 CrossRef CAS PubMed.
- Y. Huang, X. Song, J. Deng, C. Zha, W. Huang, Y. Wu and Y. Li, Ultra-dispersed molybdenum phosphide and phosphosulfide nanoparticles on hierarchical carbonaceous scaffolds for hydrogen evolution electrocatalysis, Appl. Catal., B, 2019, 245, 656–661 CrossRef CAS.
- J. S. Li, Y. Wang, C. H. Liu, S. L. Li, Y. G. Wang, L. Z. Dong, Z. H. Dai, Y. F. Li and Y. Q. Lan, Coupled molybdenum carbide and reduced graphene oxide electrocatalysts for efficient hydrogen evolution, Nat. Commun., 2016, 7, 11204 CrossRef CAS.
- X. L. Wang, Y. J. Tang, W. Huang, C. H. Liu, L. Z. Dong, S. L. Li and Y. Q. Lan, Efficient Electrocatalyst for the Hydrogen Evolution Reaction Derived from Polyoxotungstate/Polypyrrole/Graphene, ChemSusChem, 2017, 10, 2402–2407 CrossRef CAS PubMed.
- Y. Huang, J. Ge, J. Hu, J. Zhang, J. Hao and Y. Wei, Nitrogen-Doped Porous Molybdenum Carbide and Phosphide Hybrids on a Carbon Matrix as Highly Effective Electrocatalysts for the Hydrogen Evolution Reaction, Adv. Energy Mater., 2018, 8, 1701601 CrossRef.
- Y. Huang, J. Hu, H. Xu, W. Bian, J. Ge, D. Zang, D. Cheng, Y. Lv, C. Zhang, J. Gu and Y. Wei, Fine Tuning Electronic Structure of Catalysts through Atomic Engineering for Enhanced Hydrogen Evolution, Adv. Energy Mater., 2018, 8, 1800789 CrossRef.
- C. Y. Xu, J. Li, D. Sun, X. Li, X. L. Wang and Z. M. Su, Co/WC@NC electrocatalysts derived from polyoxometalates (POM) for efficient hydrogen evolution, Nanotechnology, 2021, 32, 375602 CrossRef CAS.
- H. J. Yan, C. G. Tian, L. Wang, A. P. Wu, M. C. Meng, L. Zhao and H. G. Fu, Phosphorus-modified tungsten nitride/reduced graphene oxide as a high-performance, non-noble-metal electrocatalyst for the hydrogen evolution reaction, Angew. Chem., Int. Ed., 2015, 54, 6325–6329 CrossRef CAS PubMed.
- X. B. Han, X. Y. Tang, Y. Lin, E. Gracia-Espino, S. G. Liu, H. W. Liang, G. Z. Hu, X. J. Zhao, H. G. Liao, Y. Z. Tan, T. Wagberg, S. Y. Xie and L. S. Zheng, Ultrasmall Abundant Metal-Based Clusters as Oxygen-Evolving Catalysts, J. Am. Chem. Soc., 2019, 141, 232–239 CrossRef CAS PubMed.
- N. C. S. Selvam, L. Du, B. Y. Xia, P. J. Yoo and B. You, Reconstructed Water Oxidation Electrocatalysts: The Impact of Surface Dynamics on Intrinsic Activities, Adv. Funct. Mater., 2020, 31, 2008190 CrossRef.
- F. C. Shen, S. Sun, Z. Xin, S. Li, L. Dong, Q. Huang, Y. Wang, J. Liu and Y. Lan, Hierarchically phosphorus doped bimetallic nitrides arrays with unique interfaces for efficient water splitting, Appl. Catal., B, 2019, 243, 470–480 CrossRef CAS.
- Z. Kou, Y. Yu, X. Liu, X. Gao, L. Zheng, H. Zou, Y. Pang, Z. Wang, Z. Pan, J. He, S. J. Pennycook and J. Wang, Potential-Dependent Phase Transition and Mo-Enriched Surface Reconstruction of γ-CoOOH in a Heterostructured Co-Mo2C Precatalyst Enable Water Oxidation, ACS Catal., 2020, 10, 4411–4419 CrossRef CAS.
- J. Zhang, Q. Y. Zhang and X. L. Feng, Support and Interface Effects in Water-Splitting Electrocatalysts, Adv. Mater., 2019, 31, 1808167 CrossRef PubMed.
- R. Subbaraman, D. Tripkovic, D. Strmcnik, K.-C. Chang, M. Uchimura, A. P. Paulikas, V. Stamenkovic and N. M. Markovic, Enhancing hydrogen evolution activity in water splitting by tailoring Li+-Ni(OH)2-Pt interfaces, Science, 2011, 334, 1256–1260 CrossRef CAS PubMed.
- N. Danilovic, R. Subbaraman, D. Strmcnik, K. C. Chang, A. Paulikas, V. Stamenkovic and N. M. Markovic, Enhancing the alkaline hydrogen evolution reaction activity through the bifunctionality of Ni(OH)2/metal catalysts, Angew. Chem., Int. Ed., 2012, 124, 12663–12666 CrossRef.
- Z. Zhuang, S. A. Giles, J. Zheng, G. R. Jenness, S. Caratzoulas, D. G. Vlachos and Y. Yan, Nickel supported on nitrogen-doped carbon nanotubes as hydrogen oxidation reaction catalyst in alkaline electrolyte, Nat. Commun., 2016, 7, 1–8 Search PubMed.
