DOI:
10.1039/D3NH00305A
(Review Article)
Nanoscale Horiz., 2024,
9, 334-364
Advances in screening hyperthermic nanomedicines in 3D tumor models
Received
20th July 2023
, Accepted 3rd January 2024
First published on 5th January 2024
Abstract
Hyperthermic nanomedicines are particularly relevant for tackling human cancer, providing a valuable alternative to conventional therapeutics. The early-stage preclinical performance evaluation of such anti-cancer treatments is conventionally performed in flat 2D cell cultures that do not mimic the volumetric heat transfer occurring in human tumors. Recently, improvements in bioengineered 3D in vitro models have unlocked the opportunity to recapitulate major tumor microenvironment hallmarks and generate highly informative readouts that can contribute to accelerating the discovery and validation of efficient hyperthermic treatments. Leveraging on this, herein we aim to showcase the potential of engineered physiomimetic 3D tumor models for evaluating the preclinical efficacy of hyperthermic nanomedicines, featuring the main advantages and design considerations under diverse testing scenarios. The most recent applications of 3D tumor models for screening photo- and/or magnetic nanomedicines will be discussed, either as standalone systems or in combinatorial approaches with other anti-cancer therapeutics. We envision that breakthroughs toward developing multi-functional 3D platforms for hyperthermia onset and follow-up will contribute to a more expedited discovery of top-performing hyperthermic therapies in a preclinical setting before their in vivo screening.
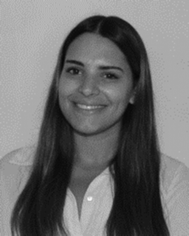
Joana F. Soeiro
| Joana Soeiro is a PhD student in the Department of Chemistry of the University of Aveiro. She obtained her MS in Biomedical Engineering from the University of Aveiro in 2021. Her PhD research is focused on studying the theranostic ability of magnetic nanoparticles for optical hyperthermia, as contrast agents, and as cellular thermometers for MRI. |
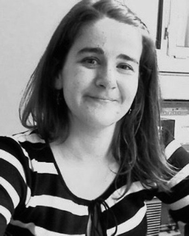
Filipa L. Sousa
| Filipa L. Sousa graduated and received her Master degree from University of Aveiro, Portugal in 2000 and 2003, respectively. Then she moved to the University of Bielefeld, Germany where she got her PhD degree in 2010. Afterwards, she joined University of Aveiro first as a postdoctoral researcher and later as Auxiliary Researcher at the Chemistry Department and CICECO. Her research interests included polyoxometalate-based materials for biomedical applications, as well as the development of magnetic nanoparticles applied to nanosensing. |
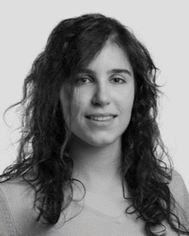
Maria V. Monteiro
| Maria V. Monteiro holds a MSc in Biotechnology where she developed novel stratified pancreatic cancer 3D models that accurately recapitulate the human pancreatic cancer signatures. Currently, she is a PhD student in Biotechnology at the COMPASS Research Group based in the Associate Laboratory CICECO – Aveiro Institute of Materials. Her currently PhD work focuses on the development of decellularized pancreatic cancer matrix-based hydrogels to be integrated with novel stratified hydrogel-based platforms for pancreatic cancer modelling. Additionally, she is working on the development of chemical modified biopolymers, 3D bioprinted constructs and bioprinted cancer-on-chip devices for advanced tumor modelling and high-throughput screening assays. |
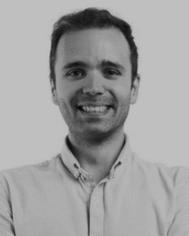
Vítor M. Gaspar
| Vítor M. Gaspar is an Assistant Researcher at the Chemistry Department of the University of Aveiro and member of COMPASS Research Group, in the Associate Laboratory CICECO – Aveiro Institute of Materials. He holds a PhD in Biochemistry and currently his research interests include the precision chemical modification of ECM-biomimetic and tissue-derived biomaterials, particularly decellularized extracellular matrix. He has been focusing on the use of these matrices for the bottom-up engineering of cell-biomaterial living 3D microtissues and organoids engineering. He has been leveraging advanced manufacturing techniques such as 3D/4D bioprinting for processing cells and materials toward tissue engineering and in vitro disease modelling applications. |
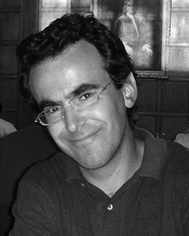
Nuno J. O. Silva
| Nuno J. O. Silva is a Principal Researcher at the University of Aveito. He studied physics at the University of Porto and University of Aveiro, where he got interested in the magnetic properties of nanoparticles and aqueous ferrofluids. He is now exploring the magnetothermal properties of nanoparticles for imaging, supported by a ERC grant. |
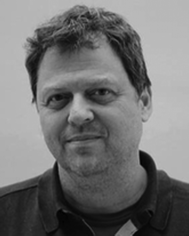
João F. Mano
| João F. Mano is a full professor in the Chemistry Department of the University of Aveiro, Portugal, and director of the COMPASS Research Group from the Associate Laboratory CICECO – Aveiro Institute of Materials. His research interests include the use of biomaterials and cells toward the progress of transdisciplinary concepts to be employed in regenerative and personalized medicine. He has applied biomimetic and nano/micro-technology approaches to polymer-based biomaterials and surfaces to develop biomedical devices with improved structural and (multi-)functional properties, or in the engineering of microenvironments to control cell behavior and organization, to be exploited clinically in advanced therapies or in drug screening. |
1 Introduction
Hyperthermia for cancer therapy is continuously evolving as a valuable strategy compared to standard chemotherapeutic treatments owing to its potential for heating tissues to induce cancer cell death and increase the immune response in a more controlled and localized mode.1,2 As cancer cells are more susceptible to heat damage than normal cells, hyperthermia can induce cancer cell death with minimal injury to the healthy tissue surrounding the tumor.3,4 The damage caused in cells depends on the achieved temperature and the duration of the procedure.5 Three ranges can be defined: 40–42 °C (mild hyperthermia), 42–45 °C (moderate hyperthermia), and ≥50 °C (ablation).6 Thermal ablation is usually performed for short periods and causes irreversible cell damage by inducing apoptosis.6,7 Mild and moderate hyperthermia are performed for longer periods, inducing changes in the blood perfusion and oxygenation of the tissue, causing protein denaturation and aggregation. Furthermore, mechanisms of DNA repair and cell proliferation can be inhibited, ultimately altering the physiology of the tumor.7,8 Recent evidence pointing toward hyperthermic activation of immune cells and increased resistance against secondary tumors further supports the validity of such hyperthermic strategies.9
In recent years, different types of nanomaterials approved by the Food and Drug Administration (FDA) for hyperthermia have been explored in the clinical setting (e.g., Aurolase®, Nanospectra Biosciences, Inc., Houston, TX; and NanoTherm®, MagForce AG, Berlin, Germany) since they can be engineered to accumulate in the desired area and generate heat upon an external stimulus, typically an electromagnetic wave or an alternating magnetic field (AMF).10 Thus, nanomaterials can improve selectivity to prevent major injury in the tissues surrounding the tumor.11 Despite relevant developments, hyperthermia mediated by nanomaterials still faces several challenges, such as guaranteeing maximum cancer cell selectivity, homogeneous heating of the target tissue, and effective methods for temperature control/real-time readout during treatments.12 Consequently, current hyperthermic strategies require further engineering and improvements of 3D tumor models prior to their widespread acceptance as a standard-of-care therapeutic modality.13
Conventionally, the preclinical validation of candidate hyperthermic nanomedicines has been routinely performed in gold-standard flat 2D cell cultures.14,15 However, these are unable to recapitulate the 3D architecture of the human tumor microenvironment (TME), as well as the complexity of its cellular (i.e., cancer, stromal, immune cells, etc.) and non-cellular elements (i.e., extracellular matrix (ECM)),16 ultimately leading to a sub-optimal in vitro/in vivo correlation that impacts human clinical trial validation stages and ultimately translation into the market.17,18 Exploring 3D in vitro tumor models as alternative preclinical platforms for hyperthermic nanomedicines validation opens the possibility to recapitulate: (i) human solid tumors gene expression profiles associated with heat/drug resistance (i.e., heat-shock proteins – Hsp70/Hsp90,14,19 drug resistance mechanisms – ABC transporters20), (ii) the modulation of the secretion of key exosomes,21 as well as (iii) the establishment of hypoxic/necrotic regions and volumetric pH gradients,22 in an approach that is significantly more similar to that found in vivo.23 The coexistence of multiple cancer-stroma cellular populations and the dynamic cell–cell or cell–TME interactions in 3D can also be explored to better contribute to evaluating hyperthermic therapeutics performance.24–28 Upgrading from the conventional use of monolayers toward volumetric tumor models also enables researchers to specifically evaluate the influence of solid tumors on heat transfer mechanisms and nanomedicines penetration in 3D in a more biomimetic set-up.18,26,29 The latter is particularly relevant, since a sub-optimal and non-homogeneous distribution of hyperthermic nanomedicines within the tumor volume may impact the overall therapeutic outcome. Leveraging 3D models and high-throughput/high-content imaging approaches to improve the selection process of top-performing hyperthermic nanomedicines at preclinical stages also contributes to reducing the use of laboratory animals and surpasses major ethical and economic issues associated with these models.24
Despite the promising advantages of advanced 3D tumor models, these have yet to be broadly adopted during hyperthermic nanomedicine design and performance screening.30 Aiming to shed light on recent advances, the key aspects of hyperthermia nanomedicines validation in advanced 3D in vitro models are herein addressed. An informative discussion focusing on the importance of heat transfer simulations in 3D and real-time temperature evaluation strategies during treatment is also provided, considering the highly required advances in technologies to monitor hyperthermia nanomedicines in a non-invasive mode. State-of-the-art examples leveraging on the use of 3D in vitro tumor models for screening and validating nano-hyperthermia technologies will be showcased and discussed, considering their contribution to further consolidate and upgrade this therapeutic methodology. It is envisaged that advances from upgraded preclinical validation models will contribute to changing the current approaches for the validation of innovative hyperthermic nanomedicines, opening new avenues for accelerating their translation toward the clinical scenario.
2 Hyperthermia technologies
Conventional hyperthermia for cancer management is performed by exploiting: (i) electromagnetic waves: radiofrequency (RF), microwaves (MW), near-infrared (NIR) light, and (ii) mechanical waves, namely ultrasound (US).31,32 Even though these methods are efficient in increasing the temperature of tissues, they fail to target only the desired tissue.11 This targeting can be dramatically enhanced using nanosized heating agents that are more efficient than tissues in absorbing the incoming wave, leading to an increase in temperature in a specific region. Hyperthermia has been rapidly emerging owing to its higher precision and therapeutic versatility, particularly in the case of photothermal therapy (PTT) and magnetic hyperthermia (MH), complementing the available toolset of hyperthermia technologies.33–35Fig. 1 highlights examples of traditional hyperthermia methods and examples of nanomaterials used as heating agents in hyperthermia procedures.11,31,36–38
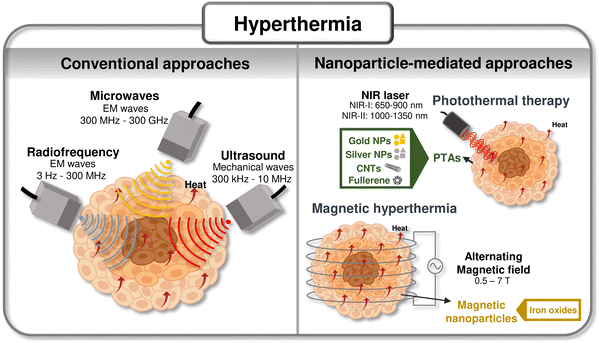 |
| Fig. 1 Summary of hyperthermic technologies: Scheme of currently available hyperthermic technologies and advanced approaches leveraging nanomaterials as heating agents. Compiled from ref. 11, 31 and 36–38. | |
2.1 Photothermal nanotherapy
In PTT approaches, nanosized photothermal agents (nano-PTAs) are leveraged to convert electromagnetic radiation, usually NIR, into thermal energy to heat cancer cells.39 The interest in NIR-II-absorbing materials (NIR-II: 1000–1350 nm40) has recently increased since they show a reduced light scattering, enable a higher spatial resolution, and higher signal-to-background ratio compared to NIR-I-absorbing materials (NIR-I: 650–900 nm40) which have been the most explored for PTT.41,42
To date, a wide range of inorganic (i.e., carbon based-nanomaterials, noble metal nanoparticles (NPs), metal-oxide NPs), and organic nanomaterials (i.e., conjugated polymers, semiconducting polymers, organic dyes, etc.) have been exploited for PTT methodologies.11,43,44Table 1 reports examples of nano-PTAs recently used for hyperthermic nanotherapy screening in 2D in vitro models. Both classes of nano-PTAs present advantages and disadvantages, with most inorganic nano-PTAs being non-biodegradable and exhibiting limited biocompatibility, while organic nano-PTAs (i.e., biodegradable, and biocompatible) commonly exhibit lower photothermal conversion efficiency (PCE) and reduced photostability.45–47 The rationale selection of nano-PTA should thus account for several factors, including the type of cancer, the tissue/organ being treated, the location of the treatment, and the optimal light wavelength.
Table 1 Examples reporting the performance of nano-PTAs for 2D in vitro PTT
Heating agent |
PCEb (%) |
Heating conditions |
2D tumor model |
Dose (μg mL−1) |
T reached (°C) |
Cell death (%) (time after treatment, technique) |
Ref. |
Core |
Coating |
Size w/coating (nm) |
Value extracted from graphs.
The conditions that are not indicated are the same as the heating conditions; N.D. – non described; PCE: photothermal conversion efficiency; MCNTs: multiwalled carbon nanotubes; SCNTs: single walled carbon nanotubes; DPP: diketopyrrolopyrrole; TPA: triphenylamine; PEG: polyethylene glycol; FC: flow cytometry; NRs: nanorods; PVP: polyvinylpyrrolidone.
Value from the literature indicated in the article.
|
SCNTs and MCNTs loaded with therapeutic siRNA |
Peptide lipid and sucrose laurate |
MCNTs: length: 0.5–2 μm; diameter: 30 nm; SCNTs: length, 1–3 μm, diameter: 24 nm, TEM |
SCNTs ∼ 59 |
808 nm, 1 W cm−2, 5 min |
HeLa |
30 |
42–45 |
SCNTs: ∼30a |
48
|
MCNTs ∼ 58 |
MCNTs: ∼40a (PTT alone) (24 h, MTT) |
MCNTs |
Amide |
Length: 591 |
N.D. |
1064 nm, 3 W, 5–45 s |
HMLERshEcadherin, HMLERshControl |
50 |
49 |
∼40a (for both cell lines) (24 h, MTT) |
49
|
Diameter: 29 |
Technique: N.D. |
DPP NPs |
TPA |
∼76, DLS |
∼35 (80 μg mL−1) |
660 nm, 0.5 and 1 W cm−2, 5 min |
HeLa |
20 |
N.D. |
0.5 W cm−2: ∼30a; 1 W cm−2: ∼70a (48 h, MTT) |
50
|
Black mesoporous silicon NPs |
PEG |
156, DLS (w/o coating) |
∼33 (100 μg mL−1) |
808 nm, 1 W cm−2, 10 min |
CT26 |
75 |
N.D. |
84 (12 h, FC) |
51
|
Chitosan-based hydrogels with nanosized {Mo154} |
— |
3.4c {Mo154}, technique: N.D. |
N.D. |
808 nm, 0.8 W cm−2, 10 min |
M21 |
0.092 wt% |
N.D. |
∼98 (after irradiation, CellTiter Glo) |
52
|
Gellan Gum and Ca2+ hydrogels with AuNRs |
PEG |
N.D. |
∼21 (100 μg mL−1) |
(1) 808 nm, 0.5 W cm−2, 2 min; and (2) 660 nm, 50 mW cm−2, 5 min |
HeLa and MCF-7 |
50 |
66.2 |
∼44 and ∼66 for HeLa and MCF-7 cells, respectively (12 h, CCK8) |
53
|
Ag NPs |
PVP |
∼93, DLS |
N.D. |
970 nm, 3 W, 70 s |
MDA-MB-231 |
12.5 |
52.4 |
>85 (72 h, MTT) |
54
|
Au25 nanoclusters conjugated with ICG |
Gluta-thione |
∼3.4, DLS |
N.D. |
808 nm |
MCF-7 |
30 μM |
N.D. |
0.5 W cm−2: ∼35a |
55
|
0–1 W cm−2 |
0.8 and 1 W cm−2: ∼100a (after irradiation, MTT) |
5 min |
|
Carbon-based materials, including graphene,56–58 carbon nanotubes (CNTs),59,60 fullerenes,61,62 and carbon dots,63,64 have been increasingly used as nano-PTAs as they display high absorption in the NIR region, suitable biocompatibility, and can generally accumulate in the tumor site due to their nano size. Furthermore, these can be easily functionalized to improve their water dispersibility, biocompatibility, and tumor-targeting ability.36,65 Carbon-based nano-PTAs have the potential for multimodal imaging, integration of PTT with other therapies, such as photodynamic therapy (PDT), and can also be used as drug delivery vehicles.66–68
Noble metal NPs, such as gold (Au),69,70 silver (Ag),54,71 or palladium (Pd) NPs,72,73 have been shown to have an adequate performance as nano-PTAs due to their biocompatibility, suitable sizes for biological applications, and strong localized surface plasmon resonance that gives them the ability to be good absorption agents.74,75 These materials can be easily functionalized and conjugated with molecules to increase their targeting ability and biocompatibility.76 Furthermore, noble metal NPs can be used for imaging applications and combined therapies, such as PDT, immunotherapy, or chemotherapy.77 By tuning the size or shape of the structure, the absorption range can be easily changed.75
Organic dyes have strong light absorption in the visible or NIR region and can be easily synthesized and functionalized for specific applications.50,78 Furthermore, they can be simultaneously used as fluorescent probes for imaging and as PTAs.46 Organic dyes can be rapidly cleared from the body, preventing high toxicity, yet this can limit the duration of the treatment. Such dyes also have limited tumor selectivity, and generally poor stability in aqueous mediums.57,79,80 To address these limitations, organic dyes have been commonly associated with other nano-sized structures, such as micelles or liposomes, in an attempt to increase their stability and tumor selectivity.80 Examples of organic dyes used as nano-PTAs include indocyanine green (ICG),55,81,82 methylene blue (MB),53,83 and IR780.84–86
2.2 Magnetic hyperthermia
In MH, magnetic nanoparticles (MNPs) are used to transduce magnetic energy produced by an AMF into heat.34,87,88 The inability of the magnetic moments of the MNPs to follow the AMF totally in phase leads to hysteresis and heat generation. This inability may be due to the physical rotation of the MNPs (Brown mechanism), to the rotation of the magnetic moment as a single moment across an energy barrier provided by the crystal lattice (Neél mechanism), or to rearrangement of the orientation of the magnetic moments in the case of multi-domain larger MNPs. The heat generated by the MNPs is dependent on the frequency of the applied magnetic field, the size and morphology of MNPs, and the biological properties of the tissue.87,89–91 The amount of heat dissipated per unit mass of MNPs is commonly quantified by the specific absorption rate (SAR), which indicates MNPs heat generation efficiency upon the application of a magnetic stimulus.92,93
The efficiency of the MNPs in MH depends on their coating, size, morphology, and magnetic properties (e.g., saturation magnetization (Ms), magnetic susceptibility, and magnetocrystalline anisotropy).34,88,94,95 The size and morphology of the MNPs are key features to be considered due to their influence on cellular uptake, which will be further discussed.96 Moreover, the size of the MNPs will influence the mechanism responsible for generating heat within the tumor: hysteresis losses due to domain rotation/reconfiguration are more significant in multi-domain NPs, and Brownian and Néel relaxation losses are dominant in single-domain NPs.90,97,98 The size distribution of MNPs should be uniform to contribute to a homogeneous distribution of heat, and the MNPs should be well dispersed in small concentrations.87,88,90 Usually, larger particles have a higher Ms, which is proportional to the heating efficiency of the MNPs. So, a compromise between the size of the NPs must be found to maximize their heating capacity and cellular uptake.87,99,100 Besides being important to guarantee a high Ms and magnetic susceptibility, MNPs should have a suitable volume and magnetocrystalline anisotropy, whose product is the energy required to change the orientation of the magnetic moment of the particles, to match the characteristic relaxation time and the AMF frequency, aiming to maximize the heating efficiency.88,101,102
Functionalization of MNPs with hydrophilic and low toxic materials is usually performed to increase both the dispersibility of NPs in water and colloidal stability.94 Moreover, it improves biocompatibility and tumor target selectivity, prevents MNPs from agglomerating, and increases the NPs' circulation time.87,95,103,104 The material of the surface coating, its thickness, and the size of the core influence the heating efficiency of the final particle. Usually, a thinner coating and a larger core size contribute to a higher SAR, while a thicker coating and a smaller core size contribute to a lower SAR by inhibiting Brownian relaxation. Moreover, MNPs with a low dispersibility behavior require a thicker coating to improve their stability and prevent aggregation.88,105 So, a compromise between the thickness of the coating and the size of the core must be found to maximize the SAR, dispersibility, and colloidal stability in aqueous medium.88 Coating MNPs with appropriate materials such as poly(lactic-co-glycolic acid) (PLGA), polyvinyl alcohol (PVA), poly(vinylpyrrolidone) (PVP), polyethylene glycol (PEG), or dextran has been shown to improve NPs-cell interactions and also prevent toxic side-effects.106,107 Lipid-based nanomaterials, such as liposomes108,109 or niosomes,110,111 have also been used as coatings for MNPs due to their biocompatibility, flexible design, and surface modification capacity. Both polymeric and lipid coatings allow for a controlled release of the inner NPs/drugs upon the use of different stimuli (e.g., temperature, pH, light, magnetic or electric fields, etc.), which is advantageous, for example, for target drug delivery and for MRI contrast.112,113 Recently, hybrid systems consisting of polymer and lipid coatings have emerged as promising approaches capable of improving NPs’ stability, biocompatibility, and drug release kinetics.114,115
Superparamagnetic iron oxide NPs (SPIONs) have been the most used for MH as they show valuable size-dependent magnetic properties, suitable biocompatibility, reduced toxicity, high surface-to-volume ratio, proper stability in aqueous suspension, can be easily functionalized, and are FDA approved.116–118 Moreover, these particles have been shown to have the potential to induce heat by optical stimulation, which, in combination with MH, can contribute for increasing the efficiency of candidate anti-cancer treatments.119Table 2 summarizes the most recent studies exploring MNPs for hyperthermic nanomedicines screening in flat 2D in vitro models. The use of MNPs has several advantages since, in addition to being used as heat sources, they can also be used as contrast agents, non-invasive temperature thermometers, or for complementary targeted drug delivery.120–122 However, it is still a challenge to guarantee that the MNPs are homogeneously distributed in the target region, achieve uniform heating, and to ensure that the magnetic properties of the NPs/administered concentration are adequate to promote an effective outcome.119,123
Table 2 Examples reporting the performance of MNPs for 2D in vitro MH
Heating agent |
Heating conditions |
SARc (W g−1) |
M
s (emu g−1) |
2D tumor model |
Dose (μg mL−1) |
T reached (°C) |
Cell death (%) (time after treatment, technique) |
Ref. |
Core |
Coating |
Size w/coating (nm) |
Value extracted from graphs.
