DOI:
10.1039/D3NH00461A
(Review Article)
Nanoscale Horiz., 2024,
9, 384-406
The potential of graphene coatings as neural interfaces
Received
15th October 2023
, Accepted 4th January 2024
First published on 10th January 2024
Abstract
Recent advances in nanotechnology design and fabrication have shaped the landscape for the development of ideal cell interfaces based on biomaterials. A holistic evaluation of the requirements for a cell interface is a highly complex task. Biocompatibility is a crucial requirement which is affected by the interface's properties, including elemental composition, morphology, and surface chemistry. This review explores the current state-of-the-art on graphene coatings produced by chemical vapor deposition (CVD) and applied as neural interfaces, detailing the key properties required to design an interface capable of physiologically interacting with neural cells. The interfaces are classified into substrates and scaffolds to differentiate the planar and three-dimensional environments where the cells can adhere and proliferate. The role of specific features such as mechanical properties, porosity and wettability are investigated. We further report on the specific brain-interface applications where CVD graphene paved the way to revolutionary advances in biomedicine. Future studies on the long-term effects of graphene-based materials in vivo will unlock even more potentially disruptive neuro-applications.
1. How to design a cell interface
Understanding the intermolecular mechanisms of the multitude of cell types in the human body has been an extensive effort in the scientific community. This understanding is crucial to the development of key areas of knowledge, such as medicine and biocybernetics. However, studying the causes and effects of cellular behavior in vivo is extremely challenging due to the numerous physiological signals and responses that occur in a living organism. To simplify the problem, scientists have turned to studying cellular behavior in a controlled and simplified environment in vitro, where the amount and variety of stimuli the cells receive, and trigger are limited. While this approach can provide a more thorough evaluation, ensuring the cell's survivability and physiology in vitro is a challenge of its own. Among all the different cell types and tissues, neural cells have been one of the most extensively studied subject of scientific research, with a focus on biomaterials and biomedical engineering techniques. The interactions within the inner workings of the brain are still poorly understood after decades of research. Growing neuronal networks in vitro is a viable method to simplify the physiological environment for academic investigation, allowing the experimentation of stimulation and/or recording of neural activity,1 together with the designing of neural networks for neuromorphic engineering.2 Furthermore, neurons in vivo are infamous for their slow and unreliable regeneration, which makes the effects of neurological afflictions devastating and often permanent.3 In addition, in the case of nerve rupture, the damage is further amplified by secondary glial mechanisms that inhibit any cellular regeneration (e.g., ischemia, post-lesion scar barrier, and free-radical formation4,5). Invasive surgery of the nervous system can also lead to the loss of neuronal tissue, either due to damage caused during intrusion into the nervous system or by the removal of tumors and surrounding tissues. Thus, there is great medical interest in developing cell replacement therapies for nerve trauma and neurodegenerative disorders through minimally invasive approaches.
The foremost, obvious requirement of any cell interface is biocompatibility, which means that the interface material or structure must be non-toxic and non-reactive to the surrounding biological environment. Additionally, it should not induce an immune response, inflammation, or adverse reactions when in contact with living tissues or cells. Achieving biocompatibility involves ensuring that the interface is compatible with the specific physiological and biochemical conditions of the target biological system, including factors like pH, temperature, and moisture levels. Beginning with fundamental biological interactions that dictate biocompatibility, an effective cellular adhesion is fundamental for cell–cell physiology and communication (e.g., differentiation, cell cycle, migration, survival).6–8 The quality of the cell adhesion to the substrate will determine their fate in terms of capacity to grow or to undertake a programmed cell death, such as apoptosis or anoikis (specific cell programmed death induced by inappropriate cell adhesion).9 Cells adhere to the surface by physical interactions between extracellular domain of plasma membrane proteins and the interface. These interactions take place at localized sites called focal adhesion (FA) points, at which transmembrane proteins, such as integrins, anchor the plasma membrane to the extracellular matrix (ECM) or to neighboring cells. These binding sites are also responsible for cell migration and bidirectional transmittance of regulatory signals, opening a pathway of communication between the intracellular cytoskeleton and ECM.7,10In vitro, the process of cell adhesion can be divided in three stages (Fig. 1). (i) First, the initial attachment of the cell body to the substrate by electrostatic forces happens; (ii) then, flattening and spreading of the cell body occur; and (iii) finally full spreading and structural organization of the actin and cytoskeleton filament with the formation of FA sites complete the process.7,10 FA points serve as a pathway of mechanical force transmission, both by deforming the cell in its last stage of adhesion and by exerting force on the ECM. FA points are thus indispensable in cell migration and maintenance of cell shape and in vivo and they are also involved in muscle contraction and the movement of cilia and flagella.11,12 Moreover, in vivo, reduced, or defective cellular adhesion is related to a wide selection of pathologies, and it allows malignant cancer cells to circumvent the body's regulations.7,13 Other than apoptosis and anoikis, there are other adverse effects to avoid when designing a cell–material interface, as several physical and chemical interactions might lead to accidental cell death (i.e., necrosis). Necrosis is usually connected to the physical disruption of the cell membrane (e.g., protruding edges from the substrate piercing the cell), which can make the cell release its intracellular contents in the extracellular environment, with a detrimental effect on the tissue.14 Whilst necrosis can be the result of undesirable physical interactions between the cells and the material, apoptosis directly depends on chemical interactions with the substrate. Unregulated biochemical events in the ECM can lead to apoptosis, and as it cannot be stopped once it has begun, the substrate must be designed in consideration of this. For instance, blocking/reduction of nutrient uptakes by cells will cause a disturbance of the redox equilibrium, which in turn might activate a systematic manifestation of reactive oxygen species (ROS). ROS are peroxides and free radicals in the cell, byproducts of the metabolism of oxygen, and harmless at low stationary levels. However, if the ROS levels rise sharply, it may cause extreme cell damage, known as oxidative stress. Oxidative stress disrupts cellular signaling and causes DNA breakage, leading to apoptosis.6,15 The biophysical relationship of a cell with its extracellular environment is a dynamic one, as several extracellular stimuli can affect or even define cell fate, such as adhesion, proliferation, and differentiation.16 In this framework, cutting-edge manufacturing techniques that enable the design, fabrication, and functionalization of materials at the nanoscale have been instrumental, since materials engineering allows the fabrication of interfaces that can provide extracellular stimuli (i.e., surface topography, spatially defined biochemical cues, stiffness gradient, etc.).16–18 Ranking highly among these nanomaterials as potential candidates for new cell replacement therapy materials are atomically thin graphene sheets. There is evidence that morphologic and electronic properties of the interface, together with surface characteristics, such as wettability and active chemical groups, play a key part in the bio-interactions.6 These interactions are essential in modulating or tailoring cellular responses by determining protein exchanges, adhesion qualities, cellular growth, proliferation, migration, and in some cases differentiation.19 Nonetheless, it needs to be considered that the interaction with cell and their environments might directly affect the material and its properties, due to humidity and temperature conditions,20,21 possible aggressive pH setting,22 mechanical corrosion due to movements (in case of joint implants),23 and enzymes interactions.24 All these actions will influence the correct functionality of the interface, strike its durability, and trigger its degradation.
