DOI:
10.1039/D3NH00578J
(Review Article)
Nanoscale Horiz., 2024,
9, 365-383
Advancing piezoelectric 2D nanomaterials for applications in drug delivery systems and therapeutic approaches
Received
20th December 2023
, Accepted 2nd January 2024
First published on 8th January 2024
Abstract
Precision drug delivery and multimodal synergistic therapy are crucial in treating diverse ailments, such as cancer, tissue damage, and degenerative diseases. Electrodes that emit electric pulses have proven effective in enhancing molecule release and permeability in drug delivery systems. Moreover, the physiological electrical microenvironment plays a vital role in regulating biological functions and triggering action potentials in neural and muscular tissues. Due to their unique noncentrosymmetric structures, many 2D materials exhibit outstanding piezoelectric performance, generating positive and negative charges under mechanical forces. This ability facilitates precise drug targeting and ensures high stimulus responsiveness, thereby controlling cellular destinies. Additionally, the abundant active sites within piezoelectric 2D materials facilitate efficient catalysis through piezochemical coupling, offering multimodal synergistic therapeutic strategies. However, the full potential of piezoelectric 2D nanomaterials in drug delivery system design remains underexplored due to research gaps. In this context, the current applications of piezoelectric 2D materials in disease management are summarized in this review, and the development of drug delivery systems influenced by these materials is forecast.
1 Introduction
At present, an estimated 90% of pharmaceuticals are administered orally, yet they frequently exhibit suboptimal efficacy.1 In response, there has been a concerted effort to develop sophisticated drug delivery systems aimed at augmenting treatment effectiveness.2 This development often incorporates additional physical stimulations in the design of controlled drug delivery mechanisms, such as thermal,3 magnetic,4 and electrical modalities.5 Intrinsic electric fields within human physiology are crucial for modulating biological functions and eliciting action potentials in neural and muscular systems.6–8 Electric pulses emanating from electrodes have been shown to enhance both molecular release and permeation in these systems.9–12 However, the current dependence on wired and battery-powered electrodes impedes precise spatial electrical stimulation.13,14
The piezoelectric effect refers to the phenomenon where specific materials generate an electrical charge upon experiencing mechanical stress. This occurrence is attributed to the polarization of molecules within the material structure, leading to the emergence of an electrical potential. Conversely, these piezoelectric materials also exhibit the capability to undergo mechanical deformation when subjected to an electrical current, illustrating the bidirectional nature of this effect. Fundamentally, the piezoelectric effect represents a transduction mechanism, converting mechanical energy into electrical energy and vice versa, thus bridging the domains of mechanical and electrical phenomena.15,16
Piezoelectric materials have been widely applied in energy sources,17 actuation,18,19 sensing,14,18,20,21 environmental protection,22 and catalysis.23,24 With the verification of their biocompatibility, piezoelectric materials have attracted considerable attention in biomedical applications.25 Usually, some drugs need to be transferred to specific tissues/organs, including brain tissues and concealed tumors.26–29 Compared with wearable or implantable bulk piezoelectric materials, nanoscale piezoelectric materials can be precisely delivered to the targeted tissue via the circulatory system.29 Piezoelectric nanomaterials can be remotely activated by external mechanical stress (e.g., ultrasound) to produce electrical charges in situ and achieve noninvasive drug release with better spatial resolution.16,30–32
Among piezoelectric nanomaterials, two-dimensional (2D) nanosheets mimic natural membranes33 and enhance the bionano interactions between the nanosheet surface and biosurface,34 including proteins and cells.35 2D nanosheets, sheet-like planar shapes under weak van der Waals forces, have sparked great potential in biomedical engineering due to their distinctive optical,36 electrical,37 and mechanical characteristics.38–40 The high surface-to-volume ratio of 2D nanosheets enables the loading of various therapeutic payloads via noncovalent interactions.41–43 Moreover, the high surface energy of 2D nanosheets with surface and edge defects offers an essential active site for surface functionalization.44–47 The increased surface tunability allows the construction of targeted drug delivery systems.48
Recent research has also reported the advanced properties of piezoelectric 2D nanosheets in the energy and catalysis fields.49–51 Although these properties meet the demands of drug delivery systems, there is a time lag in the discussion, design, and fabrication due to the gap between different fields. The remotely controlled drug delivery system with spatial-temporal precision has theoretical significance and practical value for future medication.52–54 Thus, this paper offers potential pursued research ideas and directions in the future for drug delivery system fabrication and disease treatment using piezoelectric materials based on previous studies (Scheme 1). This paper also intends to provide a foundation and guidelines for drug delivery system investigation and development.
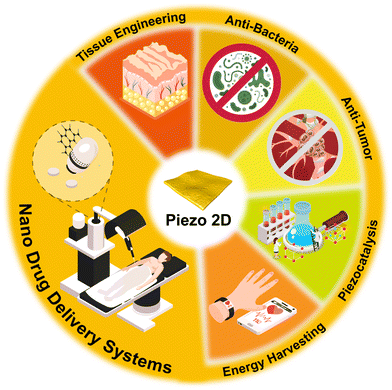 |
| Scheme 1 New evidence for piezoelectric nanomaterials across energy harvesting, piezocatalysis, and biomedicine fields, guiding and supporting future drug delivery system development. | |
2 Piezoelectricity of representative 2D materials
Piezoelectric bulk materials show promise in transducers for human–machine interfaces55 and electroactive scaffolds for tissue regeneration.56,57 Conversely, nanoscale piezoelectric materials excel in targeting specific body sites and penetrating cytomembranes to create internal electrical fields crucial for cell fate determination.16 Notably, 2D nanosheets, characterized by higher piezoelectric coefficients and greater surface-to-volume ratios,58 fall into three categories: ‘flattened’ bulk materials, ‘stripped’ asymmetrical monolayers from symmetrical bulk materials, and ‘decorated’ symmetrical 2D nanosheets with interfacial polar symmetry.59 Additionally, piezoelectric bioactive molecules, including amino acids, peptides, and proteins, have potential in developing engineered healthcare materials.
2.1 Traditional piezoelectric 2D materials
In the realm of conventional bulk piezoelectric materials, these materials are primarily classified into two categories: piezoelectric polymers and ceramics. Among piezoelectric polymers, notable examples include polyvinylidene fluoride (PVDF), poly(vinylidene fluoride-trifluoroethylene) (P(VDF-TrFE)), and polyvinyl chloride. Conversely, barium titanate (BaTiO3) and zinc oxide (ZnO) stand out as quintessential piezoelectric ceramics. PVDF is distinguished by its exceptional flexibility, straightforward processing methodology, and satisfactory biocompatibility. This polymer is characterized by its ability to exist in five distinct crystalline phase configurations, namely, α, β, γ, δ, and ε, with the α- and β-phases being the most significant. The α-phase of PVDF, owing to the reverse parallel arrangement of its dipoles, exhibits minimal piezoelectricity. However, during the electrospinning process, PVDF undergoes mechanical stretching coupled with in situ poling, resulting in a transformation of the nonpolar α-phase into a highly polar β-phase.60 The parallel-arranged dipoles in β-phase PVDF result in a strong dipole moment per unit cell and enhanced piezoelectric characteristics.61
Nanopillar morphologies are achievable on PVDF film surfaces,62 yet 2D nanosheets of PVDF and other piezoelectric polymers remain undeveloped, likely due to viscoelastic difference-induced interfacial instabilities in PVDF nanofibers.63 Nevertheless, P(VDF-TrFE) nanotubes and spheres are fabricable.16 Advancements in nanoscale piezoelectric polymers, particularly through the Langmuir–Blodgett technique, have yielded ultrathin P(VDF-TrFE) films with a minimum average thickness of 2.072 nm.64 This nanoscale innovation is set to enhance piezoelectric polymers’ applications in precision medicine, spanning scaffolds to drug delivery systems and pharmaceuticals.
Piezoelectric materials ideally require high electromechanical (k33) and piezoelectric (d33) coefficients. Yet, piezoelectric polymers often have lower k33 and d33 values compared to commonly used ferroelectric ceramics and crystals like Pb(ZrTi)O3.65 Interestingly, certain inorganic ceramics, such as hexagonal cadmium sulfide and ZnO, maintain piezoelectric properties even when reduced to nanoscale thicknesses. Specifically, 2D nanosheets of cadmium sulfide and ZnO show enhanced vertical d33 compared to their bulk counterparts, likely due to reduced carrier concentration and altered local polarization.16 Additionally, the piezoelectric efficiency of synthesized ZnO has been confirmed, revealing structure-dependent performance, with higher surface-to-volume ratio structures exhibiting superior efficacy.
For polymer–ceramic composites, a Ti3C2Tx MXene anchoring method has been recently proposed to manipulate the intermolecular interactions within the all-trans conformation of a polymer matrix.66 β-Phase programming boosts the piezoelectric response of samarium-doped Pb(Mg1/3Nb2/3)O3–PbTiO3/PVDF composite nanofibers by 160% via Ti3C2Tx 2D nanosheet inclusion.
2.2 Monolayer and few-layer piezoelectric materials
In addition to flattened conventional piezoelectric ceramics, the piezoelectric effect has been observed in 2D nanosheets whose bulk counterparts do not possess piezoelectric properties, such as MoS2. In 2014, Wang et al. first conducted an experimental study on the piezoelectric properties of MoS2 2D nanosheets. Due to the loss of centrosymmetry, the MoS2 2D nanosheets with an odd number of atomic layers produced piezoelectric voltage and current outputs, whereas the even number of layers showed no current. Similarly, piezoelectricity has been verified in transition metal dichalcogenides (TMDCs), such as WSe2. Insulating monolayer hexagonal boron nitride (BN) has also been proven to have piezoelectric properties. Owing to the 2D confinement, these materials display unique electronic, optical, and mechanical properties that are unattainable in their bulk counterparts. Cheon et al. conducted data mining for over 50
000 inorganic crystals and predicted 325 potential piezoelectric monolayers, which significantly extends the scope of 2D piezoelectric materials.
Apart from conventional piezoelectric ceramics, 2D nanosheets like MoS2, which lack piezoelectricity in bulk form, exhibit piezoelectric effects. Wang et al. in 2014 first experimentally demonstrated MoS2 2D nanosheets’ piezoelectric properties,67 where odd-layered sheets generated piezoelectric voltage and current, unlike even-layered ones. This piezoelectricity is also present in transition metal dichalcogenides like WSe2 and in insulating monolayer hexagonal boron nitride (BN).68,69 These 2D materials showcase distinct electronic, optical, and mechanical properties, divergent from their bulk forms.70 Additionally, Cheon et al.'s data mining of over 50
000 inorganic crystals identified 325 potential piezoelectric monolayers, broadening the range of 2D piezoelectric materials.71
Differing from TMDC monolayers, certain 'stripped’ 2D nanosheets like graphene lack asymmetric crystalline structures and gain piezoelectric properties only when chemically modified or structurally defected to disrupt centrosymmetry.72 Moreover, in Schottky junctions—classic interfaces between noble metals and centrosymmetric semiconductors—the built-in electric field within the depletion region can induce polar structures in the semiconductors, thereby generating piezoelectric effects.59
2.3 Bioactive piezoelectric 2D materials
Inside the human body, the principle of piezoelectricity relies on numerous biological counterparts, from basic building blocks, including amino acids and proteins, to highly organized tissues, such as skin, muscle and bone.73
Amino acids.
Amino acids, basic organic compounds, contain a carboxyl (–COOH) group, an amine (–NH2) group, hydrogen, and a specific side functional group (R). Most of them exhibit chirality and thus piezoelectricity. Glycine, unique as a nonchiral amino acid with hydrogen as its side group, crystallizes into α, β, and γ polymorphs. α-Glycine's central symmetry precludes piezoelectricity, whereas β- and γ-glycine's asymmetry confers it.74 Self-assembled glycine 2D nanosheets, created via spin-coating, demonstrate piezoelectricity, a high nonlinear optical coefficient, wide transparency, and high damage threshold in β-glycine.75 An applied force of 0.172 N on these nanosheets yields an open-circuit voltage of 0.45 V. The potential for assembling amino acids into peptides and proteins through dehydration condensation opens avenues for fabricating biological piezoelectric materials.
Peptides and proteins.
Short peptides, created by amino acid linkage, can exhibit piezoelectric properties. Notably, diphenylalanine, a dipeptide of two phenylalanine molecules, stands out as a significant piezoelectric biomolecule. Kholkin et al. synthesized diphenylalanine 2D nanosheets, nanotubes, and nanofibers through solution processes, confirming their piezoelectricity via piezoresponse force microscopy.76 The synthetic polypeptide poly-γ-benzyl-L-glutamate, with an α-helical structure, has demonstrated a piezoelectric coefficient (d14) of 26 pC N−1 when oriented in a magnetic field.77
Extracellular matrix bioactive proteins like collagen, fibrin, and keratin exhibit piezoelectricity, influencing cellular activity regulation. Collagen's piezoelectric properties are sensitive to pH and humidity, which can alter its polar bond activity and crystalline structure,78 leading to varied piezoelectric characteristics based on processing. Emulating biological piezoelectricity, Yang et al. produced an ultrathin collagen fibril nanofilm using small intestinal submucosa through van der Waals exfoliation,79 although its size isn’t customizable. Vivekananthan et al. pioneered a collagen film-based piezoelectric energy generator.80 This device, created by sandwiching a collagen film between two conducting films and laminating with polypropylene, outperforms traditional ceramic/oxide humidity sensors. It’ is a self-powered, non-toxic, eco-friendly piezoelectric biopolymer with notable sensitivity and reliability. Under a 5 N force, it generates a maximum Voc of 50 V and Isc of 250 nA. As a humidity sensor, it demonstrates linear responsiveness between 50 and 90% relative humidity, with a sensitivity of 0.1287 μA %−1 relative humidity.
The challenge of arranging ordered piezoelectric domains has hindered the creation of uniformly distributed piezoelectric 2D nanosheets through natural collagen assembly. However, given their biocompatibility and degradability, bioactive molecule-synthesized piezoelectric 2D nanosheets hold potential in regenerative medicine. Beyond intrinsic piezoelectric properties, Takano et al. discovered a piezoelectric-like effect in myosin via molecular dynamics simulation.81 Mechanical stimuli applied locally on proteins can trigger electrostatic potential changes in remote areas, a process termed piezoelectric allostery. This input/output mechanism implies that mechanical input at a specific site can cause extensive structural changes in proteins, resulting in altered electrostatic potential. Such dynamic transduction could lead to the design of protein-based biomaterials.