- F. Yu, Z. Lang, L. Yin, K. Feng, Y. Xia, H. Tan, H. Zhu, J. Zhong, Z. Kang and Y. Li, Pt-O bond as an active site superior to Pt0 in hydrogen evolution reaction, Nat. Commun., 2020, 11, 1–7 CrossRef PubMed.
- L. An, J. Feng, Y. Zhang, R. Wang, H. Liu, G. Wang, F. Cheng and P. Xi, Epitaxial Heterogeneous Interfaces on N-NiMoO4/NiS2 Nanowires/Nanosheets to Boost Hydrogen and Oxygen Production for Overall Water Splitting, Adv. Funct. Mater., 2019, 29, 1805298 CrossRef.
- G. Zhao, Y. Jiang, S.-X. Dou, W. Sun and H. Pan, Interface engineering of heterostructured electrocatalysts towards efficient alkaline hydrogen electrocatalysis, Sci. Bull., 2021, 66, 85–96 CrossRef CAS PubMed.
- J. G. Hou, B. Zhang, Z. W. Li, S. Y. Cao, Y. Q. Sun, Y. Z. Wu, Z. M. Gao and L. C. Sun, Vertically aligned oxygenated-CoS2–MoS2 heteronanosheet architecture from polyoxometalate for efficient and stable overall water splitting, ACS Catal., 2018, 8, 4612–4621 CrossRef CAS.
- Y. Lu, X. Guo, L. Yang, W. Yang, W. Sun, Y. Tuo, Y. Zhou, S. Wang, Y. Pan, W. Yan, D. Sun and Y. Liu, Highly efficient CoMoS heterostructure derived from vertically anchored Co5Mo10 polyoxometalate for electrocatalytic overall water splitting, Chem. Eng. J., 2020, 394, 124849 CrossRef CAS.
- Y. Hou, H. Pang, J. Xin, H. Ma, B. Li, X. Wang and L. Tan, Multi-Interface-Modulated CoS2@MoS2 Nanoarrays Derived by Predesigned Germanomolybdate Polymer Showing Ultrahighly Electrocatalytic Activity for Hydrogen Evolution Reaction in Wide pH Range, Adv. Mater. Interfaces, 2020, 7, 2000780 CrossRef CAS.
- G. Huang, Z. Xiao, R. Chen and S. Wang, Defect Engineering of Cobalt-Based Materials for Electrocatalytic Water Splitting, ACS Sustainable Chem. Eng., 2018, 6, 15954–15969 CrossRef CAS.
- D. Yan, Y. Li, J. Huo, R. Chen, L. Dai and S. Wang, Defect chemistry of nonprecious-metal electrocatalysts for oxygen reactions, Adv. Mater., 2017, 29, 1606459 CrossRef PubMed.
- J. Xie, H. Zhang, S. Li, R. Wang, X. Sun, M. Zhou, J. Zhou, X. W. Lou and Y. Xie, Defect-rich MoS2 ultrathin nanosheets with additional active edge sites for enhanced electrocatalytic hydrogen evolution, Adv. Mater., 2013, 25, 5807–5813 CrossRef CAS PubMed.
- Y. Tang, Y. Wang, X. Wang, S. Li, W. Huang, L. Dong, C. Liu, Y. Li and Y. Lan, Molybdenum Disulfide/Nitrogen-Doped Reduced Graphene Oxide Nanocomposite with Enlarged Interlayer Spacing for Electrocatalytic Hydrogen Evolution, Adv. Energy Mater., 2016, 6, 1600116 CrossRef.
- J. Tang, Y. Chu, K. Wang, B. Deng, Y. Li and X. Tan, Facile In Situ Synthesis of an Ultrafine and Defect-Rich Co-doped MoS2 Nanocluster Catalyst for Efficient Hydrogen Evolution Reaction in Alkaline Medium, ACS Appl. Energy Mater., 2021, 4, 2300–2306 CrossRef CAS.
- S. R. Ede and Z. Luo, Tuning the intrinsic catalytic activities of oxygen-evolution catalysts by doping: a comprehensive review, J. Mater. Chem. A, 2021, 9, 20131–20163 RSC.
- Z. F. Huang, J. Wang, Y. Peng, C. Y. Jung, A. Fisher and X. Wang, Design of efficient bifunctional oxygen reduction/evolution electrocatalyst: recent advances and perspectives, Adv. Energy Mater., 2017, 7, 1700544 CrossRef.
- H. Lin, N. Liu, Z. Shi, Y. Guo, Y. Tang and Q. Gao, Cobalt-Doping in Molybdenum-Carbide Nanowires Toward Efficient Electrocatalytic Hydrogen Evolution, Adv. Funct. Mater., 2016, 26, 5590–5598 CrossRef CAS.
- B. Huang, D. Yang and B. Han, Application of polyoxometalate derivatives in rechargeable batteries, J. Mater. Chem. A, 2020, 8, 4593–4628 RSC.
- Y. C. Huang, Y. H. Sun, X. L. Zheng, T. Aoki, B. Pattengale, J. Huang, X. He, W. Bian, S. Younan, N. Williams, J. Hu, J. X. Ge, N. Pu, X. X. Yan, X. Q. Pan, L. J. Zhang, Y. G. Wei and J. Gu, Atomically engineering activation sites onto metallic 1T-MoS2 catalysts for enhanced electrochemical hydrogen evolution, Nat. Commun., 2019, 10, 982 CrossRef CAS PubMed.