 |
| Fig. 1 Schematic diagram of the adhesion stages for single cells in vitro. | |
In a neurological setting, the necessary procedures become more arduous. The human nervous system components can be divided into two types of cells, neurons and glial cells. Neurons are the ones responsible for transmitting chemical or electrical signals, whilst glial play a major role in neuron maintenance. Its tasks include regulating neurotransmitters (tri-partite synapsis), delivering nutrition, and detecting and responding to injury. This complexity makes it a challenge to replicate the appropriate in vitro conditions for neural tissue and the bio-interactions of the newly designed interface might present different effects depending on the cellular subpopulation tested.25
2. Types of cell interfaces
In this review, we will consider substrates and scaffolds as two kinds of interfaces used to support the growth and function of cells in the context of cell/tissue culture. Substrates provide a two-dimensional (2D) flat surface for cells to attach to and grow on, as mono- or multi-layers. They can be made of various materials such as plastic, glass, or metals, and can be coated with proteins, peptides, or other bioactive molecules to promote cell attachment and growth. Scaffolds are three-dimensional (3D) structures that provide physical support for cells, which can grow in a complex arrangement and mimic the in vivo tissue organization. They can be made of biodegradable materials such as natural polymers (e.g., collagen, fibrin) or synthetic polymers (e.g., poly(lactic-co-glycolic acid), polylactic acid), and can be designed to mimic the architecture and mechanical properties of native tissues. Scaffolds are commonly used in 3D cell culture and tissue engineering applications, where they can be seeded with cells and provide a framework for the cells to grow and differentiate into functional tissue. Substrates allow the construction of simple and low-cost environments for cell cultures, which cannot fully replicate the natural structures of the tissue.26 On the contrary, scaffolds can offer physical support for cells to attach, proliferate and differentiate, as well as a microenvironment, which overall closely mimics the natural tissue.27 In this regard, the properties of each support must be tailored according to the respective purpose. The growing concern in this context is spurring the research of materials for bio-interfaces capable of bridging the world of biology and microelectronics with a focus on those materials integrating different relevant attributes.28 Since its conception, there has been ample research for graphene in bio-interfaces.29,30 Graphene and its derivatives are relished for their versatility and functionalization methods. This review focuses on pristine and functionalized graphene coatings made by chemical vapor deposition (CVD) on (i) flat substrates, onto which the cells develop along the plane, and (ii) 3D scaffolds, which allow complex network formations. Specifically, we present an updated analysis of the CVD graphene applications for neural interfaces, depicting the crucial characteristics they should possess to safely interact with brain tissues.
2.1. Substrates
A typical cell culture is carried out in a serum-containing medium, and a protein layer is naturally formed before the cells can interact with the surface. Therefore, when studying the effect that the surface has on cell responses it is crucial to take into consideration that this protein layer acts as an intermediary between cells and surface.31 Among all parameters, the hydrophilicity of the surface has a profound impact on the viability of living systems. In vitro studies showed that the wettability of the surface is crucial to control cellular adhesion.8,32 This is because, in the early stage of attachment, physicochemical linkages in the cell–substrate interface occur through ionic forces and the adsorption of these serum proteins.33 One example comes from fibronectin, a glycoprotein that plays a major role in cell adhesion and proliferation, and whilst it binds more to hydrophobic than hydrophilic surfaces,34,35 in the presence of cells, fibronectin has been shown to accumulate more on hydrophilic surfaces.34,36 In hydrophilic surfaces, there is an increase of fibronectin, accompanied by a higher proliferation and spreading of the cells. One hypothesis to explain this phenomenon is related to the exchange of proteins on the interface, which is expected to be much faster on hydrophilic surfaces.34 When the cells interact with the protein-adsorbed surface, they remove and reorganize the adsorbed fibronectin. Cells reorganize the protein into patches and fibrils beneath the cells for the development of FA sites.37 As the removal of the fibronectin depends on the exchange of plasma proteins on the surface, it makes sense that due to stronger fibronectin adsorption on the hydrophobic surface, and consequently, decreased fibronectin reorganization by the cells, leading to lower proliferation and growth on hydrophobic surfaces.36,38 This may explain why weak interactions between cells and surfaces relate to low wettability (i.e., low surface energy) and possibly result in cell death.34 In long-time frames, cell adhesion and growth will also depend on nutritive and mitogenic factors present in the serum.39 Furthermore, studies correlate van der Waals interactions to be the major force of attraction between cell and substrate, with cell adhesion increasing with increased ionic strength. This is due to a more compressed electrostatic double layer, that acts as a repulsion force, allowing a closer attachment of the cells to the solid surfaces and hence an increased effect of the van der Waals attraction, leading to better cellular adhesion. At high ionic strength, the van der Waals forces overpower the double layer repulsion completely,40 possibly due to aromatic amino acids ununiformly distributed towards the membrane interface, which are involved in hydrogen bonding with lipid carbonyl groups or interfacial water molecules.41 Modulating the hydrophilicity of the surface of the biomaterial is a common tactic in designing cell substrates for in vitro studies. This, however, tends not to be the case for cell scaffolds as these structures promote cell infiltration and integration within their porous matrix over cell adhesion.