2.4 Biocompatibility of piezoelectric 2D materials
Biocompatibility refers to the compatibility of materials with biological systems, particularly focusing on adverse biological reactions and stability within organisms.82 A material with high biocompatibility does not trigger immune responses, inflammation, or other harmful reactions, while maintaining its functionality and performance within the biological environment. Biocompatibility encompasses compatibility with cells, blood, and tissues, and also considers the material's genotoxicity and neurotoxicity.83 Exceptional biocompatibility is a critical prerequisite for clinical applications of all biomaterials.
Lead-based piezoelectric ceramics, such as lead zirconate titanate, despite their excellent electrical properties, are excluded from biomedical applications due to their widespread biotoxicity, especially in the development of drug delivery systems.84 In contrast, lead-free piezoelectric ceramics like BaTiO3 demonstrate superior biocompatibility, making them more suitable for biomedical purposes.85 Additionally, ZnO piezoelectric 2D materials not only circumvent the toxicity issues associated with lead-containing materials but also exhibit properties that enhance cell proliferation.86
Monolayer or few-layer 2D nanomaterials exhibit notable biocompatibility. For instance, 2D BN nanomaterials with graphene-like structures have demonstrated exceptional biocompatibility in both in vitro and in vivo studies. This is primarily attributed to their propensity to adsorb onto cell membrane surfaces without penetrating the cells.87 However, as the size of BN increases to the micrometer scale, its biotoxicity significantly escalates.88 Another category of 2D materials, piezoelectric TMDCs, such as MoS2,89 WS2,90 and TiS2,91 are also widely used in the field of biomaterials and have been confirmed to possess good compatibility with cells, blood, and tissues. Under physiological conditions, unmodified 2D TMDCs face challenges in direct application in drug delivery systems due to inadequate colloidal stability.92 Consequently, combining these piezoelectric 2D nanomaterials with organic coatings to enhance their solubility, dispersibility, and stability can facilitate their acceptance by biological systems. It is important to note that while these piezoelectric 2D nanomaterials do not inherently elicit adverse biological reactions, their interaction with aquatic perfluoroalkyl substances, such as MoS2 and WS2, can lead to significant accumulation in zebrafish, causing oxidative stress in the liver and intestines.93 The biotoxicity arising from such interactions between toxic substances and nanomedicines is a critical concern in the biological application of piezoelectric 2D nanomaterials.
Natural piezoelectric materials (like amino acids, peptides, and proteins) are generally considered biologically safe. However, apart from amino acids and shorter peptides, the unique three-dimensional structures and surface characteristics of longer peptides94 or proteins95 can act as antigenic determinants, triggering recognition and activation by the immune system. Therefore, the antigenicity of naturally derived piezoelectric materials should be a key consideration when selecting them for internal applications. Despite potential antigenicity concerns, bio-derived piezoelectric nanomaterials demonstrate exceptional in vivo degradability. These natural materials decompose into fundamental molecules like water and carbon dioxide under physiological conditions, or are reabsorbed by the body.96 For instance, glycine, a piezoelectric amino acid, is absorbed in the small intestine and breaks down into ammonia and carbon dioxide under the action of the glycine cleavage system enzyme.97 Collagen fibers, another piezoelectric material, exhibit variable degradation times based on their degree of crosslinking. In Sprague-Dawley rats, non-crosslinked collagen scaffolds degrade rapidly within three weeks, whereas those treated with glutaraldehyde maintain structural integrity for up to nine weeks.98 This characteristic is crucial for certain implantable piezoelectric devices, allowing the adjustment of expected degradation times to align with treatment periods, thereby minimizing the trauma associated with secondary surgeries required for device removal.
3 Piezocatalysis-related biomedical uses of piezoelectric 2D nanosheets
Piezoelectric 2D nanosheets are suggested to facilitate redox reactions in catalysis, generating oxidants, antioxidants and gas molecules that have effects on biomedical applications.
3.1 Mechanisms of piezocatalysis
Piezoelectric material catalysis fundamentally involves dipole orientation shifts, leading to bound charge changes. Stress-induced dipole alterations in the catalyst disrupt local free charge concentration, initiating redox reactions.23,99 Piezoelectric 2D nanosheets, with their ultrathin structure, reduce the energy barrier by shortening the charge carrier transport distance (for electrons and holes), thereby enhancing reactant adsorption and activation. These nanosheets are adept at fostering specific reactions while suppressing competing ones, thereby controlling catalytic reaction efficiency and selectivity.100 For instance, Fig. 1(a) illustrates single-layer MoS2 decomposing water to generate reactive oxygen species via spontaneous polarization and catalysis, whereas Fig. 1(b) depicts the decomposition of rhodamine B dye through this mechanism.24
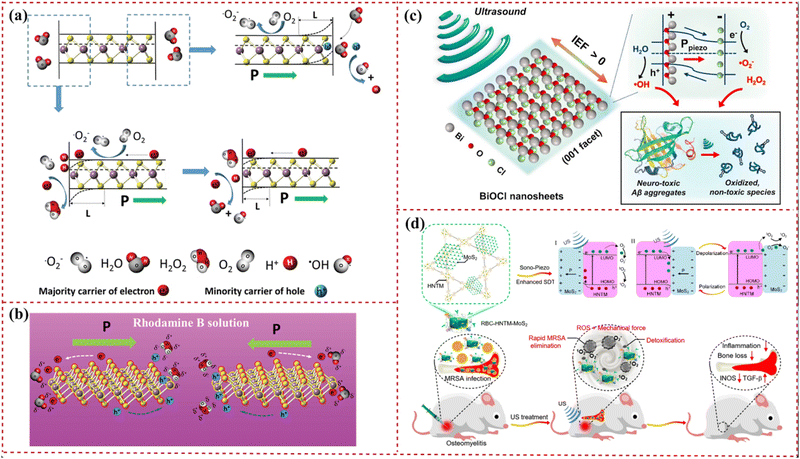 |
| Fig. 1 Schematic illustration of the catalytic effect of piezoelectric 2D nanosheets. (a) Working principle of the degradation activity through the piezocatalytic effect of single-layer MoS2. (b) The reduction and oxidation process of single-layer MoS2 for destroying the organic dye by spatially spontaneous polarization and the catalyzing process. Reprinted with permission from ref. 24. Copyright 2016, Wiley-VCH Verlag GmbH & Co. KGaA, Weinheim. (c) Illustration of piezoelectric dissociation of Alzheimer's β-amyloid (Aβ) aggregates on a bismuth oxychloride 2D nanosheet surface (IEF: internal electric field). Reprinted with permission from ref. 106. Copyright 2020, Elsevier B.V. (d) Sonocatalytic mechanism of hollow metal–organic framework-MoS2 and efficient sonodynamic therapy treatment of osteomyelitis through rapid bacterial elimination and detoxification. Reprinted with permission from ref. 109. Copyright 2022 American Chemical Society. | |
Defect engineering shapes the physicochemical properties of catalysts, enhancing catalysis through atomic rearrangement, charge redistribution, energy band changes, and optimization of intermediate adsorption–desorption and charge/mass transfer.24 This approach has been widely applied in piezocatalysis and cocatalysis.101,102 Combining piezoelectric output with piezocatalysis, piezo-photocatalysis, piezotronics, and piezophototronics has led to advancements in sensing and pollutant degradation,103–105 leveraging the established piezoelectric effect of 2D nanosheets. These nanosheets also show promise in biomedical applications, such as using ultrasound-driven piezocatalytic bismuth oxychloride 2D nanosheets for disintegrating Alzheimer's β-amyloid aggregates into harmless fragments,106 and in antitumor and antibacterial therapies.107,108 Therefore, the exploration and application of catalytic piezoelectric 2D nanosheets can significantly contribute to biomedical solutions through chemical reactions, a potential often overlooked by researchers.
3.2 Products generated via piezocatalysis
Piezoelectric 2D nanosheets are revolutionizing green catalysis by converting mechanical energy, particularly in water's hydrogen and oxygen evolution processes.110,111 Hydrogen, notably promising in tissue regeneration,112 serves as a therapeutic agent in ischemic tissue injury recovery and metabolic syndrome management.113–115 It mitigates bone loss by reducing oxidative stress and TNFα-induced damage in osteoblasts116,117 and lessens steroid-associated femoral head necrosis in rabbits through hydrogen-rich saline.118 Furthermore, hydrogen's role in diminishing neuroinflammation, closely linked to oxidative stress, is notable.119,120 In early brain injury scenarios, hydrogen protects against oxidative stress-induced damage by influencing gene transcription and controlling the generation of oxidized phospholipids.
Molecular hydrogen's extensive medical applications necessitate designing carriers for its storage and controlled release. While hydrogen is generally non-toxic, its systemic administration can cause minor side effects like heartburn, diarrhea, and headaches.121 Nanoscale hydrogen delivery systems offer precise microenvironment regulation, proving effective in treating Alzheimer's disease,122 fatty liver disease,123 diabetic feet,124 and exercise-induced inflammation.125 Interestingly, carbon monoxide (CO) and reactive oxygen species (ROS), typically deemed toxic, are byproducts of piezocatalysis. Their targeted, controlled release can yield therapeutic effects in specific tissues while minimizing systemic toxicity, applicable in tumor treatment,126 anti-infective therapy, and cardiovascular and nervous system protection.127,128 The 2D structure, with its large surface-to-volume ratio, is particularly suited for hydrogen generation and anti-inflammatory therapy.129 Piezocatalytic nanoparticles enhance hydrogen generation rates and enable targeted release within the human body (Scheme 2).
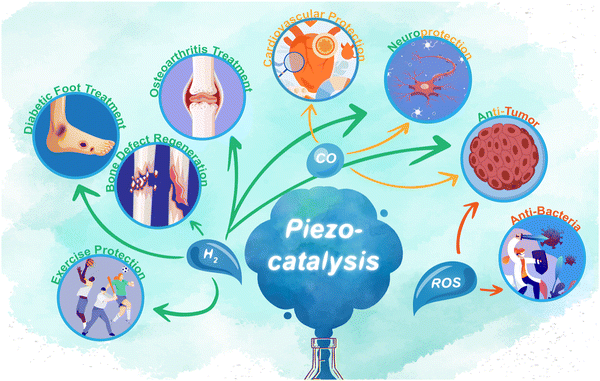 |
| Scheme 2 Schematic diagram of the applications of piezocatalysis products H2, CO, and ROS in the field of biomedical science. | |
3.3 Piezocatalysis-related neurodegenerative disease treatment
Piezocatalysis can be a potential treatment for Alzheimer's disease (AD). Research indicates that self-assembly, accumulation, and deposition of Aβ aggregates in the central nervous system are key AD pathologies.106,130 2D nanosheets are effective in absorbing amyloid peptides, impeding conformational transitions and fibrillation, thus decelerating disease progression.131 Park et al. developed an ultrasound-driven piezocatalytic platform using BiOCl 2D nanosheets, which successfully broke down Aβ aggregates into non-toxic fragments (Fig. 1(c)).106Ex vivo studies showed a significant reduction in Aβ plaque density in AD mouse brain slices from 121.6 cm−2 to 25.2 cm−2 using sono-activated BiOCl 2D nanosheets. These nanosheets are believed to disrupt Aβ aggregates’ strong side-chain interactions through piezocatalytic redox reactions.
3.4 Piezocatalysis-related antibacterial treatment
Bone defects infected by Staphylococcus aureus can lead to complications like osteomyelitis, impeding bone regeneration and causing secondary bone loss.132 Piezoelectric 2D MoS2 nanosheets have been shown to promote osteogenesis by reducing bone loss through infection and inflammation inhibition. These nanosheets are proven to induce bone integration and demonstrate good biocompatibility.133 The ROS generated via piezocatalysis can efficiently kill methicillin-resistant Staphylococcus aureus within 20 min under ultrasonic excitation, as illustrated in Fig. 1(d). The red blood cell membrane-coated MoS2 reduces inflammation and suppresses bone destruction, evidenced by a decrease in bone defect area after effective sonodynamic treatment. Consequently, piezoelectric MoS2 2D nanosheets are significant in treating bacterium-infected osteomyelitis, substantially reducing inflammation and bone loss.108,134
3.5 Piezocatalysis-related tumor therapy
Due to the acoustic excitation properties of piezoelectric catalysis, it facilitates remote stimulation for in situ controllable catalytic therapy within the body. Combined with the dimensional characteristics of piezoelectric two-dimensional nanosheets, localized catalytic treatment of tumors becomes feasible. A strategic piezoelectric-mediated drug delivery system is designed to downregulate tumor interstitial fluid pressure via water splitting of MoS2 in the tumor interstitium, which employs piezocatalysis to downregulate tumor interstitial fluid pressure and enhance intratumoral drug delivery. Piezocatalytic therapy has been demonstrated as a potential universal strategy for promoting intratumoral drug delivery, eliminating deep tumor cells, and inhibiting tumor recurrence by Gao et al. (Fig. 2(a)).135 In addition, piezocatalysis can be applied to activate tumor immunotherapy. He et al. have discussed a novel approach for treating deep tumors using ultrasound-driven piezocatalysis. The method utilizes a piezoelectric 2D nanosheet to produce hydrogen molecules and reduce lactic acid under ultrasonic stimulation. This process effectively inhibits liver tumor growth through immune regulation of the tumor microenvironment. Piezocatalytic hydrogen generation is highly responsive to ultrasound irradiation, allowing for controlled catalytic reactions and on-demand therapy (Fig. 2(b)).136
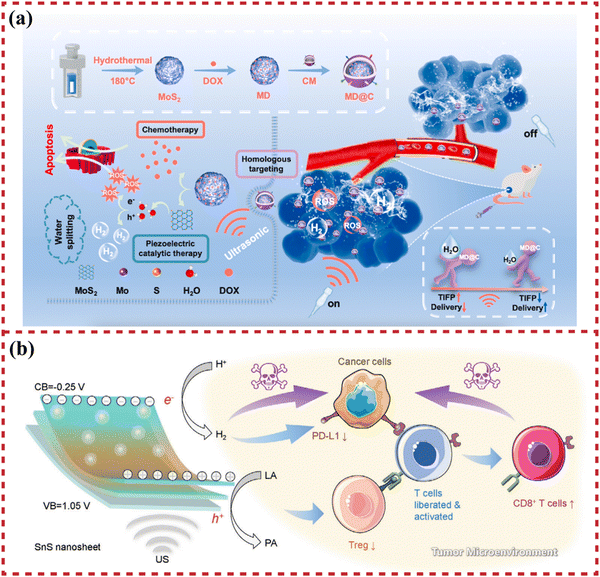 |
| Fig. 2 The applications of piezoelectric 2D nanosheets in piezocatalysis-related tumor therapy. (a) Schematic illustration of the construction procedure of the MoS2·DOX@cytomembrane (MD@C) and the mechanism of piezocatalytic enhancement of penetration ability in solid tumors. Reprinted with permission from ref. 135. Copyright 2022, Elsevier B.V. (b) Schematic illustration of the mechanism of SnS nanosheet-mediated piezocatalytic hydrogen generation and lactic acid deprivation for tumor immunoactivation. Reprinted with permission from ref. 136. Copyright 2023, Wiley-VCH Verlag GmbH & Co. KGaA, Weinheim. | |
4 Electrical signal-related biomedical uses of piezoelectric 2D nanosheets
Piezoelectric nanomaterials, including pyroelectric and ferroelectric materials,137 offer mechanical and electrical stimuli for tissue regeneration,10,43,138 capable of converting various energy types such as force, heat, electricity, and light into electrical, mechanical, and optical signals. These conversions can harness energy changes within tissue microenvironments. When integrated into scaffolds, piezoelectric nanomaterials can generate electrical signals to induce tissue regeneration, mimicking pathways in the extracellular matrix.139 Ultrathin 2D nanosheets, a type of piezoelectric nanomaterial, feature a high surface-to-volume ratio, significant anisotropy, and superior chemical and mechanical properties.140 Their unique morphology fosters special ‘nano-bio’ interactions, affecting biological activities like intracellular trafficking, biodistribution, and biodegradation. The ultrathin structure also offers advantageous transport and physicochemical properties for energy and catalytic applications.16 Additionally, these nanomaterials can function as targeted drugs, endocytosed into cells to create intracellular electrical fields. Current biomedical applications of piezoelectric 2D nanomaterials are detailed in Table 1, indicating a prospective trajectory for future biomedical strategies involving these materials.