Dose of Fe.
The conditions that are not indicated are the same as the heating conditions; N.D. – non described; FC: flow cytometry; AB: Alamar Blue; CA: citric acid; PCL: polycaprolactone; PVP: polyvinylpyrrolidone; GO: graphene oxide.
|
Fe3O4 |
Aminosi-lane |
110, DLS |
557 kHz, 300 Gauss, ∼24 kA m−1, 40 min |
338 |
N.D. |
C6 |
100b |
44 |
∼70 (N.D.; FC) |
126
|
Fe3O4 |
CA |
46, TEM |
60 kA m−1, 30 min |
2380b (325 μg mL−1 b, 29 kA m−1) |
83 |
A549 |
46b |
43.6 |
∼20a |
127
|
85.5b |
45.95 |
∼80a |
177.5b |
49.67 |
∼100% (24 h, AB) |
Fe3O4 |
PCL |
21, TEM |
318 kHz, ∼32 kA m−1, 15 min |
153 (103 μg mL−1) |
64 |
HepG2 |
100 |
43.2 |
∼39 |
128
|
313 kHz, ∼47 kA m−1, 15 min |
201 (103 μg mL−1) |
46.1 |
∼60% (24 h, MTT) |
Fe3O4 |
PVP |
145, DLS |
∼333 kHz, 170 Oe, 15 min |
160 (104 μg mL−1) |
72 |
MDA-MB-231 |
500 |
42.5 |
75 (24 h, MTT) |
129
|
CoMn–Fe2O4 |
PEG–PCL |
∼79, DLS |
420 kHz, ∼27 kA m−1, 30 min |
1237 (w/coating) |
93 (w/o coating) |
ES-2 |
50 |
55a |
∼99 (48, calcein AM) |
130
|
GO–Fe3O4 |
— |
20, XRD |
236 kHz, ∼4 kA m−1, 10 min |
70a |
50a |
HeLa |
1000 |
N.D. |
40 (2 h, MTT) |
131
|
MnFe2O4 |
— |
31, TEM |
765 kHz, 300 Oe, (1) 4–6 min followed by (2) 18–23 min after 2 days |
∼300a |
∼70 |
Saos-2 |
250 |
(1) ∼45a |
(1) 25a; (2) 75a |
132
|
500 |
(2) ∼43a-for both doses |
(1) 30a; (2) 90a (N.D.) |
MH and PTT are complementary, in the sense that while PTT is more suitable for surface and near-surface applications, MH is applicable in deep tissues, since the penetration of the AMF exceeds the penetration depth of light.94,124,125 Consequently, MH can be used to trigger hyperthermia in deeper tumors than PTT.87
When designing nanomedicines for hyperthermic applications, it is important to consider the factors that affect their penetration in tumors that is influenced by NPs’ properties (e.g., size, morphology, or surface chemistry/functionalization), and by physical and biological barriers (e.g., uptake by the immune system, shear stress under circulation, renal filtration, interstitial fluid pressure, or tumor desmoplasia).133,134 In general, NPs bigger than 200 nm are easily accumulated in the liver and spleen, and NPs smaller than 6 nm are usually filtered by the kidney, not being able to accumulate in the target site.95,135 Moreover, coating the NPs with neutral polymers generally reduces clearance mediated by the immune system.133 Since some tumor types have a leaky vasculature with increased permeability and poor lymphatic drainage, nano-sized agents are more likely to be passively internalized and retained by the tumor after systemic administration, which is referred to as the enhanced permeability and retention (EPR) effect.95,136 In fact, the internalization of nano-agents as drug carriers in tumors can exhibit over a 10-fold increase in effectiveness compared to free-drugs, even though only 10 to 15% of injected NPs successfully accumulate within the tumor.137 Approaches aiming to improve the accumulation and retention of NPs in tumors include, for example, the use of ligands or antibodies to bind with specific malignant cell receptors to promote a targeted delivery.138–140 Regardless of their physicochemical features, the preclinical validation of such systems is a requirement before they are considered for clinical applications.
To date, the preclinical performance and validation of such engineered nano-heating agents for localized heat generation has been mainly performed in 2D cell monolayers and/or mice models.141,142 Recently, the use of 3D in vitro models in the design and validation stages of hyperthermic nanomedicines has been rapidly emerging as an alternative strategy, owing to its potential to overcome the issues of 2D cultures, for significantly reduce animal model usage, and for accelerate the identification of top performing nano-heating agents.
To fully explore the potential of 3D in vitro tumor models for evaluating the performance of hyperthermic nanomedicines, it is important to discuss the relevance of the third dimension in what relates to NPs’ penetration and distribution in the complex TME, as well as heat transfer mechanisms, that are otherwise more difficult to be modeled in 2D cell cultures.
3
In vitro tumor models for nanomedicines screening
3.1. Mimicking the tumor microenvironment (TME): 2D versus 3D models
The complexity of tumors and their interactions with the surrounding microenvironment has prompted researchers to develop more advanced and sophisticated 3D tumor models that better recapitulate different tumor hallmarks in a preclinical setting when compared to the limited 2D flat cell monolayers methodologies.143 Owing to the inherent spatial differences, 2D and 3D tumor models recapitulate the TME components and its biological hallmarks differently in an in vitro setting.
In the native TME, the tri-dimensional existence of the surrounding stroma plays an important role in tumor progression, invasion, and metastasis, for example by producing enzymes to degrade the ECM, by supplying biomolecular cues to promote cancer cell growth and angiogenesis, by suppressing the immune response, or by recruiting healthy cells.144–146 The stroma is comprised by the ECM and other cell types, such as endothelial cells, cancer-associated fibroblasts (CAFs), mesenchymal supporting cells, cells of the vascular/lymphatic system, and immune system cells.147–149 In malignant tissues, the ECM undergoes significant alterations resulting in de novo deposition of ECM, where proteins (e.g., collagen, fibronectin, laminin, etc.) are upregulated and an enzyme-mediated (e.g., lysyl oxidase) stiffness increase occurs, which represents a physical barrier to therapies.27,150,151 Each cancer-associated cell type has its own function on tumor progression, making it important to consider the rationale addition of these living units in the design stage of a 3D in vitro tumor model.144 For example, fibroblasts contribute to ECM de novo deposition and remodeling of ECM proteins, facilitating the invasion of cancer cells into neighboring tissues, while immune cells can also have tumor promoting capacity after being recruited by other cancer cells.149,152
In conventional 2D platforms, cells grow as monolayers in adherent conditions. This results in a flat and homogeneous environment that deprives cell–cell and cell–ECM interactions and leads to changes in cellular morphology and function. Moreover, 2D models were shown to modify cellular polarity, morphology, secretion, gene expression, and signaling. These models generally only focus on one cell type (monotypic), making it difficult to co-culture different cellular components, which often neglects the essential contribution of stromal components to tumor development. 3D tumor models provide a far more realistic volumetric architectural rearrangement and cellular representation of the TME by allowing the inclusion of stromal components and enabling to easily recapitulate cellular diversity and heterogeneity.14,26,141,153
Since cancer cells are highly proliferative, the angiogenic process (i.e., formation of vasculature) cannot generally keep pace, leading to the disorganized formation of leaky and branch vessels with irregular sizes and increased permeability, in some cancers.154 This, in turn, results in an increase in the interstitial pressure. Consequently, the inner cells of the tumor mass become deprived of nutrients and oxygen, leading to the formation of a hypoxic and acidic environment with gradients of oxygen, nutrients, and metabolites.152,155,156 2D in vitro testing platforms, where cells are cultured in an air–liquid interface, are unable to mimic these gradients or allow to recapitulate the formation of vessel-like structures, elements that are essential to better understand tumor behavior.23
The hypoxic microenvironment leads cancer cells to adapt their metabolism to promote survival, invasion, and metastasis. These adaptative changes are further supported by proteomic and genomic alterations.157 Interactions between the stroma and cancer cells can also drive cancer progression by secreting growth factors and signaling molecules (e.g., transforming growth factor-β (TGF-β), stromal-derived factor (SDF-1), etc.).158 Hypoxia also promotes the epithelial–mesenchymal transition (EMT) that plays an important role in metastasis. These mechanisms are predominantly driven by the TME-tumor interactions, which are highly challenging to mimic in 2D models.159–162 Moreover, the oxygen-deprived environment contributes to an heightened resistance against radiation and constrains drug penetration.150,163,164 Additionally, cancer stem cells (CSCs) play a prominent role in tumor resistance and metastasis, as they can self-renew and regenerate tumor populations after treatment.150,165,166 3D models facilitate the modulation of CSCs population, which is more challenging to be modeled in standard 2D models, allowing for a better recapitulation of tumorigenesis and resistance mechanisms.167,168
In essence, 3D tumor models represent a testing platform that more closely resembles the architectural complexity, cellular interactions, environmental conditions, and underlying mechanisms that occur in tumors. By employing these models, it is possible to provide a more accurate assessment of nanomedicine penetration and efficiency, allowing to evaluate different coatings and targeting approaches. Consequently, 3D models can provide data that is not attainable in 2D and contribute to accelerate the validation of hyperthermic nanomedicines for clinical practice.
3.2 3D tumor models for preclinical screening of therapeutics
In the pursuit of better alternatives to conventional 2D monolayered models, researchers have been focusing on developing different types of 3D tumor models, with a particular emphasis on spheroids, organoids, organ-on-chip platforms, and 3D bioprinted models (Fig. 2), all of which are addressed in detail in the following section.
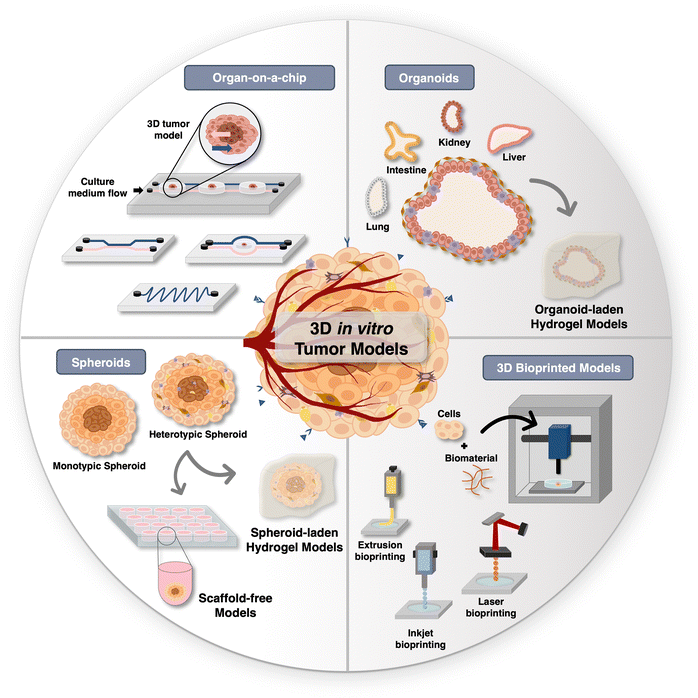 |
| Fig. 2 Overview of in vitro tumor models: Schematic of diverse 3D tumor models for hyperthermic nanomedicines screening. | |
3.2.1 Spheroids.
Spheroids are the most commonly used 3D in vitro models and consist of spheroidal, randomly self-assembled cell-rich structures.169–172 These models can be comprised of only one cell type (monotypic) or several cell types (heterotypic).173,174 Heterotypic spheroids can be bioengineered with tunable cell density/size, and have been used to screen different types of candidate therapeutics.174,175 Since heterotypic spheroids better mimic the cellular complexity of native tumors, these are considered more clinically relevant models. Heterotypic spheroids have been used to study the influence of different cell types (including stromal cells) on tumor progression, gene and protein expression patterns, to assess drug and nanoparticles penetration, as well as chemotherapeutics response.176–179 However, the generation of compact spheroids may not be achievable with all cell types, and spheroid morphology is generally dependent on the cell type and the culture conditions that are employed.24,169 Persistent challenges include standardization of spheroids production techniques and modeling vascular and immune system elements of tumors in these models.180
Spheroids generation methods can be generally categorized as scaffold-based or scaffold-free techniques. Scaffold-free techniques leverage the inherent capacity of cells to self-aggregate to form spheroidal structures and, subsequently, to potentially secrete their own ECM over time in culture. The simplicity and cost-effectiveness of these models render them highly sought-after by researchers. On the other hand, scaffold-based methods involve the incorporation of malignant and/or stromal cells within tailored hydrogels designed to mimic the ECM and to provide structural support, adhesion sites, as well as biomolecular stimulation.23,169,181
3.2.2 Organoids.
Organoids can be produced from embryonic or induced pluripotent stem cells embedded in a hydrogel matrix. Organoids resemble the heterotypic cellular composition, organization, genetic traits, and biofunctionality of the human organ of origin, being capable of self-organizing and evolving in culture over time. These models allow a co-culture of multiple cell types and can be used to model the TME and the cancer-stromal interplay.171,172,182 Organoids can be cryopreserved and used for personalized medicine, enabling researchers to explore patient-specific approaches.182–184 In the context of oncology, organoids can be derived from healthy or tumor tissues, enabling the evaluation of drugs performance in these different scenarios, under controlled conditions.182 Despite their higher correlation with the native tissue, organoids generation is generally a cumbersome and expensive process, and these models generally still lack a comprehensive representation of the vasculature, biomolecular gradients, and immune system cells present in human tumors. The widespread use of organoids encompasses several challenges particularly regarding reproducibility, standardization, cost-effectiveness, and scalability, yet they are one of the most biomimetic models of human disease available to date.182,184,185
3.2.3 Organ-on-a-chip platforms.
Organ-on-chip platforms, also termed microphysiological systems, have been widely used to recapitulate human diseases, including cancer on microfluidic chips under dynamic flow conditions.186,187 These models are generally designed to reconstitute the structural, microenvironmental, and functional complexity of human organs, as well as modulate the mechanical properties at different fluid-related shear stress, the nutrient supply, waste removal events, and the dynamic interactions occurring between cells and the flowing culture medium to recapitulate human pathophysiology.187–189 This is a relatively low-cost and reproducible methodology that enables the manipulation of parameters of the TME in the space and time domains. Additionally, some microfluidic platforms enable a real-time and quantitative assessment of tumor development and progression.27,186–188,190 Gathering on their modularity and versatility, researchers have been exploring tumor-on-a-chip models to integrate microvascular systems and their fluid dynamics. Since virtually any spheroid or organoid model can be included in microfluidics platforms, these microphysiological platforms are considered highly advanced and more biomimetic.191 Their development holds great promise for evaluating drug or nanoparticles penetration and their biological performance under dynamic conditions.191–194
From a practical perspective, organ-on-a-chip platforms are often fabricated by using poly(dimethylsiloxane) (PDMS), a biocompatible silicon-based polymer. In addition, hydrogels capable of closely mimicking ECM properties, such as Gelatin methacryloyl (GelMA), can be included in the microfluidic chambers to provide support to cancer cells/spheroids or organoids.27,195,196 Tumor-on-a-chip models have been widely used in cell biology, single-cell studies, drug discovery, genetic assays, intracellular signaling, toxicology studies, and tissue engineering.197,198 Breast,199,200 colorectal,201,202 and brain203,204 tumor-on-a-chip models have already been assembled.
3.2.4 3D bioprinting.
In recent years, 3D bioprinting has rapidly emerged as a valuable technique for generating customized and larger scale (mm up to cm scale) 3D tumor models. Extrusion bioprinting is one of the most widely used 3D bioprinting methods and involves the sequential deposition of layers of a given bioink (i.e., polymer + cells) to construct a tailored 3D tumor model that can comprise cancer and stromal cells. This technique allows a precise volumetric placement of cells, bioactive factors, and biomaterials to mimic the TME, having the ability to create geometrically complex scaffolds with low cost and reproducibility. Biomimicry of tumor heterogeneity can be achieved by printing different cell types and ECM components. Furthermore, the dimension and geometry of the scaffold can be adapted, and the underlying ECM mimetic biomaterial properties can be tuned, enabling the creation of personalized microarchitectures with biomimetic capabilities.189,205,206 Other methodologies explored to generate 3D tumor models by bioprinting include laser, droplet, or dynamic light projection (DLP).207,208 3D bioprinting techniques face significant challenges related to bioink formulation, reproducibility, and standardization, maintenance of the integrity of the printed components, preserving cell viability, and optimizing the rheological or viscoelastic properties of the biomaterial inks used for assembling the tumor models.189,207
3D bioprinting has been used to assemble spheroids, organoids, and tumor-on-a-chip models. Moreover, attempts have been made to implement vasculature in tumor models with this technique, particularly within microfluidic platforms.209,210 Adding to this, 3D bioprinting can be helpful to generate customizable 3D in vitro models with a user defined size that better mimics that of the human scale,211 which is advantageous for pre-clinical imaging techniques, such as magnetic resonance imaging (MRI), positron emission tomography (PET), or computed tomography (CT), that have limitations in sample size due to their spatial resolution.212
In addition to the discussed differences between 2D and 3D in vitro tumor models, the latter may also offer the ability to manipulate the non-cellular microenvironment through the encapsulation of cells in ECM-mimetic hydrogels. These, so-termed scaffold-based 3D in vitro models, are highly valuable to study the influence of the biophysical and biomechanical properties of the ECM, particularly through the modulation of various parameters that exist in the tumors matrix (e.g., establishment of chemical gradients, shifts in stiffness/viscoelasticity, evolving biomolecular composition, mass transfer phenomena), thus enabling to more accurately mimic in vivo conditions.213,214 Furthermore, these scaffold-based in vitro models provide the possibility to model the influence of each parameter in different stages of tumor development.213 In this framework, recent endeavors have focused on bioengineering ECM-mimetic hydrogel 3D tumor models with on-demand tailored mechanical properties to study tumor growth,215 migration/invasion,175,216,217 or metastasis.218 Adding to this, cutting-edge bioengineering methods, such as 3D bioprinting, offer the ability not only to generate in vitro models with controlled mechanical properties but also with a physiomimetic architecture of the microenvironment.214,219 In a complementary approach, the integration of 3D bioprinting with microfluidic technology has been proven to be crucial in integrating mechanical cues within 3D in vitro models, encompassing shear flow, gradients, and mechanical stimulus. These cues, in turn, can influence cellular signals, cell adhesion molecules, cytoskeleton dynamics, and activation of membrane transporters and ion channels.220,221 By manipulating these properties in 3D in vitro models, researchers can create a more realistic and tailored model to better predict cells' response to treatments.
3.3 Heat response and transfer mechanisms: probing dimensional impacts on heat response in 2D and 3D models
During stress, such as oxidative, pH, hypoxic, heat, or radiation, an overexpression of heat shock proteins (Hsp) is initiated to promote cell survival, especially involving Hsp70 and Hsp90. This includes binding to denatured proteins, preventing incorrect aggregations, assisting protein assembly, secretion, and degradation, as well as transporting those proteins through membranes.14,222 Hsp are mainly regulated by the heat shock factor 1 (Hsf1), which normally resides in the cytoplasm in an inactive state. In response to a stress situation, Hsf1 enters the nucleus and initiates the transcription process, resulting in the production of Hsp. Once the stress is removed, Hsf1 returns to its inactive state in the cytoplasm.19,223 This response enables cells to maintain their functionality, evade the signals that trigger cell death, and mitigate the effects of drugs, leading to the development of resistance to treatments.224 Consequently, researchers have directed their efforts towards discovering strategies to target Hsp with inhibitors, aiming to increase cancer cells death upon treatment.19,225 Moreover, hyperthermia has shown to promote the infiltration of immune cells in tumors, enhancing immune response and modulating the TME. This dynamic interplay can improve tumor cell recognition and destruction by the immune system.6,226
The response of cells to stress is influenced by the conditions of the culture. In 2D models, cells are simultaneously and uniformly placed under similar conditions, leading to a higher rate of cell death when compared to 3D cultures. This is primarily due to the additional architectural, cellular, and environmental components of 3D models.14,227 Such testing platforms are highly valuable to better understand and predict the performance of candidate hyperthermia treatments.