Whilst considering the hydrophilicity of the surface of great importance in planning a cell/substrate interface, surface topography features (e.g., roughness, elasticity, porosity, grain size), and shear stress have proved to have a detrimental influence on cellular behavior (Fig. 2a).42,43 Altogether, these physical characteristics are considered sufficient to direct and influence cell behavior (e.g., cell adhesion, migration), without the need to rely on surface chemical modifications.44 In addition, 2D substrates should also be mechanically stable and do not significantly deform under the forces exerted by cells during attachment and spreading.45 The general view is that the extent of contractile force generation depends on the cell's mechano-sensing abilities.46 That is, cells demonstrate mechano-transductive responses by probing the stiffness of their substrate. However, recent studies show that the contractile forces generated are indeed independent of the stiffness of the substrate.46,47 Nonetheless, contractile forces directly influence the material, possibly compromising its mechanical strength by deformation and redefinition of its surface chemistry.45 In general, elastic materials show greater deformations than stiffer materials.45 Furthermore, substrate shrinkage leads to cellular degeneration and shape deformation, leading to a higher probability of cell detachment and subsequent apoptosis.48,49 In the case of a substrate that presents surface stiffness gradients, the cell interface in the softer area promotes cell migration towards the stiffer region, a process referred as durotaxis.50 As cells spread on stiff surfaces, they receive higher stress than on elastic areas, thus promoting the assembly of cytoskeleton into actin fibers and FA points, and ultimately signaling cell spreading.51 Finally, it has been shown that ECM elasticity plays a crucial role in determining stem cell differentiation lineage in vitro (Fig. 2b1-2),52,53
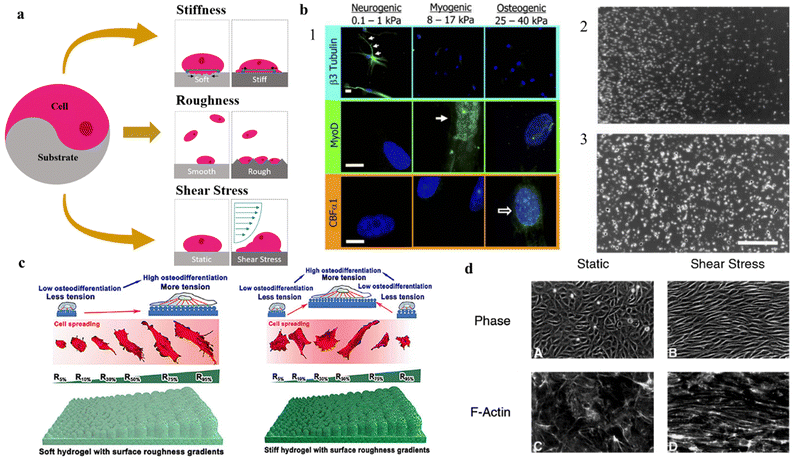 |
| Fig. 2 Impact of topographical features on cell adhesion, differentiation and migration on 2D substrates. Effect of (a) physical properties of 2D substrates on (b) cell differentiation and migration, (c) adhesion, and (d) shape and network formation. (a) Schematic diagram of different physical variables in cell adhesion to substrates. (b1) Stiffness of the underlying substrate determines the differentiation lineage of MSCs. For soft, neurogenic matrices, the neuronal cytoskeletal marker β3 tubulin is expressed in branches (arrows). On myogenic matrices, the muscle transcription factor MyoD1 is heavily expressed. On stiff matrices, is expressed the marker of osteoblast transcription factor CBFα1. Scale bar is 5 μm. Reproduced from ref. 52 with permission from Cell, copyright 2006. (b2) and (b3) Soft vs. Stiff substrates on determination of cellular spreading and migration. The interplay between the shortening velocity and the generated force from the cells leads to stiff substrates generating larger forces and displaying slower migration when compared with softer substrates. Reproduced from ref. 53 with permission from Experimental Cell Research, copyright 2013. (c) Schematic illustration of the effect of soft and stiff hydrogels with roughness gradients on cell adhesion and differentiation to osteogenic lineage. Reproduced from ref. 54 with permission from Nano Letters, copyright 2020. (d) Phase-contrast micrographs of confluent BAE monolayers reveal a distinct difference in endothelial cell morphology and microfilament network organization between static environment and the environment under shear stress. Under shear stress, the cells alter the actin cytoskeleton with the induction of actin stress fibers and cables in the direction of the flow. Reproduced from ref. 55 with permission from Journal of Cell Science, copyright 1996. | |
Studies have shown that roughness can influence various cellular processes (Fig. 2c).42–44,54,56–59 In general, an increase in surface roughness can enhance cell adhesion and proliferation, likely due to an increase in surface area available for cell attachment.42–44,56,58,59 However, if surface roughness increases too much it may cause changes in cell morphology and cytoskeletal organization, which could impact cellular adhesion.57–59 Furthermore, if roughness hits a critical ratio, the cells will fail to penetrate the surface grooves and the cell–substrate adhesion will drop drastically.56 When adherence increases with roughness, the cells can form tight junctions and establish a functional network in vitro. Increasing the roughness of the surface leads to higher surface energy area, rising serum protein adsorption, and thus cell adhesion60–62 In one study, XPS analysis showed that the rough substrate bound 10 times more fibronectin than the smooth substrate.61
Other from topography features of the surface, further external stimuli can be applied to the cells after seeding. Shear stress is a common mechanical stimulus applied in in vitro studies. Shear stress is a physical force that arises from the frictional drag of a fluid on a surface, can also have a significant impact on cellular development onto planar substrates by inducing a reorganization of actin cytoskeleton. The mechanical stimulus of shear stress can alter the expression of key genes and signaling pathways, leading to changes in cell morphology, proliferation, alignment, and migration (Fig. 2d).55,63–65 For example, shear stress has been shown to enhance the migration of endothelial cells in vitro by inducing cytoskeletal rearrangements55,66,67 and the formation of lamellipodial68,69 and filopodia extensions.70 Shear stress can also affect the differentiation of stem cells and the organization of tissue structures by modulating gene expression and distribution of growth factors.71
2.1.1. Substrates for neural cells.
Applying these principles to the demanding requirements of neural cells, results can differ entirely or from the other types of cells. Neuronal cells prefer an environment that mimics the elasticity of the in vivo tissue.52,72 Since brain tissue is distinctly elastic,73 this limits the array of choices for the substrate material in the extremely compliant category. For instance, spinal cord neuronal cells grown on softer substrates formed significantly more branches than those grown on stiffer substrates.74 In addition, neurites from chick dorsal root ganglia explants grew longer as the gradient of stiffness decreased in a three-dimensional genipin gel.75 When testing the surface characteristics conductive to neuronal adhesion and functionality, and optimal roughness range of up to 64 nm has been associated with promoting the adhesion and longevity of primary neurons.76 Mechanical stimuli have long been considered for stimulating neuronal differentiation from stem cells.77 Furthermore, Schwann cells were grown on a fibronectin surface and sustained under shear stress in the form of laminar fluid flow, results show that the mechanical stress can be used to better simulate the host natural environment for peripheral nerve regeneration by enhancing control of nerve guidance channels manifestation.78
Neurons are cells capable of electrical excitability, uphold a voltage gradient across their membranes through ion pumps, thus creating dissimilar ion concentration gradients for elements such as calcium, chloride, potassium, and sodium.79 The application of an external electrical field has been shown to affect cellular membrane potential through hyperpolarization and depolarization and voltage-dependent Ca2+ channels (VDCCs).80 Indeed, intracellular calcium ion signaling is a crucial mechanism in neuronal development, responding to changes in ionic flow on the cellular surface.81,82 Generally, the activation of VDCCs is mediated by cell adhesion molecules (CAMs) on the neuronal plasma membrane, which in turn induces physiological changes in neurons.80 Voltage pulse stimuli in neural cells, differentiated from human NSCs, have been shown to open Ca2+ channels and increase calcium concentration.83 Utilizing electrical fields on neural cells in vitro has proved to incentivize neurite outgrowth and altered cell orientation and motility.83,84 Thus, introducing electrical stimuli to the cell culture in synergy with the physicochemical factors of the substrate opens new doors in tissue engineering and biomaterials for recreating the perfect neuronal cell niche.85 The downside to this is that the biomaterial must be sufficiently electrically conductive in order to support electrical signaling among neurons.