Table 1 2D piezoelectric nanomaterials for biomedical uses
Nanostructured material |
Length (L)/diameter (D)/thickness (T) |
Synthesis method |
Piezoelectric strain coefficient |
Applied load/ultrasonic intensity |
Output voltage |
Output current |
Application |
Animal model |
Ref. |
ZnO nanosheets |
D ≈ 1–2 μm |
Hydrothermal method |
d
33 = 4–6 pC N−1 |
0.65 N |
0.5–50 mV |
— |
Osteogenic differentiation of cells |
— |
141
|
T ≈ 20 nm |
|
GaN/AlGaN heterostructures |
T ≈ 50 nm |
Metal–organic chemical vapour deposition |
— |
— |
0.311 mV |
— |
Promotion of bone regeneration |
Rat distal femur defect |
142
|
|
Hierarchical PVDF/ZIF-8 |
D ≈ 500 nm |
solid-state shear milling, salt leaching method |
d
33 = 3.5 pC N−1 |
— |
10 V |
— |
Vascularized bone regeneration |
Critical size calvarial defect model in rats and femoral defect model in mice |
143
|
|
MoS2 nanosheets |
D ≈ 20 nm |
Hydrothermal method |
— |
1.5 W cm−2, 50% duty cycle, 1 MHz |
— |
0.15 μA cm−2 |
Control of osteomyelitis and promotion of bone formation |
Rat osteomyelitis model |
109
|
|
BN nanosheets |
D ≈ 1.3 μm |
Electrospinning technique, oleylamine-assisted exfoliation method |
— |
Sound pressure of 80–110 dB at a fixed frequency of 1 kHz |
10–15 V |
— |
Promotion of bone regeneration |
— |
144
|
|
Black phosphorus nanosheets |
D = 300–500 nm |
Liquid exfoliating method |
— |
1.5 W cm−2, 50% duty cycle, 1 MHz |
— |
— |
Anti-infection and improved bone-implant integration |
Rat infection and bone-implant integration |
145
|
|
CaO2-loaded titanium carbide nanosheets |
T = 2–3 nm |
Facile exfoliation and intercalation method |
— |
1 W cm−2, 50% duty cycle, 1 MHz |
— |
— |
Anti-infection and promotion of bone regeneration |
Wound healing model and prosthetic joint infection model in rats |
146
|
|
Nb2C nanosheets |
D ≈ 2 μm |
Hydrothermal method |
— |
1.5 W cm−2, 50% duty cycle, 1 MHz |
— |
— |
Anti-infection and promotion of bone regeneration |
Rat infected bone defect model |
147
|
|
Ti3C2 nanosheets |
T = 50 nm |
Chemical exfoliation method |
— |
1.5 W cm−2, 50% duty cycle, 1 MHz |
— |
0.02 μA cm−2 |
Anti-infection |
Mouse bony tissue infection model |
148
|
|
WS2 nanosheets |
D ≈ 100 nm |
Hydrothermal method |
d
11 = 2.12–3.23 pC N−1 |
0.5 W cm−2, 2 min, 1 MHz |
— |
— |
Tumor treatment |
MCF-7 tumor-bearing mice |
149
|
T = 5.5 nm |
|
MoS2 nanosheets |
D ≈ 300 nm |
Lithium intercalation method |
— |
1 W/cm2, 50% duty cycle, 1 MHz |
0.02 V |
— |
Piezocatalysis of tumor treatment |
4T1 tumor-bearing mouse model |
150
|
|
Graphene oxide-TiO2–MnOx nanosheets |
D ≈ 230 nm |
Seeded growth method |
— |
1 W cm−2, 50% duty cycle, 60 sec, 1 MHz |
2.1 V |
— |
Piezocatalysis of tumor treatment |
4T1 tumor-bearing mouse model |
151
|
|
FePS3 nanosheets |
T = 4 nm |
Ultrasound-assisted liquid phase exfoliation method |
— |
0.5 W cm−2, 50% duty cycle, 1 MHz |
— |
— |
Chemodynamic/sonodynamic catalysis of tumor treatment |
4T1 tumor-bearing mouse model |
152
|
|
MoS2 nanoflowers |
D ≈ 100 nm |
Hydrothermal method |
— |
1.5 W cm−2, 50% duty cycle, 1 MHz |
— |
0.01 μA |
Piezocatalysis of water decomposition to reduce tumor pressure |
U14 tumor-bearing mouse model |
135
|
|
2D niobate-lead titanate piezo-array |
L ≈ 10 mm |
Dicing-and-filling method |
d
33 = 1200 pC N−1 |
3.3-MHz ultrasonic pulse |
— |
— |
Piezoelectricity-induced retinal stimulation |
— |
153
|
|
ZnO nanosheets |
D ≈ 87 nm |
Chemical bath deposition method |
— |
— |
— |
— |
Nerve agent detection |
— |
154
|
|
BiOCl nanosheets |
D = 0.8–2.5 μm |
Hydrothermal method |
— |
70 W, 40 kHz, 24 h |
— |
— |
Piezocatalysis of protein structure decomposition |
Alzheimer 's disease mouse model |
106
|
T = 200–470 nm |
|
SnS nanosheets |
L ≈ 150 nm |
Solvothermal method |
— |
2–3 W cm−2, 10% duty cycle, 10 min, 40 kHz |
— |
— |
Piezocatalysis for intratumoral immune activation |
Hepa 1–6-Luc cells tumor-bearing mouse model |
136
|
T = 0.8 nm |
4.1 Bone repair and regeneration
Piezoelectricity in natural bone.
It has been reported that piezoelectricity is essential in regulating natural bone metabolic activities, such as bone growth, structural remodelling and fracture healing. Halperin et al. found that the variation in d33 ranges from 7.7–8.7 pC N−1 for the human tibia.108 The asymmetric structure of collagen molecules leads to the piezoelectricity of natural bone.78 The collagen molecule is made from three polypeptide strands connected via hydrogen bonds and twisted to form a triple helix structure. It can generate piezoelectricity by changing the orientation of dipoles of –NH and –CO groups. Under mechanical stress, collagen fibres undergo various motions, such as rotation and sliding, leading to the generation of electrical charges. The broken hexagonal symmetry of their structure at the nanometric level is what causes the piezoelectric action. Additionally, ion motions in the mineralized matrix control the production of electrical potentials in the bone under mechanical stress.155,156
The piezoelectricity of collagen fibres, the key component of the bone tissue extracellular matrix, stimulates various cellular activities within the bone microenvironment. Compressive loads increase the negative charge on collagen fibres in the periosteum, thus facilitating osteoblast perception of electrical cues.157,158 The voltage-gated calcium channels on the cytomembrane open under the effect of electrical signals, resulting in a Ca2+ influx into the cytoplasm. The signaling pathways triggered by Ca2+ ultimately activate Ras and the extracellular signal-related protein kinase signaling pathway, which is critical for Runx2 activation and the induction of several growth factors, such as TGF-β and BMP.159
Osteogenic piezoelectric 2D nanosheets.
ZnO.
ZnO crystals, featuring three rapid growth directions, enable a range of morphologies from 0D to 3D.160 Their nanomaterials, synthesized through various crystal-growth techniques, exhibit notable semiconductivity, piezoelectricity, chemical stability, and biocompatibility.108,161 Bone tissue generates electrical stimulation in response to mechanical stress, a process reflecting the piezoelectric materials’ ability to harvest and redistribute energy, thus countering energy entropy increase. This mechanical energy is partially converted into electricity through piezoelectricity to regulate cellular activities. Esteve et al. demonstrated this by culturing osteoblast-like cells (Saos-2) on piezoelectric ZnO 2D nanosheets,141 as depicted in Fig. 3(b). The nanosheets’ reduced thickness (around 20 nm) and high aspect ratio enable deflection from inherent cell forces without external stimulation. These minute cell forces, in the nanonewton range, generate a piezoelectric potential of 0.5–50 mV, activating voltage-gated and stretch-activated calcium channels. Consequently, this leads to elevated expression of osteoblast differentiation genes.
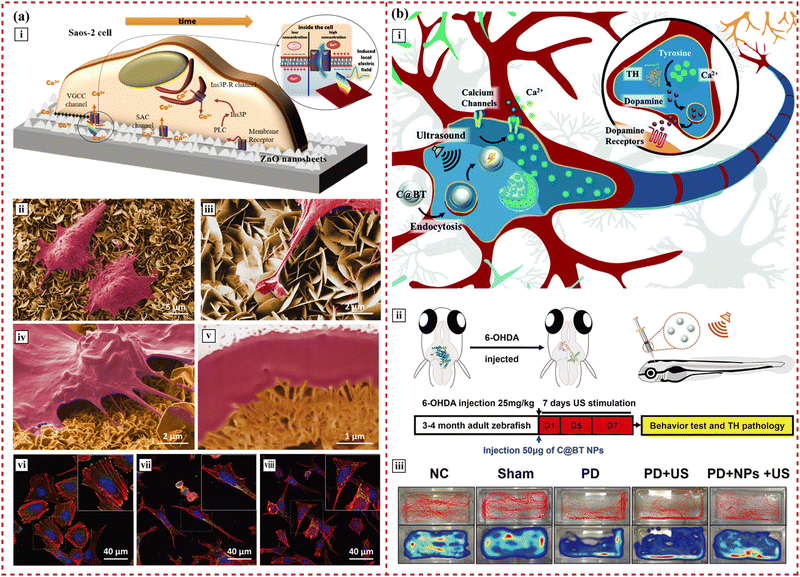 |
| Fig. 3 The applications of piezoelectric 2D nanosheets in bone and nerve tissue engineering. (a) Self-powered piezoelectric nanogenerators based on a ZnO 2D nanosheet network. (i) Sketch of a cell growing on the top array of ZnO 2D nanosheets, indicating the possible pathways involved in cytosolic Ca2+ concentration changes in the osteoblast-like cells. (ii)–(v) The nanogenerator–cell interaction that is assessed by scanning electron microscopy and focused ion beam shows the firm adhesion of osteoblast-like cells on the 2D nanosheets and the emitted long projections that anchored on the 2D nanosheets. (vi)–(viii) Confocal laser scanning microscopy analysis shows a high number of focal contacts at the end of long parallel bundles of actin filaments on cells grown on AlN, displaying a polygonal shape. Reprinted with permission from ref. 141. Copyright 2017, Wiley-VCH Verlag GmbH & Co. KGaA, Weinheim. (b) Piezoelectric nanoparticles modulate neural plasticity and recovery of degenerative dopaminergic neurons. (i) The piezoelectric BaTiO3 nanoparticle mediates neuron plasticity. (ii) Illustration of zebrafish injected with 6-OHDA to develop Parkinson's disease symptoms and schematic diagram of PD treatment via ultrasound-induced electromagnetic fields using nanoparticles. (iii) Representative spatiotemporal behavioral phenotyping in adult zebrafish under different treatments (control, sham, 6-OHDA, 6-OHDA + US, and 6-OHDA + nanoparticles + US). Reprinted with permission from ref. 194. Copyright 2020, Wiley-VCH Verlag GmbH & Co. KGaA, Weinheim. | |
Cell movement strains ZnO 2D nanosheets, producing local electric fields through piezoelectricity. These fields open voltage-gated calcium channels, enhancing cell proliferation and early differentiation. Furthermore, when used as drug nanocarriers, the electric signals from cell–nanosheet interactions can synergize with drug internalization.162 The applied electric field also influences cell alignment and migration, known as electrotaxis—the directed cell movement in response to electric cues.163 This property is advantageous for targeted drug delivery.
BN.
When considering piezoelectricity, BN nanotube hybrid piezoelectric scaffolds show enhanced proliferation and osteogenic differentiation of MC3T3-E1 cells and Saos-2 cells.164,165 Although the form of the nanotube is not quite the same as that of the 2D nanosheet, the piezoelectricity of BN can be further applied in bone tissue engineering.