- G. Eda, T. Fujita, H. Yamaguchi, D. Voiry, M. Chen and M. Chhowalla, Coherent atomic and electronic heterostructures of single-layer MoS2, ACS Nano, 2012, 6, 7311–7317 CrossRef CAS PubMed.
- Y. Zhang, H. Guo, M. Song, L. Sun and R. Song, Modulation of the morphology and electronic structure of Ni3S2 nano-forests via P and Mo co-doping in polyoxometalates to promote the urea oxidation reaction, J. Mater. Chem. A, 2023, 11, 3584–3593 RSC.
- Y. Shi, Y. Zhou, Y. Lou, Z. Chen, H. Xiong and Y. Zhu, Homogeneity of Supported Single-Atom Active Sites Boosting the Selective Catalytic Transformations, Adv. Sci., 2022, 9, 2201520 CrossRef CAS PubMed.
- F.-Y. Yu, Z.-L. Lang, Y.-J. Zhou, K. Feng, H.-Q. Tan, J. Zhong, S.-T. Lee, Z.-H. Kang and Y.-G. Li, Revealing hydrogen evolution performance of single-atom platinum electrocatalyst with polyoxometalate molecular models, ACS Energy Lett., 2021, 6, 4055–4062 CrossRef CAS.
- S. Niu, J. Yang, H. Qi, Y. Su, Z. Wang, J. Qiu, A. Wang and T. Zhang, Single-atom Pt promoted Mo2C for electrochemical hydrogen evolution reaction, J. Energy Chem., 2021, 57, 371–377 CrossRef CAS.
- S. H. Talib, B. Ali, S. Mohamed, X.-L. Jiang, K. Ahmad, A. Qurashi and J. Li, Computational screening of M1/PW12O40 single-atom electrocatalysts for water splitting and oxygen reduction reactions, J. Mater. Chem. A, 2023, 11, 16334–16348 RSC.
- P. M. Vitousek, H. A. Mooney, J. Lubchenco and J. M. Melillo, Human domination of Earth's ecosystems, Science, 1997, 277, 494–499 CrossRef CAS.
- X. Wang, X. Lv, G. Zheng and Y. Fu, Room-temperature Electrochemical C1-to-fuel Conversion: Perspectives from Material Engineering and Device Design, EnergyChem, 2022, 4, 100086 CrossRef CAS.
- C. I. Ezugwu, S. Liu, C. Li, S. Zhuiykov, S. Roy and F. Verpoort, Engineering metal-organic frameworks for efficient photocatalytic conversion of CO2 into solar fuels, Coord. Chem. Rev., 2022, 450, 214245 CrossRef CAS.
- Z. Sun, N. Talreja, H. Tao, J. Texter, M. Muhler, J. Strunk and J. Chen, Catalysis of carbon dioxide photoreduction on nanosheets: fundamentals and challenges, Angew. Chem., Int. Ed., 2018, 57, 7610–7627 CrossRef CAS PubMed.
- Y. Zhao, L. Zheng, D. Jiang, W. Xia, X. Xu, Y. Yamauchi, J. Ge and J. Tang, Nanoengineering metal–organic framework-based materials for use in electrochemical CO2 reduction reactions, Small, 2021, 17, 2006590 CrossRef CAS PubMed.
- J. Du, Z.-L. Lang, Y.-Y. Ma, H.-Q. Tan, B.-L. Liu, Y.-H. Wang, Z.-H. Kang and Y.-G. Li, Polyoxometalate-based electron transfer modulation for efficient electrocatalytic carbon dioxide reduction, Chem. Sci., 2020, 11, 3007–3015 RSC.
- X. Duan, J. Xu, Z. Wei, J. Ma, S. Guo, S. Wang, H. Liu and S. Dou, Metal-free carbon materials for CO2 electrochemical reduction, Adv. Mater., 2017, 29, 1701784 CrossRef PubMed.
- Z.-Z. Wu, F.-Y. Gao and M.-R. Gao, Regulating the oxidation state of nanomaterials for electrocatalytic CO2 reduction, Energy Environ. Sci., 2021, 14, 1121–1139 RSC.
- D. Gao, T. Liu, G. Wang and X. Bao, Structure sensitivity in single-atom catalysis toward CO2 electroreduction, ACS Energy Lett., 2021, 6, 713–727 CrossRef CAS.
- J. Gu, W. Chen, G.-G. Shan, G. Li, C. Sun, X.-L. Wang and Z. Su, The roles of polyoxometalates in photocatalytic reduction of carbon dioxide, Mater. Today, Energy, 2021, 21, 100760 CAS.
- G. Gao, F. Li, L. Xu, X. Liu and Y. Yang, CO2 coordination by inorganic polyoxoanion in water, J. Am. Chem. Soc., 2008, 130, 10838–10839 CrossRef CAS PubMed.
- S. H. Szczepankiewicz, C. M. Ippolito, B. P. Santora, T. J. Van de Ven, G. A. Ippolito, L. Fronckowiak, F. Wiatrowski, T. Power and M. Kozik, Interaction of carbon dioxide with transition-metal-substituted heteropolyanions in nonpolar solvents. Spectroscopic evidence for complex formation, Inorg. Chem., 1998, 37, 4344–4352 CrossRef CAS PubMed.
-
J. Paul, P. Page, P. Sauers, K. Ertel, C. Pasternak, W. Lin and M. Kozik, Transition-Metal-Substituted Heteropoly Anions in Nonpolar Solvents—Structures and Interaction with Carbon Dioxide, Springer US, Boston, MA, 2002, pp. 205–215 Search PubMed.