Surface coatings.
To improve cell attachment and morphogenesis, surface coatings can be applied to the substrates.85,86 Surface coatings and functionalization might be key parameters in modulating the biocompatibility of neuron–substrate interfaces, playing a crucial role in the future development of innovative implantable brain devices. The modulation of the surface properties will indeed have a sizable influence on the behavior of the anchoring points of neurons on solid substrates, and as a consequence, on cell adhesion, neurogenesis, synaptic plasticity and formation of mature neuronal networks.87 A selective surface coating can also enable patterned neuronal networks, which are crucial in several applications. Synthetic polymers are commonly used in vitro. Poly-D or (L)-lysine (PDL; PLL) coatings do not affect signaling pathways or get degraded by cellular proteases. They are standard molecules used in in vitro neural cultures that significantly improve the attachment and growth of neural cells. PLL coating, for example, increases the hydrophilicity of the interface and improves neurite sprouting.88 Synthetic polymers are not the only tools to enhance neuronal affinity. Research groups have used ECM protein coatings to artificially influence cell adhesion. ECM proteins are proteins that link the extracellular cues to intracellular components, typically responsible for cell–cell adhesion. These molecules are also thought to stimulate neurite outgrowth and therefore are being studied as candidates for enhancing interfaces in nerve reconnecting.89 Examples of proteins used in coatings in vitro include laminin,90,91N-cadherin,92 fibronectin.90,93 One study tested with human embryonic stem cells (hESCs) the viability of neural progenitor generation on 5 different ECM coatings (PDL, fibronectin, PDL/laminin, type I collagen, and Matrigel). They found that laminin is the key molecule to enhance neural progenitor generation.90 It also provides chemotropism by binding chemical factors such as nerve growth factors to the surface.94 On the other hand, to better mimic in vivo physiology of neuronal networks, astrocyte cells have been used in place of coating agents with primary neurons to study their effect on adhesion and stem cell differentiation.93,95
Despite its atomic thickness, a graphene coating on the biomaterial can change the cell–substrate interactions substantially. Its single layer of covalently bonded carbon atoms interacting with organic molecules of carbon backbone enhances the biocompatibility of the interface. With CVD graphene being the most reliable way to produce high quality monolayer graphene, CVD graphene-based are coatings ideal candidates in biomedical engineering. The prowess of cells to be capable of sensing such tiny features is what allowed for the creation of this review. Surface coatings on substrates might help close the gap between the intrinsic physiological differences between cells and the underlying biomaterial, however, in vivo, cell layers form one on the top of each other in a three-dimensional conformation (forming tissue structures and organs). In this scenario, 2D substrates present the limitation of restricting cell attachment to the substrate by forcing the formation of cell monolayers, thus moderating the complexity and size of the population to the area of the surface.96 Once all available surface is covered, cell viability decreases, and cell death occurs. Due to their structure and properties, scaffolds can instead be used to easily provide trophic support to damaged tissue, to deliver transplanted cells and neuroprotective compounds, including proteins and oligonucleotides, that can be embedded in the scaffold matrix and released upon chemical/mechanical/biological stimuli.97 Thus, as 3D interfaces, the versatility of scaffolds, with interconnected networks for different biological applications holds great potential for the development of future implantable devices.51
2.2. Scaffolds
If substrates aim at advancing the lab-on-a-chip systems by improving in vitro setups for the understanding of specific biological and physiological questions, scaffolding brought an effective solution to several branches in the medicine-application field, such as biosensing,98 drug delivery,99,100 and the regeneration and restoration of damaged tissues.101,102 When producing biomaterial-based scaffolds, their mechanical strength, pore size, biocompatibility and biodegradation need to be considered. Furthermore, newly designed scaffolds need to specifically adapt to the biological environment by mimicking the ECM where the implant is to be placed, which later ensures that the functions displayed by the scaffold itself are approximated to the role played by the physiological ECM.103 Depending on which biomaterial is used and how it is designed, scaffolds can be differentiated into various types in order to satisfy the biological requirement that innovative neuro-interfaces need, such as biodegradability. Biodegradability is of particular importance in their engineering as it must be coordinated with tissue generation to effectively sustain the mechanical stresses in the ECM.104 The materials used should possess very low toxicity and should not interfere with any other tissues when the biodegradation process occurs. If the degradation is faster than the healing process, the formed tissue may turn defective or even unfeasible. On the contrary, if the degradation is too slow, it might lead to the encapsulation of the scaffold and corresponding possible tissue rejection.104 Moreover, the biocompatibility and efficiency of a scaffold is dependent on its proper mechanical strength to support cellular behavior. Parameters like tensile strength, stiffness and elastic modulus need to be taken into consideration when designing and fabricating a scaffold as they determine its integrity and durability, thus depending on the application, scaffolds require different ranges of mechanical properties. Ideally, scaffolds should mimic the mechanical properties of the anatomical site where they are intended to be implanted.105 Moreover, they should be able to avoid stress-shielding side effects, i.e., changes in the typical stress held by the scaffold,105 and resist structural collapse during implantation, which can result in necrosis. These characteristics are determinant in the scaffold functionality and viability. A study reported the role of alumina (Al2O3) nanowires and chitosan (CHS) on a polyhydroxybutyrate (PHB) scaffold for cartilage tissue engineering.106 Overall, the tensile strength increased from 2.8 MPa to 11 MPa, while maintaining appropriate cell morphology and proliferation. Also, chondrocyte cells were shown to spread more on PHB-CHS and PHB-CHS/3% Al2O3 scaffolds than on PHB scaffolds (Fig. 3a).