MXene.
MXenes (Mn+1XnTx, n = 1–3), graphene-like transition metal carbides and nitrides, are established as highly oriented piezoelectric materials.166,167 Their surface functional groups disrupt inversion symmetry in lattice structures, endowing them with piezoelectric properties. When integrated into polymer-based scaffolds, these piezoelectric MXenes enhance the piezoelectric response.66
The role of MXenes (i.e., Ti3C2Tx) in bone tissue engineering has been explored168–170 and can be used as an auxiliary additive to functionalize bone regeneration scaffolds.10,170,171 MXene-loaded scaffolds show a positive effect on spontaneous osteogenic differentiation of MC3T3-E1 preosteoblasts by accelerating the absorption of Ca2+.170 The programmable functionalization of Ti3C2Tx MXenes endows them with stimuli-responsiveness, such as pH-responsiveness and chiral biorecognition of certain enzymes. This characteristic helps to recognize and activate MXene nanosheets in complex microenvironments, indicating their potential in targeted therapeutic nanomaterial fabrication and drug delivery systems.172,173 However, the applications of piezoelectric MXenes are limited in bone tissue engineering thus far, which seems to be a feasible idea for future material design.
4.2 Nerve repair and regeneration
Electrical signals in neuron growth.
Electric fields significantly influence neurite outgrowth and nerve tissue regeneration, paralleling osteogenesis effects.174,175 Jaffe and Poo initially demonstrated electric fields’ positive impact on neuritic growth.176 They observed increased neuritic outgrowth in embryonic chick dorsal root ganglia cultured on polylysine-coated glass under 70–140 mV mm−1 electric fields. This growth is hypothesized to occur as neurite growth cones react to nerve growth factor signaling, with its receptors rearranged by electric fields. Patel and Poo further found that steady electric fields (0.1–10 V cm−2) lead to pronounced neurite outgrowth towards the cathodal current and increased neuritic density, attributed to the redistribution of growth-controlling surface proteins like Concanavalin A receptors.177
In nerve tissue, intrinsic pulsatile electric fields create nonuniform and focal electric fields. To study neuritic outgrowth orientation in embryonic Xenopus, three types of extracellular electric fields are employed: focally applied pulsed fields, steady fields, and spatially uniform pulsed fields. While alternating currents typically induce osteogenesis, neural cell stimulation necessitates direct currents due to transmembrane potential dynamics.178 Application of a 250 mV mm−1 direct electric field attracts the growth cone to negative polarities and causes deflection towards positive polarities. Resultantly, Xenopus neurons develop longer neurites oriented towards the cathodal side and shorter neurites on the anodal side.
Electrical signal-related neurogenesis.
Electrical signals are crucial not only in neural development but also in nerve repair and regeneration post-injury.179 Injuries to the central or peripheral nervous system can impair cognitive and sensorimotor functions.180,181 Research indicates that low-frequency pulsed electrical stimulation facilitates nerve injury regeneration.182–184 Consequently, piezoelectric materials, which generate electrical signals under mechanical stress, can be engineered into devices for treating nerve injuries to aid nerve regeneration.
Capitalizing on the exceptional characteristics of 2D nanosheets, piezoelectric BN nanosheet-enhanced polycaprolactone channel scaffolds have been developed. BN inclusion elevates the piezoelectric and mechanical properties of these scaffolds. It mitigates the immune response and corrects energy metabolic disturbances by lowering ROS levels. Enhanced slow-type myosin expression in the BN@polycaprolactone group indicates BN nanosheets’ role in muscle structure recovery and amyotrophy reduction, likely due to microelectrical stimulation and injury area microenvironment rebalancing. This suggests concurrent regeneration of motor and sensory neurons.185
Piezoelectric nanomaterials in auditory nerve recovery mimic cochlear acoustic-electrical transduction by vibrating at distinct sound frequencies and conveying electrical impulses to the vestibulocochlear nerve.182 Additionally, electroconductive 2D nanomaterials excel in differentiating mesenchymal stem cells into neural-like cells, supporting their growth.186,187 A chip-like flexible biomimetic device, combining MXene artificial synapse with an ion-conductive elastomer, simulates the afferent nervous system.188 This device, using piezoelectric 2D MXene layers, not only transmits external stimuli but also emulates biological neural responses like ionic migration. It converts electronic variation in ion-conductive elastomers into neuro-electric signals, detecting joint flexion and radial artery pulse. This leads to neural tissue engineering and smart medical robot design.
Li et al. have demonstrated neural stem cells cultured on Ti3C2Tx MXene 2D nanosheets showed enhanced differentiation, neurite growth, and synaptic transmission.189 Ti3C2Tx increases voltage-gated Ca2+ currents in mature neurons, boosting spiking activity without altering passive membrane properties. Moreover, Ti3C2Tx MXene interfaces maintain basal neuronal physiology, evidenced by calcium imaging and synaptic current recordings.190
Electrical signal-related neurodegenerative disease treatment.
Neurodegenerative diseases, characterized by the progressive loss of neuronal function, prominently include Alzheimer's and Parkinson's diseases, noted for their severe complications and high prevalence.191,192 Electromagnetic field exposure has been found to influence cell epigenetics and facilitate efficient somatic cell reprogramming, offering a potential approach for recovering degenerative neurons.193
Carbon-shelled BaTiO3 nanoparticles, designed for endocytosis by neurons, generate intracellular electromagnetic fields under ultrasound, as depicted in Fig. 3(b).194 These electrical signals boost Ca2+ influx in nerve cells via voltage-dependent L-type Ca2+ channels. The resultant rise in intracellular Ca2+ concentration leads to phosphorylation of tyrosine hydroxylase and heightened hydroxylase activity.195 This piezoelectric effect modulates neural plasticity, aiding in the recovery of degenerative dopaminergic neurons in the midbrain.
5 Piezoelectric 2D nanosheets for future drug delivery applications
Piezoelectric 2D nanomaterials are emerging as effective agents in targeted regenerative drug delivery systems. They release drugs at specific sites in response to mechanical stimuli like ultrasound,106 friction,196 and vibration.197 These nanomaterials, with their adaptable surface properties, are increasingly used in intelligent drug delivery for biomedical applications.41,198 They offer more active sites and unique action mechanisms compared to other materials. These nanomaterials interact with protons,199,200 substrates,201 enzymes,202 and cell surface proteins and lipids, facilitating piezoelectric responsiveness and microcurrent stimulation. They can anchor nanodrugs to cellular membranes using antibodies and aptamers.203–206
Furthermore, piezoelectric nanomaterials play a significant role in controlling inflammation and maintaining immune microenvironment homeostasis at nano-bio interfaces.34 Piezoelectric 2D nanosheet drug release systems are being combined with polymers,207 biomolecules,208,209 and nanodots210 to create multifunctional materials. Various 2D materials exhibiting piezoelectricity show promise in drug delivery nanoplatforms and as therapeutic agents, including metal–organic frameworks,211 graphene,212 black phosphorus,209 BN,213 transition metal oxides,214 MXenes,198 TMDCs,215 and layered double hydroxides (LDH).216
5.1 Wearable and implantable devices
Electroactive materials can control drug release by electrically activating the release of adsorbed molecules. This method allows fine-tuning of release kinetics through the applied potential's magnitude, ensuring the drug delivery systems respond to physiologically safe mechanical forces without interference from daily human activities. Recently, Su et al. introduced a stretchable electronic facial mask (SEFM) to enhance the delivery of large-sized hyaluronic acid molecules for facial healthcare (Fig. 4(a)).55 Utilizing sonophoresis, which increases skin permeability for a wide range of molecular sizes without harming living cells, the SEFM has proven effective in animal/human experiments. It notably increased human facial skin moisture by 20%. These developments affirm the potential of piezoelectric-stimuli materials in localized controlled drug release.
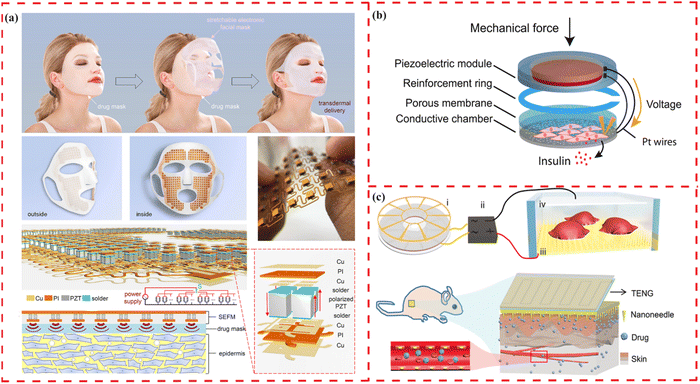 |
| Fig. 4 Illustrations of wearable and implantable devices for drug delivery using piezoelectric materials. (a) Schematic illustration of a stretchable electronic facial mask as a platform for facial healthcare enabling the promotion of the delivery effect. Reprinted with permission from ref. 55. Copyright 2022, American Chemical Society. (b) Schematic illustration of a push button-controlled rapid drug delivery system from a piezoelectrically activated subcutaneous cell implant. Reprinted with permission from ref. 217. Copyright 2022, American Association for the Advancement of Science. (c) Schematic illustration of the in vitro electroporation and the in vivo electroporation system of a TENG-driven electroporation system to enhance delivery efficiency with minimized impairment to cell viability. Reprinted with permission from ref. 218. Copyright 2019, Wiley-VCH Verlag GmbH & Co. KGaA, Weinheim. | |
Piezoelectricity-based controlled release systems can import not only large molecules but also small molecules. A self-sufficient, subcutaneous implant, controlled by the push-button and powered by finger pressure, has been developed, enabling the release of biopharmaceuticals without the need for complex electronics and external energy (Fig. 4(b)).217 This implant, featuring a piezoelectric membrane, facilitates the rapid and adjustable release of drugs from engineered cells, effectively normalizing blood sugar levels in a diabetic mouse model.
Furthermore, a wearable drug delivery device has been designed to achieve delicate contact on the nanoscale interfaces between materials and cells (Fig. 4(c)).218 With a sufficiently high local electric field in the limited area of the nanoneedle–cell interface, the device shows elevated delivery efficiency and minimal damage caused to cells. The integrated system achieves efficient delivery (up to 90%) of exogenous materials into different types of cells, with cell viability over 94%. These results demonstrate that the ’nano-bio’ interaction enables precise and safe drug release.
5.2 Targeted drug delivery systems
The rapid development of nanodrug delivery systems has initiated a revolution in tissue engineering.219,220 They can effectively stimulate cellular responses at the nanoscale with improved drug loading efficiency, targeted capability and uptake rate.35,221 Owing to both direct and indirect piezoelectric effects, mechanical and electrical energy can be effectively harnessed in the design of drug-release media.
Mechanical energy-mediated drug delivery and release.
A smart nanoeel has been designed according to the coupled concept of the piezoelectric effect and electromagnetism (Fig. 5(a)).32 Once alternating magnetic fields are applied, the magnetic Ni head module oscillates, subsequently causing the ferroelectric tail to flex and generating electric polarization changes via the piezoelectric effect. It is possible to precisely steer the nanoeels to the targeted location and trigger drug release by switching the magnetic field. Under magnetic fields of 15 mT and 11 Hz, the carriers remained in swimming mode, whereas doxorubicin release could not be observed. In contrast, in the drug release mode of 10 mT and 7 Hz, doxorubicin delivered into the cancer cells can be observed. Similarly, magneto-piezoelectric Janus particle-based micromachines have been fabricated to precisely control nanorobot motions under magnetic fields.31
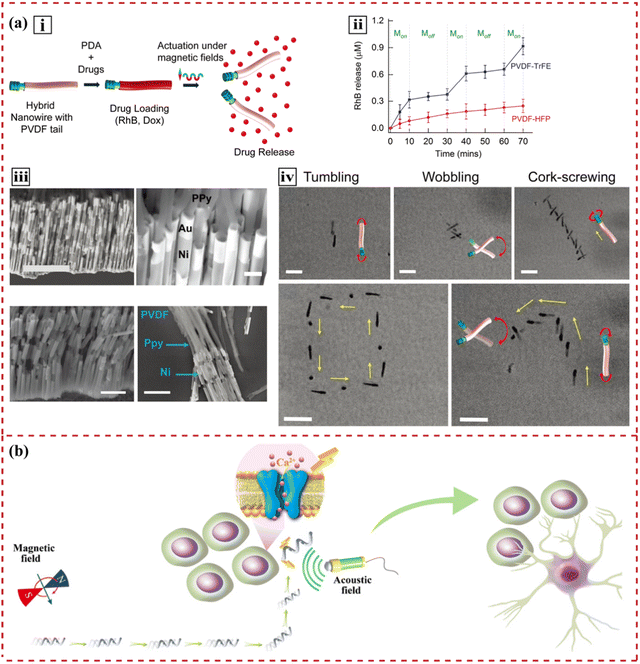 |
| Fig. 5 Illustration of targeted drug delivery systems using piezoelectric materials. (a) Images of a soft hybrid nanowire that mimics an electric eel to deliver drugs in a controlled manner. (i) Scheme depicting functionalization of hybrid nanoeels with polydopamine and drugs, followed by magnetically triggered drug release. (ii) Continuous rhodamine-B release at 10 mT and 7 Hz shows three times higher release for the piezoelectric P(VDF-TrFE) nanowires than for the nonpiezoelectric nanowires. (iii) Steps of fabricating nanowires, characterized by SEM. (iv) Characterization of the swimming behaviors of the hybrid nanowires. Reprinted with permission from ref. 32. Copyright 2019, Wiley-VCH Verlag GmbH & Co. KGaA, Weinheim. (b) Schematic illustration of a highly controllable micromotor to induce targeted neural stem-like cell differentiation. Reprinted with permission from ref. 30. Copyright 2020, Wiley-VCH Verlag GmbH & Co. KGaA, Weinheim. | |
Electrical energy-mediated drug release.