- H. Yang, D. Yang, Y. Zhou and X. Wang, Polyoxometalate Interlayered Zinc–Metallophthalocyanine Molecular Layer Sandwich as Photocoupled Electrocatalytic CO2 Reduction Catalyst, J. Am. Chem. Soc., 2021, 143, 13721–13730 CrossRef CAS PubMed.
- Y.-R. Wang, Q. Huang, C.-T. He, Y. Chen, J. Liu, F.-C. Shen and Y.-Q. Lan, Oriented electron transmission in polyoxometalate-metalloporphyrin organic framework for highly selective electroreduction of CO2, Nat. Commun., 2018, 9, 4466 CrossRef PubMed.
- M. L. Sun, Y. R. Wang, W. W. He, R. L. Zhong, Q. Z. Liu, S. Xu, J. M. Xu, X. L. Han, X. Ge, S. L. Li, Y.-Q. Lan, A. M. Al-Enizi, A. Nafady and S. Ma, Efficient electron transfer from electron-sponge polyoxometalate to single-metal site metal–organic frameworks for highly selective electroreduction of carbon dioxide, Small, 2021, 17, 2100762 CrossRef CAS PubMed.
- Q. Huang, Q. Niu, X.-F. Li, J. Liu, S.-N. Sun, L.-Z. Dong, S.-L. Li, Y.-P. Cai and Y.-Q. Lan, Demystifying the roles of single metal site and cluster in CO2 reduction via light and electric dual-responsive polyoxometalate-based metal-organic frameworks, Sci. Adv., 2022, 8, eadd5598 CrossRef CAS PubMed.
- S.-X. Guo, F. Li, L. Chen, D. R. MacFarlane and J. Zhang, Polyoxometalate-promoted electrocatalytic CO2 reduction at nanostructured silver in dimethylformamide, ACS Appl. Mater. Interfaces, 2018, 10, 12690–12697 CrossRef CAS PubMed.
- S. X. Guo, D. R. MacFarlane and J. Zhang, Bioinspired Electrocatalytic CO2 Reduction by Bovine Serum Albumin-Capped Silver Nanoclusters Mediated by [α-SiW12O40]4−, ChemSusChem, 2016, 9, 80–87 CrossRef CAS PubMed.
- D. Zang, Q. Li, G. Dai, M. Zeng, Y. Huang and Y. Wei, Interface engineering of Mo8/Cu heterostructures toward highly selective electrochemical reduction of carbon dioxide into acetate, Appl. Catal., B, 2021, 281, 119426 CrossRef CAS.
- M. H. Hasan, T. M. I. Mahlia, M. Mofijur, I. Rizwanul Fattah, F. Handayani, H. C. Ong and A. Silitonga, A comprehensive review on the recent development of ammonia as a renewable energy carrier, Energies, 2021, 14, 3732 CrossRef CAS.
- M. Kitano, Y. Inoue, Y. Yamazaki, F. Hayashi, S. Kanbara, S. Matsuishi, T. Yokoyama, S.-W. Kim, M. Hara and H. Hosono, Ammonia synthesis using a stable electride as an electron donor and reversible hydrogen store, Nat. Chem., 2012, 4, 934–940 CrossRef CAS PubMed.
- G. Qing, R. Ghazfar, S. T. Jackowski, F. Habibzadeh, M. M. Ashtiani, C.-P. Chen, M. R. Smith III and T. W. Hamann, Recent advances and challenges of electrocatalytic N2 reduction to ammonia, Chem. Rev., 2020, 120, 5437–5516 CrossRef CAS PubMed.
- X. Cui, C. Tang and Q. Zhang, A review of electrocatalytic reduction of dinitrogen to ammonia under ambient conditions, Adv. Energy Mater., 2018, 8, 1800369 CrossRef.
- N. Cao and G. Zheng, Aqueous electrocatalytic N2 reduction under ambient conditions, Nano Res., 2018, 11, 2992–3008 CrossRef CAS.
- G. Lin, Q. Ju, X. Guo, W. Zhao, S. Adimi, J. Ye, Q. Bi, J. Wang, M. Yang and F. Huang, Intrinsic electron localization of metastable MoS2 boosts electrocatalytic nitrogen reduction to ammonia, Adv. Mater., 2021, 33, 2007509 CrossRef CAS PubMed.
- C. Liu, X. Guo, Z.-F. Huang, J. Li, L. Gan, L. Pan, C. Shi, X. Zhang, G. Yang and J.-J. Zou, Surface defect-regulated PdCu/TiO2−x promoting efficient electrocatalytic nitrogen reduction, Mater. Chem. Front., 2022, 6, 2190–2200 RSC.
- Y. Ren, C. Yu, X. Tan, Q. Wei, Z. Wang, L. Ni, L. Wang and J. Qiu, Strategies to activate inert nitrogen molecules for efficient ammonia electrosynthesis: current status, challenges, and perspectives, Energy Environ. Sci., 2022, 15, 2776–2805 RSC.
- Y. Sun, Y. Wang, H. Li, W. Zhang, X.-M. Song, D.-M. Feng, X. Sun, B. Jia, H. Mao and T. Ma, Main group metal elements for ambient-condition electrochemical nitrogen reduction, J. Energy Chem., 2021, 62, 51–70 CrossRef CAS.