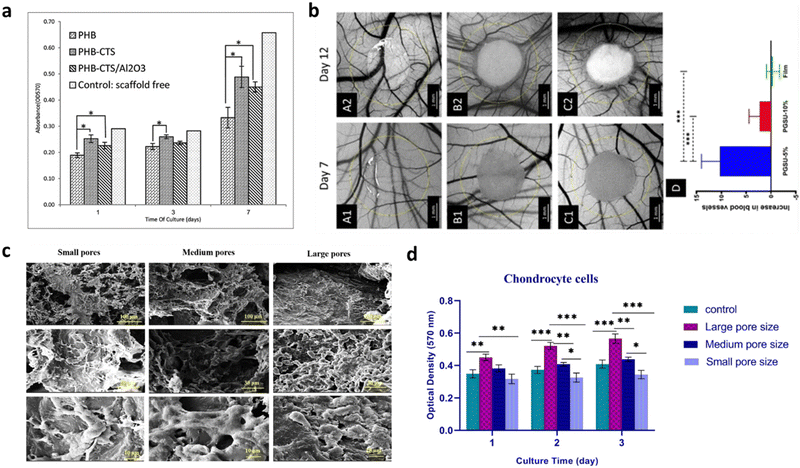 |
| Fig. 3 Effect of (a) mechanical properties and (b)–(d) pore size and porosity on 3D scaffold's culture development and cell viability. (a) Cell viability as indicated by MTT assay on electrospun PHB, PHB-CTS and PHB-CTS/Al2O3 in days 1, 3 and 7 of cell culture. The minimum of chondrocyte cell viability was observed for PHB scaffolds, while the other two scaffolds had higher amounts (*p < 0.05), illustrating the importance of tailoring the mechanical features of the scaffold to comply with certain requirements. Reproduced from ref. 106 with permission from Journal of Medical Signals and Sensors, copyright 2019. (b) Optical micrographs of (A1-2) PGSU film, (B1-2) PGSU-5% scaffold and (C1-2) PGSU-15% scaffold and surrounding vasculature at day 7 and day 12 post fertilization. (D) shows the increase in number of blood vessels between day 7 and day 12 (***p < 0.001). The PGSU-5% scaffold (bigger pore size and higher porosity) had a higher angiogenic response when compared to the scaffold with a smaller pore size and lower porosity (PGSU-10%). Reproduced from ref. 107 with permission from Materials Science and Engineering: C, copyright 2020. (c) SEM pictures and (d) cell viability indicated by MTT assay of chondrocyte cells loaded on small, medium, and large pore size scaffolds (*p < 0.05, **p < 0.01, ***p < 0.001). More cell attachment and proliferation can be seen on the scaffolds with medium and large pore sizes, whilst they are drastically reduced on the scaffold with smaller pore sizes. Reproduced from ref. 108 with permission from Mechanics of Advanced Materials and Structures, copyright 2022. | |
As the field evolved, lot of effort has been placed on the mechanical properties a biomaterial should have for scaffold engineering. However, many materials, which display the needed requirements in vitro, have failed to succeed when integrated in vivo, due to the lack of vascularization.109 It is then clear that an equilibrium between the mechanical properties and the porosity of the scaffold is required to allow cell infiltration and, more importantly, new blood-vessel formation and integration. Although high porosity structures might compromise the mechanical properties of the scaffold, using materials with high intrinsic mechanical strength can work to compensate for this issue. Porosity and pore size play an important role in 3D cell cultures. When it comes to 3D structures, porous scaffolds can promote cells’ sprouting and penetration as well as their distribution in the scaffolds.110 Specifically, high porosity and interconnected inner structures ensure that nutrients and gases diffuse inward into the deep zone of the scaffold and carry out metabolic wastes from the inside.111 By preference, a scaffold should have a 50–90% porosity (50% already guarantees cell migration and proliferation, but a 90% porosity is more favorable).111,112 For instance, for bone regeneration purposes, it has been reported that higher porosity is expected to enhance osteogenesis. Samourides et al. demonstrated the ability of large 3D poly(glycerol sebacate urethane) (PGSU) scaffolds with appropriate porous structure and porosity to facilitate cell proliferation and high collagen production.107 According to the porosity and pore size values, the scaffolds were categorized as PGSU-5%, PGSU-10% and PGSU-15%. It was concluded that PGSU-5% (5 wt% polymer concentration) scaffolds, possessing a high porosity (96%) and medium range pore size (∼26–28 μm), not only facilitated the ingrowth of tissue and blood vessels without requiring supplementary biochemical stimuli (Fig. 3b), but also reproduced higher collagen concentration.107 The pore size has also a critical role in the scaffold's viability as it should always be greater than the size of the cell. However, the size parameter may influence the scaffold behavior in a way that if it is too small, it might limit cell infiltration. On the other hand, if it goes too far beyond the size of the cells, it will affect their ability and speed of migration, which is a consequence of a reduction in the surface area.111 Recently, a study showed that PCL scaffolds with 100–150 μm pores size (medium-large) provided the appropriate mechanical strength along with the optimal condition for chondrocyte survival and proliferation for cartilage tissue engineering (Fig. 3c and d).108 Collagen porous scaffolds prepared with ice particulates in the range of 150–250 μm showed the highest promoting effect on the gene expression and the production of cartilaginous matrix proteins, as well as on cartilage regeneration.113
Among all the materials tested for scaffold fabrication, polymers are widely used can be mainly categorized as natural and synthetic polymers. Natural polymers such as chitosan, alginate, or bacterial cellulose exhibit great biological activity as well as remarkable biocompatibility and provide compositional uniqueness.114–117 However, their poor mechanical properties and fast degradation rates represent a limitation to multiple applications. On the other hand, synthetic polymers like poly(lactide-co-glycolic acid) (PLGA), polycaprolactone (PCL), and poly(ethylene glycol) (PEG) are man-made polymers.118–120 They can be tuned to display enhanced characteristics like mechanical, chemical, and rate of degradation, but lack cell recognition, and, in certain cases, biocompatibility and non-toxicity.121 Other than polymers, ceramics and metals are also used in the fabrication of scaffolds.122,123 Each biomaterial has its advantages and drawbacks. To overcome their limitations, composites have been commonly used as they allow the collection of multiple properties in the same scaffold.124 That is, forging two or more materials in the same scaffold grants the integration of properties that are lacking in a single-component scaffold.