Unlike devices releasing drugs based on the indirect piezoelectric effect, the electrical signals generated via the direct piezoelectric effect can trigger drug release by charge repulsion. A targeted nanoscale platform has been fabricated to treat glioblastoma multiforme according to the responsive release of molecules under the piezoelectric effect.222 Functionalized P(VDF-TrFE) nanoparticles loaded with the small-molecule inhibitor nutlin-3a were designed for antitumour treatment in vivo. The nanoparticles can be remotely activated by ultrasound-based mechanical stimuli to induce drug release and deliver anticancer electric signals.
Flexible FeGa@P(VDF-TrFE) core–shell magnetoelectric nanowires have demonstrated that they can be actuated by different magnetic fields for targeted drug delivery.16 Upon magnetic stimulation, the magnetostrictive FeGa core deforms. The strain transferred onto the piezoelectric P(VDF-TrFE) shell induces surface polarization and releases the loaded paclitaxel. Similarly, the piezoelectric effect can be coupled with electromagnetism to generate loco-motion and migrate to the targeted cells,30 as shown in Fig. 5(b). Subject to the appropriate magnetic stimulation, magnetoelectric materials change their electric polarization, induce surface charge redistribution and trigger chemical processes.
5.3 Controlled molecule release
Growth factors, key elements in tissue engineering, stimulate cell differentiation, migration and proliferation.223,224 Bioactive ions, such as Zn2+,225 Mg2+,226 and Sr2+,227 have been identified to regulate cell metabolism and tissue regeneration either alone or in combination with growth factors. Due to the short half-life, growth factors and bioactive ions require targeted delivery and a precise release pattern.228
2D materials are attractive substrates for protein immobilization.41 The high protein adsorption capacity is supported by the large area-to-volume ratio of 2D materials.229 The atomic thickness provided mechanical flexibility for interactions between the material surface and adsorbed protein. Other surface characteristics, including functionality, crystallinity and curvature, can be altered to regulate protein adsorption and profoundly influence material–protein interactions.41,229
A piezoelectric polymer (poly(3-hydroxybutyrate)) loaded with silica microcapsules is investigated, demonstrating its capability to anchor bioactive molecules (such as bovine serum albumin) onto the scaffold surface.230 The change in surface charges, brought about by the matrix's piezoelectric properties, favors the adherence of more bioactive compounds. An investigation into the impact of ultrasound, enzymes, and laser radiation on initiating reactions reveals that ultrasound (20 kHz, 50 W for 120 s) rapidly alters the kinetic profile of the drug. According to piezoelectric Pb[Zr(x)Ti(1−x)]O3 and biocompatible polydimethylsiloxane (PDMS), Tseng et al. fabricated a flexible and stretchable electronic device for drug delivery.231 The advantages of piezoelectric 2D nanosheet flexibility and stretchability in drug release applications have been proven.
Piezoelectricity has been proven to boost interfacial cation transport in a controlled way. Zhang et al. have designed an integrative material built using a piezoelectric–dielectric flexible PVDF film that involves reduced graphene oxide 2D nanosheet fillers.232 The enhanced release may be attributed to the release of charged small molecules, which are produced by random and alternating positive/negative piezoelectric potentials under mechanical disturbances. They have also developed a similar enzyme release system that preserves enzyme activities.233 All the devices can be utilized in active drug release by responding to extensive mechanical disturbances of human movement.
Furthermore, piezocatalysis offers a viable approach for prodrug activation. The employment of piezoelectric 2D nanocarriers for prodrug loading enables not only efficient delivery to targeted sites but also increased concentration of prodrugs at these specific locations.234 Under the influence of ultrasound, the catalytic action facilitated by piezoelectric 2D nanomaterials converts inactive prodrugs into highly active pharmaceutical compounds, thus enabling targeted and precise drug therapy.235
6 Conclusions and perspectives
Since the demonstration of the piezoelectric properties of MoS2 2D nanosheets, 2D-structural piezoelectric nanomaterials have become popular in sensing, catalysis, energy and biomedicine areas. The strong targeting ability and minimized side effects are major advantages of these nanoscale drug delivery systems. In this review, the potential suitability of piezoelectric 2D nanosheets for drug delivery systems is discussed. When seeking solutions for more structurally demanding targets, such as bone defects and nerve injuries, the remote fields, for example, the acoustic fields, can provide biomedical modes in driving directional or local cell responses within in vivo tissue culture. On the one hand, the piezoelectric 2D nanosheet inside the human body can act as an energy converter to generate electrical signals without an open wound when applying an acoustic field. These essential electrical cues can trigger osteogenic-/neurogenic-related cell pathways or alter the local microenvironment to switch on the regenerative mode. Conversely, in the absence of remote fields, 2D nanosheets are capable of harvesting energy from cellular activity and modulating cell behaviors. This process inherently represents a method of inverse entropy increase in energy dissipation.
Moreover, piezoelectric 2D nanosheets can interact with the fundamental components of tissue regeneration: cells, materials, and biochemical factors. For cells, ultrathin electrical 2D nanosheets can build a unique nano-bio interface for cell activation. Regarding materials, 2D nanosheets can either be considered as standalone entities or employed as enhancements for scaffold materials. By connecting the scaffold material with the seed cells, the scaffolds can be functionalized for precise regulation. For biochemical factors (i.e., ions and proteins), 2D nanosheets can be transformed into drug delivery systems. The vast canvas – the large surface of the 2D structure – provides active sites for molecular binding and is beneficial for drug loading, tissue targeting and stimulus response. The controllable surface polarization brings programmable electrical signals, guaranteeing a release pattern of bioactive molecules.
For tumor treatment, piezocatalysis of 2D nanosheets can provide a synergistic effect with therapeutic drugs. Piezocatalysis causes ROS generation and glutathione depletion in tumor cells, leading to a sharp decrease in the activities of tumor cells. Stimuli-responsive released chemotherapeutic or immunotherapeutic drugs can obtain excellent antitumour effects. In addition, piezoelectric-related water splitting can remodel the tumor microenvironment by reducing tumor interstitial pressure, thereby achieving deep penetration of drugs. The treatment of degenerative diseases can be conducted in the same way. Given that piezoelectric 2D nanosheets can harvest energy from the surrounding subtle mechanical cues, drug delivery systems with high piezoelectric coefficients can even have a sustainable treatment effect after removing the external stimulus, realizing energy recycling.
Therefore, considering the increasing mechanisms and applications of piezoelectric 2D nanosheets being revealed, this review aims to build bridges between diverse academic disciplines. Looking towards the future of drug delivery, the design of drug delivery systems should ideally adopt a top-down approach, emphasizing energy-efficient fabrication and the attainment of effective functionalities. Using piezoelectric 2D nanosheets as an example, it is possible to discover multiple functions of a single material from various perspectives across different fields. This may simplify the complicated design and fabrication of drug delivery systems and optimize the applications of nanoscale drug delivery systems.
Conflicts of interest
There are no conflicts to declare.
Acknowledgements
The authors are grateful for the support and funding from the National Natural Science Foundation of China [no. 82130027] and the Innovative Research Group Project of the National Natural Science Foundation of China [no. 81921002].
References
- M. S. Alqahtani, M. Kazi, M. A. Alsenaidy and M. Z. Ahmad, Front. Pharmacol., 2021, 12, 618411 CrossRef CAS PubMed.
- H. Marwah, T. Garg, A. K. Goyal and G. Rath, Drug Delivery, 2016, 23, 564–578 CrossRef CAS PubMed.
- M. Ying-Yan, L. Meng, W. Jin-Da, W. Kai-Jie, Z. Jing-Shang, C. Shu-Ying, L. Xu, L. Qing-Feng, G. Fei and W. Xiu-Hua, J. Controlled Release, 2021, 339, 391–402 CrossRef.
- K. Albinali, M. Zagho, Y. Deng and A. Elzatahry, Int. J. Nanomed., 2019, 14, 1707–1723 CrossRef CAS PubMed.
- R. Avila, C. Li, Y. Xue, J. A. Rogers and Y. Huang, Proc. Natl. Acad. Sci. U. S. A., 2021, 118, e2026405118 CrossRef CAS PubMed.
- K. Kapat, Q. T. H. Shubhra, M. Zhou and S. Leeuwenburgh, Adv. Funct. Mater., 2020, 30, 1909045 CrossRef CAS.
- L. Edgar, T. Pu, B. Porter, J. M. Aziz, C. La Pointe, A. Asthana and G. Orlando, Br. J. Surg., 2020, 107, 793–800 CrossRef CAS.
- F. Berthiaume, T. J. Maguire and M. L. Yarmush, Annu. Rev. Chem. Biomol. Eng., 2011, 2, 403–430 CrossRef.
- S. Yao, M. Zheng, Z. Wang, Y. Zhao, S. Wang, Z. Liu, Z. Li, Y. Guan, Z. L. Wang and L. Li, Adv. Mater., 2022, 2205881 CrossRef CAS.
- P. Li, Y. Bräuniger, J. Kunigkeit, M. R. O. Vega, E. Zhang, J. Grothe and S. Kaskel, Angew. Chem., Int. Ed., 2022, 61, 10 Search PubMed.
- M. Hasan, A. Khatun, T. Fukuta and K. Kogure, Adv. Drug Delivery Rev., 2020, 154–155, 227–235 CrossRef CAS PubMed.
- M. Toyoda, S. Hama, Y. Ikeda, Y. Nagasaki and K. Kogure, Int. J. Pharm., 2015, 483, 110–114 CrossRef CAS PubMed.
- W.-C. Huang, C.-C. Lin, T.-W. Chiu and S.-Y. Chen, ACS Appl. Mater. Interfaces, 2022, 14, 46188–46200 CrossRef CAS PubMed.
- M. Mishra, G. Sen Gupta and X. Gui, Sensors, 2022, 22, 7685 CrossRef PubMed.
- J. M. Marmolejo-Tejada, J. De La Roche-Yepes, C. A. Pérez-López, J. A. P. Taborda, A. Ávila and A. Jaramillo-Botero, J. Chem. Inf. Model., 2021, 61, 4537–4543 CrossRef CAS PubMed.
- P. Lin, C. Pan and Z. L. Wang, Mater. Today Nano, 2018, 4, 17–31 CrossRef.
- J. Tao, Y. Chen, A. Bhardwaj, L. Wen, J. Li, O. V. Kolosov, Y. Lin, Z. Hong, Z. Huang and S. Mathur, Proc. Natl. Acad. Sci. U. S. A., 2022, 119, e2211059119 CrossRef CAS PubMed.
- M. T. Chorsi, E. J. Curry, H. T. Chorsi, R. Das, J. Baroody, P. K. Purohit, H. Ilies and T. D. Nguyen, Adv. Mater., 2019, 31, 1802084 CrossRef PubMed.
- M. Lee, J. R. Renshof, K. J. van Zeggeren, M. J. A. Houmes, E. Lesne, M. Šiškins, T. C. van Thiel, R. H. Guis, M. R. van Blankenstein, G. J. Verbiest, A. D. Caviglia, H. S. J. van der Zant and P. G. Steeneken, Adv. Mater., 2022, 2204630 CrossRef CAS PubMed.
- X. Li, Y. Li, Y. Li, J. Tan, J. Zhang, H. Zhang, J. Liang, T. Li, Y. Liu, H. Jiang and P. Li, ACS Appl. Mater. Interfaces, 2022, 14, 46789–46800 CrossRef CAS PubMed.
- Y. Dobashi, D. Yao, Y. Petel, T. N. Nguyen, M. S. Sarwar, Y. Thabet, C. L. W. Ng, E. Scabeni Glitz, G. T. M. Nguyen, C. Plesse, F. Vidal, C. A. Michal and J. D. W. Madden, Science, 2022, 376, 502–507 CrossRef CAS PubMed.
- Y. Zhang, H. Zhang, L. Chen, J. Wang, J. Wang, J. Li, Y. Zhao, M. Zhang and H. Zhang, Environ. Sci. Technol., 2022, 56, 16271–16280 CrossRef CAS PubMed.
- K. Wang, C. Han, J. Li, J. Qiu, J. Sunarso and S. Liu, Angew. Chem., Int. Ed., 2022, 61, e202110429 CrossRef CAS PubMed.
- J. M. Wu, W. E. Chang, Y. T. Chang and C. Chang, Adv. Mater., 2016, 28, 3718–3725 CrossRef CAS PubMed.
- A. Cafarelli, A. Marino, L. Vannozzi, J. Puigmartí-Luis, S. Pané, G. Ciofani and L. Ricotti, ACS Nano, 2021, 15, 11066–11086 CrossRef CAS PubMed.
- R. Sun, M. Liu, J. Lu, B. Chu, Y. Yang, B. Song, H. Wang and Y. He, Nat. Commun., 2022, 13, 5127 CrossRef CAS PubMed.
- L. Wang, Y. Shi, J. Jiang, C. Li, H. Zhang, X. Zhang, T. Jiang, L. Wang, Y. Wang and L. Feng, Small, 2022, 2203678 CrossRef CAS PubMed.
- M. Yu, W. Yang, W. Yue and Y. Chen, Adv. Sci., 2022, 2204335 CrossRef CAS PubMed.
- A. Li, W. Gao, X. Zhang, Y. Deng, Y. Zhu, H. Gu, J. Wen and X. Jiang, Biomater. Adv., 2022, 138, 212935 CrossRef CAS PubMed.
- L. Liu, B. Chen, K. Liu, J. Gao, Y. Ye, Z. Wang, N. Qin, D. A. Wilson, Y. Tu and F. Peng, Adv. Funct. Mater., 2020, 30, 1910108 CrossRef CAS.
- X.-Z. Chen, N. Shamsudhin, M. Hoop, R. Pieters, E. Siringil, M. S. Sakar, B. J. Nelson and S. Pané, Mater. Horiz., 2016, 3, 113–118 RSC.
- F. Mushtaq, H. Torlakcik, M. Hoop, B. Jang, F. Carlson, T. Grunow, N. Läubli, A. Ferreira, X. Chen, B. J. Nelson and S. Pané, Adv. Funct. Mater., 2019, 29, 1808135 CrossRef.
- C. Li, Z. Xiong, L. Zhou, W. Huang, Y. He, L. Li, H. Shi, J. Lu, J. Wang, D. Li and S. Yin, Adv. Healthcare Mater., 2022, 11, 2201471 CrossRef CAS PubMed.
- G. Peng and B. Fadeel, Adv. Drug Delivery Rev., 2022, 188, 114422 CrossRef CAS PubMed.