The temperature in tissues is the result of a balance of factors, including ambient temperature, heat generation as a result of metabolic activity, and heat transfer from hot to cold regions of the body by conduction and convection.228,229 It is worth noticing that convection is quite more efficient in transporting heat.230 According to a study, the size and shape of NPs, blood flow, and vessel geometry can all impact the distribution of NPs inside tumors, which in turn can affect the temperature distribution.231 Moreover, nanosized particles are more efficient in penetrating deeper into tumors but have high elimination rates.231,232 Another study demonstrated that the rate of heat generation and its impact on surrounding tissues are influenced by the infusion rate, blood flow, and distribution of nanoparticles within the tumor. A lower infusion rate was shown to be more successful in raising the concentration of NPs in the TME, causing less heat to dissipate to the surrounding tissue which can damage it.233 Heat dissipation to the surrounding tissues is another important consideration, as it can have an impact on the safety and effectiveness of hyperthermia therapy. As the volume of the tumor grows, less heat is dissipated, resulting in a greater temperature differential between the tumor and the surrounding media, allowing for the selective heating of the tumor, as more heat is carried away by perfusion on the normal tissues.234–237 Due to all the complex factors that have to be considered for hyperthermia, advanced techniques and technologies for heat transfer modeling have been developed. The heat balance of the tissues was first described by the bioheat equation proposed by Pennes’, which is now a standard model for studying the temperature distribution in tissues. This model states that the heat stored in the tissue is equal to the balance between the heat generated by metabolic activity and the heat dissipated by conduction and convection.238,239 Beyond the Pennes’ bioheat model, others have been developed, such as the local thermal equilibrium (LTE) and local thermal non-equilibrium (LTNE) equations, and dual-phase-lag bioheat model.239,240 The LTE and LTNE models consider the existence of a porous medium that consists of a solid matrix (i.e., tissue) and blood vessels that are in thermal equilibrium or non-equilibrium, respectively. The LTNE model represents a more realistic situation since it considers a temperature gradient between both phases.239–242 Pennes’, LTE, and LTNE models consider that the blood velocity is infinite (based on Fourier's law), meaning that when applying heat, the temperature of a tissue changes immediately, which does not happen in non-homogeneous tissues. The dual-phase-lag (DPL) bioheat model considers that the temperature and heat flux have a lag time. Consequently, this model focuses on the micro-structural interactions and considers that the tissues and blood can have different temperatures.239,240,243–245Table 3 summarizes the main advantages and disadvantages of each model, as well as examples of the application of these models to predict heat distribution in tumors.
Table 3 Advantages and disadvantages of the bioheat models used to predict heat distribution in tumors, compiled from references239–245,258,259
Model |
Description |
Advantages |
Disadvantages |
Application of the model to predict heat distribution in tumors |
Pennes’ bioheat equation |
The heat stored in the tissue is equal to the balance between the heat generated by metabolic activity and the heat dissipated by conduction and convection |
Simplicity; consideration of blood perfusion |
Assumes a homogeneous and isotropic tissue; constant blood perfusion; restricted spatial variability; do not consider the vascular geometry |
247, 254, 255 and 257
|
Local thermal equilibrium equation |
Considers the existence of a porous media that consists on a solid matrix (i.e., tissue) and blood vessels that are in thermal equilibrium |
Simplicity; can consider blood flow direction |
Assumes that the temperature of the blood and tissue is the same; it can only be applied in scenarios with limited vessels diameter and blood velocities |
260
|
Local thermal non-equilibrium equation |
Considers the existence of a porous media that consists of a solid matrix (i.e., tissue) and blood vessels that are in thermal non-equilibrium |
Consider the temperature of tissues and blood; can consider blood flow direction; improved model compared to the one-equation model |
Complexity; detailed information about thermal properties of tissues is required |
247 and 260–262
|
Dual-phase-lag bioheat equation |
Considers that the there is a lag time in the temperature and heat flux |
Consider the temperature of tissues and blood; consider micro-structural interactions effects |
Complexity; detailed information about thermal properties of tissues is required |
263–266
|
When predicting hyperthermia effects in 3D in vitro tumor models, the choice of the bioheat equation depends on the specific characteristics of the tissue. For instance, a study concluded that the DPL model can better predict heat transfer in tissues with larger blood diameters, but when considering tissues with micro-capillaries, the Pennes’ model and the DPL model had similar performances.246 In another study, the performance of the LTNE model with variable porosity and the Pennes’ bioheat model with variable perfusion was compared when simulating thermal ablation in tumor tissue, and it was shown that the LTNE model was more accurate in predicting heat distribution after comparing the results with in vivo experiments.247 Although, due to its simplicity, the Pennes’ bioheat model has been the most used to study heat transfer in biological tissues.248 In a balance between simplicity and accuracy, the Pennes’ bioheat equation has undergone some modifications over the years, aiming to predict heat distribution in tumors as close as possible to an in vivo situation. Different parameters, such as the direction of the blood flux, spatial and temporal variations of the velocity of the blood, size, geometry, and density of the vasculature, and the distribution of nanomedicines, have been considered.231,249–253Fig. 3 shows the use of advanced tumor models to better understand heat distribution, particularly by varying the density of tumor vasculature (Fig. 3A1 and A2),254 and by using Fe3O4 MNPs for MH (Fig. 3B1–B4).255 Even though recent studies have been providing important information, it is difficult to resemble a perfect in vivo situation due to the complex and unpredicted variables, such as tumor size or the geometry and density of the vasculature, that have a huge influence on how the NPs and heat are distributed. Moreover, it is difficult to confirm the veracity of the obtained results due to the lack of experiments performed in clinical settings.254 Currently, researchers are dedicated to improving mechanisms to predict heat delivery in tissues by introducing variables such as the size and morphology of the NPs.231 Significant advances in this direction are expected in the upcoming years, especially considering that 3D hyperthermic simulations are an important tool to accelerate the clinical translation of nano-hyperthermia.
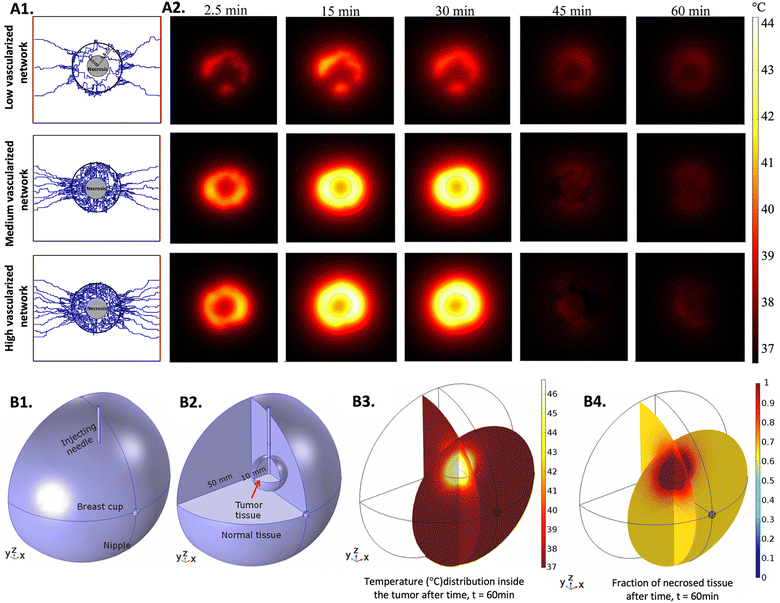 |
| Fig. 3 Simulations of heat distribution in 3D tumor models: (A1) three capillary models with low, medium, and high vascularization used to perform the numerical studies, (A2) computational estimation of temperature distribution for three different tumor vasculature densities at five time points. The MNPs were injected at constant rate for 30 minutes. Reprinted from ref. 254, Copyright (2022), with permission from Elsevier and (B1) designed model representing the breast cup with the injecting needle, (B2) designed model enhancing the tumor and the normal tissue, (B3) 3D simulation of the temperature distribution in the breast cup and (B4) 3D simulation of the necrotic tissue. Reprinted from ref. 255, Copyright (2020), with permission from Elsevier. | |
Numerical simulation methods are a valuable resource for hyperthermia treatment planning. These methods can help to predict how the chosen treatment for the specific size and location of the tumor can affect both tumor and healthy tissue, and to adapt the heat and heating agents’ doses needed for personalized treatment. Moreover, by comparing the outcomes of these methods with the ones obtained from 3D in vitro models, it is possible to validate these theoretical techniques for modulating the tumor response to hyperthermia. These improvements, combined with the increasing computational power and the advent of artificial intelligence (AI) algorithms for simulation, are envisioned to become a major part of the preclinical optimization/validation process of hyperthermia therapies and of the research to predict their effect on healthy tissues.239,256,257
4 Advances in preclinical screening of hyperthermic nanomedicines in 3D tumor models
The development of 3D tumor models has revolutionized the field of cancer research and encouraged their application for the screening of hyperthermic nanomedicines.14 By using these models, it is expected to have a deeper understanding of the interactions between hyperthermic nanomedicines and cancer cells, as well as the influence of the TME on drug delivery, nanomedicines distribution in the tumor, and therapeutic response. With a clear understanding of these mechanisms, researchers can optimize treatment strategies and develop more clinically relevant hyperthermic approaches for cancer therapy.14,267,268
This chapter aims to provide an overview of the recent advances in the preclinical screening of hyperthermic nanomedicines using 3D tumor models, with a prominent role in PTT, MH, and hyperthermia combined with conventional therapies. By examining the cutting-edge research in this field, it is expected to provide valuable insight into the potential of 3D tumor models as powerful tools for preclinical screening, allowing the identification of hyperthermic nanomedicines with optimal therapeutic efficacy and safety profiles.
4.1 3D tumor models for photothermal nanomedicines screening
The emergence of 3D in vitro tumor models represents a relatively recent development, and as such, numerous studies are still dedicated to understanding the differences in the hyperthermic response between 2D and 3D models. Comparative studies evaluating the efficacy of hyperthermic nanomedicines within monolayers and tumor spheroids revealed that 3D models are more resistant to the treatment.269,270 Moreover, an additional study showed that 2D monolayers needed less NPs incubation time and had more efficiency in binding the NPs to cells than 3D models, enhancing the importance of replacing 2D with 3D models for treatment parameters optimization, aiming to obtain more relevant outcomes.271 More complex models using microfluidic platforms to grow spheroids have been focused on understanding the penetration, distribution, and effectiveness of hyperthermic nanomedicines through more complex 3D models. As an example, a microfluid system consisting of PDMS was used to grow multicellular tumor spheroids to evaluate the PTT performance of hollow Au nanoshells modified with an anti-MUC1 aptamer (HGNs@anti-MUC1) (Fig. 4A1–A3).272 Human lung epithelial carcinoma cells (A549) and human breast adenocarcinoma cells (MCF-7) were used to assemble the tumor spheroids. The tested nano-PTA showed to be efficient in reducing the viability and size of tumor spheroids after irradiation, which was more pronounced with a double dose of irradiation with a 1 hour interval from the first one (Fig. 4A4). The study also tested the nano-PTA in spheroids of non-tumorous cell lines, and besides proving that the internalization of the nanomedicine was less effective compared to tumor spheroids, it was also observed that under the same treatment conditions, non-tumorous spheroids exhibited less pronounced effects, assuming the efficiency of HGNs@anti-MUC1 to specifically treat tumors. Furthermore, spheroids from different cell lines had different treatment outcomes, reinforcing the fact that the response to hyperthermia is tissue-dependent.272
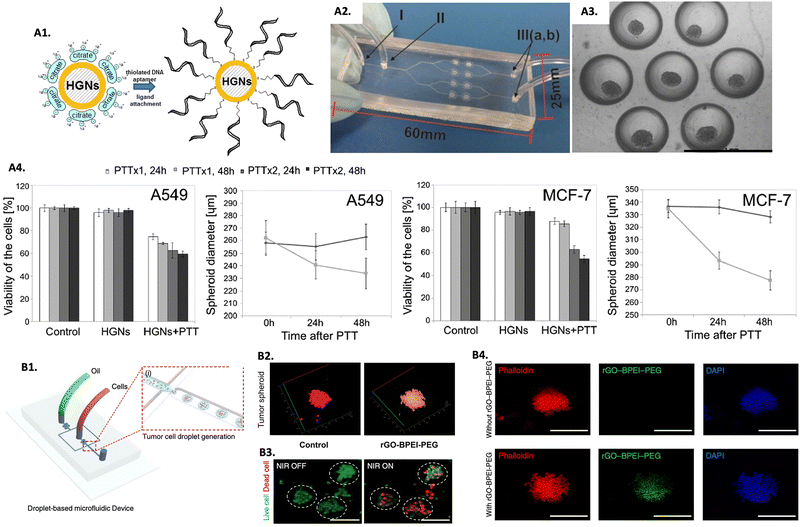 |
| Fig. 4 Screening PTT nanomedicines in 3D in vitro tumor models for PTT: (A1) scheme showing the modification of HGNs with the anti-MUC1 aptamer, (A2) microfluidic system used to implement the spheroids (I, III – inlets/outlets and II – vent hole), (A3) image of a chamber of the microsystem containing spheroids, and (A4) spheroids viability and diameter submitted to one (PTTx1) and two (PTTx2) NIR doses, after 24 and 48 hours. Reprinted with permission from ref. 272, Copyright (2019), with permission from Elsevier; (B1) scheme of the generation of 3D tumor spheroids using the drop-based microfluidic device, (B2) Z-stack confocal laser scanning microscopy image of 3D tumor spheroids treated with rGO-BPEI-PEG (scale bar = 100 μm), (B3) live/dead images of brain tumor spheroids before and after PTT (scale bar = 100 μm), and (B4) fluorescence microscopy images of brain tumor spheroids with and without rGO-BPEI-PEG internalized, reprinted with permission from ref. 274, Creative Commons (CC) License 4.0 (https://creativecommons.org/licenses/by/4.0/). | |
Treating brain tumors presents significant challenges as it involves the risk of damaging surrounding healthy tissue, which can lead to serious complications and low survival rates. The non-invasive nature of hyperthermia, its potential for selective tumor treatment, and the challenges posed by the complexity of the brain TME and the blood–brain barrier have encouraged the exploration of this therapy in 3D brain tumor spheroids.34,273 As an example, U87MG glioblastoma cells spheroids generated in a PDMS microfluidic device (Fig. 4B1) were used as a screening platform to evaluate a reduced graphene oxide-branched polyethyleneimine-polyethylene glycol (rGO-BPEI-PEG) nanocomposite for PTT.274 After confirming the efficient uptake of the nano-PTAs in the brain tumor spheroids (Fig. 4B2 and B4), a significant decrease in cell viability was observed after PTT (Fig. 4B3).274 In another study, macrophages were used as vehicles to transport gold–silica nanoshells (AuNS) and gold nanorods (AuNR) to human glioma (ACBT) spheroids.275 A better uptake was observed with the AuNR due to their shape and smaller size, however, the AuNS presented more efficiency than the AuNR in reducing the spheroids volume after PTT, a difference that increased with the increase in intensity of irradiation. The authors explained these findings by the larger cross-sectional area of the AuNS that resulted in greater efficiency in transforming light into heat.275
A recent study using 4T1 tumorspheres showed that cypate (Cy)-loaded hyaluronic acid (HA)-black phosphorus nanosheets (Cy@HBPN) were able to significantly suppress the growth of the 3D models and inhibit their regenerative potential.276 After proving the in vitro potential, a mouse xenograft tumor model administered with Cy@HBPN showed to completely inhibit tumor growth after laser irradiation, validating the in vitro results.276 In another study, organoids were explored as testing platforms to access black phosphorus quantum dots into exome vector nanospheres (BEs) hyperthermic efficiency.277 BEs were able to internalize cells, inhibit tumor progression, and suppress angiogenesis. Further in vivo assessment in a nude mouse model bearing a subcutaneous bladder tumor confirmed the ability of BEs to inhibit tumor growth and recurrence, revealing BEs good photothermal and targeting capability.277
Beyond demonstrating the feasibility of using PTT to replace traditional methods by being suited to perform treatment in tumors localized in deeper and sensitive parts of the body, such as the brain, the reported studies showed that 3D in vitro platforms are important tools to predict the ability of nano-PTAs on penetrating and accumulating in the tumor, to understand what NPs physicochemical properties can be modulated (e.g., surface, size, shape modifications, or targeting ability) to reach maximum retention of the agents at the tumor site and improve the outcome of the treatment. Furthermore, 3D platforms can enable the optimization of treatment parameters, such as the intensity or duration of laser exposure, depending on the type of tissue, location of the tumor, and TME, aiming to guarantee the safety of the healthy tissue around the tumor.
4.2 3D tumor models for magnetic hyperthermia nanomedicines screening
MH allows a high penetration depth and selectivity, which has many benefits when the tumor is localized in sensitive tissues, such as the brain, or more deeply in the body.87 Ongoing research and development efforts are focused on optimizing MNPs’ design and targeting strategies. As mentioned before, iron oxide MNPs have been the most explored for MH, which is why they are the most used MNPs for hyperthermia screening in 3D tumor models. To explore the influence that the location, amount, and heterogeneity of iron oxide MNPs have on MH, a study was carried out to evaluate their performance for MH in 3D cell culture gels based on collagen of a murine macrophage RAW-264.7 cell line.278 MNPs distribution within the model was manipulated, namely: (i) the In Model had MNPs homogeneously localized only inside the cells, while (ii) the In&Out model had MNPs heterogeneously localized inside and outside the cells (Fig. 5A1 and A2). Results showed that AMF exposure promoted the uptake of MNPs, that could be related to an increase in collagen permeability. Furthermore, it was shown that the cell death mechanisms triggered after treatment were dependent on the iron concentration inside the cells. Additionally, the heterogeneous distribution of particles in the In&Out setup was shown to decelerate the rate of cell death (Fig. 5A3).278 These findings are extremely important since it was shown that the distribution and concentration of MNPs in cells influence the treatment, something accessible only using 3D models.
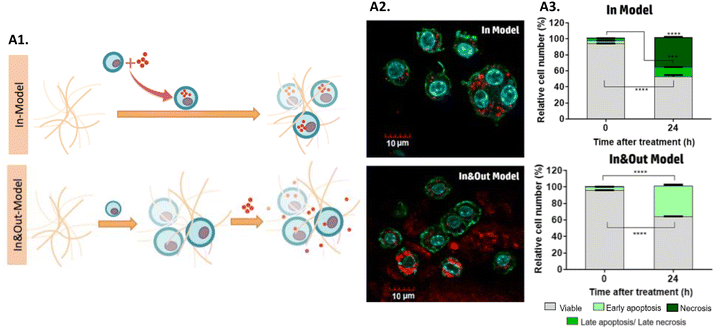 |
| Fig. 5 Screening MH nanomedicines in 3D in vitro tumor models: (A1) scheme of the two models used in this work: in and In&Out models; (A2) confocal images evaluating the distribution of MNPs in both models, and (A3) cell death mechanisms for both models studied at 0 and 24 hours after the treatment. Reprinted with permission from ref. 278, Copyright 2018 American Chemical Society. | |
Several studies have also focused on assessing the effectiveness of MNPs for MH, comparing the outcomes of 2D and 3D screening in vitro platforms. As an example, SPIONs coated with triarylphosphonium cation (TPP), a mitochondrial-targeting molecule, were tested in liver tumor spheroids with HepG2 cells and 3T3 fibroblasts, and HepG2 monolayer cultures.279 Coating SPIONs with TPP allowed them to successfully target the mitochondria, subsequently inhibiting its function and inducing cell death. The uptake of the NPs in the spheroids was lower than in the monolayers. In fact, the authors estimated that a mature spheroid only contained ∼40% of cells in contact with MNPs, which resulted in significant cell death at the proliferative layer of the spheroid. Additionally, 3D spheroids were shown to be more resistant to treatment compared to 2D models, and cancer cells were found to be more sensitive to heat than healthy cells when exposed to the same heating conditions.279 Recently, other approach explored MNPs screening in patient-derived organoids.280 In this approach, pancreatic ductal adenocarcinoma (PDAC) human-derived organoids were incubated with SPIONs stabilized with a phospholipid-bilayer and used to understand how these nanomedicines penetrated into the 3D model. It was observed that the MNPs were unable to be internalized, being only located in the ECM surrounding the organoid. Furthermore, following MH treatment, organoids viability was significantly lower at 2 hours, when compared to that obtained at 24 hours post-treatment. The authors hypothesized that this behavior could be related to a short-term cytotoxicity caused by the treatment in this type of tumor model. This study also showed how 2D models can be inaccurate in predicting tumor responses and that different cell lines from PDAC had different responses to MH treatment.280
Apart from demonstrating the efficiency of MH in eradicating cancer cells within 3D cultures, the presented studies for MH screening in 3D tumor models highlight the potential for improved and targeted outcomes when coupling targeting agents with MNPs. The mechanisms of cell death triggered can vary based on the concentration of iron present in cells,278 and can also depend on the properties of the MNPs, frequency, intensity of the AMF, and type of tissue.118 Furthermore, the location of the MNPs within the 3D tumor models was shown to influence the efficiency of the treatment. Overall, 3D tumor models showed to be suitable platforms to understand the diffusion mechanisms, penetration, and efficiency of different nanomedicines for MH in different treatment conditions and tissues.