- W. Liao, L. Qi, Y. Wang, J. Qin, G. Liu, S. Liang, H. He and L. Jiang, Interfacial engineering promoting electrosynthesis of ammonia over Mo/phosphotungstic acid with high performance, Adv. Funct. Mater., 2021, 31, 2009151 CrossRef CAS.
- C. Feng, J. Liu, Q. Li, L. Ji, P. Wu, X. Yuan, H. Hu, H. Y. Jiang and G. Xue, Fabricating Ag/PW12/Zr-mTiO2 Composite via Doping and Interface Engineering: An Efficient Catalyst with Bifunctionality
in Photo-and Electro-Driven Nitrogen Reduction Reactions, Adv. Sustainable Syst., 2022, 6, 2100307 CrossRef CAS.
- L. Gao, F. Wang, M. Yu, F. Wei, J. Qi, S. Lin and D. Xie, A novel phosphotungstic acid-supported single metal atom catalyst with high activity and selectivity for the synthesis of NH3 from electrochemical N2 reduction: a DFT prediction, J. Mater. Chem. A, 2019, 7, 19838–19845 RSC.
- L. Lin, L. Gao, K. Xie, R. Jiang and S. Lin, Ru–polyoxometalate as a single-atom electrocatalyst for N2 reduction to NH3 with high selectivity at applied voltage: a perspective from DFT studies, Phys. Chem. Chem. Phys., 2020, 22, 7234–7240 RSC.
- S. H. Talib, X. Yu, Z. Lu, K. Ahmad, T. Yang, H. Xiao and J. Li, A polyoxometalate cluster-based single-atom catalyst for NH3 synthesis via an enzymatic mechanism, J. Mater. Chem. A, 2022, 10, 6165–6177 RSC.
- Y. Wang, R.-C. Qin, D. Wang and C.-G. Liu, Reduction of N2 to NH3 catalyzed by a Keggin-type polyoxometalate-supported dual-atom catalyst, Inorg. Chem. Front., 2022, 9, 845–858 RSC.
- L. Lin, F. Wei, R. Jiang, Y. Huang and S. Lin, The role of central heteroatom in electrochemical nitrogen reduction catalyzed by polyoxometalate-supported single-atom catalyst, Nano Res., 2023, 16, 309–317 CrossRef CAS.
- L. Zeng, Z. Qiao, X. Peng, Z. Liu, Z. Li, B. Yang, L. Lei, G. Wu and Y. Hou, Progress in Mo/W-based electrocatalysts for nitrogen reduction to ammonia under ambient conditions, Chem. Commun., 2022, 58, 2096–2111 RSC.
- H.-Q. Yin, L.-L. Yang, H. Sun, H. Wang, Y.-J. Wang, M. Zhang, T.-B. Lu and Z.-M. Zhang, W/Mo-polyoxometalate-derived electrocatalyst for high-efficiency nitrogen fixation, Chin. Chem. Lett., 2023, 34, 107337 CrossRef CAS.
- X. Wang, Z. Feng, B. Xiao, J. Zhao, H. Ma, Y. Tian, H. Pang and L. Tan, Polyoxometalate-based metal–organic framework-derived bimetallic hybrid materials for upgraded electrochemical reduction of nitrogen, Green Chem., 2020, 22, 6157–6169 RSC.
- C. Wang, M. Yang, X. Wang, H. Ma, Y. Tian, H. Pang, L. Tan and K. Gao, Hierarchical CoS2/MoS2 flower-like heterostructured arrays derived from polyoxometalates for efficient electrocatalytic nitrogen reduction under ambient conditions, J. Colloid Interface Sci., 2022, 609, 815–824 CrossRef CAS PubMed.
- M.-L. Yang, Z.-X. Jin, X.-X. Cao, X.-M. Wang, H.-Y. Ma, H.-J. Pang and G.-X. Yang, Polyoxometalates-derived ternary metal oxides electrocatalyst for N2 reduction under ambient conditions, Tungsten, 2023 DOI:10.1007/s42864-023-00206-4.
- L. G. H. Staaf, P. Lundgren and P. Enoksson, Present and future supercapacitor carbon electrode materials for improved energy storage used in intelligent wireless sensor systems, Nano Energy, 2014, 9, 128–141 CrossRef CAS.
- S. Peng, L. Li, H. B. Wu, S. Madhavi and X. W. D. Lou, Controlled Growth of NiMoO4Nanosheet and Nanorod Arrays on Various Conductive Substrates as Advanced Electrodes for Asymmetric Supercapacitors, Adv. Energy Mater., 2015, 5, 1401172 CrossRef.
- S. Zhang and N. Pan, Supercapacitors performance evaluation, Adv. Energy Mater., 2015, 5, 1401401 CrossRef.
- P. Á. Szilágyi and A. J. Sobrido, Performance and potential of porous carbons derived of electrospun metal–organic frameworks for supercapacitor applications, J. Energy Chem., 2022, 73, 348–353 CrossRef.
- M. Genovese and K. Lian, Polyoxometalate modified pine cone biochar carbon for supercapacitor electrodes, J. Mater. Chem. A, 2017, 5, 3939–3947 RSC.
- D. Cheng, K. Li, H. Zang and J. Chen, Recent Advances on Polyoxometalate-Based Ion-Conducting Electrolytes for Energy-Related Devices, Energy Environ. Mater., 2022, 6, e12341 CrossRef.