2.2.1. Scaffolds for neural cells.
For what concerns nerve tissue engineering, some common scaffold formats and materials include fibers, conduits, membranes, and aligned scaffolds,125 However, neural cells grow and develop optimally when provided with spherical or cylindrical structures, likely due to their resemblance to natural neural elements like nerves, the spinal cord, or brain tracts.126 If there's a gap within the neuronal network, neurons can reconnect by forming a kind of bridge. This bridge can be quite long, but it does not give clear directions to the growing nerve fibers. This misdirection can cause them to grow in the wrong way. Thus, nerve guidance conduits (NGCs) can work as a physical guide to direct axons sprouting, displaying greater potential for nerve trauma therapies.127 NGCs can be made of polymeric materials. Polymeric biomaterials have been preferred as scaffolds for nerve tissue regeneration. This is because polymers can be programmed to have controlled biodegradability, ideal mechanical properties, high porosity and non-toxicity.128 Altogether, these properties can tune a scaffold to support neurite outgrowth and differentiation of human neural stem cells.129,130 Conduits could also be used as conductive materials to cause electrical stimulation, which was shown to promote neurite extension and nerve regeneration after sciatic nerve crush injury in a rat model.131
3. Graphene interfaces
As mentioned above, several biomaterials have been proposed to create the ideal scaffold for cell/neural interfaces. Some display exceptional properties but lack certain requirements, especially when it comes to in vivo applications. Composites of two or more materials can solve this issue by integrating different features into the same scaffold. However, they too come with their own set of challenges, such as the complicated fabrication techniques, high complexity and higher cost than single-phase scaffolds. In this scenario, graphene could play a crucial role for the new generation of brain-scaffold interfaces. Graphene is a monolayer of carbon atoms in a honeycomb lattice and, is a promising nanomaterial for scaffolding, contrarily to the usual biomaterials (e.g., polymers), its atomically thin nature allows, simultaneously, for exceptional thermo-electrical conductivity, high mechanical strength, biocompatibility and easy functionalization. Graphene has been making waves in nanotechnology since its first isolation in 2004 for its outstanding intrinsic properties and its versatility in tuning them.132 Graphene holds favorable conditions for biological interactions as it is biocompatible133,134 and its high surface area with atomic thickness allows for easy calibration of its properties132 and strong response to adsorption events.135 In particular, the biocompatibility of graphene and graphene-based materials (GBMs) with neural cells, including neurons, astrocytes and endothelial cells of brain microvascular capillaries, has been extensively studied in recent years.136–138 Studies have shown that GBMs do not cause significant toxicity to neural cells and can even promote cell adhesion and proliferation. However, the effects of GBMs on neural cells can vary depending on the size, shape, and concentration of the materials, as well as the duration of exposure.139,140 Hence graphene and GBMs have been tested for biomedical applications in tissue regeneration scaffolds, biosensors,135,141 carries for drug delivery,142,143 antibacterial agents,144 and high contrast MRI image analysis.145
3.1. Graphene properties
Graphene is a two-dimensional allotrope of carbon, first discovered in 2004 by mechanical exfoliation of highly oriented pyrolytic graphite.146 Endowed with unique and outstanding properties, the interest in integrating graphene in multiple fields quickly rose. It can be described as an atomically-thin single layer of firmly packed carbon atoms, arranged in a sp2-hybridized honeycomb lattice. This arrangement, resulting in strong carbon-to-carbon σ bonds, along with graphene electronic band structure, is responsible for the remarkable thermal and electrical conductivity the material displays.147 Moreover, graphene was found to be one of the strongest nanomaterials ever tested, with high Young's modulus (∼1 TPa) and high intrinsic strength (130 GPa), to have chemical stability and good biocompatibility.148,149 The delocalized electrons in graphene pi-bonds make the material incredibly conductive, with high intrinsic carrier mobility of 2 × 105 cm2 V−1 s−1.150 Despite its formidable strength, graphene is a flexible material, allowing better distribution of forces and reduced localized stress in the cells. Flexible substrates/scaffolds not only promote longer neurites, but a greater density of outgrowths.74,75,151 However, the effect of substrate flexibility on neural cell migration is complex and not fully understood. Furthermore, graphene conformability is useful for coating irregular shaped scaffolds.15 Graphene is a transparent 2D material, from a low optical absorbance of around 2.3%.152 Therefore, it allows for optical imaging and monitoring of cells grown in vitro.135 Being a 2D material, its thickness is much smaller than its lateral dimensions. When fully exposed, graphene has a theoretical surface area of ∼2630 m2 g−1.153 Such a large surface area provides high degree of interaction with cells.135,154 That being the case, graphene and GBMs, such as graphene oxide (GO), reduced graphene oxide (rGO) and graphene nanocomposites,155 appear to be attractive candidates for the biomedical applications field. However, a main concern arises: GBMs can either be biocompatible or toxic to cells.149 Ensuring a non-toxic interaction between GBMs and cells relies on how their properties are tuned. An ideal material to use in neuroscience and regenerative biology is one that avoids damaging the cells physically, such as its plane edges piercing the cell membranes, and chemically, like triggering a strong immunological reaction when interfacing with living cells.6,156 How living cells respond to these nanomaterials is highly dependent on their properties like size, surface chemistry, hydrophilicity, surface area, lateral size, etc.157 For example, the biocompatibility of graphene can be further improved with surface functionalization of the basal plane or edges.156 In a perfect graphene plane, the surface is chemically inert, this would turn surface functionalization costly energy wise. But this would imply an infinite-scale film without edges and defects. However, this is practically impossible as graphene unavoidably comes with edges, vacancies, impurities, Stone–Wales defects, etc.157,158 Without these, a covalent bond to a carbon atom in the basal plane dictates that the atom adopts a tetrahedral sp3 geometry. This change not only presents a sizeable energy requirement but induces stress on the plane.159 However, edge atoms and vacancies can adopt the tetrahedral geometry without causing stress to the plane, therefore these regions have greater chemical reactivity than the basal plane atoms.160 High chemical reactivity regions together with graphene's high surface-to-volume ratio allow for easy and extensive functionalization options for graphene surfaces in order to tune their electrical, optical, magnetic, hydrophilic properties and more, for enhanced cellular compatibility.121,156 Furthermore, increasing the hydrophilicity of the graphene also serves for a more homogenous dispersion of the material in an aqueous solution for the purpose of drug deliveries in vivo.161,162 The application of graphene and related materials across all relevant fields is constrained by their current production methods. The realization of their transformative impact hinges on surmounting the challenges posed by existing fabrication techniques. Whether it is scalability, cost-effectiveness, or reproducibility, these challenges serve as important obstacles to overcome in GBM device manufacturing.