4.3 3D tumor models for screening synergistic effects of hyperthermic nanomedicines
Hyperthermia exhibits a prominent advantage in its potential to synergistically enhance treatment efficacy when combined with traditional cancer treatments, such as chemotherapy and radiotherapy.281 Following thermal exposure,blood flow and vascular permeability increase, leading to improved drug uptake and distribution, and increased cellular stress. Moreover, the interstitial fluid pressure decreases and the mechanisms to repair DNA are inhibited, which prevents irradiated cells from being repaired. Because of this, cancer cells become more susceptible to radiation and chemotherapy after heat treatment.282–284 Ongoing research with 3D in vitro models is being held to investigate the synergistic effects of hyperthermia when combined with traditional treatments, aiming to find optimal protocols and strategies for integrating heat therapies into multidisciplinary cancer treatment approaches. In combinational PTT and chemotherapy (PTT-CHT), nano-agents can serve as multifunctional platforms. Besides being selective heating sources, they can also function as carriers for chemotherapeutic drugs, enabling controlled and localized drug delivery to the tumor site. The heat generated by the nano-heating agents can enhance drug release from the carriers, improving drug penetration into the tumor and increasing therapeutic efficacy.285,286 A study reported the use of gold nanoroses (AuNs) loaded with doxorubicin (DOX), ICG, and a naive chimeric peptide B-anti G (AuNDIPs) for glioma targeting in C6 glioma cells and 3D spheroids.287 AuNDIPs were efficiently uptaken both in 2D and 3D models, which was facilitated by the peptide B-anti G. After laser exposure, the authors observed maximum cell death in spheroids treated with AuNDIPs when compared to spheroids treated only with AuNs or a DOX-ICG and peptide Mix solution (Fig. 6A1), which was due to the combinational effect of the DOX, ICG, and the peptide.287 In another study, researchers developed gold nanospheres within silica nanocapsules (aAuYSs) to perform PTT-CHT in A2780 ovarian cancer spheroids.288 It was shown that only the combination of NIR radiation and aAuYSs significantly reduced the viability of the spheroids (Fig. 6B1). Furthermore, a DOX-resistant cell line (A2780-R) was used to evaluate the synergistic effect of aAuYSs combined with DOX and proved that the number of dead cells was more significant when performing both treatments. This was also confirmed in an in vivo xenograft tumor model since only the combination of treatments was capable of inhibiting tumor growth (Fig. 6B2).288
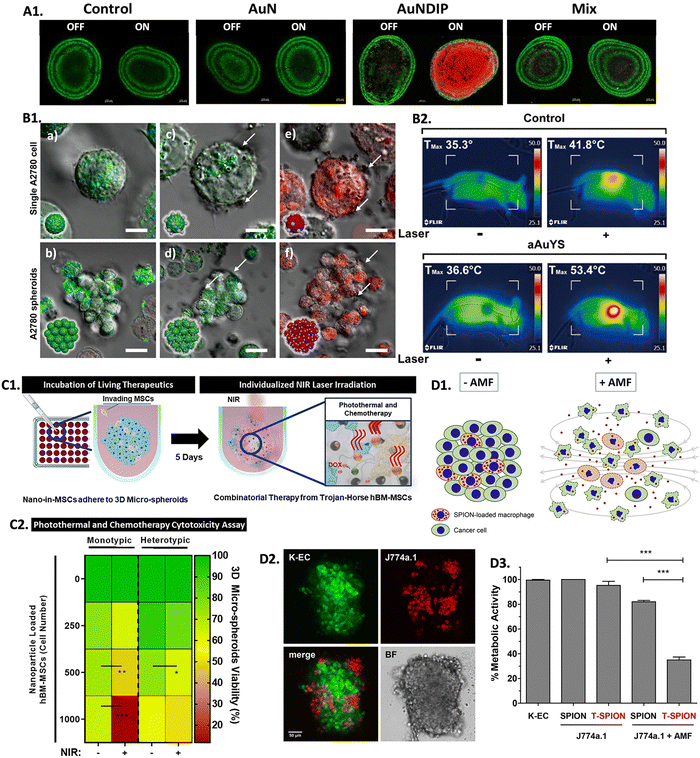 |
| Fig. 6 Screening combinational hyperthermia and chemotherapy in 3D in vitro tumor models: (A1) live/dead confocal images of control untreated C6 spheroids, and spheroids treated with AuN, AuNDIP, and Mix (DOX-ICG and B-anti G)), with and without laser exposure (scale bar = 100 μm). Reprinted from ref. 287, Copyright (2022), with permission from Elsevier. (B1) Confocal images of A2780 cells and spheroids: (a) and (b) non-treated with aAuYs under laser irradiation; (c) and (d) treated with aAuYS without laser irradiation, and (e) and (f) treated with aAuYS under laser irradiation (scale bars in the upper and lower panels represent 6 and 20 μm, respectively), and (B2) infrared thermal images of a mice treated with DOX and aAuYS submitted to NIR laser, Reprinted with permission from ref. 288. Copyright 2021 American Chemical Society; (C1) scheme representing the performed procedure of hBM-MSCs NPs delivery in 3D spheroids followed by laser irradiation; (C2) heat map enhancing the influence of the number of MSCs loaded with PDA-ICG-DOX NPs in 3D spheroids viability, 3 days after NIR laser treatment (irradiation (NIR +), and without irradiation (NIR −)). Reprinted with permission from ref. 174, Copyright (2021), with permission from Elsevier; (D1) scheme representing the incorporation of macrophage loaded with SPIONs into cancer cells and its effect when submitted to an AMF; (D2) confocal microscopy showing the integration of J774a.1 macrophages (red) in K-EC spheroids (green) (scale bar = 50 μm); and (D3) metabolic activity of re-cultured spheroids containing T-SPION loaded macrophages with or without AMF application after 48 h of treatment. Reprinted with permission from ref. 292, Copyright (2019), with permission from Elsevier. | |
Mesenchymal stem cells (MSCs) have been explored as drug vehicles due to their tropism (i.e., the ability to travel to damaged tissues, such as tumors), which has already been demonstrated in several studies.289,290 Moreover, it was also demonstrated that hyperthermia can improve the ability of stem cells to penetrate tumors, making them a suitable platform to transport drugs and NPs to tumors to promote localized hyperthermia therapy.291 In this scope, a study used monotypic (only one cell type) and heterotypic (multiple co-cultured cell types) 3D breast cancer spheroids to evaluate the potential of human bone-marrow-derived MSCs (hBM-MSCs) as nanocarriers of polydopamine NPs dual-loaded with ICG and DOX (PDA-ICG-DOX) and to evaluate their efficiency for PTT-CHT (Fig. 6C1).174 The authors observed that the MSCs were able to adhere to the tumor models efficiently. The combination of PTT and chemotherapy led to a decrease in spheroids viability, which was more pronounced with an increased number of PDA-ICG-DOX NPs loaded MSCs (Fig. 6C2). It was concluded that the heterotypic spheroids were more resistant to treatment than the monotypic spheroids and that the use of MSCs as nanocarriers was more efficient in reducing the viability of tumors than the introduction of free nanomedicines in the 3D tumor models.174 These findings are important for understanding how a heterotypic environment can confer more resistance to treatment, mimicking a more in vivo-like scenario.
Recently, a study intending to understand the feasibility of macrophages in transporting SPIONs coupled with a toxin (T-SPIONs) to KSHV-infected human endothelial cells (K-EC) spheroids for a combination of MH and chemotherapy (MH-CHT) was carried out (Fig. 6D1 and D2).292 Even without being submitted to an AMF, after 48 hours in culture, spheroids containing T-SPIONs presented a significant loss of structure, whereas spheroids with only SPIONs or without SPIONs did not show any significant structural change. The spheroid's structure was even more compromised after treatment with AMF, being more evident when the toxin was coupled to the SPIONs, accompanied by a decrease in the metabolic activity of spheroids (Fig. 6D3).292
Hyperthermia before radiotherapy was shown to increase the efficiency of the treatment since heat can selectively target and sensitize cancer cells, making them more vulnerable to radiation-induced DNA damage and cell death. Consequently, hyperthermia can improve tumor response, overcome radioresistance, and potentially reduce the side effectsassociated with high radiation doses.293 In an elegant approach, copper sulphide NPs (CuS NPs) were associated at the surface of upconversion NPs (UCNPs) due to manganese dioxide (MnO2) coating, forming multifunctional nanoplatforms (UCCM).294 Formulated UCCMs were internalized in mouse colon (CT26) spheroids to evaluate internalization in a solid tumor model. It was observed that after 4 hours, CuS NPs were at the center of the spheroid. This allowed MnO2 to release Mn2+ and oxygen, consequently reducing hypoxia and resistance to radiation. Furthermore, the released Mn2+ was shown to be a proper contrast agent for MRI, allowing real-time control of the tumor site that can be helpful for treatment guidance. The therapeutic effect of the UCCM NPs was then evaluated in mice bearing a human liver tumor, which revealed that the tumors shrank compared to non-treated tumors and that the normal tissues were minimally affected. Moreover, the combination of the treatments was far more effective in destroying the cancer cells than the treatments alone.294
Studies using 3D in vitro models for screening nanomedicines for a combination of hyperthermia and radiotherapy are still a novel approach, thus accounting for the lack of literature reports on this subject. Recent studies continue to rely on standard 2D in vitro cultures,296–299 which is also important, but there is a need to embrace novel and innovative in vitro testing platforms that can more closely recapitulate human tissues response to treatments and ultimately contribute to understanding the underlying mechanisms that contribute to the observed synergistic effects that can accelerate translation to clinical practice. Table 4 showcases studies reporting the application of 3D tumor models as screening platforms to evaluate nano-heating agents for hyperthermia as a stand-alone or combinatory treatment. The examples reported in this section have provided valuable insights into the synergistic interactions between hyperthermia and traditional cancer treatments. This approach allows improved drug penetration, enhanced cancer cell killing, modulation of drug resistance mechanisms, and reduction of the radiation dose and/or chemotherapeutic agents, which is very important to guarantee the safety of healthy tissues. Optimizing treatment parameters, such as exposure time, and nanomedicine or radiation doses, remains crucial. Alongside, recent advances in the combination of hyperthermia with modern and more sophisticated therapies, such as immunotherapy, have been shown to provide better immune recognition and destruction of the tumor since the heat induced by hyperthermia can activate and boost immune cells’ anti- tumoral activity.125
Table 4 Examples screening the effectiveness of 3D tumor models in predicting the efficiency of nano-heating agents for human and hyperthermia
Heating agent |
Heating efficiencyc |
Technique, heating conditions |
Tumor model |
Dose (μg mL−1) |
T reached during treatment (°C) |
Cell death (%) (time after treatment, technique) |
Ref. |
Core |
Coating |
Size w/coating (nm) |
Value extracted from graphs.
Fe concentration.
The conditions that are not indicated are the same as the heating conditions; N.D. – non described; MD – microfluidic device; DOPA: dopamine; rGO: reduced graphene oxide; BPEI: branched polyethyleneimine; AFM: atomic force microscopy; HA: hyaluronic acid; PMAO: poly(maleic anhydride-alt-1-octadecene; TPP: triphenylphosphonium cation; PDAC: pancreatic ductal adenocarcinoma; PDA: polydopamine; hBM-MSCs: human bone-marrow derived mesenchymal stem cells; BCAFs: breast cancer-associated fibroblasts.
|
DOPA-rGO |
Thiol-terminated poly(2-ethyl-2-oxazoline) |
100–200, DLS |
PCE N.D. |
PTT (808 nm, 1.7 W cm−2, 5 min) |
MCF-7 monolayers and spheroids |
75 |
N.D. |
2D model: 97% (24 h, AB) |
270
|
3D model: 70% (48 h, AB) |
Au nanoshells |
Anti-MUC1 aptamer |
N.D. |
PCE N.D. |
PTT, 808 nm, 5 min, single (PTTx1) or double irradiation (PTTx2), 1 h interval |
A549 and MCF-7 spheroids in MD |
100 μM |
N.D. |
A549: 31% (PTTx1) and 41% (PTTx2) |
272
|
MCF-7: 15%a (PTTx1) and 50%a (PTTx2) (48 h, AB) |
rGO |
BPEI–PEG |
50–60, AFM |
PCE N.D. |
PTT, 808 nm, 1 W cm−2, 10 min |
U87MG spheroids in MD |
60 |
N.D. |
∼45% (after treatment, live/dead) |
274
|
Black phosohorus nanosheets |
HA coating with loaded cypate |
187, DLS |
PCE ∼ 49% (at 0.5 W cm−2) |
PTT, 808 nm, 1 W cm−2, 3 min |
4T1 monolayers and spheroids |
25 |
N.D. |
2D model: 60% (24 h, MTT) |
276
|
3D model: ∼75% (7 days, FC) |
Fe3O4 NPs |
PMAO functiona-lized with glucose |
48–67, DLS |
SAR = 253 W gFe−1 (103 μgFe mL−1, 20 kA m−1, 829 kHz) |
MH, 13 kA m−1, 377.5 kHz, 30 min |
ATCC TIB71 culture gels |
200 |
N.D. |
In model: ∼40% |
278
|
In & out model: 38% (24 h, FC) |
∼96%, both models (48 h, FC) |
Fe3O4 NPs |
Aminosilane |
100, N.D. |
SAR ∼ 115 W g−1 (104 μgFe mL−1) |
MH, 300 Gauss, 305 kHz, 10 and 30 min |
C6 spheroids in MD |
104 b |
41–43 |
10 min: 20% |
295
|
30 min: 100% (after treatment, live/dead) |
Fe3O4 NPs |
TPP |
20, N.D. |
SAR = 619 W g−1 (340 μg mL−1) |
MH, 30 A, 300 kHz, 10 min |
HepG2 monolayers; 3T3 and HepG2 spheroids |
50b |
N.D. |
2D model: ∼50%a |
279
|
3D model: ∼20%a (after treatment, trypan blue) |
SPIONs |
Phospholipid bilayer |
100, DLS |
SAR = 406 W gFe−1 |
MH, 40–47 kA m−1 |
PANC-1 monolayers and PDAC organoids |
225b |
N.D. |
2D model: 27% |
280
|
270 kHz, 30 min |
3D model: 48% and 13% (2 h, 24 h, CellTiter-Glo®) |
Gold nanoroses (AuNs) loaded with DOX and ICG |
Peptide B-anti G |
295, (just AuNs) DLS |
N.D. |
PTT-CHT, 808 nm, 2 W cm−2, 10 min |
C6 monolayers and spheroids |
100 |
N.D. |
2D model: ∼85% |
287
|
3D model: ∼88% (24 h, MTT) |
PDA loaded with DOX and ICG NPs |
hBM-MSCs |
328, DLS |
PCE ∼ 90% |
PTT-CHT, 808 nm, 1.6 W cm−2, 5 min |
Monotypic (MDA-MB-231) and heterotypic (MDA-MB-231 and BCAFs) spheroids |
500 |
N.D. |
Monotypic spheroids: ∼60%a |
174
|
Heterotypic spheroids: ∼40%a (3 days, CellTiter-Glo®) |
SPIONs coupled with a toxin |
Silica coating; loaded in mouse macro-phages (J774a.1 cells) |
N.D. |
N.D. |
MH-CHT, 4.8 kA m−1, 779 kHz, 40 min |
K-EC spheroids |
30 μg/105 cells |
N.D. |
∼65%a (48 h, WST-1) |
292
|
Besides being used to predict the efficiency of the treatment, 3D in vitro models can be useful platforms to assist the design process of the nano-agents for parameters such as size, morphology, coating, stabilization, and targeting optimization, in a specific and relevant tumor context. A study using colorectal cancer spheroids was carried out to understand how the internalization of NPs was affected by their size, surface, and bulk characteristics.300 The authors considered poly(styrene) NPs with different sizes and different coatings and concluded that unmodified NPs with smaller sizes had more efficiency in accumulating at the core of the spheroids. Regarding surface charge, it was shown that better penetration ability was achieved with positively charged NPs, due to the presence of negatively charged elements in the ECM that can repel negative charges. Moreover, it was discussed that lower molecular weight NPs have greater efficiency in penetrating tumors.300 In another study, the influence of the shape of the NPs on penetrating 3D HeLa tumor spheroids was assessed.301 Sphere, long rod, and short rod-shaped NPs were studied, and the uptake efficiency in spheroids was shown to be strongly dependent on the length-to-width ratio. The authors concluded that NPs with higher length-to-width ratios had more difficulty penetrating tumors than those with lower length-to-width ratios. Additionally, the uptake efficiency was different when considering 3D tumor spheroids and monolayers.301 The presented studies emphasize the importance of considering the physicochemical properties of NPs during the design phase, aiming to optimize their hyperthermic efficiency.
5. Conclusions and future challenges
With the developments achieved in the last few years, organotypic 3D models have emerged as feasible biomimetic platforms, capable of replacing the standard 2D models that continue to be widely explored for screening hyperthermic nanomedicines. These advanced biomimetic models enable a closer examination of the effects induced by heat within tumors, taking into consideration factors such as tri-dimensionality, size, composition of the TME, availability of nutrients and oxygen, intercellular signaling, and nanomedicines properties. The tissue-specific characteristics of 3D tumor models that allow the incorporation of several ECM components and other cell types can help in the design of cell-specific cancer platforms to optimize treatment outcomes. Due to the inherent unpredictability, complexity, and specificity of tumors for each patient, this approach allows for personalized treatment models that offer improved accessibility and predictive capabilities in accessing the response to hyperthermia therapies. Despite these improvements at the preclinical stage, the protocols of hyperthermic procedures must be standardized, as well as the characterization techniques.302,303 As discussed above (Tables 1, 2 and 4), highly relevant information regarding treatment procedures is lacking from literature reports, which may impede the establishment of standardized protocols.
It is pertinent to highlight the importance of considering the design of more complex cellular 3D in vitro structures (i.e., regarding ECM components, cellular types, incorporation of vascularization and diffusion mechanisms, etc.) in the future, aiming to provide more realistic screening platforms. As it was understood by the reported studies, spheroids are the predominant model as they are the most simple and cost-effective testing platforms. Certainly, organoids have gained increasing attention recently, offering more complexity and a more in vivo-predictive response to treatments. This development aims to facilitate the translation of hyperthermic nanomedicines into clinical practice. Furthermore, combining spheroids or organoids with tumor-on-a-chip platforms and 3D bioprinting techniques can increase the complexity of the models, as these allow to introduce components of the tumor vasculature and flow dynamics/mechanisms. Also, some studies reported here provided both 3D in vitro and in vivo assessment of the hyperthermic nanomedicines, which enables to confirm that these models can provide closer in vivo responses and consequently contribute to accelerating hyperthermic nanotherapies preclinical validation and subsequent transition to clinical practice.
Even though hyperthermia demonstrates notable efficacy in tumors, its widespread clinical implementation is being delayed by prominent limitations, including inadequate penetration and heterogeneous distribution of heating agents in tumors, and insufficient control over temperature regulation at the tumor site. As shown and discussed herein, researchers have been focused on studying different carriers and targeting agents capable of improving the internalization of heating agents in the tumor, which have been shown to provide a more localized treatment with better therapeutic outcomes. Approaches that involve focalized delivery are also envisioned to be further explored in the future.
Regarding temperature control, there is a need to have a reliable method for real-time assessment, thereby mitigating damage to healthy tissues around the tumor mass. However, invasive devices (e.g., optical fibers and thermocouples) currently represent the prevailing method for temperature control in hyperthermia procedures.304 As noted in the examples documented in Table 4, a substantial number of studies did not present the temperature that the 3D models reached during the treatment, which can be due to the difficulty in controlling the temperature in real-time. Currently, non-invasive thermometry systems based on MRI,305,306 magnetic particle imaging (MPI),307 or fluorescence thermometry308,309 are being developed, representing an outstanding breakthrough in the field by allowing temperature control in real-time and in a minimally invasive way. However, 3D tumor models are challenging tools when it comes to obtaining temperature maps with adequate spatial resolution and for imaging. With increasing tumor size, light has less penetration ability, as it gets more scattered. Consequently, the ability to acquire temperature maps or high-resolution images declines, particularly considering deeper regions within the tumor. Furthermore, larger tumor sizes can be a barrier to prompt acquisition of images, thus compromising the real-time control of the heat therapy.23,304,310 To address these limitations, researchers have been exploring strategies to confer nanomedicines with theranostic capabilities, i.e., the ability to perform an image-guided therapy.311–313 By using nanomedicines for temperature control, the problem of spatial resolution can be addressed, but imaging techniques capable of capturing such fine-scale response are needed.310 MRI has a spatial resolution in the order of the millimeters,314 which can be crucial to provide high-resolution temperature maps, as the ones obtained in.315,316 By tuning the transition temperature of MNPs to room temperature, it is possible to use those nanomaterials as temperature sensors in the range of hyperthermia.317 Consequently, these agents can be simultaneously used as contrast agents and thermometers for MRI during hyperthermia treatments. However, MRI-based thermometry still faces challenges with low signal-to-noise ratio and inaccurate temperature calibrations.318
Integrating advancements in 3D in vitro tumor models with emerging hyperthermia techniques and non-invasive 3D thermometry systems could mitigate the disparities in readouts between preclinical and clinical trials for screening advanced anti-cancer therapeutics (Fig. 7). This convergence of technologies holds promise for enhancing the translational relevance of treatments, foreseeably facilitating their widespread use in clinical practice.
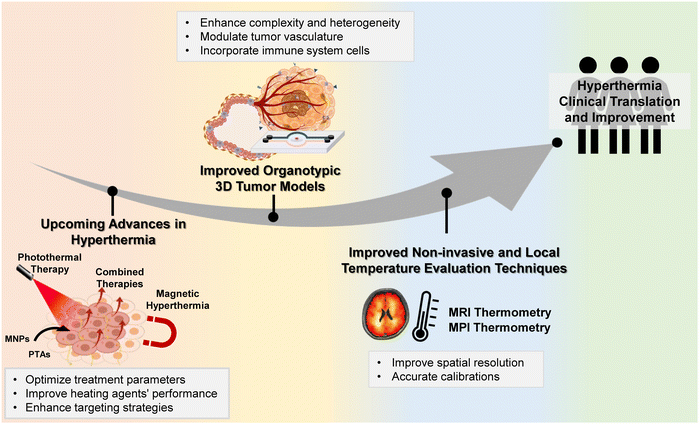 |
| Fig. 7 Developments in hyperthermia for clinical translation: progressive advancements of hyperthermia treatments, 3D tumor models, and non-invasive thermometry techniques that can accelerate the translation of hyperthermia to clinical practice. | |
Conflicts of interest
The authors declare no competing interests.
Acknowledgements
This research was funded by project CICECO – Aveiro Institute of Materials, UIDB/50011/2020 & UIDP/50011/2020, financed by national funds through the Portuguese Foundation for Science and Technology/MCTES. This work was also supported by the Programa Operacional Competitividade e Internacionalização (POCI), in the component FEDER, and by national funds (OE) through FCT/MCTES, in the scope of the projects PANGEIA (PTDC/BTM-SAL/30503/2017). The authors acknowledge the financial support by the Portuguese Foundation for Science and Technology (FCT) through the Doctoral Grants (2022.10039.BD, Joana Soeiro, DFA/BD/7692/2020, Maria V. Monteiro) and through an Assistant Researcher contract (DOI: 10.54499/2022.02106.CEECIND/CP1720/CT0028, Vítor M. Gaspar). Additionally, this work was supported by Grants PTDC/NAN-MAT/3901/2020 (DOI: 10.54499/PTDC/NAN-MAT/3901/2020 supported by POCI, FEDER and FCT/MCTES) and Grant ERC-2019-CoG-865437 from the European Research Council (ERC) under the European Union's Horizon 2020 research and innovation programme.
References
- B. Hildebrandt, P. Wust, O. Ahlers, A. Dieing, G. Sreenivasa, T. Kerner, R. Felix and H. Riess, The cellular and molecular basis of hyperthermia, Crit. Rev. Oncol. Hematol., 2002, 43, 33–56 CrossRef PubMed.
- M. Payne, S. H. Bossmann and M. T. Basel, Direct treatment versus indirect: Thermo-ablative and mild hyperthermia effects, Wiley Interdiscip. Rev.: Nanomed. Nanobiotechnol., 2020, 12, e1638 Search PubMed.
- A. Bettaieb, P. K. Wrzal and D. A. Averill-Bates, Hyperthermia: Cancer Treatment and Beyond, Cancer Treat.: Conv. Innovative Approaches, 2013, 257–283 Search PubMed.