- M. A. Garakani, S. Bellani, V. Pellegrini, R. Oropesa-Nuñez, A. E. D. R. Castillo, S. Abouali, L. Najafi, B. Martín-García, A. Ansaldo, P. Bondavalli, C. Demirci, V. Romano, E. Mantero, L. Marasco, M. Prato, G. Bracciale and F. Bonaccorso, Scalable spray-coated graphene-based electrodes for high-power electrochemical double-layer capacitors operating over a wide range of temperature, Energy Storage Mater., 2021, 34, 1–11 CrossRef.
- Y. Yusran, H. Li, X. Guan, D. Li, L. Tang, M. Xue, Z. Zhuang, Y. Yan, V. Valtchev, S. Qiu and Q. Fang, Exfoliated Mesoporous 2D Covalent Organic Frameworks for High-Rate Electrochemical Double-Layer Capacitors, Adv. Mater., 2020, 32, 1907289 CrossRef CAS PubMed.
- L. Wei and G. Yushin, Nanostructured activated carbons from natural precursors for electrical double layer capacitors, Nano Energy, 2012, 1, 552–565 CrossRef CAS.
- H.-Y. Chen, R. Al-Oweini, J. Friedl, C. Y. Lee, L. Li, U. Kortz, U. Stimming and M. Srinivasan, A novel SWCNT-polyoxometalate nanohybrid material as an electrode for electrochemical supercapacitors, Nanoscale, 2015, 7, 7934–7941 RSC.
- M. Wang, Y. Zhang, T. Zhang, Y. Li, M. Cui, X. Cao, Y. Lu, D. Peng, W. Liu, X. Liu, T. Wang and Y. Huang, Confinement of single polyoxometalate clusters in molecular-scale cages for improved flexible solid-state supercapacitors, Nanoscale, 2020, 12, 11887–11898 RSC.
- P. Yang, J. Xie, L. Wang, X. Chen, F. Wu and Y. Huang, Coaxial Cable-Like Carbon Nanotubes-Based Active Fibers for Highly Capacitive and Stable Supercapacitor, Adv. Mater. Interfaces, 2020, 7, 2000949 CrossRef CAS.
- D. Zhou and B.-H. Han, Graphene-Based Nanoporous Materials Assembled by Mediation of Polyoxometalate Nanoparticles, Adv. Funct. Mater., 2010, 20, 2717–2722 CrossRef CAS.
- J. Suárez-Guevara, V. Ruiz and P. Gomez-Romero, Hybrid energy storage: high voltage aqueous supercapacitors based on activated carbon–phosphotungstate hybrid materials, J. Mater. Chem. A, 2014, 2, 1014–1021 RSC.
- P. Palomino, J. Suarez-Guevara, M. Olivares-Marín, V. Ruiz, D. P. Dubal, P. Gómez-Romero, D. Tonti and E. Enciso, Influence of texture in hybrid carbon-phosphomolybdic acid materials on their performance as electrodes in supercapacitors, Carbon, 2017, 111, 74–82 CrossRef CAS.
- C. Hu, E. Zhao, N. Nitta, A. Magasinski, G. Berdichevsky and G. Yushin, Aqueous solutions of acidic ionic liquids for enhanced stability of polyoxometalate-carbon supercapacitor electrodes, J. Power Sources, 2016, 326, 569–574 CrossRef CAS.
- S. Maity, A. A. Vannathan, T. Kella, D. Shee, P. P. Das and S. S. Mal, Electrochemical performance of activated carbon-supported vanadomolybdates electrodes for energy conversion, Ceram. Int., 2021, 47, 27132–27141 CrossRef CAS.
- M. Yang, B. G. Choi, S. C. Jung, Y.-K. Han, Y. S. Huh and S. B. Lee, Polyoxometalate-coupled Graphene via Polymeric Ionic Liquid Linker for Supercapacitors, Adv. Funct. Mater., 2014, 24, 7301–7309 CrossRef CAS.
- Y. Hou, D. Chai, B. Li, H. Pang, H. Ma, X. Wang and L. Tan, Polyoxometalate-Incorporated Metallacalixarene@Graphene
Composite Electrodes for High-Performance Supercapacitors, ACS Appl. Mater. Interfaces, 2019, 11, 20845–20853 CrossRef CAS PubMed.
- D. Pakulski, A. Gorczyński, W. Czepa, Z. Liu, L. Ortolani, V. Morandi, V. Patroniak, A. Ciesielski and P. Samorì, Novel Keplerate type polyoxometalate-surfactant-graphene hybrids as advanced electrode materials for supercapacitors, Energy Storage Mater., 2019, 17, 186–193 CrossRef.
- S. Yu, M. Yang, Y. Liu and M. Liu, Recent advances in separation membranes based on porous organic molecular materials, Mater. Chem. Front., 2023, 7, 3560–3575 RSC.
- S. Zeb, G. Sun, Y. Nie, H. Xu, Y. Cui and X. Jiang, Advanced developments in nonstoichiometric tungsten oxides for electrochromic applications, Mater. Adv., 2021, 2, 6839–6884 RSC.
- M. Wang, Y. Yu, M. Cui, X. Cao, W. Liu, C. Wu, X. Liu, T. Zhang and Y. Huang, Development of polyoxometalate-anchored 3D hybrid hydrogel for high-performance flexible pseudo-solid-state supercapacitor, Electrochim. Acta, 2020, 329, 135181 CrossRef CAS.
- J. Kim, Y. Kim, J. Yoo, G. Kwon, Y. Ko and K. Kang, Organic batteries for a greener rechargeable world, Nat. Rev. Mater., 2023, 8, 54–70 CrossRef.