- Z. Li, X. Zhu, J. Li, J. Zhong, J. Zhang and J. Fan, Nanoscale, 2022, 14, 5384–5391 RSC.
- Y. Meng, H. Zhong, Z. Xu, T. He, J. S. Kim, S. Han, S. Kim, S. Park, Y. Shen, M. Gong, Q. Xiao and S.-H. Bae, Nanoscale Horiz., 2023, 8, 1345–1365 RSC.
- X. Yang, C. Shang, S. Zhou and J. Zhao, Nanoscale Horiz., 2020, 5, 1106–1115 RSC.
- L. Qi, S. Ruan and Y. Zeng, Adv. Mater., 2021, 33, 2005098 CrossRef CAS PubMed.
- A. Corletto, A. V. Ellis, N. A. Shepelin, M. Fronzi, D. A. Winkler, J. G. Shapter and P. C. Sherrell, Adv. Mater., 2022, 34, 2203849 CrossRef CAS PubMed.
- A. B. Puthirath, X. Zhang, A. Krishnamoorthy, R. Xu, F. S. Samghabadi, D. C. Moore, J. Lai, T. Zhang, D. E. Sanchez, F. Zhang, N. R. Glavin, D. Litvinov, R. Vajtai, V. Swaminathan, M. Terrones, H. Zhu, P. Vashishta and P. M. Ajayan, Adv. Mater., 2022, 34, 2206425 CrossRef CAS PubMed.
- S. H. Chen, D. R. Bell and B. Luan, Adv. Drug Delivery Rev., 2022, 186, 114336 CrossRef CAS PubMed.
- X. He, Y. Zhu, B. Ma, X. Xu, R. Huang, L. Cheng and R. Zhu, Adv. Drug Delivery Rev., 2022, 187, 114379 CrossRef CAS PubMed.
- M. Zhao, X. Gao, J. Wei, C. Tu, H. Zheng, K. Jing, J. Chu, W. Ye and T. Groth, Front. Bioeng. Biotechnol., 2022, 10, 991855 CrossRef PubMed.
- B. Das, S. Maity, S. Paul, K. Dolui, S. Paramanik, S. Naskar, S. R. Mohanty, S. Chakraborty, A. Ghosh, M. Palit, K. Watanabe, T. Taniguchi, K. S. R. Menon and S. Datta, ACS Nano, 2021, 15, 20203–20213 CrossRef CAS PubMed.
- Y. Wu, S. Dong, N. Lv, Z. Xu, R. Ren, G. Zhu, B. Huang, Y. Zhang and X. Dong, Small, 2022, 2204888 CrossRef CAS PubMed.
- S. Xiao, Y. Zheng, X. Wu, M. Zhou, X. Rong, L. Wang, Y. Tang, X. Liu, L. Qiu and C. Cheng, Small, 2022, 18, 2203281 CrossRef CAS PubMed.
- M. Li, Y. Gao, X. Fan, Y. Wei, Q. Hao and T. Qiu, Nanoscale Horiz., 2021, 6, 186–191 RSC.
- C. Dai, R. Hu, C. Wang, Z. Liu, S. Zhang, L. Yu, Y. Chen and B. Zhang, Nanoscale Horiz., 2020, 5, 857–868 RSC.
- S. Li, Z. Zhao, J. Li, H. Liu, M. Liu, Y. Zhang, L. Su, A. I. Pérez-Jiménez, Y. Guo, F. Yang, Y. Liu, J. Zhao, J. Zhang, L. Zhao and Y. Lin, Small, 2022, 18, 2202507 CrossRef CAS PubMed.
- Z. Ye, Y. Fan, T. Zhu, D. Cao, X. Hu, S. Xiang, J. Li, Z. Guo, X. Chen, K. Tan and N. Zheng, ACS Appl. Mater. Interfaces, 2022, 14, 23194–23205 CrossRef CAS PubMed.
- Y. Kang, Z. Mao, Y. Wang, C. Pan, M. Ou, H. Zhang, W. Zeng and X. Ji, Nat. Commun., 2022, 13, 2425 CrossRef CAS PubMed.
- P. Ma, X. Lai, Z. Luo, Y. Chen, X. J. Loh, E. Ye, Z. Li, C. Wu and Y.-L. Wu, Nanoscale Adv., 2022, 4, 3462–3478 RSC.
- B. T. Mai, J. S. Conteh, H. Gavilán, A. Di Girolamo and T. Pellegrino, ACS Appl. Mater. Interfaces, 2022, 14, 48476–48488 CrossRef CAS PubMed.
- J. P. K. Armstrong and M. M. Stevens, Trends Biotechnol., 2020, 38, 254–263 CrossRef CAS PubMed.
- S. Li, J. Xu, R. Li, Y. Wang, M. Zhang, J. Li, S. Yin, G. Liu, L. Zhang, B. Li, Q. Gu and Y. Su, ACS Nano, 2022, 16, 5961–5974 CrossRef CAS PubMed.
- X. Li, B. C. Heng, Y. Bai, Q. Wang, M. Gao, Y. He, X. Zhang, X. Deng and X. Zhang, Bioact. Mater., 2023, 20, 81–92 CAS.
- X. Dai, B. C. Heng, Y. Bai, F. You, X. Sun, Y. Li, Z. Tang, M. Xu, X. Zhang and X. Deng, Bioact. Mater., 2021, 6, 2029–2038 CAS.
- M. B. Ghasemian, T. Daeneke, Z. Shahrbabaki, J. Yang and K. Kalantar-Zadeh, Nanoscale, 2020, 12, 2875–2901 RSC.
- M.-M. Yang, Z.-D. Luo, Z. Mi, J. Zhao, S. P. E and M. Alexe, Nature, 2020, 584, 377–381 CrossRef CAS PubMed.
- S. Bairagi and S. W. Ali, Energy, 2020, 198, 117385 CrossRef CAS.
- X. Zuo, K. Chang, J. Zhao, Z. Xie, H. Tang, B. Li and Z. Chang, J. Mater. Chem. A, 2016, 4, 51–58 RSC.
- L. Liang, C. Sun, R. Zhang, S. Han, J. Wang, N. Ren and H. Liu, Nano Energy, 2021, 90, 106634 CrossRef CAS.
- J. Wang, D. Adami, B. Lu, C. Liu, A. Maazouz and K. Lamnawar, Polymers, 2020, 12, 2596 CrossRef CAS PubMed.
- Y. Liu, W.-G. Liu, D.-B. Lin, X.-L. Niu, S. Zhou, J. Zhang, S.-B. Ge, Y.-C. Zhu, X. Meng and Z.-L. Chen, Nanomaterials, 2022, 12, 588 CrossRef CAS PubMed.
- Z.-X. Wang and W.-Q. Liao, Science, 2022, 375, 1353–1354 CrossRef CAS PubMed.
- Y. Su, W. Li, X. Cheng, Y. Zhou, S. Yang, X. Zhang, C. Chen, T. Yang, H. Pan, G. Xie, G. Chen, X. Zhao, X. Xiao, B. Li, H. Tai, Y. Jiang, L.-Q. Chen, F. Li and J. Chen, Nat. Commun., 2022, 13, 4867 CrossRef CAS PubMed.
- W. Wu, L. Wang, Y. Li, F. Zhang, L. Lin, S. Niu, D. Chenet, X. Zhang, Y. Hao, T. F. Heinz, J. Hone and Z. L. Wang, Nature, 2014, 514, 470–474 CrossRef CAS PubMed.
- L. Rogée, L. Wang, Y. Zhang, S. Cai, P. Wang, M. Chhowalla, W. Ji and S. P. Lau, Science, 2022, 376, 973–978 CrossRef PubMed.
- V. J. González, A. M. Rodríguez, I. Payo and E. Vázquez, Nanoscale Horiz., 2020, 5, 331–335 RSC.
- S. Rahman and Y. Lu, Nanoscale Horiz., 2022, 7, 849–872 RSC.
- G. Cheon, K.-A. N. Duerloo, A. D. Sendek, C. Porter, Y. Chen and E. J. Reed, Nano Lett., 2017, 17, 1915–1923 CrossRef CAS PubMed.
- M. Zelisko, Y. Hanlumyuang, S. Yang, Y. Liu, C. Lei, J. Li, P. M. Ajayan and P. Sharma, Nat. Commun., 2014, 5, 4284 CrossRef CAS PubMed.
- D. Kim, S. A. Han, J. H. Kim, J. Lee, S. Kim and S. Lee, Adv. Mater., 2020, 32, 1906989 CrossRef CAS PubMed.
- S. Guerin, A. Stapleton, D. Chovan, R. Mouras, M. Gleeson, C. McKeown, M. R. Noor, C. Silien, F. M. F. Rhen, A. L. Kholkin, N. Liu, T. Soulimane, S. A. M. Tofail and D. Thompson, Nat. Mater., 2018, 17, 180–186 CrossRef CAS PubMed.
- P. Zelenovskiy, D. Vasileva, A. Nuraeva, S. Vasilev, T. Khazamov, E. Dikushina, V. Ya Shur and A. L. Kholkin, Ferroelectrics, 2016, 496, 10–19 CrossRef CAS.
- R. M. F. Baptista, E. de Matos Gomes, M. M. M. Raposo, S. P. G. Costa, P. E. Lopes, B. Almeida and M. S. Belsley, Nanoscale Adv., 2019, 1, 4339–4346 RSC.
- T. Nakiri, K. Imoto, M. Ishizuka, S. Okamoto, M. Date, Y. Uematsu, E. Fukada and Y. Tajitsu, Jpn. J. Appl. Phys., 2004, 43, 6769 CrossRef CAS.
- D. Denning, M. V. Paukshto, S. Habelitz and B. J. Rodriguez, J. Biomed. Mater. Res., 2014, 102, 284–292 CrossRef CAS PubMed.
- Z. Zhang, S. Liu, Q. Pan, Y. Hong, Y. Shan, Z. Peng, X. Xu, B. Liu, Y. Chai and Z. Yang, Adv. Mater., 2022, 34, 2200864 CrossRef CAS PubMed.
- V. Vivekananthan, N. R. Alluri, Y. Purusothaman, A. Chandrasekhar, S. Selvarajan and S.-J. Kim, ACS Appl. Mater. Interfaces, 2018, 10, 18650–18656 CrossRef CAS PubMed.
- J. Ohnuki, T. Sato and M. Takano, Phys. Rev. E, 2016, 94, 012406 CrossRef PubMed.
-
B. D. Ratner and F. J. Schoen, Biomaterials Science, Elsevier, 2020, pp. 843–849 Search PubMed.
- N. Wiesmann, W. Tremel and J. Brieger, J. Mater. Chem. B, 2020, 8, 4973–4989 RSC.
- J. Sun, H. Guo, J. Ribera, C. Wu, K. Tu, M. Binelli, G. Panzarasa, F. W. M. R. Schwarze, Z. L. Wang and I. Burgert, ACS Nano, 2020, 14, 14665–14674 CrossRef CAS PubMed.
- A. Sood, M. Desseigne, A. Dev, L. Maurizi, A. Kumar, N. Millot and S. S. Han, Small, 2023, 19, 2206401 CrossRef CAS PubMed.
- T. Webster, P. Maschhoff and B. Geilich, Int. J. Nanomed., 2013, 257 CrossRef PubMed.
- T. A. Hilder and N. Gaston, ChemPhysChem, 2016, 17, 1573–1578 CrossRef CAS PubMed.
- S. Mateti, C. S. Wong, Z. Liu, W. Yang, Y. Li, L. H. Li and Y. Chen, Nano Res., 2018, 11, 334–342 CrossRef CAS.
- T. Liu, C. Wang, W. Cui, H. Gong, C. Liang, X. Shi, Z. Li, B. Sun and Z. Liu, Nanoscale, 2014, 6, 11219–11225 RSC.
- L. Cheng, J. Liu, X. Gu, H. Gong, X. Shi, T. Liu, C. Wang, X. Wang, G. Liu, H. Xing, W. Bu, B. Sun and Z. Liu, Adv. Mater., 2014, 26, 1886–1893 CrossRef CAS PubMed.
- X. Qian, S. Shen, T. Liu, L. Cheng and Z. Liu, Nanoscale, 2015, 7, 6380–6387 RSC.
- S. Zhu, L. Gong, J. Xie, Z. Gu and Y. Zhao, Small Methods, 2017, 1, 1700220 CrossRef.
- G. Liu, X. Yan, C. Li, S. Hu, J. Yan and B. Yan, J. Hazard. Mater., 2023, 443, 130303 CrossRef CAS PubMed.
- H. J. Dyson and P. E. Wright, FASEB J., 1995, 9, 37–42 CrossRef CAS PubMed.
- D. W. Kulp and W. R. Schief, Curr. Opin. Virol., 2013, 3, 322–331 CrossRef CAS PubMed.
- J. Li, Y. Long, F. Yang and X. Wang, Curr. Opin. Solid State Mater. Sci., 2020, 24, 100806 CrossRef CAS PubMed.
- W. Wang, Z. Wu, Z. Dai, Y. Yang, J. Wang and G. Wu, Amino Acids, 2013, 45, 463–477 CrossRef CAS PubMed.
- K. A. Alberti and Q. Xu, Regen Biomater., 2016, 3, 1–11 CrossRef CAS PubMed.
- I. C. Amaechi, A. Hadj Youssef, A. Dörfler, Y. González, R. Katoch and A. Ruediger, Angew. Chem., Int. Ed., 2022, 61, e202207975 CrossRef CAS PubMed.
- X. Wang, J. Wu, Y. Zhang, Y. Sun, K. Ma, Y. Xie, W. Zheng, Z. Tian, Z. Kang and Y. Zhang, Adv. Mater., 2022, 2206576 Search PubMed.
- A. Ranjan, K.-Y. Hsiao, C.-Y. Lin, Y.-H. Tseng and M.-Y. Lu, ACS Appl. Mater. Interfaces, 2022, 14, 35635–35644 CrossRef CAS PubMed.
- H. Xiao, J. He, X. Lu, F. Wang and Y. Guo, Chemosphere, 2022, 306, 135543 CrossRef CAS PubMed.