- J. Jose, R. Kumar, S. Harilal, G. E. Mathew, D. G. T. Parambi, A. Prabhu, Md. S. Uddin, L. Aleya, H. Kim and B. Mathew, Magnetic nanoparticles for hyperthermia in cancer treatment: an emerging tool, Environ. Sci. Pollut. Res., 2020, 27, 19214–19225 CrossRef PubMed.
- P. S. Yarmolenko, E. J. Moon, C. Landon, A. Manzoor, D. W. Hochman, B. L. Viglianti and M. W. Dewhirst, Thresholds for thermal damage to normal tissues: an update, Int. J. Hyperthermia, 2011, 27, 320–343 CrossRef PubMed.
- G. Hannon, F. L. Tansi, I. Hilger and A. Prina-Mello, The Effects of Localized Heat on the Hallmarks of Cancer, Adv. Ther., 2021, 4, 2000267 CrossRef.
- I. Pereira Gomes, J. Aparecida Duarte, A. L. Chaves Maia, D. Rubello, D. M. Townsend, A. L. Branco de Barros and E. A. Leite, Thermosensitive Nanosystems Associated with Hyperthermia for Cancer Treatment, Pharmaceuticals, 2019, 12, 171 CrossRef PubMed.
-
S. Masunaga, Tumor microenvironment and hyperthermia, in Hyperthermic Oncology from Bench to Bedside, 2016, pp. 151–169.
- S. Toraya-Brown, M. R. Sheen, P. Zhang, L. Chen, J. R. Baird, E. Demidenko, M. J. Turk, P. J. Hoopes, J. R. Conejo-Garcia and S. Fiering, Local hyperthermia treatment of tumors induces CD8(+) T cell-mediated resistance against distal and secondary tumors, Nanomedicine, 2014, 10, 1273–1285 CrossRef CAS PubMed.
- P. Kaur, M. L. Aliru, A. S. Chadha, A. Asea and S. Krishnan, Hyperthermia using nanoparticles–Promises and pitfalls, Int. J. Hyperthermia, 2016, 32, 76–88 CrossRef CAS PubMed.
- J. Beik, Z. Abed, F. S. Ghoreishi, S. Hosseini-Nami, S. Mehrzadi, A. Shakeri-Zadeh and S. K. Kamrava, Nanotechnology in hyperthermia cancer therapy: From fundamental principles to advanced applications, J. Controlled Release, 2016, 235, 205–221 CrossRef CAS PubMed.
- A. J. Giustini, A. A. Petryk, S. M. Cassim, J. A. Tate, I. Baker and P. Jack Hoopes, Magnetic Nanoparticle Hyperthermia in Cancer Treatment, Nano LIFE, 2010, 01, 17–32 CrossRef CAS PubMed.
- S. Dutz and R. Hergt, Magnetic particle hyperthermia – A promising tumour therapy?, Nanotechnology, 2014, 25, 452001 CrossRef PubMed.
- R. Gupta and D. Sharma, Therapeutic response differences between 2D and 3D tumor models of magnetic hyperthermia, Nanoscale Adv., 2021, 3, 3663–3680 RSC.
- K. K. Johnson, P. Koshy, J.-L. Yang and C. C. Sorrell, Preclinical Cancer Theranostics—From Nanomaterials to Clinic: The Missing Link, Adv. Funct. Mater., 2021, 31, 2104199 CrossRef CAS.
- L. P. Ferreira, V. M. Gaspar and J. F. Mano, Decellularized Extracellular Matrix for Bioengineering Physiomimetic 3D in Vitro Tumor Models, Trends Biotechnol., 2020, 38, 1397–1414 CrossRef CAS PubMed.
- G. Bahcecioglu, G. Basara, B. W. Ellis, X. Ren and P. Zorlutuna, Breast cancer models: Engineering the tumor microenvironment, Acta Biomater., 2020, 106, 1–21 CrossRef CAS PubMed.
- P. Benien and A. Swami, 3D tumor models: History, advances and future perspectives, Future Oncol., 2014, 10, 1311–1327 CrossRef CAS PubMed.
- E. M. Scutigliani, Y. Liang, H. Crezee, R. Kanaar and P. M. Krawczyk, Modulating the Heat Stress Response to Improve Hyperthermia-Based Anticancer Treatments, Cancers, 2021, 13, 1243 CrossRef CAS PubMed.
- U. Steint, K. Jürchott, W. Walther, S. Bergmann, P. M. Schlag and H. D. Royer, Hyperthermia-induced Nuclear Translocation of Transcription Factor YB-1 Leads to Enhanced Expression of Multidrug Resistance-related ABC Transporters, J. Biol. Chem., 2001, 276, 28562–28569 CrossRef PubMed.
- J. Tu, X. Luo, H. Liu, J. Zhang and M. He, Cancer spheroids derived exosomes reveal more molecular features relevant to progressed cancer, Biochem. Biophys. Rep., 2021, 26, 101026 CAS.
- K. Stock, M. F. Estrada, S. Vidic, K. Gjerde, A. Rudisch, V. E. Santo, M. Barbier, S. Blom, S. C. Arundkar, I. Selvam, A. Osswald, Y. Stein, S. Gruenewald, C. Brito, W. van Weerden, V. Rotter, E. Boghaert, M. Oren, W. Sommergruber, Y. Chong, R. de Hoogt and R. Graeser, Capturing tumor complexity in vitro: Comparative analysis of 2D and 3D tumor models for drug discovery, Sci. Rep., 2016, 6, 28951 CrossRef CAS PubMed.
- M. A. G. Barbosa, C. P. R. Xavier, R. F. Pereira, V. Petrikaitė and M. H. Vasconcelos, 3D Cell Culture Models as Recapitulators of the Tumor Microenvironment for the Screening of Anti-Cancer Drugs, Cancers, 2021, 14, 190 CrossRef PubMed.
- A. K. Mapanao and V. Voliani, Three-dimensional tumor models: Promoting breakthroughs in nanotheranostics translational research, Appl. Mater Today, 2020, 19, 100552 CrossRef.
- B. Blanco-Fernandez, V. M. Gaspar, E. Engel and J. F. Mano, Proteinaceous Hydrogels for Bioengineering Advanced 3D Tumor Models, Adv. Sci., 2021, 8, 2003129 CrossRef CAS PubMed.
- J. Rodrigues, M. A. Heinrich, L. M. Teixeira and J. Prakash, 3D In Vitro Model (R)evolution: Unveiling Tumor–Stroma Interactions, Trends Cancer, 2021, 7, 249–264 CrossRef CAS PubMed.
- M. V. Monteiro, Y. S. Zhang, V. M. Gaspar and J. F. Mano, 3D-bioprinted cancer-on-a-chip: level-up organotypic in vitro models, Trends Biotechnol., 2021, 40, 432–447 CrossRef PubMed.
- K. C. Valkenburg, A. E. de Groot and K. J. Pienta, Targeting the tumour stroma to improve cancer therapy, Nat. Rev. Clin. Oncol., 2018, 15, 366–381 CrossRef PubMed.
- X. Xu, M. C. Farach-Carson and X. Jia, Three-dimensional in vitro tumor models for cancer research and drug evaluation, Biotechnol. Adv., 2014, 32, 1256–1268 CrossRef CAS PubMed.
- J. U. Menon, 3D tumor models for cancer drug discovery: Current status and outlook, J. Med. Ther., 2018, 2, 1–2 Search PubMed.
- M. M. Paulides, H. Dobsicek Trefna, S. Curto and D. B. Rodrigues, Recent technological advancements in radiofrequency- andmicrowave-mediated hyperthermia for enhancing drug delivery, Adv. Drug Delivery Rev., 2020, 163–164, 3–18 CrossRef CAS PubMed.
- H. P. Kok, E. N. K. Cressman, W. Ceelen, C. L. Brace, R. Ivkov, H. Grüll, G. ter Haar, P. Wust and J. Crezee, Heating technology for malignant tumors: a review, Int. J. Hyperthermia, 2020, 37, 711–741 CrossRef CAS PubMed.
- C. J. Diederich and K. Hynynen, Ultrasound technology for hyperthermia, Ultrasound Med. Biol., 1999, 25, 871–887 CrossRef CAS PubMed.
- G. P. Skandalakis, D. R. Rivera, C. D. Rizea, A. Bouras, J. G. Jesu Raj, D. Bozec and C. G. Hadjipanayis, Hyperthermia treatment advances for brain tumors, Int. J. Hyperthermia, 2020, 37, 3–19 CrossRef CAS PubMed.
- S. Gao, M. Zheng, X. Ren, Y. Tang and X. Liang, Local hyperthermia in head and neck cancer: Mechanism, application and advance, Oncotarget, 2016, 7, 57367 CrossRef PubMed.
- B.-P. Jiang, B. Zhou, Z. Lin, H. Liang and X.-C. Shen, Recent Advances in Carbon Nanomaterials for Cancer Phototherapy, Chem. – Eur. J., 2019, 25, 3993–4004 CrossRef CAS PubMed.
- C. Yu, L. Xu, Y. Zhang, P. S. Timashev, Y. Huang and X.-J. Liang, Polymer-Based Nanomaterials for Noninvasive Cancer Photothermal Therapy, ACS Appl. Polym. Mater., 2020, 2, 4289–4305 CrossRef CAS.
- L. Kafrouni and O. Savadogo, Recent progress on magnetic nanoparticles for magnetic hyperthermia, Prog. Biomater., 2016, 5, 147–160 CrossRef CAS PubMed.
- D. Zhi, T. Yang, J. O’Hagan, S. Zhang and R. F. Donnelly, Photothermal therapy, J. Controlled Release, 2020, 325, 52–71 CrossRef CAS PubMed.
- D. An, J. Fu, B. Zhang, N. Xie, G. Nie, H. Ågren, M. Qiu and H. Zhang, NIR-II Responsive Inorganic 2D Nanomaterials for Cancer Photothermal Therapy: Recent Advances and Future Challenges, Adv. Funct. Mater., 2021, 31, 2101625 CrossRef CAS.
- Y. Zhang, S. Zhang, Z. Zhang, L. Ji, J. Zhang, Q. Wang, T. Guo, S. Ni, R. Cai, X. Mu, W. Long and H. Wang, Recent Progress on NIR-II Photothermal Therapy, Front. Chem., 2021, 9, 728066 CrossRef CAS PubMed.
- Y. Chen, L. Xue, Q. Zhu, Y. Feng and M. Wu, Recent Advances in Second Near-Infrared Region (NIR-II) Fluorophores and Biomedical Applications, Front. Chem., 2021, 9, 750404 CrossRef CAS PubMed.
- E. A. Hussein, M. M. Zagho, G. K. Nasrallah and A. A. Elzatahry, Recent advances in functional nanostructures as cancer photothermal therapy, Int. J. Nanomed., 2018, 13, 2897–2906 CrossRef CAS PubMed.
- L. Cheng, C. Wang, L. Feng, K. Yang and Z. Liu, Functional Nanomaterials for Phototherapies of Cancer, Chem. Rev., 2014, 114, 10869–10939 CrossRef CAS PubMed.
- S. Lv, Y. Miao, D. Liu and F. Song, Recent Development of Photothermal Agents (PTAs) Based on Small Organic Molecular Dyes, ChemBioChem, 2020, 21, 2098–2110 CrossRef CAS PubMed.
- X. Song, Q. Chen and Z. Liu, Recent advances in the development of organic photothermal nano-agents, Nano Res., 2015, 8, 340–354 CrossRef CAS.
- J. Wang and J. Qiu, A review of organic nanomaterials in photothermal cancer therapy, Cancer Res. Front., 2016, 2, 67–84 CrossRef.
- Y. Zhao, T. Zhao, Y. Cao, J. Sun, Q. Zhou, H. Chen, S. Guo, Y. Wang, Y. Zhen, X.-J. Liang and S. Zhang, Temperature-Sensitive Lipid-Coated Carbon Nanotubes for Synergistic Photothermal Therapy and Gene Therapy, ACS Nano, 2021, 15, 6517–6529 CrossRef CAS PubMed.
- A. R. Burke, R. N. Singh, D. L. Carroll, J. C. S. Wood, R. B. D’Agostino, P. M. Ajayan, F. M. Torti and S. V. Torti, The resistance of breast cancer stem cells to conventional hyperthermia and their sensitivity to nanoparticle-mediated photothermal therapy, Biomaterials, 2012, 33, 2961–2970 CrossRef CAS PubMed.
- Y. Cai, P. Liang, Q. Tang, X. Yang, W. Si, W. Huang, Q. Zhang and X. Dong, Diketopyrrolopyrrole–Triphenylamine Organic Nanoparticles as Multifunctional Reagents for Photoacoustic Imaging-Guided Photodynamic/Photothermal Synergistic Tumor Therapy, ACS Nano, 2017, 11, 1054–1063 CrossRef CAS PubMed.
- W. Xu, K. Tamarov, L. Fan, S. Granroth, J. Rantanen, T. Nissinen, S. Peräniemi, O. Uski, M.-R. Hirvonen and V.-P. Lehto, Scalable Synthesis of Biodegradable Black Mesoporous Silicon Nanoparticles for Highly Efficient Photothermal Therapy, ACS Appl. Mater. Interfaces, 2018, 10, 23529–23538 CrossRef CAS PubMed.
- G. Guedes, S. Wang, F. Fontana, P. Figueiredo, J. Lindén, A. Correia, R. J. B. Pinto, S. Hietala, F. L. Sousa and H. A. Santos, Dual-Crosslinked Dynamic Hydrogel Incorporating {Mo154} with pH and NIR Responsiveness for Chemo-Photothermal Therapy, Adv. Mater., 2021, 33, 2007761 CrossRef CAS PubMed.
- X. Jin, S. Yao, F. Qiu, Z. Mao and B. Wang, A multifunctional hydrogel containing gold nanorods and methylene blue for synergistic cancer phototherapy, Colloids Surf., A, 2021, 614, 126154 CrossRef CAS.
- J. Sears, J. Swanner, C. D. Fahrenholtz, C. Snyder, M. Rohde, N. Levi-Polyachenko and R. Singh, Combined Photothermal and Ionizing Radiation Sensitization of Triple-Negative Breast Cancer Using Triangular Silver Nanoparticles, Int. J. Nanomed., 2021, 16, 851–865 CrossRef PubMed.
- X. Jiang, B. Du, Y. Huang, M. Yu and J. Zheng, Cancer Photothermal Therapy with ICG-Conjugated Gold Nanoclusters, Bioconjugate Chem., 2020, 31, 1522–1528 CrossRef CAS PubMed.
- J. H. Lim, D. E. Kim, E.-J. Kim, C. D. Ahrberg and B. G. Chung, Functional Graphene Oxide-Based Nanosheets for Photothermal Therapy, Macromol. Res., 2018, 26, 557–565 CrossRef CAS.
- S. Su, J. Wang, J. Wei, R. Martínez-Zaguilán, J. Qiu and S. Wang, Efficient photothermal therapy of brain cancer through porphyrin functionalized graphene oxide, New J. Chem., 2015, 39, 5743–5749 RSC.
- Y.-W. Chen, Y.-L. Su, S.-H. Hu and S.-Y. Chen, Functionalized graphene nanocomposites for enhancing photothermal therapy in tumor treatment, Adv. Drug Delivery Rev., 2016, 105, 190–204 CrossRef CAS PubMed.
- Z. Sobhani, M. A. Behnam, F. Emami, A. Dehghanian and I. Jamhiri, Photothermal therapy of melanoma tumor using multiwalled carbon nanotubes, Int. J. Nanomed., 2017, 12, 4509–4517 CrossRef CAS PubMed.
- P. McKernan, N. A. Virani, G. N. F. Faria, C. G. Karch, R. Prada Silvy, D. E. Resasco, L. F. Thompson and R. G. Harrison, Targeted Single-Walled Carbon Nanotubes for Photothermal Therapy Combined with Immune Checkpoint Inhibition for the Treatment of Metastatic Breast Cancer, Nanoscale Res. Lett., 2021, 16, 9 CrossRef CAS PubMed.
- Q. Li, L. Hong, H. Li and C. Liu, Graphene oxide-fullerene C60 (GO-C60) hybrid for photodynamic and photothermal therapy triggered by near-infrared light, Biosens. Bioelectron., 2017, 89, 477–482 CrossRef CAS PubMed.
- A. Chen, S. R. Grobmyer and V. B. Krishna, Photothermal Response of Polyhydroxy Fullerenes, ACS Omega, 2020, 5, 14444–14450 CrossRef CAS PubMed.
- X. Bao, Y. Yuan, J. Chen, B. Zhang, D. Li, D. Zhou, P. Jing, G. Xu, Y. Wang, K. Holá, D. Shen, C. Wu, L. Song, C. Liu, R. Zbořil and S. Qu, In vivo theranostics with near-infrared-emitting carbon dots – highly efficient photothermal therapy based on passive targeting after intravenous administration, Light: Sci. Appl., 2018, 7, 91 CrossRef PubMed.
- B. Geng, D. Yang, D. Pan, L. Wang, F. Zheng, W. Shen, C. Zhang and X. Li, NIR-responsive carbon dots for efficient photothermal cancer therapy at low power densities, Carbon, 2018, 134, 153–162 CrossRef CAS.
- X. Wang, L. Zhu, Z. Gu and L. Dai, Carbon nanomaterials for phototherapy, Nanophotonics, 2022, 11, 4955–4976 CrossRef CAS.
- L. D. Dias, H. H. Buzzá, M. D. Stringasci and V. S. Bagnato, Recent Advances in Combined Photothermal and Photodynamic Therapies against Cancer Using Carbon Nanomaterial Platforms for In Vivo Studies, Photochem, 2021, 1, 434–447 CrossRef.
- K. J. Lagos, H. H. Buzzá, V. S. Bagnato and M. P. Romero, Carbon-Based Materials in Photodynamic and Photothermal Therapies Applied to Tumor Destruction, Int. J. Mol. Sci., 2022, 23, 22 CrossRef CAS PubMed.
- S. K. Debnath and R. Srivastava, Drug Delivery With Carbon-Based Nanomaterials as Versatile Nanocarriers: Progress and Prospects, Front. Nanotechnol., 2021, 3, 644564 CrossRef.
- M. L. Taylor, R. E. Wilson, K. D. Amrhein and X. Huang, Gold Nanorod-Assisted Photothermal Therapy and Improvement Strategies, Bioengineering, 2022, 9, 200 CrossRef CAS PubMed.
- J.-L. Li, L. Wang, X.-Y. Liu, Z.-P. Zhang, H.-C. Guo, W.-M. Liu and S.-H. Tang, In vitro cancer cell imaging and therapy using transferrin-conjugated gold nanoparticles, Cancer Lett., 2009, 274, 319–326 CrossRef CAS PubMed.
- B. H. Marghani, A. Fehaid, A. I. Ateya, M. A. Ezz and R. M. Saleh, Photothermal therapeutic potency of plasmonic silver nanoparticles for apoptosis and anti-angiogenesis in testosterone induced benign prostate hyperplasia in rats, Life Sci., 2022, 291, 120240 CrossRef CAS PubMed.
- Y. Liu, D.-D. Wang, L. Zhao, M. Lin, H.-Z. Sun, H.-C. Sun and B. Yang, Polypyrrole-coated flower-like Pd nanoparticles (Pd NPs@PPy) with enhanced stability and heat conversion efficiency for cancer photothermal therapy, RSC Adv., 2016, 6, 15854–15860 RSC.
- J.-W. Xiao, S.-X. Fan, F. Wang, L.-D. Sun, X.-Y. Zheng and C.-H. Yan, Porous Pd nanoparticles with high photothermal conversion efficiency for efficient ablation of cancer cells, Nanoscale, 2014, 6, 4345–4351 RSC.
- R. Zhao, J. Xiang, B. Wang, L. Chen and S. Tan, Recent Advances in the Development of Noble Metal NPs for Cancer Therapy, Bioinorg. Chem. Appl., 2022, 2022, 2444516 Search PubMed.
- P. K. Jain, X. Huang, I. H. El-Sayed and M. A. El-Sayed, Noble Metals on the Nanoscale: Optical and Photothermal Properties and Some Applications in Imaging, Sensing, Biology, and Medicine, Acc. Chem. Res., 2008, 41, 1578–1586 CrossRef CAS PubMed.
- B. Klębowski, J. Depciuch, M. Parlińska-Wojtan and J. Baran, Applications of Noble Metal-Based Nanoparticles in Medicine, Int. J. Mol. Sci., 2018, 19, 4031 CrossRef PubMed.
- Z. Lv, S. He, Y. Wang and X. Zhu, Noble Metal Nanomaterials for NIR-Triggered Photothermal Therapy in Cancer, Adv. Healthcare Mater., 2021, 10, 2001806 CrossRef CAS PubMed.
- B. Zhou, Y. Li, G. Niu, M. Lan, Q. Jia and Q. Liang, Near-Infrared Organic Dye-Based Nanoagent for the Photothermal Therapy of Cancer, ACS Appl. Mater. Interfaces, 2016, 8, 29899–29905 CrossRef CAS PubMed.
- S. Luo, E. Zhang, Y. Su, T. Cheng and C. Shi, A review of NIR dyes in cancer targeting and imaging, Biomaterials, 2011, 32, 7127–7138 CrossRef CAS PubMed.
- X. Zheng, F. Zhou, B. Wu, W. R. Chen and D. Xing, Enhanced Tumor Treatment Using Biofunctional Indocyanine Green-Containing Nanostructure by Intratumoral or Intravenous Injection, Mol. Pharmaceutics, 2012, 9, 514–522 CrossRef CAS PubMed.
- H.-J. Yoon, H.-S. Lee, J.-Y. Lim and J.-H. Park, Liposomal Indocyanine Green for Enhanced Photothermal Therapy, ACS Appl. Mater. Interfaces, 2017, 9, 5683–5691 CrossRef CAS PubMed.
- M. Zheng, P. Zhao, Z. Luo, P. Gong, C. Zheng, P. Zhang, C. Yue, D. Gao, Y. Ma and L. Cai, Robust ICG Theranostic Nanoparticles for Folate Targeted Cancer Imaging and Highly Effective Photothermal Therapy, ACS Appl. Mater. Interfaces, 2014, 6, 6709–6716 CrossRef CAS PubMed.