- Y. Zhang, L. Tao, C. Xie, D. Wang, Y. Zou, R. Chen, Y. Wang, C. Jia and S. Wang, Defect engineering on electrode materials for rechargeable batteries, Adv. Mater., 2020, 32, 1905923 CrossRef CAS PubMed.
- F. Cheng, J. Liang, Z. Tao and J. Chen, Functional materials for rechargeable batteries, Adv. Mater., 2011, 23, 1695–1715 CrossRef CAS PubMed.
- R. Raccichini, A. Varzi, S. Passerini and B. Scrosati, The role of graphene for electrochemical energy storage, Nat. Mater., 2015, 14, 271–279 CrossRef CAS PubMed.
- M. M. Rahman, J.-Z. Wang, M. F. Hassan, S. Chou, Z. Chen and H. K. Liu, Nanocrystalline porous α-LiFeO2–C composite—an environmentally friendly cathode for the lithium-ion battery, Energy Environ. Sci., 2011, 4, 952–957 RSC.
- K. Jia, J. Ma, J. Wang, Z. Liang, G. Ji, Z. Piao, R. Gao, Y. Zhu, Z. Zhuang, G. Zhou and H. M. Cheng, Long-Life Regenerated LiFePO4 from Spent Cathode by Elevating the d-Band Center of Fe, Adv. Mater., 2022, 35, 2208034 CrossRef PubMed.
- D. Liu, Z. J. Liu, X. Li, W. Xie, Q. Wang, Q. Liu, Y. Fu and D. He, Group IVA Element (Si, Ge, Sn)-Based Alloying/Dealloying Anodes as Negative Electrodes for Full-Cell Lithium-Ion Batteries, Small, 2017, 13, 1702000 CrossRef PubMed.
- M. Zheng, H. Tang, L. Li, Q. Hu, L. Zhang, H. Xue and H. Pang, Hierarchically Nanostructured Transition Metal Oxides for Lithium-Ion Batteries, Adv. Sci., 2018, 5, 1700592 CrossRef PubMed.
- S. Liang, Y. J. Cheng, J. Zhu, Y. Xia and P. Müller-Buschbaum, A Chronicle Review of Nonsilicon (Sn, Sb, Ge)-Based Lithium/Sodium-Ion Battery Alloying Anodes, Small Methods, 2020, 4, 2000218 CrossRef CAS.
- H. Hou, X. Qiu, W. Wei, Y. Zhang and X. Ji, Carbon Anode Materials for Advanced Sodium-Ion Batteries, Adv. Energy Mater., 2017, 7, 1602898 CrossRef.
- H. Wang, S. Hamanaka, Y. Nishimoto, S. Irle, T. Yokoyama, H. Yoshikawa and K. Awaga, In Operando X-ray Absorption Fine Structure Studies of Polyoxometalate Molecular Cluster Batteries: Polyoxometalates as Electron Sponges, J. Am. Chem. Soc., 2012, 134, 4918–4924 CrossRef CAS PubMed.
- E. Ni, S. Uematsu and N. Sonoyama, Anderson type polyoxomolybdate as cathode material of lithium battery and its reaction mechanism, J. Power Sources, 2014, 267, 673–681 CrossRef CAS.
- J.-J. Chen, M. D. Symes, S.-C. Fan, M.-S. Zheng, H. N. Miras, Q.-F. Dong and L. Cronin, High-Performance Polyoxometalate-Based Cathode Materials for Rechargeable Lithium-Ion Batteries, Adv. Mater., 2015, 27, 4649–4654 CrossRef CAS PubMed.
- J. Wang, L. Wang, C. Liu, Y. Wang, F. Ye, W. Yan and B. Liu, Polyoxovanadate ionic crystals with open tunnels stabilized by macrocations for lithium-ion storage, Nano Res., 2023, 16, 9267–9272 CrossRef CAS.
- J. Wang, Y. Liu, Q. Sha, D. Cao, H. Hu, T. Shen, L. He and Y.-F. Song, Electronic Structure Reconfiguration of Self-Supported Polyoxometalate-Based Lithium-Ion Battery Anodes for Efficient Lithium Storage, ACS Appl. Mater. Interfaces, 2021, 14, 1169–1176 CrossRef PubMed.
- J. Hu, Y. Ji, W. Chen, C. Streb and Y.-F. Song, “Wiring” redox-active polyoxometalates to carbon nanotubes using a sonication-driven periodic functionalization strategy, Energy Environ. Sci., 2016, 9, 1095–1101 RSC.
- L. Huang, J. Hu, Y. Ji, C. Streb and Y.-F. Song, Pyrene-Anderson-Modified CNTs as Anode Materials for Lithium-Ion Batteries, Chem. Eur. J., 2015, 21, 18799–18804 CrossRef CAS PubMed.
- W. Chen, L. Huang, J. Hu, T. Li, F. Jia and Y.-F. Song, Connecting carbon nanotubes to polyoxometalate clusters for engineering high-performance anode materials, Phys. Chem. Chem. Phys., 2014, 16, 19668–19673 RSC.
- K. Kume, N. Kawasaki, H. Wang, T. Yamada, H. Yoshikawa and K. Awaga, Enhanced capacitor effects in polyoxometalate/graphene nanohybrid materials: a synergetic approach to high performance energy storage, J. Mater. Chem. A, 2014, 2, 3801–3807 RSC.