3.2. Graphene production
When it comes to producing GBMs, there are generally two approaches: the bottom-up and top-down. The former relies on the use of physical and chemical processes to trigger the assembly of simple units to larger nanostructures. The latter involves breaking down larger structures of a material to produce the desired nanostructures from them. Mechanical exfoliation, liquid phase exfoliation (LPE) and the exfoliation of previously oxidized graphene to obtain GO and respective reduction to rGO are examples of top-down fabrication techniques of graphene. On the other hand, the bottom-up approach includes processes like chemical vapor deposition and epitaxial growth on silicon carbide.163 The exfoliation of bulk graphite has been a viable method to produce high-yield pristine graphene. Among carbon materials, bulk graphite has the particularity of having strong covalent (in plane) bonds but weak van der Waals (vdW) interactions (out-of-plane). Accordingly, liquid phase exfoliation involves breaking down bulk graphite into graphene flakes in a liquid solvent by using an energy form (e.g., ultrasonication), by overcoming these weak vdW forces.164 The flakes can then be separated and collected through filtration or other methods. Generally, LPE includes two main different strategies for the exfoliation of bulk graphite: cavitation via ultrasonication165 and shear forces through a high-shear mixer.166 Both processes facilitate the exfoliation process and are followed by a washing/purification step to yield pure single- and few-layer graphene. CVD is a bottom-up synthesis method in which substances, in a vapor phase, go through a chemical reaction to generate the deposition of a solid phase material in a target substrate, usually under vacuum. Typically, a CVD equipment is composed by a gas delivery system, a reaction chamber, a vacuum system; an energy system, an exhaust gas treatment system, and a control system.167 The elementary steps of a typical CVD process occur on or in the vicinity of a substrate. First, the reactant gases enter the reactor. They either (i) undergo gas-phase reactions to form intermediate reactants and gaseous by-products, which then adsorb onto the heated substrate and diffuse on the surface, or (ii) diffuse directly to the substrate. This leads to a continuous thin film formation via nucleation, growth, and coalescence.167 Finally, gaseous products from the reaction and unreacted species desorb from the surface and are carried away. Transition metal substrates such as nickel (Ni) and copper (Cu) are commonly used for the CVD synthesis of graphene as they also work as reaction catalysts. This growth process follows two steps: (i) desegregation of carbon from the carbon gas precursor (usually CH4 or C2H2); and (ii) formation of graphene on the surface of the metal catalyst, using the segregated carbon.168 Regarding this technique, Li et al. were the first to report large-area high-quality monolayer graphene growth on copper substrates. The films were reported to be mainly single-layer graphene (95%) and were continuous across copper surface steps and grain boundaries.169 On the other hand, Yu et al. reported the fabrication of few-layer graphene (FLG) on polycrystalline Ni foils.170 The main conclusion was that the cooling rate affected the thickness of the graphene layers, with FLG (3–4 layers) being produced with a cooling rate of 10 °C s−1.171 Among all production techniques, CVD graphene is the most suitable for designing a biological interface with high reproducibility and uniformity in properties over large areas,172–174 For in vitro cell interfaces studies, CVD graphene showed to improve cell viability and adhesion compared with its uncoated counterparts for a range of cell types, including endothelial,175,176 fibroblast,177 and osteoblast cells.174,178,179 However, CVD graphene still remains limited to surface coating applications, as opposed to the versatility of composites or other graphene fabrication techniques.
3.3. Biocompatibility of graphene-based materials
Biocompatibility, defined as the ability of a material to interact harmoniously with living organisms without inducing adverse reactions, is a critical determinant in the success of neural implants. The brain's delicate environment demands materials that not only exhibit favourable electrochemical properties but also foster a symbiotic relationship with neural tissues. As a prospective class of materials for neural interfaces, GBMs need to be fully investigated in terms of biocompatibility.180 Studies on the cytotoxicity and biocompatibility of 2D materials have been carried out both in vitro and in vivo, however, the molecular and biochemical pathways underlying 2D material toxicity when in contact with biological systems is still a major challenge, as multiple parameters have to be considered leading to discrepancy in the present literature.29,181,182 As of today, the scientific community is reaching an agreement regarding such key characteristics that might affect the material biocompatibility, such as lateral dimensions, thickness, surface chemistry and functionalization, oxidation degree, formulation and the biological system used to test the biocompatibility.183,184 For these reasons, we should remember that it is not correct to generalize on 2D materials biointeractions and biocompatibility. In the specific case of GBMs and nervous tissue, there is a wide and extensive literature highlighting their safe use for the development of brain implants and interfaces, displaying encouraging results both for graphene sheets (2D-flakes) in solution and graphene-based supports and scaffolds (2D-films). This is a necessary distinction to make as one of the main safety concerns for GBMs come from their nanoforms, as nanosheet liquid suspensions or aerosols.30,180,181,185 At the same time, implantable devices might release fragments due to degradation/delamination of the 2D-films, thus making the investigation of the biological effects over time of outmost importance as well as the biodistribution of such fragments. Graphene has indeed been proved to cross biological the blood–brain barrier in vitro without affecting its permeability and the microvascular endothelium viability.186
GBMs intended for neurological applications necessitate further assessments to evaluate their biocompatibility. For instance, for drug delivery it becomes crucial to conduct tests on concentration-dependent cytotoxicity. This involves investigating the mechanisms of cytotoxicity in vitro and examining the material's biodegradability in vivo. Meanwhile, in the context of scaffolds for tissue engineering or biosensing it is imperative to continuously monitor the GBMs in terms of mechanical stability, biocompatibility, and potential long-term toxicity to prevent the initiation of chronic neuro-inflammation. So far, GBMs produced in liquid phase have already been tested on different cell types, including primary neurons187,188 and astrocytes,189,190 blood–brain barrier endothelium,186,191 both in vitro and in vivo studies.188,192 The main results display an extremely good interaction of GBMs with neural cells, without showing any sign of cytotoxicity and neuro-inflammation due to astrocytic increased activity or astrogliosis. Specifically, it was seen that chronic graphene and GO exposure did not cause alterations in cell viability and network formation. However, investigations on cell functionality revealed some physiological effects specific to GO exposure at synaptic signaling level, indicating GO can interfere and alter neuronal post-synaptic currents, calcium oscillations and neuron-to-astrocyte communication.187,188 These lates results could now be exploited to introduces GO nanosheets for the treatment of specific synaptic pathologies. On the other hand, BMIs are in need of implants designed for transient usage. In in vitro studies, the feasibility of neuronal cell growth on flat substrates coated with GBMs has already been tested and demonstrated. These studies underscore the capacity of GBM films to support the growth and differentiation of neuronal cells, as well as to enhance neuronal activity.185,193–195 Generally, CVD graphene films with high hydrophilicity have considered more biocompatible and secure when compared to graphene nanosheets, making CVD graphene potentially viable for future implantable devices.140 In summary, reports about the neural biocompatibility of GBM exist in the literature with studies suggesting their benign effects with poor or null cytotoxicity, low inflammatory cell response and positive physiological effects on neuronal synaptic plasticity.