- X. Xu, H. Wu, Y. Yang, B. Liu, J. Tian, H. Bao and T. Liu, PLGA-coated methylene blue nanoparticles for photoacoustic imaging and photodynamic/photothermal cascaded precisely synergistic therapy of tumor, RSC Adv., 2022, 12, 1543–1549 RSC.
- B. He, H. Hu, T. Tan, H. Wang, K. Sun, Y. Li and Z. Zhang, IR-780-loaded polymeric micelles enhance the efficacy of photothermal therapy in treating breast cancer lymphatic metastasis in mice, Acta Pharmacol. Sin., 2018, 39, 132–139 CrossRef CAS PubMed.
- K. Wang, Y. Zhang, J. Wang, A. Yuan, M. Sun, J. Wu and Y. Hu, Self-assembled IR780-loaded transferrin nanoparticles as an imaging, targeting and PDT/PTT agent for cancer therapy, Sci. Rep., 2016, 6, 27421 CrossRef PubMed.
- M. Liu, P. Zhang, L. Deng, D. Guo, M. Tan, J. Huang, Y. Luo, Y. Cao and Z. Wang, IR780-based light-responsive nanocomplexes combining phase transition for enhancing multimodal imaging-guided photothermal therapy, Biomater. Sci., 2019, 7, 1132–1146 RSC.
- S. K. Sharma, N. Shrivastava, F. Rossi, L. D. Tung and N. T. K. Thanh, Nanoparticles-based magnetic and photo induced hyperthermia for cancer treatment, Nano Today, 2019, 29, 100795 CrossRef CAS.
- X. Yu, S. Ding, R. Yang, C. Wu and W. Zhang, Research progress on magnetic nanoparticles for magnetic induction hyperthermia of malignant tumor, Ceram. Int., 2021, 47, 5909–5917 CrossRef CAS.
- J. Pearce, A. Giustini, R. Stigliano and P. Jack Hoopes, Magnetic Heating of Nanoparticles: The Importance of Particle Clustering to Achieve Therapeutic Temperatures, J. Nanotechnol. Eng. Med., 2013, 4, 110071 Search PubMed.
- A. E. Deatsch and B. A. Evans, Heating efficiency in magnetic nanoparticle hyperthermia, J. Magn. Magn. Mater., 2014, 354, 163–172 CrossRef CAS.
- D. Egea-Benavente, J. G. Ovejero, M. D. Morales and D. F. Barber, Understanding MNPs Behaviour in Response to AMF in Biological Milieus and the Effects at the Cellular Level: Implications for a Rational Design That Drives Magnetic Hyperthermia Therapy toward Clinical Implementation, Cancers, 2021, 13, 4583 CrossRef CAS PubMed.
- M. Osaci and M. Cacciola, Specific loss power in superparamagnetic hyperthermia: nanofluid versus composite, IOP Conf. Ser.: Mater. Sci. Eng., 2017, 163, 12008 Search PubMed.
- G. Vallejo-Fernandez, O. Whear, A. G. Roca, S. Hussain, J. Timmis, V. Patel and K. O’Grady, Mechanisms of hyperthermia in magnetic nanoparticles, J. Phys. D: Appl. Phys., 2013, 46, 312001 CrossRef.
- P. Das, M. Colombo and D. Prosperi, Recent advances in magnetic fluid hyperthermia for cancer therapy, Colloids Surf., B, 2019, 174, 42–55 CrossRef CAS PubMed.
- V. Vilas-Boas, F. Carvalho and B. Espiña, Magnetic hyperthermia for cancer treatment: Main parameters affecting the outcome of in vitro and in vivo studies, Molecules, 2020, 25, 1–30 CrossRef PubMed.
- D. Ortega and Q. A. Pankhurst, Magnetic hyperthermia, Nanoscience, 2013, 1, 60–88 CAS.
- Q. A. Pankhurst, J. Connolly, S. K. Jones and J. Dobson, Applications of magnetic nanoparticles in biomedicine, J. Phys. D: Appl. Phys., 2003, 36, R167–R181 CrossRef CAS.
- I. Raouf, S. Khalid, A. Khan, J. Lee, H. S. Kim and M.-H. Kim, A review on numerical modeling for magnetic nanoparticle hyperthermia: Progress and challenges, J. Therm. Biol., 2020, 91, 102644 CrossRef CAS PubMed.
- H. Fatima, T. Charinpanitkul and K.-S. Kim, Fundamentals to Apply Magnetic Nanoparticles for Hyperthermia Therapy, Nanomaterials, 2021, 11, 1203 CrossRef CAS PubMed.
- A. Millan, A. Urtizberea, N. J. O. Silva, F. Palacio, V. S. Amaral, E. Snoeck and V. Serin, Surface effects in maghemite nanoparticles, J. Magn. Magn. Mater., 2007, 312, L5–L9 CrossRef CAS.
- C. L. Dennis and R. Ivkov, Physics of heat generation using magnetic nanoparticles for hyperthermia, Int. J. Hyperthermia, 2013, 29, 715–729 CrossRef PubMed.
- A. G. Kolhatkar, A. C. Jamison, D. Litvinov, R. C. Willson and T. R. Lee, Tuning the Magnetic Properties of Nanoparticles, Int. J. Mol. Sci., 2013, 14, 15977–16009 CrossRef PubMed.
- H. Shirzadfar, M. Nadi, D. Kourtiche, S. Yamada and T. Hauet, Needle-type GMR sensor to estimate the magnetic properties of diluted ferrofluid for biomedicine application, Irbm, 2015, 36, 178–184 CrossRef.
- A. K. Gupta and M. Gupta, Synthesis and surface engineering of iron oxide nanoparticles for biomedical applications, Biomaterials, 2005, 26, 3995–4021 CrossRef CAS PubMed.
- S. Prijic, J. Scancar, R. Romih, M. Cemazar, V. B. Bregar, A. Znidarsic and G. Sersa, Increased cellular uptake of biocompatible superparamagnetic iron oxide nanoparticles into malignant cells by an external magnetic field, J. Membr. Biol., 2010, 236, 167–179 CrossRef CAS.
- X. Chu, J. Yu and Y. L. Hou, Surface modification of magnetic nanoparticles in biomedicine, Chin. Phys. B, 2015, 24, 014704 CrossRef.
- H. Aslam, S. Shukrullah, M. Y. Naz, H. Fatima, H. Hussain, S. Ullah and M. A. Assiri, Current and future perspectives of multifunctional magnetic nanoparticles based controlled drug delivery systems, J. Drug Delivery Sci. Technol., 2022, 67, 102946 CrossRef CAS.
- B. Dorjsuren, B. Chaurasiya, Z. Ye, Y. Liu, W. Li, C. Wang, D. Shi, C. E. Evans, T. J. Webster and Y. Shen, Cetuximab-Coated Thermo-Sensitive Liposomes Loaded with Magnetic Nanoparticles and Doxorubicin for Targeted EGFR-Expressing Breast Cancer Combined Therapy, Int. J. Nanomed., 2020, 15, 8201–8215 CrossRef CAS.
- A. Toro-Cordova, M. Flores-Cruz, J. Santoyo-Salazar, E. Carrillo-Nava, R. Jurado, P. Figueroa-Rodriguez, P. Lopez-Sanchez, L. Medina and P. Garcia-Lopez, Liposomes Loaded with Cisplatin and Magnetic Nanoparticles: Physicochemical Characterization, Pharmacokinetics, and In-Vitro Efficacy, Molecules, 2018, 23, 2272 CrossRef PubMed.
- L. Tavano, C. Oliviero Rossi, N. Picci and R. Muzzalupo, Spontaneous temperature-sensitive Pluronic® based niosomes: Triggered drug release using mild hyperthermia, Int. J. Pharm., 2016, 511, 703–708 CrossRef CAS PubMed.
- R. Juneja and I. Roy, Iron oxide-doped niosomes as drug carriers for magnetically targeted drug delivery, Int. J. Nanomed., 2018, 13, 7–9 CrossRef CAS PubMed.
- P. S. Zangabad, S. Mirkiani, S. Shahsavari, B. Masoudi, M. Masroor, H. Hamed, Z. Jafari, Y. D. Taghipour, H. Hashemi, M. Karimi and M. R. Hamblin, Stimulus-responsive liposomes as smart nanoplatforms for drug delivery applications, Nanotechnol. Rev., 2018, 7, 95–122 CrossRef CAS PubMed.
- C. M. Wells, M. Harris, L. Choi, V. P. Murali, F. D. Guerra and J. A. Jennings, Stimuli-Responsive Drug Release from Smart Polymers, J. Funct. Biomater., 2019, 10, 34 CrossRef CAS PubMed.
- K. S. Joshy, R. Augustine, A. Mayeen, S. M. Alex, A. Hasan, S. Thomas and H. Chi, NiFe2O4/poly(ethylene glycol)/lipid–polymer hybrid nanoparticles for anti-cancer drug delivery, New J. Chem., 2020, 44, 18162–18172 RSC.
- A. Mohanty, S. Uthaman and I.-K. Park, Utilization of Polymer-Lipid Hybrid Nanoparticles for Targeted Anti-Cancer Therapy, Molecules, 2020, 25, 4377 CrossRef CAS PubMed.
- M. Bañobre-López, A. Teijeiro and J. Rivas, Magnetic nanoparticle-based hyperthermia for cancer treatment, Rep. Pract. Oncol. Radiother., 2013, 18, 397–400 CrossRef.
- M. Nabavinia and J. Beltran-Huarac, Recent Progress in Iron Oxide Nanoparticles as Therapeutic Magnetic Agents for Cancer Treatment and Tissue Engineering, ACS Appl. Bio Mater., 2020, 3, 8172–8187 CrossRef CAS PubMed.
- C. Pucci, A. Degl’Innocenti, M. Belenli Gümü
and G. Ciofani, Superparamagnetic iron oxide nanoparticles for magnetic hyperthermia: recent advancements, molecular effects, and future directions in the omics era, Biomater. Sci., 2022, 10, 2103–2121 RSC.
- H. Etemadi and P. G. Plieger, Magnetic Fluid Hyperthermia Based on Magnetic Nanoparticles: Physical Characteristics, Historical Perspective, Clinical Trials, Technological Challenges, and Recent Advances, Adv. Ther., 2020, 3, 2000061 CrossRef.
- E. M. Materón, C. M. Miyazaki, O. Carr, N. Joshi, P. H. S. Picciani, C. J. Dalmaschio, F. Davis and F. M. Shimizu, Magnetic nanoparticles in biomedical applications: A review, Appl. Surf. Sci. Adv., 2021, 6, 100163 CrossRef.
- M. I. Anik, M. K. Hossain, I. Hossain, A. M. U. B. Mahfuz, M. T. Rahman and I. Ahmed, Recent progress of magnetic nanoparticles in biomedical applications: A review, Nano Sel., 2021, 2, 1146–1186 CrossRef CAS.
- A. J. Biacchi, T. Q. Bui, C. L. Dennis, S. I. Woods and A. R. Hight Walker, Design and engineering colloidal magnetic particles for nanoscale thermometry, Int. J. Magn. Part. Imaging, 2020, 6 DOI:10.18416/IJMPI.2020.2009068.
- F. Soetaert, P. Korangath, D. Serantes, S. Fiering and R. Ivkov, Cancer therapy with iron oxide nanoparticles: Agents of thermal and immune therapies, Adv. Drug Delivery Rev., 2020, 163–164, 65–83 CrossRef CAS PubMed.
- E. A. Périgo, G. Hemery, O. Sandre, D. Ortega, E. Garaio, F. Plazaola and F. J. Teran, Fundamentals and advances in magnetic hyperthermia, Appl. Phys. Rev., 2015, 2, 041302 Search PubMed.
- M. Chang, Z. Hou, M. Wang, C. Li and J. Lin, Recent Advances in Hyperthermia Therapy-Based Synergistic Immunotherapy, Adv. Mater., 2020, 33, 2004788 CrossRef PubMed.
- J. B. Mamani, T. K. F. Souza, M. P. Nucci, F. A. Oliveira, L. P. Nucci, A. H. Alves, G. N. A. Rego, L. Marti and L. F. Gamarra, In Vitro Evaluation of Hyperthermia Magnetic Technique Indicating the Best Strategy for Internalization of Magnetic Nanoparticles Applied in Glioblastoma Tumor Cells, Pharmaceutics, 2021, 13, 1219 CrossRef CAS PubMed.
- C. Iacovita, I. Fizeşan, A. Pop, L. Scorus, R. Dudric, G. Stiufiuc, N. Vedeanu, R. Tetean, F. Loghin, R. Stiufiuc and C. M. Lucaciu, In Vitro Intracellular Hyperthermia of Iron Oxide Magnetic Nanoparticles, Synthesized at High Temperature by a Polyol Process, Pharmaceutics, 2020, 12, 424 CrossRef CAS.
- Z. Hedayatnasab, A. Dabbagh, F. Abnisa and W. M. A. Wan Daud, Polycaprolactone-coated superparamagnetic iron oxide nanoparticles for in vitro magnetic hyperthermia therapy of cancer, Eur. Polym. J., 2020, 133, 109789 CrossRef CAS.
- K. El-Boubbou, O. M. Lemine, R. Ali, S. M. Huwaizi, S. Al-Humaid and A. AlKushi, Evaluating magnetic and thermal effects of
various Polymerylated magnetic iron oxide nanoparticles for combined chemo-hyperthermia, New J. Chem., 2022, 46, 5489–5504 RSC.
- H. A. Albarqi, L. H. Wong, C. Schumann, F. Y. Sabei, T. Korzun, X. Li, M. N. Hansen, P. Dhagat, A. S. Moses, O. Taratula and O. Taratula, Biocompatible Nanoclusters with High Heating Efficiency for Systemically Delivered Magnetic Hyperthermia, ACS Nano, 2019, 13, 6383–6395 CrossRef CAS PubMed.
- V. Narayanaswamy, I. M. Obaidat, A. S. Kamzin, S. Latiyan, S. Jain, H. Kumar, C. Srivastava, S. Alaabed and B. Issa, Synthesis of Graphene Oxide-Fe3O4 Based Nanocomposites Using the Mechanochemical Method and in Vitro Magnetic Hyperthermia, Int. J. Mol. Sci., 2019, 20, 3368 CrossRef CAS PubMed.
- A. Makridis, K. Topouridou, M. Tziomaki, D. Sakellari, K. Simeonidis, M. Angelakeris, M. P. Yavropoulou, J. G. Yovos and O. Kalogirou, In vitro application of Mn-ferrite nanoparticles as novel magnetic hyperthermia agents, J. Mater. Chem. B, 2014, 2, 8390–8398 RSC.
- S. Wilhelm, A. J. Tavares, Q. Dai, S. Ohta, J. Audet, H. F. Dvorak and W. C. W. Chan, Analysis of nanoparticle delivery to tumours, Nat. Rev. Mater., 2016, 1, 16014 CrossRef CAS.
- S. Barua and S. Mitragotri, Challenges associated with penetration of nanoparticles across cell and tissue barriers: A review of current status and future prospects, Nano Today, 2014, 9, 223–243 CrossRef CAS PubMed.
- M. Longmire, P. L. Choyke and H. Kobayashi, Clearance properties of nano-sized particles and molecules as imaging agents: considerations and caveats, Nanomedicine, 2008, 3, 703–717 CrossRef CAS PubMed.
- Y. Nakamura, A. Mochida, P. L. Choyke and H. Kobayashi, Nanodrug Delivery: Is the Enhanced Permeability and Retention Effect Sufficient for Curing Cancer?, Bioconjugate Chem., 2016, 27, 2225–2238 CrossRef CAS PubMed.
- M. J. Mitchell, M. M. Billingsley, R. M. Haley, M. E. Wechsler, N. A. Peppas and R. Langer, Engineering precision nanoparticles for drug delivery, Nat. Rev. Drug Discovery, 2021, 20, 101–124 CrossRef CAS PubMed.
- J. Yoo, C. Park, G. Yi, D. Lee and H. Koo, Active Targeting Strategies Using Biological Ligands for Nanoparticle Drug Delivery Systems, Cancers, 2019, 11, 640 CrossRef CAS PubMed.
- Y. Wu, Q. Li, Y. Kong, Z. Wang, C. Lei, J. Li, L. Ding, C. Wang, Y. Cheng, Y. Wei, Y. Song, Z. Yang, C. Tu, Y. Ding and T. Ying, A highly stable human single-domain antibody-drug conjugate exhibits superior penetration and treatment of solid tumors, Mol. Ther., 2022, 30, 2785–2799 CrossRef CAS PubMed.
- L. Chen, Y. Wu, H. Wu, J. Li, J. Xie, F. Zang, M. Ma, N. Gu and Y. Zhang, Magnetic targeting combined with active targeting of dual-ligand iron oxide nanoprobes to promote the penetration depth in tumors for effective magnetic resonance imaging and hyperthermia, Acta Biomater., 2019, 96, 491–504 CrossRef CAS PubMed.
- M. Kapałczyńska, T. Kolenda, W. Przybyła, M. Zajączkowska, A. Teresiak, V. Filas, M. Ibbs, R. Bliźniak, Ł. Łuczewski and K. Lamperska, 2D and 3D cell cultures - a comparison of different types of cancer cell cultures, Arch. Med. Sci., 2018, 14, 910–919 Search PubMed.
- B. D. Cardoso, E. M. S. Castanheira, S. Lanceros-Méndez and V. F. Cardoso, Recent Advances on Cell Culture Platforms for In Vitro Drug Screening and Cell Therapies: From Conventional to Microfluidic Strategies, Adv. Healthcare Mater., 2023, 12, 2202936 CrossRef CAS PubMed.
- S. Pozzi, A. Scomparin, S. Israeli Dangoor, D. Rodriguez Ajamil, P. Ofek, L. Neufeld, A. Krivitsky, D. Vaskovich-Koubi, R. Kleiner, P. Dey, S. Koshrovski-Michael, N. Reisman and R. Satchi-Fainaro, Meet me halfway: Are in vitro 3D cancer models on the way to replace in vivo models for nanomedicine development?, Adv. Drug Delivery Rev., 2021, 175, 113760 CrossRef CAS PubMed.
- X. Xin, H. Yang, F. Zhang and S. T. Yang, 3D cell coculture tumor model: A promising approach for future cancer drug discovery, Process Biochem., 2019, 78, 148–160 CrossRef CAS.
- L. C. Kimlin, G. Casagrande and V. M. Virador, In vitro three-dimensional (3D) models in cancer research: An update, Mol. Carcinog., 2013, 52, 167–182 CrossRef PubMed.
- M. Xu, T. Zhang, R. Xia, Y. Wei and X. Wei, Targeting the tumor stroma for cancer therapy, Mol. Cancer, 2022, 21, 208 CrossRef PubMed.
- P. I. Ribeiro Franco, A. P. Rodrigues, L. B. de Menezes and M. Pacheco, Miguel, Tumor microenvironment components: Allies of cancer progression, Pathol., Res. Pract., 2020, 216, 152729 CrossRef CAS PubMed.
- M. Sund and R. Kalluri, Tumor stroma derived biomarkers in cancer, Cancer Metastasis Rev., 2009, 28, 177–183 CrossRef PubMed.
- Z. Werb and P. Lu, The Role of Stroma in Tumor Development, Cancer J., 2015, 21, 250–253 CrossRef CAS PubMed.
- G. Yeldag, A. Rice and A. Del Río Hernández, Chemoresistance and the Self-Maintaining Tumor Microenvironment, Cancers, 2018, 10, 471 CrossRef CAS PubMed.
- Z. Yuan, Y. Li, S. Zhang, X. Wang, H. Dou, X. Yu, Z. Zhang, S. Yang and M. Xiao, Extracellular matrix remodeling in tumor progression and immune escape: from mechanisms to treatments, Mol. Cancer, 2023, 22, 48 CrossRef CAS PubMed.
- E. L. S. Fong, D. A. Harrington, M. C. Farach-Carson and H. Yu, Heralding a new paradigm in 3D tumor modeling, Biomaterials, 2016, 108, 197–213 CrossRef CAS PubMed.
- J. C. Fontoura, C. Viezzer, F. G. dos Santos, R. A. Ligabue, R. Weinlich, R. D. Puga, D. Antonow, P. Severino and C. Bonorino, Comparison of 2D and 3D cell culture models for cell growth, gene expression and drug resistance, Mater. Sci. Eng., C, 2020, 107, 110264 CrossRef CAS PubMed.
- A. R. Cantelmo, A. Pircher, J. Kalucka and P. Carmeliet, Vessel pruning or healing: endothelial metabolism as a novel target?, Expert Opin. Ther. Targets, 2017, 21, 239–247 CrossRef CAS PubMed.
- D. W. Siemann, The unique characteristics of tumor vasculature and preclinical evidence for its selective disruption by Tumor-Vascular Disrupting Agents, Cancer Treat. Rev., 2011, 37, 63–74 CrossRef CAS PubMed.
- B. Pinto, A. C. Henriques, P. M. A. Silva and H. Bousbaa, Three-dimensional spheroids as in vitro preclinical models for cancer research, Pharmaceutics, 2020, 12, 1–38 CrossRef.
- E. B. Rankin and A. J. Giaccia, Hypoxic control of metastasis, Science, 2016, 352, 175–180 CrossRef CAS PubMed.
- Y. Mao, E. T. Keller, D. H. Garfield, K. Shen and J. Wang, Stromal cells in tumor microenvironment and breast cancer, Cancer Metastasis Rev., 2013, 32, 303–315 CrossRef PubMed.
- C. E. Weber and P. C. Kuo, The tumor microenvironment, Surg. Oncol., 2012, 21, 172–177 CrossRef PubMed.
- V. Gouirand, F. Guillaumond and S. Vasseur, Influence of the tumor microenvironment on cancer cells metabolic reprogramming, Front. Oncol., 2018, 8, 117 CrossRef PubMed.
- P. Vaupel, The Role of Hypoxia-Induced Factors in Tumor Progression, Oncologist, 2004, 9, 10–17 CrossRef CAS PubMed.
- R. J. Seager, C. Hajal, F. Spill, R. D. Kamm and M. H. Zaman, Dynamic interplay between tumour, stroma and immune system can drive or prevent tumour progression, Convergent Sci. Phys. Oncol., 2017, 3, 034002 CrossRef PubMed.