- J. Xie, Y. Zhang, Y. Han and C. Li, High-Capacity Molecular Scale Conversion Anode Enabled by Hybridizing Cluster-Type Framework of High Loading with Amino-Functionalized Graphene, ACS Nano, 2016, 10, 5304–5313 CrossRef CAS PubMed.
- F.-C. Shen, Y.-R. Wang, S.-L. Li, J. Liu, L.-Z. Dong, T. Wei, Y.-C. Cui, X. L. Wu, Y. Xu and Y.-Q. Lan, Self-assembly of polyoxometalate/reduced graphene oxide composites induced by ionic liquids as a high-rate cathode for batteries: “killing two birds with one stone”, J. Mater. Chem. A, 2018, 6, 1743–1750 RSC.
- J.-H. Liu, M.-Y. Yu, J. Yang, Y.-Y. Liu and J.-F. Ma, Polyoxometalate-based complex/graphene for high-rate lithium-ion batteries, Microporous Mesoporous Mater., 2021, 310, 110666 CrossRef CAS.
- H. Chao, H. Qin, M. Zhang, Y. Huang, L. Cao, H. Guo, K. Wang, X. Teng, J. Cheng, Y. Lu, H. Hu and M. Wu, Boosting the Pseudocapacitive and High Mass-Loaded Lithium/Sodium Storage through Bonding Polyoxometalate Nanoparticles on MXene Nanosheets, Adv. Funct. Mater., 2021, 31, 2007636 CrossRef CAS.
- H. Chao, Y. Li, Y. Lu, Y. Yao, Y. Zhu, H. Yang, K. Wang, Y. Wan, Q. Xu, L. Guan, H. Hu and M. Wu, MXene-mediated regulation of local electric field surrounding polyoxometalate nanoparticles for improved lithium storage, Sci. China Mater., 2022, 65, 2958–2966 CrossRef CAS.
- Y. Yue, Y. Li, Z. Bi, G. M. Veith, C. A. Bridges, B. Guo, J. Chen, D. R. Mullins, S. P. Surwade and S. M. Mahurin, A POM–organic framework anode for Li-ion battery, J. Mater. Chem. A, 2015, 3, 22989–22995 RSC.
- Q. Huang, T. Wei, M. Zhang, L.-Z. Dong, A. M. Zhang, S.-L. Li, W.-J. Liu, J. Liu and Y.-Q. Lan, A highly stable polyoxometalate-based metal–organic framework with π–π stacking for enhancing lithium ion battery performance, J. Mater. Chem. A, 2017, 5, 8477–8483 RSC.
- Z. Wang, R. Bi, J. Liu, K. Wang, F. Mao, H. Wu, Y. Bu and N. Song, Polyoxometalate-based Cu/Zn-MOFs with diverse stereo dimensions as anode materials in lithium ion batteries, Chem. Eng. J., 2021, 404, 127117 CrossRef CAS.
- T. Wei, M. Zhang, P. Wu, Y.-J. Tang, S.-L. Li, F.-C. Shen, X.-L. Wang, X.-P. Zhou and Y.-Q. Lan, POM-based metal-organic framework/reduced graphene oxide nanocomposites with hybrid behavior of battery-supercapacitor for superior lithium storage, Nano Energy, 2017, 34, 205–214 CrossRef CAS.
- M. Zhang, A.-M. Zhang, X.-X. Wang, Q. Huang, X. Zhu, X.-L. Wang, L.-Z. Dong, S.-L. Li and Y.-Q. Lan, Encapsulating ionic liquids into POM-based MOFs to improve their conductivity for superior lithium storage, J. Mater. Chem. A, 2018, 6, 8735–8741 RSC.
- L. Ni, G. Yang, Y. Liu, Z. Wu, Z. Ma, C. Shen, Z. Lv, Q. Wang, X. Gong and J. Xie, Self-assembled polyoxometalate nanodots as bidirectional cluster catalysts for polysulfide/sulfide redox conversion in lithium–sulfur batteries, ACS Nano, 2021, 15, 12222–12236 CrossRef CAS PubMed.
- J. Lei, X.-X. Fan, T. Liu, P. Xu, Q. Hou, K. Li, R.-M. Yuan, M.-S. Zheng, Q.-F. Dong and J.-J. Chen, Single-dispersed polyoxometalate clusters embedded on multilayer graphene as a bifunctional electrocatalyst for efficient Li-S batteries, Nat. Commun., 2022, 13, 202 CrossRef CAS PubMed.
- K. Liang, H. Zhao, J. Li, X. Huang, S. Jia, W. Chen and Y. Ren, Engineering Crystal Growth and Surface Modification of Na3V2(PO4)2F3 Cathode for High-Energy-Density Sodium-Ion Batteries, Small, 2023, 19, 2207562 CrossRef CAS PubMed.
- J. Liu, Z. Chen, S. Chen, B. Zhang, J. Wang, H. Wang, B. Tian, M. Chen, X. Fan and Y. Huang, “Electron/ion sponge”-like V-based polyoxometalate: toward high-performance cathode for rechargeable sodium ion batteries, ACS Nano, 2017, 11, 6911–6920 CrossRef CAS PubMed.
- T. C. Nagaiah, D. Gupta, S. D. Adhikary, A. Kafle and D. Mandal, Tuning polyoxometalate composites with carbonaceous materials towards oxygen bifunctional activity, J. Mater. Chem. A, 2021, 9, 9228–9237 RSC.
|
This journal is © the Partner Organisations 2024 |
Click here to see how this site uses Cookies. View our privacy policy here.