- P. C. Sherrell, M. Fronzi, N. A. Shepelin, A. Corletto, D. A. Winkler, M. Ford, J. G. Shapter and A. V. Ellis, Chem. Soc. Rev., 2022, 51, 650–671 RSC.
- M. B. Ghasemian, T. Daeneke, Z. Shahrbabaki, J. Yang and K. Kalantar-Zadeh, Nanoscale, 2020, 12, 2875–2901 RSC.
- T. Zhao, S. Zhang, Y. Guo and Q. Wang, Nanoscale, 2016, 8, 233–242 RSC.
- J. Jang, K. Kim, J. Yoon and C. B. Park, Biomaterials, 2020, 255, 120165 CrossRef CAS PubMed.
- Z. Li, T. Zhang, F. Fan, F. Gao, H. Ji and L. Yang, J. Phys. Chem. Lett., 2020, 11, 1228–1238 CrossRef CAS PubMed.
- K. Su, L. Tan, X. Liu, Z. Cui, Y. Zheng, B. Li, Y. Han, Z. Li, S. Zhu, Y. Liang, X. Feng, X. Wang and S. Wu, ACS Nano, 2020, 14, 2077–2089 CrossRef CAS PubMed.
- X. Feng, L. Ma, J. Lei, Q. Ouyang, Y. Zeng, Y. Luo, X. Zhang, Y. Song, G. Li, L. Tan, X. Liu and C. Yang, ACS Nano, 2022, 16, 2546–2557 CrossRef CAS PubMed.
- M. Xu, M. Lu, G. Qin, X. Wu, T. Yu, L. Zhang, K. Li, X. Cheng and Y. Lan, Angew. Chem., Int. Ed., 2022, 61, e202210700 CrossRef CAS PubMed.
- Y. He, Z. Xu, Y. He, G. Cao, S. Ni, Y. Tang, J. Wang, Y. Yuan, Z. Ma, D. Wang and D. Gao, Biomaterials, 2022, 290, 121816 CrossRef CAS PubMed.
- M. Yoshida, JACC, 2022, 7, 162–163 Search PubMed.
- I. Ohsawa, M. Ishikawa, K. Takahashi, M. Watanabe, K. Nishimaki, K. Yamagata, K. Katsura, Y. Katayama, S. Asoh and S. Ohta, Nat. Med., 2007, 13, 688–694 CrossRef CAS PubMed.
- S. Takeuchi, K. Nagatani, N. Otani, H. Nawashiro, T. Sugawara, K. Wada and K. Mori, BMC Neurosci., 2015, 16, 22 CrossRef PubMed.
- N. Kamimura, K. Nishimaki, I. Ohsawa and S. Ohta, Obesity, 2011, 19, 1396–1403 CrossRef CAS PubMed.
- W.-W. Cai, M.-H. Zhang, Y.-S. Yu and J.-H. Cai, Mol. Cell. Biochem., 2013, 373, 1–9 CrossRef CAS PubMed.
- Y. Sun, F. Shuang, D. M. Chen and R. B. Zhou, Osteoporosis Int., 2013, 24, 969–978 CrossRef CAS PubMed.
- S.-L. Huang, J. Jiao and H.-W. Yan, Exp. Ther. Med., 2016, 11, 177–182 CrossRef CAS PubMed.
- M. Iketani and I. Ohsawa, Curr. Neuropharmacol., 2017, 15, 324–331 CrossRef CAS PubMed.
- R. Pluta, S. Januszewski and S. J. Czuczwar, Int. J. Mater. Sci., 2022, 23, 6591 CAS.
- K. Nagatani, H. Nawashiro, S. Takeuchi, S. Tomura, N. Otani, H. Osada, K. Wada, H. Katoh, N. Tsuzuki and K. Mori, Med. Gas Res., 2013, 3, 13 CrossRef CAS PubMed.
- L. Zhang, P. Zhao, C. Yue, Z. Jin, Q. Liu, X. Du and Q. He, Biomaterials, 2019, 197, 393–404 CrossRef CAS PubMed.
- Z. Jin, Y. Sun, T. Yang, L. Tan, P. Lv, Q. Xu, G. Tao, S. Qin, X. Lu and Q. He, Biomaterials, 2021, 276, 121030 CrossRef CAS PubMed.
- S. Chen, Y. Zhu, Q. Xu, Q. Jiang, D. Chen, T. Chen, X. Xu, Z. Jin and Q. He, Nat. Commun., 2022, 13, 5684 CrossRef CAS PubMed.
- T. W. LeBaron, I. Laher, B. Kura and J. Slezak, Can. J. Physiol. Pharmacol., 2019, 97, 797–807 CrossRef CAS PubMed.
- P. Agostinis, K. Berg, K. A. Cengel, T. H. Foster, A. W. Girotti, S. O. Gollnick, S. M. Hahn, M. R. Hamblin, A. Juzeniene, D. Kessel, M. Korbelik, J. Moan, P. Mroz, D. Nowis, J. Piette, B. C. Wilson and J. Golab, Ca-Cancer J. Clin., 2011, 61, 250–281 CrossRef PubMed.
- H.-H. Kim and S. Choi, Int. J. Mater. Sci., 2018, 19, 2381 Search PubMed.
- L. Wu and R. Wang, Pharmacol. Rev., 2005, 57, 585–630 CrossRef CAS PubMed.
- Y. You, Y.-X. Zhu, J. Jiang, M. Wang, Z. Chen, C. Wu, J. Wang, W. Qiu, D. Xu, H. Lin and J. Shi, J. Am. Chem. Soc., 2022, 144, 14195–14206 CrossRef CAS PubMed.
- D. J. Selkoe and J. Hardy, EMBO Mol. Med., 2016, 8, 595–608 CrossRef CAS PubMed.
- D. Brambilla, R. Verpillot, B. Le Droumaguet, J. Nicolas, M. Taverna, J. Kóňa, B. Lettiero, S. H. Hashemi, L. De Kimpe, M. Canovi, M. Gobbi, V. Nicolas, W. Scheper, S. M. Moghimi, I. Tvaroška, P. Couvreur and K. Andrieux, ACS Nano, 2012, 6, 5897–5908 CrossRef CAS PubMed.
- J. Lei, C. Wang, X. Feng, L. Ma, X. Liu, Y. Luo, L. Tan, S. Wu and C. Yang, Chem. Eng. J., 2022, 435, 134624 CrossRef CAS.
- Z. Yuan, B. Tao, Y. He, J. Liu, C. Lin, X. Shen, Y. Ding, Y. Yu, C. Mu, P. Liu and K. Cai, Biomaterials, 2019, 217, 119290 CrossRef CAS PubMed.
- X. Feng, J. Lei, L. Ma, Q. Ouyang, Y. Zeng, H. Liang, C. Lei, G. Li, L. Tan, X. Liu and C. Yang, Small, 2022, 18, 2105775 CrossRef CAS PubMed.
- Y. He, Z. Xu, Y. He, G. Cao, S. Ni, Y. Tang, J. Wang, Y. Yuan, Z. Ma, D. Wang and D. Gao, Biomaterials, 2022, 290, 121816 CrossRef CAS PubMed.
- A. Wu, L. Jiang, C. Xia, Q. Xu, B. Zhou, Z. Jin, Q. He and J. Guo, Adv. Sci., 2023, 10, 2303016 CrossRef CAS PubMed.
- P. Lheritier, A. Torelló, T. Usui, Y. Nouchokgwe, A. Aravindhan, J. Li, U. Prah, V. Kovacova, O. Bouton, S. Hirose and E. Defay, Nature, 2022, 609, 718–721 CrossRef CAS PubMed.
- D. Tan, X. Cao, J. Huang, Y. Peng, L. Zeng, Q. Guo, N. Sun, S. Bi, R. Ji and C. Jiang, Adv. Sci., 2022, 9, 2201443 CrossRef CAS PubMed.
- N. A. Kamel, Biophys. Rev., 2022, 14, 717–733 CrossRef CAS PubMed.
- S. Roy, K. A. Deo, K. A. Singh, H. P. Lee, A. Jaiswal and A. K. Gaharwar, Adv. Drug Delivery Rev., 2022, 187, 114361 CrossRef CAS PubMed.
- G. Murillo, A. Blanquer, C. Vargas-Estevez, L. Barrios, E. Ibáñez, C. Nogués and J. Esteve, Adv. Mater., 2017, 29, 1605048 CrossRef PubMed.
- C. Zhang, W. Wang, X. Hao, Y. Peng, Y. Zheng, J. Liu, Y. Kang, F. Zhao, Z. Luo, J. Guo, B. Xu, L. Shao and G. Li, Adv. Funct. Mater., 2021, 31, 2007487 CrossRef CAS.
- J. Chen, L. Song, F. Qi, S. Qin, X. Yang, W. Xie, K. Gai, Y. Han, X. Zhang, Z. Zhu, H. Cai, X. Pei, Q. Wan, N. Chen, J. Wang, Q. Wang and Y. Li, Nano Energy, 2023, 106, 108076 CrossRef CAS.
- M. Sekkarapatti Ramasamy, V. Krishnamoorthi Kaliannagounder, A. Rahaman, C. H. Park, C. S. Kim and B. Kim, ACS Biomater. Sci. Eng., 2022, 8, 3542–3556 CrossRef CAS PubMed.
- J. Zeng, C. Gu, X. Geng, K. Lin, Y. Xie and X. Chen, Biomaterials, 2023, 297, 122122 CrossRef CAS PubMed.
- Y. Yu, H. Sun, Q. Lu, J. Sun, P. Zhang, L. Zeng, K. Vasilev, Y. Zhao, Y. Chen and P. Liu, J. Nanobiotechnol., 2023, 21, 193 CrossRef CAS PubMed.
- L. Ma, X. Zhang, H. Wang, X. Feng, J. Lei, Y. He, J. Wei, Y. Zhang, L. Tan and C. Yang, Sci. China Mater., 2023, 66, 2913–2924 CrossRef CAS.
- C. Mao, W. Jin, Y. Xiang, Y. Zhu, J. Wu, X. Liu, S. Wu, Y. Zheng, K. M. C. Cheung and K. W. K. Yeung, Adv. Mater., 2023, 35, 2208681 CrossRef CAS PubMed.
- Q. Truong Hoang, K. A. Huynh, T. G. Nguyen Cao, J. H. Kang, X. N. Dang, V. Ravichandran, H. C. Kang, M. Lee, J. Kim, Y. T. Ko, T. I. Lee and M. S. Shim, Adv. Mater., 2023, 35, 2300437 CrossRef CAS PubMed.
- L. Xia, J. Chen, Y. Xie, S. Zhang, W. Xia, W. Feng and Y. Chen, J. Mater. Chem. B, 2023, 11, 2895–2903 RSC.
- C. Dai, S. Zhang, Z. Liu, R. Wu and Y. Chen, ACS Nano, 2017, 11, 9467–9480 CrossRef CAS PubMed.
- S. Lin, M. Yang, J. Chen, W. Feng, Y. Chen and Y. Zhu, Small, 2023, 19, 2204992 CrossRef CAS PubMed.
- L. Jiang, G. Lu, Y. Zeng, Y. Sun, H. Kang, J. Burford, C. Gong, M. S. Humayun, Y. Chen and Q. Zhou, Nat. Commun., 2022, 13, 3853 CrossRef CAS PubMed.
- D. I. Kim, R. H. Jeong, J. W. Lee, S. Park and J.-H. Boo, Funct. Mater. Lett., 2021, 14, 2151020 CrossRef CAS.
- A. Rubinacci, M. Covini, C. Bisogni, I. Villa, M. Galli, C. Palumbo, M. Ferretti, M. A. Muglia and G. Marotti, Am. J. Physiol.: Endocrinol. Metab., 2002, 282, E851–E864 CrossRef CAS PubMed.
- W. R. Walsh and N. Guzelsu, Biomaterials, 1993, 14, 331–336 CrossRef CAS PubMed.
- D. Khare, Biomaterials, 2020, 258, 120280 CAS.
- T. Wang, X. Zhang and D. D. Bikle, J. Cell. Physiol., 2017, 232, 913–921 CrossRef CAS PubMed.
- F.-C. Kao, P.-Y. Chiu, T.-T. Tsai and Z.-H. Lin, Sci. Technol. Adv. Mater., 2019, 20, 1103–1117 CrossRef PubMed.
- J. Liu, B. Zhu, L. Zhang, J. Fan and J. Yu, J. Colloid Interface Sci., 2021, 600, 898–909 CrossRef CAS PubMed.
- A. T. Le, M. Ahmadipour and S.-Y. Pung, J. Alloys Compd., 2020, 844, 156172 CrossRef CAS.
- J. Wei, Y. Liu, Y. Li, Z. Zhang, J. Meng, S. Xie and X. Li, Adv. Healthcare Mater., 2023, 2300338 CrossRef CAS PubMed.
-
G. Tai, B. Reid, L. Cao and M. Zhao, in Chemotaxis, ed. T. Jin and D. Hereld, Humana Press, Totowa, NJ, 2009, vol. 571, pp. 77–97 Search PubMed.
- G. G. Genchi, E. Sinibaldi, L. Ceseracciu, M. Labardi, A. Marino, S. Marras, G. De Simoni, V. Mattoli and G. Ciofani, Nanomedicine, 2018, 14, 2421–2432 CrossRef CAS PubMed.
- M. Sekkarapatti Ramasamy, V. Krishnamoorthi Kaliannagounder, A. Rahaman, C. H. Park, C. S. Kim and B. Kim, ACS Biomater. Sci. Eng., 2022, 8, 3542–3556 CrossRef CAS PubMed.
- D. Lei, N. Liu, T. Su, Q. Zhang, L. Wang, Z. Ren and Y. Gao, Adv. Mater., 2022, 34, 2110608 CrossRef CAS PubMed.
- D. Tan, C. Jiang, N. Sun, J. Huang, Z. Zhang, Q. Zhang, J. Bu, S. Bi, Q. Guo and J. Song, Nano Energy, 2021, 90, 106528 CrossRef CAS.
- Y. Zhong, S. Huang, Z. Feng, Y. Fu and A. Mo, J. Biomed. Mater. Res., 2022, 110, 1840–1859 CrossRef CAS PubMed.
- J. Zhang, Y. Fu and A. Mo, Int. J. Nanomed., 2019, 14, 10091–10103 CrossRef CAS PubMed.