- H. Willers, C. G. Azzoli, W. L. Santivasi and F. Xia, Basic mechanisms of therapeutic resistance to radiation and chemotherapy in lung cancer, Cancer J., 2013, 19, 200–207 CrossRef CAS PubMed.
- T. Stylianopoulos, L. L. Munn and R. K. Jain, Reengineering the Physical Microenvironment of Tumors to Improve Drug Delivery and Efficacy: From Mathematical Modeling to Bench to Bedside, Trends Cancer, 2018, 4, 292–319 CrossRef CAS PubMed.
- G. M. Balachander, R. Kotcherlakota, B. Nayak, D. Kedaria, A. Rangarajan and K. Chatterjee, 3D Tumor Models for Breast Cancer: Whither We Are and What We Need, ACS Biomater. Sci. Eng., 2021, 7, 3470–3486 CrossRef CAS.
- Q. Zhang, S. Shi, Y. Yen, J. Brown, J. Q. Ta and A. D. Le, A subpopulation of CD133+ cancer stem-like cells characterized in human oral squamous cell carcinoma confer resistance to chemotherapy, Cancer Lett., 2010, 289, 151–160 CrossRef CAS PubMed.
- L. Chen, Z. Xiao, Y. Meng, Y. Zhao, J. Han, G. Su, B. Chen and J. Dai, The enhancement of cancer stem cell properties of MCF-7 cells in 3D collagen scaffolds for modeling of cancer and anti-cancer drugs, Biomaterials, 2012, 33, 1437–1444 CrossRef CAS PubMed.
- S. Feng, X. Duan, P.-K. Lo, S. Liu, X. Liu, H. Chen and Q. Wang, Expansion of breast cancer stem cells with fibrous scaffolds, Integr. Biol., 2013, 5, 768–777 CrossRef CAS PubMed.
- E. C. Costa, A. F. Moreira, D. de Melo-Diogo, V. M. Gaspar, M. P. Carvalho and I. J. Correia, 3D tumor spheroids: an overview on the tools and techniques used for their analysis, Biotechnol. Adv., 2016, 34, 1427–1441 CrossRef.
- W. Mueller-Klieser, Tumor biology and experimental therapeutics, Crit. Rev. Oncol. Hematol., 2000, 36, 123–139 CrossRef CAS.
- Z. Gilazieva, A. Ponomarev, C. Rutland, A. Rizvanov and V. Solovyeva, Promising applications of tumor spheroids and organoids for personalized medicine, Cancers, 2020, 12, 1–19 CrossRef PubMed.
- S. Gunti, A. T. K. Hoke, K. P. Vu and N. R. London, Organoid and spheroid tumor models: Techniques and applications, Cancers, 2021, 13, 1–18 CrossRef PubMed.
- F. Vakhshiteh, Z. Bagheri, M. Soleimani, A. Ahvaraki, P. Pournemat, S. E. Alavi and Z. Madjd, Heterotypic tumor spheroids: a platform for nanomedicine evaluation, J. Nanobiotechnol., 2023, 21, 249 CrossRef PubMed.
- L. P. Ferreira, V. M. Gaspar, M. V. Monteiro, B. Freitas, N. J. O. Silva and J. F. Mano, Screening of dual chemo-photothermal cellular nanotherapies in organotypic breast cancer 3D spheroids, J. Controlled Release, 2021, 331, 85–102 CrossRef CAS PubMed.
- L. P. Ferreira, V. M. Gaspar, L. Mendes, I. F. Duarte and J. F. Mano, Organotypic 3D decellularized matrix tumor spheroids for high-throughput drug screening, Biomaterials, 2021, 275, 120983 CrossRef CAS PubMed.
- J. Ahn, D.-H. Kim, D.-J. Koo, J. Lim, T.-E. Park, J. Lee, J. Ko, S. Kim, M. Kim, K.-S. Kang, D.-H. Min, S.-Y. Kim, Y. Kim and N. L. Jeon, 3D microengineered vascularized tumor spheroids for drug delivery and efficacy testing, Acta Biomater., 2023, 165, 153–167 CrossRef CAS PubMed.
- D. S. Reynolds, K. M. Tevis, W. A. Blessing, Y. L. Colson, M. H. Zaman and M. W. Grinstaff, Breast Cancer Spheroids Reveal a Differential Cancer Stem Cell Response to Chemotherapeutic Treatment, Sci. Rep., 2017, 7, 10382 CrossRef PubMed.
- J. Källberg, A. Harrison, V. March, S. Bērziņa, I. Nemazanyy, O. Kepp, G. Kroemer, S. Mouillet-Richard, P. Laurent-Puig, V. Taly and W. Xiao, Intratumor heterogeneity and cell secretome promote chemotherapy resistance and progression of colorectal cancer, Cell Death Dis., 2023, 14, 306 CrossRef PubMed.
- M. Magan, E. Wiechec and K. Roberg, CAFs affect the proliferation and treatment response of head and neck cancer spheroids during co-culturing in a unique in vitro model, Cancer Cell Int., 2020, 20, 599 CrossRef CAS PubMed.
- B.-W. Huang and J.-Q. Gao, Application of 3D cultured multicellular spheroid tumor models in tumor-targeted drug delivery system research, J. Controlled Release, 2018, 270, 246–259 CrossRef CAS PubMed.
- L. P. Ferreira, V. M. Gaspar and J. F. Mano, Design of spherically structured 3D in vitro tumor models -Advances and prospects, Acta Biomater., 2018, 75, 11–34 CrossRef CAS PubMed.
- J. Drost and H. Clevers, Organoids in cancer research, Nat. Rev. Cancer, 2018, 18, 407–418 CrossRef CAS.
- S. Nantasanti, A. de Bruin, J. Rothuizen, L. C. Penning and B. A. Schotanus, Concise Review: Organoids Are a Powerful Tool for the Study of Liver Disease and Personalized Treatment Design in Humans and Animals, Stem Cells Transl. Med., 2016, 5, 325–330 CrossRef CAS PubMed.
- J. Kim, B.-K. Koo and J. A. Knoblich, Human organoids: model systems for human biology and medicine, Nat. Rev. Mol. Cell Biol., 2020, 21, 571–584 CrossRef CAS PubMed.
- V. Veninga and E. E. Voest, Tumor organoids: Opportunities and challenges to guide precision medicine, Cancer Cell, 2021, 39, 1190–1201 CrossRef CAS PubMed.
- X. Liu, J. Fang, S. Huang, X. Wu, X. Xie, J. Wang, F. Liu, M. Zhang, Z. Peng and N. Hu, Tumor-on-a-chip: from bioinspired design to biomedical application, Microsyst. Nanoeng., 2021, 7, 50 CrossRef CAS PubMed.
- R. O. Rodrigues, P. C. Sousa, J. Gaspar, M. Bañobre-López, R. Lima and G. Minas, Organ-on-a-Chip: A Preclinical Microfluidic Platform for the Progress of Nanomedicine, Small, 2020, 16, 2003517 CrossRef CAS PubMed.
- K. E. Sung and D. J. Beebe, Microfluidic 3D models of cancer, Adv. Drug Delivery Rev., 2014, 79–80, 68–78 CrossRef CAS PubMed.
- Y. Fang and R. Eglen, Three-Dimensional Cell Cultures in Drug Discovery and Development, SLAS discovery, 2017, 22, 456–472 CrossRef CAS.
- S. J. Hachey and C. C. W. Hughes, Applications of tumor chip technology, Lab Chip, 2018, 18, 2893–2912 RSC.
- G. Fang, Y.-C. Chen, H. Lu and D. Jin, Advances in Spheroids and Organoids on a Chip, Adv. Funct. Mater., 2023, 33, 2215043 CrossRef CAS.
- Y. Nashimoto, R. Okada, S. Hanada, Y. Arima, K. Nishiyama, T. Miura and R. Yokokawa, Vascularized cancer on a chip: The effect of perfusion on growth and drug delivery of tumor spheroid, Biomaterials, 2020, 229, 119547 CrossRef CAS PubMed.
- D. Kim, K. S. Hwang, E. U. Seo, S. Seo, B. C. Lee, N. Choi, J. Choi and H. N. Kim, Vascularized Lung Cancer Model for Evaluating the Promoted Transport of Anticancer Drugs and Immune Cells in an Engineered Tumor Microenvironment, Adv. Healthcare Mater., 2022, 11, 2102581 CrossRef CAS PubMed.
- Y. Liu, C. Sakolish, Z. Chen, D. T. T. Phan, R. H. F. Bender, C. C. W. Hughes and I. Rusyn, Human in vitro vascularized micro-organ and micro-tumor models are reproducible organ-on-a-chip platforms for studies of anticancer drugs, Toxicology, 2020, 445, 152601 CrossRef CAS PubMed.
- L. Wan, C. A. Neumann and P. R. LeDuc, Tumor-on-a-chip for integrating a 3D tumor microenvironment: chemical and mechanical factors, Lab Chip, 2020, 20, 873–888 RSC.
- C. Ding, X. Chen, Q. Kang and X. Yan, Biomedical Application of Functional Materials in Organ-on-a-Chip, Front. Bioeng. Biotechnol., 2020, 8, 823 CrossRef PubMed.
- X. J. Li, A. V. Valadez, P. Zuo and Z. Nie, Microfluidic 3D cell culture: potential application for tissue-based bioassays, Bioanalysis, 2012, 4, 1509–1525 CrossRef CAS PubMed.
- N. Gupta, J. R. Liu, B. Patel, D. E. Solomon, B. Vaidya and V. Gupta, Microfluidics-based 3D cell culture models: Utility in novel drug discovery and delivery research, Bioeng. Transl. Med., 2016, 1, 63–81 CrossRef PubMed.
- J. M. Ayuso, S. Rehman, M. Virumbrales-Munoz, P. H. McMinn, P. Geiger, C. Fitzgerald, T. Heaster, M. C. Skala and D. J. Beebe, Microfluidic tumor-on-a-chip model to evaluate the role of tumor environmental stress on NK cell exhaustion, Sci. Adv., 2023, 7, eabc2331 CrossRef PubMed.
- X. Cao, R. Ashfaq, F. Cheng, S. Maharjan, J. Li, G. Ying, S. Hassan, H. Xiao, K. Yue and Y. S. Zhang, A Tumor-on-a-Chip System with Bioprinted Blood and Lymphatic Vessel Pair, Adv. Funct. Mater., 2019, 29, 1807173 CrossRef PubMed.
- M. R. Carvalho, D. Barata, L. M. Teixeira, S. Giselbrecht, R. L. Reis, J. M. Oliveira, R. Truckenmüller and P. Habibovic, Colorectal tumor-on-a-chip system: A 3D tool for precision onco-nanomedicine, Sci. Adv., 2023, 5, eaaw1317 CrossRef PubMed.
- J. M. Ayuso, M. Virumbrales-Munoz, P. H. McMinn, S. Rehman, I. Gomez, M. R. Karim, R. Trusttchel, K. B. Wisinski, D. J. Beebe and M. C. Skala, Tumor-on-a-chip: a microfluidic model to study cell response to environmental gradients, Lab Chip, 2019, 19, 3461–3471 RSC.
- A. Marino, M. Battaglini, A. Carmignani, F. Pignatelli, D. De Pasquale, O. Tricinci and G. Ciofani, Magnetic self-assembly of 3D multicellular microscaffolds: A biomimetic brain tumor-on-a-chip for drug delivery and selectivity testing, APL Bioeng., 2023, 7, 036103 CrossRef CAS PubMed.
- G. Silvani, C. Basirun, H. Wu, C. Mehner, K. Poole, P. Bradbury and J. Chou, A 3D-Bioprinted Vascularized Glioblastoma-on-a-Chip for Studying the Impact of Simulated Microgravity as a Novel Pre-Clinical Approach in Brain Tumor Therapy, Adv. Ther., 2021, 4, 2100106 CrossRef.
- P. Datta, M. Dey, Z. Ataie, D. Unutmaz and I. T. Ozbolat, 3D bioprinting for reconstituting the cancer microenvironment, npj Precis. Oncol., 2020, 4, 18 CrossRef PubMed.
- R. Sharma, M. Restan Perez, V. A. da Silva, J. Thomsen, L. Bhardwaj, T. A. M. Andrade, A. Alhussan and S. M. Willerth, 3D bioprinting complex models of cancer, Biomater. Sci., 2023, 11, 3414–3430 RSC.
- Y. Kang, P. Datta, S. Shanmughapriya and I. T. Ozbolat, 3D Bioprinting of Tumor Models for Cancer Research, ACS Appl. Bio Mater., 2020, 3, 5552–5573 CrossRef CAS PubMed.
- N. Germain, M. Dhayer, S. Dekiouk and P. Marchetti, Current Advances in 3D Bioprinting for Cancer Modeling and Personalized Medicine, Int. J. Mol. Sci., 2022, 23, 3432 CrossRef CAS.
- S. Han, S. Kim, Z. Chen, H. K. Shin, S. Y. Lee, H. E. Moon, S. H. Paek and S. Park, 3D bioprinted vascularized tumour for drug testing, Int.
J. Mol. Sci., 2020, 21, 2993 CrossRef CAS PubMed.
- D. Nothdurfter, C. Ploner, D. C. Coraça-Huber, D. Wilflingseder, T. Müller, M. Hermann, J. Hagenbuchner and M. J. Ausserlechner, 3D bioprinted, vascularized neuroblastoma tumor environment in fluidic chip devices for precision medicine drug testing, Biofabrication, 2022, 14, 035002 CrossRef PubMed.
- S. B. Gugulothu, S. Asthana, S. Homer-Vanniasinkam and K. Chatterjee, Trends in Photopolymerizable Bioinks for 3D Bioprinting of Tumor Models, JACS Au, 2023, 3, 2086–2106 CrossRef CAS.
- C. G. Mueller, C. Gaiddon and A. Venkatasamy, Current Clinical and Pre-Clinical Imaging Approaches to Study the Cancer-Associated Immune System, Front. Immunol., 2021, 12, 716860 CrossRef CAS PubMed.
- M. Wu and M. A. Swartz, Modeling Tumor Microenvironments In Vitro, J. Biomech. Eng., 2014, 136, 0210111 CrossRef PubMed.
- Y. Yang, Y. Jia, Q. Yang and F. Xu, Engineering bio-inks for 3D bioprinting cell mechanical microenvironment, Int. J. Bioprint., 2022, 9, 632 CrossRef PubMed.
- A. V. Taubenberger, S. Girardo, N. Träber, E. Fischer-Friedrich, M. Kräter, K. Wagner, T. Kurth, I. Richter, B. Haller, M. Binner, D. Hahn, U. Freudenberg, C. Werner and J. Guck, 3D Microenvironment Stiffness Regulates Tumor Spheroid Growth and Mechanics via p21 and ROCK, Adv. Biosyst., 2019, 3, 1900128 CrossRef PubMed.
- D.-H. T. Nguyen, E. Lee, S. Alimperti, R. J. Norgard, A. Wong, J. J.-K. Lee, J. Eyckmans, B. Z. Stanger and C. S. Chen, A biomimetic pancreatic cancer on-chip reveals endothelial ablation via ALK7 signaling, Sci. Adv., 2023, 5, eaav6789 CrossRef PubMed.
- C. Liu, D. Lewin Mejia, B. Chiang, K. E. Luker and G. D. Luker, Hybrid collagen alginate hydrogel as a platform for 3D tumor spheroid invasion, Acta Biomater., 2018, 75, 213–225 CrossRef CAS PubMed.
- F. Conceição, D. M. Sousa, J. Loessberg-Zahl, A. R. Vollertsen, E. Neto, K. Søe, J. Paredes, A. Leferink and M. Lamghari, A metastasis-on-a-chip approach to explore the sympathetic modulation of breast cancer bone metastasis, Mater. Today Bio, 2022, 13, 100219 CrossRef PubMed.
-
Q. Yang, X. Lv, B. Gao, Y. Ji and F. Xu, in Advances in Applied Mechanics, ed. S. P. A. Bordas and D. S. Balint, Elsevier, 2021, vol. 54, pp. 285–318 Search PubMed.
-
R. Ogawa, Mechanobiology and Mechanotherapy in Tissue Engineering, in In Situ Tissue Regeneration, ed. S. J. Lee, J. J. Yoo and A. Atala, Academic Press, Boston, 2016, pp. 165–181 Search PubMed.
- Z. Zhou, T. Vessella, P. Wang, F. Cui, Q. Wen and H. S. Zhou, Mechanical cues in tumor microenvironment on chip, Biosens. Bioelectron.: X, 2023, 14, 100376 CAS.
- S. Chatterjee and T. F. Burns, Targeting heat shock proteins in cancer: A promising therapeutic approach, Int. J. Mol. Sci., 2017, 18, 1978 CrossRef PubMed.
- H.-Y. Wang, J. C.-M. Fu, Y.-C. Lee and P.-J. Lu, Hyperthermia Stress Activates Heat Shock Protein Expression via Propyl Isomerase 1 Regulation with Heat Shock Factor 1, Mol. Cell. Biol., 2013, 33, 4889–4899 CrossRef CAS PubMed.
- C. Hu, J. Yang, Z. Qi, H. Wu, B. Wang, F. Zou, H. Mei, J. Liu, W. Wang and Q. Liu, Heat shock proteins: Biological functions, pathological roles, and therapeutic opportunities, MedComm, 2022, 3, e161 CrossRef CAS.
- D. Schilling, A. Kühnel, S. Konrad, F. Tetzlaff, C. Bayer, J. Yaglom and G. Multhoff, Sensitizing tumor cells to radiation by targeting the heat shock response, Cancer Lett., 2015, 360, 294–301 CrossRef CAS PubMed.
- E. A. Repasky, S. S. Evans and M. W. Dewhirst, Temperature Matters! And Why It Should Matter to Tumor Immunologists, Cancer Immunol. Res., 2013, 1, 210–216 CrossRef CAS PubMed.
- A. S. Song, A. M. Najjar and K. R. Diller, Thermally Induced Apoptosis, Necrosis, and Heat Shock Protein Expression in Three-Dimensional Culture, J. Biomech. Eng., 2014, 136, 071006 CrossRef PubMed.
-
J. W. Valvano, Bioheat Transfer, Encyclopedia of Medical Devices and Instrumentation, 2006, pp. 188–197 Search PubMed.
- O. Levenspiel, The three mechanisms of heat transfer: Conduction, convection, and radiation, Engineering Flow and Heat Exchange, 2014, 179–210 Search PubMed.
- Yu. I. Luchakov and A. D. Nozdrachev, Mechanism of heat transfer in different regions of human body, Biol. Bull., 2009, 36, 53–57 CrossRef.
- M. Nabil, P. Decuzzi and P. Zunino, Modelling mass and heat transfer in nano-based cancer hyperthermia, R. Soc. Open Sci., 2015, 2, 150447 CrossRef CAS PubMed.
- M. U. Munir, Nanomedicine Penetration to Tumor: Challenges, and Advanced Strategies to Tackle This Issue, Cancers, 2022, 14, 2904 CrossRef CAS PubMed.
- V. Darvishi, M. Navidbakhsh and S. Amanpour, Heat and mass transfer in the hyperthermia cancer treatment by magnetic nanoparticles, Heat Mass Transfer, 2022, 58, 1029–1039 CrossRef CAS PubMed.
- A. Chanmugam, R. Hatwar and C. Herman, Thermal analysis of cancerous breast model, Int. Mech. Eng. Congr. Expo., 2012, 2012, 134–143 Search PubMed.
- A. Kazemi Alamouti, M. R. Habibi, M. Mazidi Sharfabadi and H. Akbari Lalimi, Numerical study on the effects of blood perfusion and tumor metabolism on tumor temperature for targeted hyperthermia considering a realistic geometrical model of head layers using the finite element method, SN Appl. Sci., 2021, 3, 462 CrossRef.
- C. W. Song, Effect of Local Hyperthermia on Blood Flow and Microenvironment: A Review, Cancer Res., 1984, 44, 4721s–4730s CAS.
- C. W. Song, A. Lokshina, J. G. Rhee, M. Patten and S. H. Levitt, Implication of Blood Flow in Hyperthermic Treatment of Tumors, IEEE Trans. Biomed. Eng., 1984, BME-31, 9–16 CrossRef.
- H. H. Pennes, Analysis of Tissue and Arterial Blood Temperatures in the Resting Human Forearm, J. Appl. Physiol., 1948, 1, 93–122 CrossRef CAS PubMed.
- A. Andreozzi, L. Brunese, M. Iasiello, C. Tucci and G. P. Vanoli, Modeling Heat Transfer in Tumors: A Review of Thermal Therapies, Ann. Biomed. Eng., 2019, 47, 676–693 CrossRef PubMed.
- J. Hristov, Bio-Heat Models Revisited: Concepts, Derivations, Nondimensalization and Fractionalization Approaches, Front. Phys., 2019, 1224, 012001 Search PubMed.
- S. Pati, A. Borah, M. P. Boruah and P. R. Randive, Critical review on local thermal equilibrium and local thermal non-equilibrium approaches for the analysis of forced convective flow through porous media, Int. Commun. Heat Mass Transfer, 2022, 132, 105889 CrossRef CAS.
- M. Parhizi, M. Torabi and A. Jain, Local thermal non-equilibrium (LTNE) model for developed flow in porous media with spatially-varying Biot number, Int. J. Heat Mass Transfer, 2021, 164, 120538 CrossRef.
- K.-C. Liu and Y.-S. Chen, Analysis of Bio-Heat Transfer Using the Equation From the DPL Model, J. Chin. Soc. Mech. Eng., 2012, 33, 133–140 CrossRef.
- Z. Shomali, R. Kovács, P. Ván, I. V. Kudinov and J. Ghazanfarian, Lagging heat models in thermodynamics and bioheat transfer: a critical review, Continuum Mech. Thermodyn., 2022, 34, 637–679 CrossRef CAS.
- Y. Zhang, Generalized dual-phase lag bioheat equations based on nonequilibrium heat transfer in living biological tissues, Int. J. Heat Mass Transfer, 2009, 52, 4829–4834 CrossRef.
- A. Dinda, J. Acharya, D. Bhanja and S. Nath, Local thermal non-equilibrium bioheat transfer model for interstitial hyperthermia treatment of tumour cell: A numerical approach, J. Therm. Biol., 2022, 110, 103368 CrossRef PubMed.