- S. H. Lee, S. Jeon, X. Qu, M. S. Kang, J. H. Lee, D.-W. Han and S. W. Hong, Nano Convergence, 2022, 9, 38 CrossRef CAS PubMed.
- R. Nie, Y. Sun, H. Lv, M. Lu, H. Huangfu, Y. Li, Y. Zhang, D. Wang, L. Wang and Y. Zhou, Nanoscale, 2022, 14, 8112–8129 RSC.
- C. Buten, L. Kortekaas and B. J. Ravoo, Adv. Mater., 2020, 32, 1904957 CrossRef CAS PubMed.
- P. Mayorga-Burrezo, J. Muñoz, D. Zaoralová, M. Otyepka and M. Pumera, ACS Nano, 2021, 15, 10067–10075 CrossRef CAS PubMed.
- I. De, P. Sharma and M. Singh, Eur. J. Pharm. Biopharm., 2022, 173, 73–91 CrossRef PubMed.
- Y. Zhang, S. Chen, Z. Xiao, X. Liu, C. Wu, K. Wu, A. Liu, D. Wei, J. Sun, L. Zhou and H. Fan, Adv. Healthcare Mater., 2021, 10, 2100695 CrossRef CAS PubMed.
- L. F. Jaffe and M.-M. Poo, J. Exp. Zool., 1979, 209, 115–127 CrossRef CAS PubMed.
- N. Patel and M. Poo, J. Neurosci., 1982, 2, 483–496 CrossRef CAS PubMed.
- A. Kotwal, Biomaterials, 2001, 22, 1055–1064 CrossRef CAS PubMed.
- Y. Shan, X. Cui, X. Chen and Z. Li, Wiley Interdiscip. Rev.: Nanomed. Nanobiotechnol., 2023, 15, e01827 Search PubMed.
- Y. Zhang, L. Zhou, X. Gao, C. Liu, H. Chen, H. Zheng, J. Gui, C. Sun, L. Yu and S. Guo, Nano Energy, 2021, 89, 106319 CrossRef CAS.
- S. Vijayavenkataraman, Acta Biomater., 2020, 106, 54–69 CrossRef CAS PubMed.
- G. Viola, J. Chang, T. Maltby, F. Steckler, M. Jomaa, J. Sun, J. Edusei, D. Zhang, A. Vilches, S. Gao, X. Liu, S. Saeed, H. Zabalawi, J. Gale and W. Song, ACS Appl. Mater. Interfaces, 2020, 12, 34643–34657 CrossRef CAS PubMed.
- A. C. Pinho, A. C. Fonseca, A. C. Serra, J. D. Santos and J. F. J. Coelho, Adv. Healthcare Mater., 2016, 5, 2732–2744 CrossRef CAS PubMed.
- M. Sarker, S. Naghieh, A. D. McInnes, D. J. Schreyer and X. Chen, Biotechnol. J., 2018, 13, 1700635 CrossRef PubMed.
- Y. Qian, Y. Xu, Z. Yan, Y. Jin, X. Chen, W.-E. Yuan and C. Fan, Nano Energy, 2021, 83, 105779 CrossRef CAS.
- S. Danti, B. Azimi, M. Candito, A. Fusco, M. S. Sorayani Bafqi, C. Ricci, M. Milazzo, C. Cristallini, M. Latifi, G. Donnarumma, L. Bruschini, A. Lazzeri, L. Astolfi and S. Berrettini, Biointerphases, 2020, 15, 031004 CrossRef CAS PubMed.
- E. Esmaeili, M. Soleimani, M. A. Ghiass, S. Hatamie, S. Vakilian, M. S. Zomorrod, N. Sadeghzadeh, M. Vossoughi and S. Hosseinzadeh, J. Cell. Physiol., 2019, 234, 13617–13628 CrossRef CAS PubMed.
- K. Wang, Y. Jia and X. Yan, Nano Energy, 2022, 100, 107486 CrossRef CAS.
- Y. Li, Y. Hu, H. Wei, W. Cao, Y. Qi, S. Zhou, P. Zhang, H. Li, G.-L. Li and R. Chai, J. Nanobiotechnol., 2022, 20, 398 CrossRef CAS PubMed.
- M. Xiao, X. Li, S. Pifferi, B. Pastore, Y. Liu, M. Lazzarino, V. Torre, X. Yang, A. Menini and M. Tang, Nanoscale, 2022, 14, 10992–11002 RSC.
- A. Gustavsson, P. Brinck, N. Bergvall, K. Kolasa, A. Wimo, B. Winblad and L. Jönsson, Alzheimer's Dementia, 2011, 7, 318–327 CrossRef PubMed.
- D. Eleftheriadou, D. Kesidou, F. Moura, E. Felli and W. Song, Small, 2020, 16, 1907308 CrossRef CAS PubMed.
- Y. Jin, J. Seo, J. S. Lee, S. Shin, H.-J. Park, S. Min, E. Cheong, T. Lee and S.-W. Cho, Adv. Mater., 2016, 28, 7365–7374 CrossRef CAS PubMed.
- D. Zhao, P. Feng, J. Liu, M. Dong, X. Shen, Y. Chen and Q. Shen, Adv. Mater., 2020, 32, 2003800 CrossRef CAS PubMed.
- R. Yamaji, K. Fujita, S. Takahashi, H. Yoneda, K. Nagao, W. Masuda, M. Naito, T. Tsuruo, K. Miyatake, H. Inui and Y. Nakano, Biochim. Biophys. Acta, Mol. Cell Res., 2003, 1593, 269–276 CrossRef CAS PubMed.
- F. Lavini, A. Calò, Y. Gao, E. Albisetti, T.-D. Li, T. Cao, G. Li, L. Cao, C. Aruta and E. Riedo, Nanoscale, 2018, 10, 8304–8312 RSC.
- C. Giuliani, Antioxidants, 2019, 8, 112 CrossRef CAS PubMed.
- N. Gao, J. Zhao, X. Zhu, J. Xu, G. Ling and P. Zhang, Acta Biomater., 2022, S1742706122006614 Search PubMed.
- G. Li, Y. Fan, L. Lin, R. Wu, M. Shen and X. Shi, Sci. China: Chem., 2021, 64, 817–826 CrossRef CAS.
- S. Lin, X. Hu, J. Lin, S. Wang, J. Xu, F. Cai and J. Lin, Analyst, 2021, 146, 4391–4399 RSC.
- Y. Nie, W. Zhang, W. Xiao, W. Zeng, T. Chen, W. Huang, X. Wu, Y. Kang, J. Dong, W. Luo and X. Ji, Biomaterials, 2022, 289, 121791 CrossRef CAS PubMed.
- H. Zhang, H. Yang, P. Liu, X. Qin and G. Liu, Talanta, 2022, 237, 122906 CrossRef CAS PubMed.
- A. Ahmadi, S. M. Khoshfetrat, S. Kabiri, P. S. Dorraji, B. Larijani and K. Omidfar, Microchim. Acta, 2021, 188, 296 CrossRef CAS PubMed.
- N. Gong, Y. Zhang, X. Teng, Y. Wang, S. Huo, G. Qing, Q. Ni, X. Li, J. Wang, X. Ye, T. Zhang, S. Chen, Y. Wang, J. Yu, P. C. Wang, Y. Gan, J. Zhang, M. J. Mitchell, J. Li and X.-J. Liang, Nat. Nanotechnol., 2020, 15, 1053–1064 CrossRef CAS PubMed.
- Q. Ouyang, B. Wang, W. Ahmad, Y. Yang and Q. Chen, Anal. Bioanal. Chem., 2022, 414, 8179–8189 CrossRef CAS PubMed.
- R. Ye, H. Chen and H. Li, Anal. Chim. Acta, 2022, 1225, 340227 CrossRef CAS PubMed.
- L. Vannozzi, L. Ricotti, C. Filippeschi, S. Sartini, V. Coviello, V. Piazza, P. Pingue, C. La Motta, P. Dario and A. Menciassi, Int. J. Nanomed., 2015, 69 CrossRef PubMed.
- Y. Ma, L. Jiang, J. Hu, E. Zhu and N. Zhang, ACS Appl. Mater. Interfaces, 2022, 14, 44065–44083 CrossRef CAS PubMed.
- D. Huang, T. Wu, S. Lan, C. Liu, Z. Guo and W. Zhang, Biomaterials, 2022, 289, 121808 CrossRef CAS PubMed.
- B. Li, G. Hao, B. Sun, Z. Gu and Z. P. Xu, Adv. Funct. Mater., 2020, 30, 1909745 CrossRef CAS.
- M. Coluccia, V. Parisse, P. Guglielmi, G. Giannini and D. Secci, Eur. J. Med. Chem., 2022, 244, 114801 CrossRef CAS PubMed.
- S. Zheng, Y. Tian, J. Ouyang, Y. Shen, X. Wang and J. Luan, Front. Chem., 2022, 10, 990362 CrossRef CAS PubMed.
- D. V. Shtansky, A. T. Matveev, E. S. Permyakova, D. V. Leybo, A. S. Konopatsky and P. B. Sorokin, Nanomaterials, 2022, 12, 2810 CrossRef CAS PubMed.
- K. Vimala, K. Shanthi, S. Sundarraj and S. Kannan, J. Colloid Interface Sci., 2017, 488, 92–108 CrossRef CAS PubMed.
- C. Mo, Z. Wang, J. Yang, Y. Ouyang, Q. Mo, S. Li, P. He, L. Chen and X. Li, J. Photochem. Photobiol., B, 2022, 233, 112487 CrossRef CAS PubMed.
- T. Hu, Z. Gu, G. R. Williams, M. Strimaite, J. Zha, Z. Zhou, X. Zhang, C. Tan and R. Liang, Chem. Soc. Rev., 2022, 51, 6126–6176 RSC.
- H. Zhao, S. Xue, M.-D. Hussherr, A. P. Teixeira and M. Fussenegger, Sci. Adv., 2022, 8, eabm4389 CrossRef CAS PubMed.
- Z. Liu, J. Nie, B. Miao, J. Li, Y. Cui, S. Wang, X. Zhang, G. Zhao, Y. Deng, Y. Wu, Z. Li, L. Li and Z. L. Wang, Adv. Mater., 2019, 31, 1807795 CrossRef PubMed.
- E. Abu-El-Rub, R. R. Khasawneh and F. Almahasneh, World J. Stem Cells, 2022, 14, 513–526 CrossRef PubMed.
- M. Jeong, Y. Jung, J. Yoon, J. Kang, S. H. Lee, W. Back, H. Kim, M. J. Sailor, D. Kim and J.-H. Park, ACS Nano, 2022, 16, 16118–16132 CrossRef CAS PubMed.
- N. Muzzio, M. Eduardo Martinez-Cartagena and G. Romero, Adv. Drug Delivery Rev., 2022, 190, 114554 CrossRef CAS PubMed.
- C. Pucci, A. Marino, Ö. Şen, D. De Pasquale, M. Bartolucci, N. Iturrioz-Rodríguez, N. di Leo, G. de Vito, D. Debellis, A. Petretto and G. Ciofani, Acta Biomater., 2022, 139, 218–236 CrossRef CAS PubMed.
- Y. Chen, B. Ma, X. Wang, X. Zha, C. Sheng, P. Yang and S. Qu, J. Diabetes Res., 2021, 2021, 1–10 Search PubMed.
- J. Zhuang, X. Zhang, Q. Liu, M. Zhu and X. Huang, Theranostics, 2022, 12, 6223–6241 CrossRef CAS PubMed.
- Y. Qiao, W. Zhang, P. Tian, F. Meng, H. Zhu, X. Jiang, X. Liu and P. K. Chu, Biomaterials, 2014, 35, 6882–6897 CrossRef CAS PubMed.
- S. Lin, S. Yin, J. Shi, G. Yang, X. Wen, W. Zhang, M. Zhou and X. Jiang, Bioact. Mater., 2022, 18, 116–127 CAS.
- Q. Wu, L. Hu, R. Yan, J. Shi, H. Gu, Y. Deng, R. Jiang, J. Wen and X. Jiang, Bone Res., 2022, 10, 55 CrossRef CAS PubMed.
- X. Yao, S. Lu, C. Feng, R. Suo, H. Li, Y. Zhang, Q. Chen, J. Lu, B. Wu and J. Guo, Biomaterials, 2022, 289, 121801 CrossRef CAS PubMed.
- M. Mathesh, B. Luan, T. O. Akanbi, J. K. Weber, J. Liu, C. J. Barrow, R. Zhou and W. Yang, ACS Catal., 2016, 6, 4760–4768 CrossRef CAS.
- A. S. Timin, A. R. Muslimov, M. V. Zyuzin, O. O. Peltek, T. E. Karpov, I. S. Sergeev, A. I. Dotsenko, A. A. Goncharenko, N. D. Yolshin, A. Sinelnik, B. Krause, T. Baumbach, M. A. Surmeneva, R. V. Chernozem, G. B. Sukhorukov and R. A. Surmenev, ACS Appl. Mater. Interfaces, 2018, 10, 34849–34868 CrossRef CAS PubMed.
- G.-H. Feng and W.-M. Tseng, IEEE Sens. J., 2018, 18, 9736–9743 CAS.
- Y. Zhang, Q. An, W. Tong, H. Li, Z. Ma, Y. Zhou, T. Huang and Y. Zhang, Small, 2018, 14, 1802136 CrossRef PubMed.
- Y. Zhang, C. Tong, Z. Ma, L. Lu, H. Fu, S. Pan, W. Tong, X. Li, Y. Zhang and Q. An, Nanoscale, 2019, 11, 14372–14382 RSC.
- Q. Liang, J. Xi, X. J. Gao, R. Zhang, Y. Yang, X. Gao, X. Yan, L. Gao and K. Fan, Nano Today, 2020, 35, 100935 CrossRef CAS.
- Y. Wang, Q. Tang, R. Wu, S. Sun, J. Zhang, J. Chen, M. Gong, C. Chen and X. Liang, ACS Nano, 2023, 17, 3557–3573 CrossRef CAS PubMed.
Footnote |
† These authors contributed equally to this work. |
|
This journal is © The Royal Society of Chemistry 2024 |
Click here to see how this site uses Cookies. View our privacy policy here.