- A. Andreozzi, L. Brunese, M. Iasiello, C. Tucci and G. Peter Vanoli, Variable porosity-based bioheat model vs variable perfusion-based Pennes’ equation: A comparison with in vivo experimental data, Therm. Sci. Eng. Prog., 2022, 35, 101469 CrossRef.
- M. Ragab, A. E. Abouelregal, H. F. AlShaibi and R. A. Mansouri, Heat Transfer in Biological Spherical Tissues during Hyperthermia of Magnetoma, Biology, 2021, 10, 1259 CrossRef PubMed.
- S. R. Gunakala, V. M. Job, S. Sakhamuri, P. V. S. N. Murthy and B. V. Chowdary, Numerical study of blood perfusion and nanoparticle transport in prostate and muscle tumours during intravenous magnetic hyperthermia, Alexandria Eng. J., 2021, 60, 859–876 CrossRef.
- Y.-D. Tang, J. Zou, R. C. C. Flesch and T. Jin, Effect of injection strategy for nanofluid transport on thermal damage behavior inside biological tissue during magnetic hyperthermia, Int. Commun. Heat Mass Transfer, 2022, 133, 105979 CrossRef CAS.
- N. Mahesh, N. Singh and P. Talukdar, A mathematical model for understanding nanoparticle biodistribution after intratumoral injection in cancer tumors, J. Drug Delivery Sci. Technol., 2022, 68, 103048 CrossRef CAS.
- S. C. Brüningk, P. Ziegenhein, I. Rivens, U. Oelfke and G. ter Haar, A cellular automaton model for spheroid response to radiation and hyperthermia treatments, Sci. Rep., 2019, 9, 1–12 CrossRef PubMed.
- N. Mahesh, N. Singh and P. Talukdar, A mathematical model of intratumoral infusion, particle distribution and heat transfer in cancer tumors: In-silico investigation of magnetic nanoparticle hyperthermia, Int. J. Therm. Sci., 2023, 183, 107887 CrossRef CAS.
- M. Sefidgar, L. Alinezhad, E. Bashooki and P. Shojaee, Effect of different dynamic microvasculature in a solid tumor with the necrotic region during magnetic hyperthermia: An in-silico study, Int. J. Heat Mass Transfer, 2022, 189, 122662 CrossRef.
- M. Suleman and S. Riaz, 3D in silico study of magnetic fluid hyperthermia of breast tumor using Fe3O4 magnetic nanoparticles, J. Therm. Biol., 2020, 91, 102635 CrossRef CAS PubMed.
- H. Chen, K. Wang, Z. Du, W. Liu and Z. Liu, Predicting the thermophysical properties of skin tumor based on the surface temperature and deep learning, Int. J. Heat Mass Transfer, 2021, 180, 121804 CrossRef.
- G. R. Fatigate, M. Lobosco and R. F. Reis, A 3D Approach Using a Control Algorithm to Minimize the Effects on the Healthy Tissue in the Hyperthermia for Cancer Treatment, Entropy, 2023, 25, 684 CrossRef PubMed.
- C. Tucci, M. Trujillo, E. Berjano, M. Iasiello, A. Andreozzi and G. P. Vanoli, Pennes’ bioheat equation vs. porous media approach in computer modeling of radiofrequency tumor ablation, Sci. Rep., 2021, 11, 5272 CrossRef CAS PubMed.
- A. Andreozzi, L. Brunese, M. Iasiello, C. Tucci and G. Peter Vanoli, Bioheat transfer in a spherical biological tissue: A comparison among various models, J. Phys.: Conf. Ser., 2019, 1224, 012001 CrossRef.
- S. Hassanpour and A. Saboonchi, Validation of local thermal equilibrium assumption in a vascular tissue during interstitial hyperthermia treatment, J. Mech. Med. Biol., 2017, 17, 1750087 CrossRef.
- J. Dutta, B. Kundu and S.-J. Yook, Three-dimensional thermal assessment in cancerous tumors based on local thermal non-equilibrium approach for hyperthermia treatment, Int. J. Therm. Sci., 2021, 159, 106591 CrossRef.
- A. Andreozzi, L. Brunese, M. Iasiello, C. Tucci and G. P. Vanoli, Numerical analysis of the pulsating heat source effects in a tumor tissue, Comput. Methods Programs Biomed., 2021, 200, 105887 CrossRef PubMed.
- E. Majchrzak and M. Stryczyński, Dual-phase lag model of heat transfer between blood vessel and biological tissue, Math. Biosci. Eng., 2021, 18, 1573–1589 Search PubMed.
- P. Kumar, D. Kumar and K. N. Rai, A numerical study on dual-phase-lag model of bio-heat transfer during hyperthermia treatment, J. Therm. Biol., 2015, 49–50, 98–105 CrossRef CAS PubMed.
- T. Kumari, D. Kumar, K. N. Rai and S. K. Singh, Numerical solution of DPL heat transfer model in multi-layer biological skin tissue of the living body during hyperthermia treatment, Mech. Based Des. Struct. Mach., 2023, 51, 159–178 CrossRef.
- A. Bera, S. Dutta, J. C. Misra and G. C. Shit, Computational modeling of the effect of blood flow and dual phase lag on tissue temperature during tumor treatment by magnetic hyperthermia, Math. Comput. Simul., 2021, 188, 389–403 CrossRef.
- J. Pape, M. Emberton and U. Cheema, 3D Cancer Models: The Need for a Complex Stroma, Compartmentalization and Stiffness, Front. Bioeng. Biotechnol., 2021, 9, 1–8 CrossRef.
- E. Darrigues, Z. A. Nima, R. J. Griffin, J. M. Anderson, A. S. Biris and A. Rodriguez, 3D cultures for modeling nanomaterial-based photothermal therapy, Nanoscale Horiz., 2020, 5, 400–430 RSC.
- D. P. N. Gonçalves, R. D. Rodriguez, T. Kurth, L. J. Bray, M. Binner, C. Jungnickel, F. N. Gür, S. W. Poser, T. L. Schmidt, D. R. T. Zahn, A. Androutsellis-Theotokis, M. Schlierf and C. Werner, Enhanced targeting of invasive glioblastoma cells by peptide-functionalized gold nanorods in hydrogel-based 3D cultures, Acta Biomater., 2017, 58, 12–25 CrossRef PubMed.
- R. Lima-Sousa, C. G. Alves, B. L. Melo, A. F. Moreira, A. G. Mendonça, I. J. Correia and D. de Melo-Diogo, Poly(2-ethyl-2-oxazoline) functionalized reduced graphene oxide: Optimization of the reduction process using dopamine and application in cancer photothermal therapy, Mater. Sci. Eng., C, 2021, 130, 112468 CrossRef CAS PubMed.
- B. McCarthy, A. Cudykier, R. Singh, N. Levi-Polyachenko and S. Soker, Semiconducting polymer nanoparticles for photothermal ablation of colorectal cancer organoids, Sci. Rep., 2021, 11, 1532 CrossRef CAS PubMed.
- D. Kalinowska, I. Grabowska-Jadach, M. Liwinska, M. Drozd, M. Pietrzak, A. Dybko and Z. Brzozka, Studies on effectiveness of PTT on 3D tumor model under microfluidic conditions using aptamer-modified nanoshells, Biosens. Bioelectron., 2019, 126, 214–221 CrossRef CAS PubMed.
- C. Bastiancich, A. Da Silva and M.-A. Estève, Photothermal Therapy for the Treatment of Glioblastoma: Potential and Preclinical Challenges, Front. Oncol., 2021, 10, 610356 CrossRef PubMed.
- J. M. Lee, J. W. Choi, C. D. Ahrberg, H. W. Choi, J. H. Ha, S. G. Mun, S. J. Mo and B. G. Chung, Generation of tumor spheroids using a droplet-based microfluidic device for photothermal therapy, Microsyst. Nanoeng., 2020, 6, 52 CrossRef CAS PubMed.
- C. Christie, S. J. Madsen, Q. Peng and H. Hirschberg, Photothermal therapy employing gold nanoparticle-loaded macrophages as delivery vehicles: Comparing the efficiency of nanoshells versus nanorods, J. Environ. Pathol., Toxicol. Oncol., 2017, 36, 229–235 CrossRef PubMed.
- Y. Li, P. Feng, C. Wang, W. Miao and H. Huang, Black phosphorus nanophototherapeutics with enhanced stability and safety for breast cancer treatment, Chem. Eng. J., 2020, 400, 125851 CrossRef CAS.
- J. Liu, K. Yi, Q. Zhang, H. Xu, X. Zhang, D. He, F. Wang and X. Xiao, Strong Penetration-Induced Effective Photothermal Therapy by Exosome-Mediated Black Phosphorus Quantum Dots, Small, 2021, 17, 2104585 CrossRef CAS PubMed.
- L. Beola, L. Asín, R. M. Fratila, V. Herrero, J. M. De La Fuente, V. Grazú and L. Gutiérrez, Dual Role of Magnetic Nanoparticles as Intracellular Hotspots and Extracellular Matrix Disruptors Triggered by Magnetic Hyperthermia in 3D Cell Culture Models, ACS Appl. Mater. Interfaces, 2018, 10, 44301–44313 CrossRef CAS PubMed.
- X. Peng, B. Wang, Y. Yang, Y. Zhang, Y. Liu, Y. He, C. Zhang and H. Fan, Liver Tumor Spheroid Reconstitution for Testing Mitochondrial Targeted Magnetic Hyperthermia Treatment, ACS Biomater. Sci. Eng., 2019, 5, 1635–1644 CrossRef CAS PubMed.
- J. Palzer, B. Mues, R. Goerg, M. Aberle, S. S. Rensen, S. W. M. Olde Damink, R. D. W. Vaes, T. Cramer, T. Schmitz-Rode, U. P. Neumann, I. Slabu and A. A. Roeth, Magnetic Fluid Hyperthermia as Treatment Option for Pancreatic Cancer Cells and Pancreatic Cancer Organoids, Int. J. Nanomed., 2021, 16, 2965–2981 CrossRef PubMed.
- P. Kaur, M. D. Hurwitz, S. Krishnan and A. Asea, Combined Hyperthermia and Radiotherapy for the Treatment of Cancer, Cancers, 2011, 3, 3799–3823 CrossRef CAS PubMed.
- C. Tydings, K. V. Sharma, A. Kim and P. S. Yarmolenko, Emerging hyperthermia applications for pediatric oncology, Adv. Drug Delivery Rev., 2020, 163–164, 157–167 CrossRef CAS PubMed.
- M. Dunne, M. Regenold and C. Allen, Hyperthermia can alter tumor physiology and improve chemo- and radio-therapy efficacy, Adv. Drug Delivery Rev., 2020, 163–164, 98–124 CrossRef CAS PubMed.
- N. R. Datta, S. G. Ordóñez, U. S. Gaipl, M. M. Paulides, H. Crezee, J. Gellermann, D. Marder, E. Puric and S. Bodis, Local hyperthermia combined with radiotherapy and-/or chemotherapy: Recent advances and promises for the future, Cancer Treat. Rev., 2015, 41, 742–753 CrossRef CAS PubMed.
- H. Tian, T. Zhang, S. Qin, Z. Huang, L. Zhou, J. Shi, E. C. Nice, N. Xie, C. Huang and Z. Shen, Enhancing the therapeutic efficacy of nanoparticles for cancer treatment using versatile targeted strategies, J. Hematol. Oncol., 2022, 15, 132 CrossRef PubMed.
- Z. Edis, J. Wang, M. K. Waqas, M. Ijaz and M. Ijaz, Nanocarriers-Mediated Drug Delivery Systems for Anticancer Agents: An Overview and Perspectives, Int. J. Nanomed., 2021, 16, 1313–1330 CrossRef.
- T. Dube, U. B. Kompella and J. J. Panda, Near infrared triggered chemo-PTT-PDT effect mediated by glioma directed twin functional-chimeric peptide-decorated gold nanoroses, J. Photochem. Photobiol., B, 2022, 228, 112407 CrossRef CAS PubMed.
- H.-S. Choe, M. J. Shin, S. G. Kwon, H. Lee, D. K. Kim, K. U. Choi, J.-H. Kim and J. H. Kim, Yolk–Shell-Type Gold Nanoaggregates for Chemo- and Photothermal Combination Therapy for Drug-Resistant Cancers, ACS Appl. Mater. Interfaces, 2021, 13, 53519–53529 CrossRef CAS PubMed.
- S. Kidd, E. Spaeth, J. L. Dembinski, M. Dietrich, K. Watson, A. Klopp, V. L. Battula, M. Weil, M. Andreeff and F. C. Marini, Direct evidence of mesenchymal stem cell tropism for tumor and wounding microenvironments using in vivo bioluminescent imaging, Stem Cells, 2009, 27, 2614–2623 CrossRef CAS PubMed.
- M. Luo, Y. Zhou, N. Gao, W. Cheng, X. Wang, J. Cao, X. Zeng, G. Liu and L. Mei, Mesenchymal stem cells transporting black phosphorus-based biocompatible nanospheres: Active trojan horse for enhanced photothermal cancer therapy, Chem. Eng. J., 2020, 385, 123942 CrossRef CAS.
- M. Tutter, C. Schug, K. A. Schmohl, S. Urnauer, C. Kitzberger, N. Schwenk, M. Petrini, C. Zach, S. Ziegler, P. Bartenstein, W. A. Weber, G. Multhoff, E. Wagner, L. H. Lindner, P. J. Nelson and C. Spitzweg, Regional Hyperthermia Enhances Mesenchymal Stem Cell Recruitment to Tumor Stroma: Implications for Mesenchymal Stem Cell-Based Tumor Therapy, Mol. Ther., 2020, 29, 788–803 CrossRef PubMed.
- S. Ullah, K. Seidel, S. Türkkan, D. P. Warwas, T. Dubich, M. Rohde, H. Hauser, P. Behrens, A. Kirschning, M. Köster and D. Wirth, Macrophage entrapped silica coated superparamagnetic iron oxide particles for controlled drug release in a 3D cancer model, J. Controlled Release, 2019, 294, 327–336 CrossRef CAS PubMed.
- S. Kwon, S. Jung and S. H. Baek, Combination Therapy of Radiation and Hyperthermia, Focusing on the Synergistic Anti-Cancer Effects and Research Trends, Antioxidants, 2023, 12, 924 CrossRef CAS PubMed.
- J. Ni, H. Xu, Y. Zhong, Y. Zhou and S. Hu, Activatable UCL/CT/MR-enhanced in vivo imaging-guided radiotherapy and photothermal therapy, J. Mater. Chem. B, 2022, 10, 549–561 RSC.
- J. B. Mamani, B. S. Marinho, G. N. de, A. Rego, M. P. Nucci, F. Alvieri, R. S. Dos Santos, J. V. M. Ferreira, F. A. de Oliveira and L. F. Gamarra, Magnetic hyperthermia therapy in glioblastoma tumor on-a-Chip model, Einstein, 2020, 18, eAO4954 CrossRef PubMed.
- F. Hadi, S. Tavakkol, S. Laurent, V. Pirhajati, S. R. Mahdavi, A. Neshastehriz and A. Shakeri-Zadeh, Combinatorial effects of radiofrequency hyperthermia and radiotherapy in the presence of magneto-plasmonic nanoparticles on MCF-7 breast cancer cells, J. Cell. Physiol., 2019, 234, 20028–20035 CrossRef CAS PubMed.
- H. Lin, L. Yin, B. Chen and Y. Ji, Design of functionalized magnetic silica multi-core composite nanoparticles for synergistic magnetic hyperthermia/radiotherapy in cancer cells, Colloids Surf., B, 2022, 219, 112814 CrossRef CAS PubMed.
- H. Esmaeili Govarchin Ghaleh, L. Zarei, B. Mansori Motlagh and N. Jabbari, Using CuO nanoparticles and hyperthermia in radiotherapy of MCF-7 cell line: synergistic effect in cancer therapy, Artif. Cells, Nanomed., Biotechnol., 2019, 47, 1396–1403 CrossRef CAS PubMed.
- S. E. Minaei, S. Khoei, S. Khoee and S. R. Mahdavi, Sensitization of glioblastoma cancer cells to radiotherapy and magnetic hyperthermia by targeted temozolomide-loaded magnetite tri-block copolymer nanoparticles as a nanotheranostic agent, Life Sci., 2022, 306, 120729 CrossRef CAS PubMed.
- A. Tchoryk, V. Taresco, R. H. Argent, M. Ashford, P. R. Gellert, S. Stolnik, A. Grabowska and M. C. Garnett, Penetration and Uptake of Nanoparticles in 3D Tumor Spheroids, Bioconjugate Chem., 2019, 30, 1371–1384 CrossRef CAS PubMed.
- L. Zhang, Y. Wang, D. Yang, W. Huang, P. Hao, S. Feng, D. Appelhans, T. Zhang and X. Zan, Shape Effect of Nanoparticles on Tumor Penetration in Monolayers Versus Spheroids, Mol. Pharmaceutics, 2019, 16, 2902–2911 CrossRef CAS PubMed.
- H. Villuendas, C. Vilches and R. Quidant, Standardization of In Vitro Studies for Plasmonic Photothermal therapy, ACS Nanosci. Au, 2023, 3, 347–352 CrossRef CAS PubMed.
- E. De Jaeghere, E. De Vlieghere, J. Van Hoorick, S. Van Vlierberghe, G. Wagemans, L. Pieters, E. Melsens, M. Praet, J. Van Dorpe, M. N. Boone, R. Ghobeira, N. De Geyter, M. Bracke, C. Vanhove, S. Neyt, G. Berx, B. G. De Geest, P. Dubruel, H. Declercq, W. Ceelen and O. De Wever, Heterocellular 3D scaffolds as biomimetic to recapitulate the tumor microenvironment of peritoneal metastases in vitro and in vivo, Biomaterials, 2018, 158, 95–105 CrossRef CAS PubMed.
- M. Zhu, Z. Sun and C. K. Ng, Image-guided thermal ablation with MR-based thermometry, Quant. Imaging Med. Surg., 2017, 7, 356–368 CrossRef PubMed.
- J. H. Hankiewicz, J. A. Stoll, J. Stroud, J. Davidson, K. L. Livesey, K. Tvrdy, A. Roshko, S. E. Russek, K. Stupic, P. Bilski, R. E. Camley and Z. J. Celinski, Nano-sized ferrite particles for magnetic resonance imaging thermometry, J. Magn. Magn. Mater., 2019, 469, 550–557 CrossRef CAS.
- J. H. Hankiewicz, Z. Celinski, K. F. Stupic, N. R. Anderson and R. E. Camley, Ferromagnetic particles as magnetic resonance imaging temperature sensors, Nat. Commun., 2016, 7, 1–8 Search PubMed.
- K.-J. Janssen, J. Zhong, T. Viereck, M. Schilling and F. Ludwig, Quantitative temperature visualization with single harmonic-based magnetic particle imaging, J. Magn. Magn. Mater., 2022, 563, 169915 CrossRef CAS.
- K. Xue, C. Wang, J. Wang, S. Lv, B. Hao, C. Zhu and B. Z. Tang, A Sensitive and Reliable Organic Fluorescent Nanothermometer for Noninvasive Temperature Sensing, J. Am. Chem. Soc., 2021, 143, 14147–14157 CrossRef CAS PubMed.
- L. Meng, S. Jiang, M. Song, F. Yan, W. Zhang, B. Xu and W. Tian, TICT-Based Near-Infrared Ratiometric Organic Fluorescent Thermometer for Intracellular Temperature Sensing, ACS Appl. Mater. Interfaces, 2020, 12, 26842–26851 CrossRef CAS PubMed.
-
R. Piñol, C. D. S. Brites, N. J. Silva, L. D. Carlos and A. Millán, Nanomaterials for Magnetic and Optical Hyperthermia Applications, in Micro and Nano Technologies, ed. R. M. Fratila and J. M. De La Fuente, Elsevier, 2019, pp. 139–172 Search PubMed.
- E. Umut, M. Coşkun, F. Pineider, D. Berti and H. Güngüne
, Nickel ferrite nanoparticles for simultaneous use in magnetic resonance imaging and magnetic fluid hyperthermia, J. Colloid Interface Sci., 2019, 550, 199–209 CrossRef CAS PubMed.
- H. Yan, W. Shang, X. Sun, L. Zhao, J. Wang, Z. Xiong, J. Yuan, R. Zhang, Q. Huang, K. Wang, B. Li, J. Tian, F. Kang and S.-S. Feng, “All-in-One” Nanoparticles for Trimodality Imaging-Guided Intracellular Photo-magnetic Hyperthermia Therapy under Intravenous Administration, Adv. Funct. Mater., 2018, 28, 1705710 CrossRef.
- K.-W. Hu, T.-M. Liu, K.-Y. Chung, K.-S. Huang, C.-T. Hsieh, C.-K. Sun and C.-S. Yeh, Efficient Near-IR Hyperthermia and Intense Nonlinear Optical Imaging Contrast on the Gold Nanorod-in-Shell Nanostructures, J. Am. Chem. Soc., 2009, 131, 14186–14187 CrossRef CAS PubMed.
- X. Yang, G. Shao, Y. Zhang, W. Wang, Y. Qi, S. Han and H. Li, Applications of Magnetic Particle Imaging in Biomedicine: Advancements and Prospects, Front. Physiol., 2022, 13, 898426 CrossRef PubMed.
- M. Parmala, M. Eriksson, M. Rytioja, J. Tanttu and M. Köhler, Temperature measurement in human fat with T2 imaging, J. Magn. Reson. Imaging, 2016, 43, 1171–1178 CrossRef PubMed.
- N. Todd, U. Vyas, J. De Bever, A. Payne and D. L. Parker, Reconstruction of fully three-dimensional high spatial and temporal resolution MR temperature maps for retrospective applications, Magn. Reson. Med., 2012, 67, 724–730 CrossRef PubMed.
- R. Oliveira-Silva, R. A. Pereira, F. M. Silva, V. M. Gaspar, A. Ibarra, Á. Millán, F. L. Sousa, J. F. Mano and N. J. O. Silva, Temperature-responsive nanomagnetic logic gates for cellular hyperthermia, Mater. Horiz., 2019, 6, 524–530 RSC.
- V. Rieke and K. Butts Pauly, MR thermometry, J. Magn. Reson. Imaging, 2008, 27, 376–390 CrossRef PubMed.
|
This journal is © The Royal Society of Chemistry 2024 |