3.4. CVD graphene for substrates
In the last decade, graphene incorporation in biological scaffolds has been tested with the goal of improving the substrate intrinsic properties. Due to its outstanding qualities, including high mechanical strength, chemical stability and biocompatibility, CVD graphene is quite an attractive candidate for designing and developing neural tissue in scaffolds. Graphene-based coatings have been already applied to various types of neural scaffold materials, such as polymers and ceramics. Moreover, these coatings can also be functionalized with different molecules and chemical groups, such as growth factors or adatoms, to further promote neural cell adhesion, proliferation, migration, and differentiation. The flexibility of graphene on polymeric substrates (e.g., PET) allows for the creation of soft and flexible interfaces that can adapt to the mechanical properties needed to promote cell migration and neurite outgrowth.8,196 The crystalline quality of graphene seems to affect the cellular adhesion of neurons. Various reports have been showing this material can enhance the performance of neural interfaces, namely in terms of biocompatibility,134,178 cell adhesion and proliferation174,197 and neural cell-to-cell interactions.198,199 Particularly, pristine CVD graphene was reportedly transferred to glass coverslips and cultured with hippocampal neurons.88 It was found that as disorder rises within the grid, the surface shifts from cell adhesive to fully repellent, suggesting that high crystalline quality of graphene enhance cellular adhesion of neurons. In another work, Park et al. discovered the enhanced neuronal differentiation of human neural stem cells (hNSCs) on graphene.83 Graphene was grown by CVD, transferred onto glass substrates, and placed into a laminin solution, to aid hNSC attachment. The hNSCs were seeded on the substrate and were differentiated for approximately one month. During the differentiation process, some of hNSCs differentiated into neurons, whereas others differentiated to glia, supporting the activity of neurons. Overall, graphene worked as an excellent cell-adhesion layer during the long-term differentiation process and induced the differentiation of hNSCs more toward neurons than glial cells (Fig. 4a). Furthermore, the authors reported the neural activity of the differentiated cells and suggested that graphene can be used as an excellent nanostructured scaffold for promoting NSC adhesion and differentiation for long-term periods. In addition, Hong and colleagues examined neural cell adhesion and neurite outgrowth on graphene-based biomimetic substrates, as well as the biocompatibility between neural cells and graphene or CNTs.200 Graphene membranes were synthesized by CVD and transferred onto glass coverslips and graphene sheets were patterned on SiO2/Si substrates. For the neural cell adhesion, both graphene surfaces were covered with 50% fetal bovine. The graphene-coated substrates were shown to substantially enhance the adhesion, neurite outgrowth, and proliferation of neural cells (Fig. 4b and c). The results also suggest that the graphene-patterned substrates have a specific surface property that can promote neural cells. Ideally, a neuron-friendly interface should possess high wettability and electrical conductivity.201,202 However, studies show that pristine graphene, although having high intrinsic conductivity, has poor wetting behavior,203 which can limit some biological applications. Accordingly, using different kinds of GBMs can contribute to maximizing properties such as biocompatibility and wettability. GO has higher wettability than graphene itself and was reported to interact more easily with neurons in terms of membrane adhesion and intracellular uptake.204,205 Even so, GO and rGO generally have low electrical conductivity.8 Alternatively, the functionalization of pristine graphene appears to be a viable solution. Functionalized forms206,207 are promising approaches of graphene manipulation which have quickly gathered interest in the neural interface applications. Moschetta et al. developed a biological interface for primary neuronal networks.8 As-grown CVD graphene was hydrogenated by a hydrogen plasma treatment and transferred to a PET substrate. The results showed the growth of primary neurons with high cellular adhesion and viability (Fig. 4d) and preserved physiological intrinsic firing properties. Also, the PET/HGr substrates exhibited an enhanced excitatory transmission, two times higher than PET/Gr ones. The authors suggest that, in addition to conductivity, wettability plays a critical role in the formation of mature neuronal networks and may even be of greater importance. Similarly, graphene functionalization with oxygen or fluorine, by using pre-treatment in O2 or C3F8 gas environments has been reported.208 The proliferation of SH-SY5Y cells was found to be enhanced by oxygenated graphene sheets compared to pristine graphene. The hydrophilic environment of the graphene sheets facilitated cell adhesion, leading to increased cell viability and neuronal proliferation of SH-SY5Y cells compared to the hydrophobic environment of the pristine graphene sheets. Additionally, fluorinated graphene (FG) sheets were used as a scaffold for stem cell growth.209 Pristine graphene (G), partially fluorinated graphene (PFG) and FG were transferred to SiO2/Si substrates. The results showed that the full fluorination of graphene caused the MSCs to proliferate faster, with increased cell density, when compared with G and PFG, which proves that the C–F bonding facilitates better cell adhesion and proliferation. Also, MSCs cultured on FG demonstrated neuron-like morphologies with visible neurite protrusions indicating further neuronal maturation. The expression of neuronal gene markers was further enhanced by adding the neuro-inductive agent retinoic acid (RA) (Fig. 4e). The fluorinated graphene films were then patterned into microchannels (30 μm) by printing PDMS line on top of the FG. As the MSCs preferentially adhere to FG, their cell bodies and infrastructure conform to the shape of the adhesive substrate, the results show enhanced neurogenesis via confinement-induced cell elongation. Patterning the cell substrate to create specific adhesive and non-adhesive areas for modulating neural development (e.g., neural growth, cell migration and differentiation) has shown to be an effective method of enhancing and generating custom neuronal networks in the absence of chemical stimulants.210–213 Asymmetric geometries of the substrate force rearrangement of cytoskeleton structures and stimulate the sprouting of neurites, which are an early event of neuronal differentiation,214 by induced cell polarity,210,211,215 and guide growing axons down handmade pathways.216 Methods for patterning graphene have been extensively researched for its many applications in device designing and manufacturing.217 Photolithography has been used to pattern CVD graphene to columns and grid-like patterns. When interfaced with bone marrow-derived hMSCs, the in vitro cell culture displayed increased neuronal markers expressions when compared to unmodified CVD graphene. The advanced neural differentiation is hypothesized to result from hMSCs alignment on the patterned graphene.218 Single pulse UV laser ablation technique proved to an effective way to pattern monolayer CVD graphene while minimizing the laser damage effects. Primary embryonic hippocampal neurons were cultured on the substrate and developed a neuron network mimicking the pattern design on the graphene.219 Using the same patterning method on CVD graphene seeded with primary cortical neurons, one group applied single-molecule localization microscopy combined with cluster analysis to quantitatively study the distribution of the adhesion protein vinculin in the cells of neurons on glass and graphene substrates. Cells on graphene expressed higher number and larger size of vinculin clusters than on glass.220 Neurons displayed rearrangement and fragmentation of vinculin clusters over time, with higher cluster density on graphene compared to glass. Which explains to cell migration towards graphene stripes on micropattern substrates. Graphene-based substrates have been a reliable and relatively easy-to-produce method for studying cellular interactions in a lab environment. However, as studies grow ever more specialized in their relative field of research, so do the requirements for the substrate's properties. This evolution in research demands new and more effective platforms that not only display biocompatibility with the cell culture, but also mimic the intricate physiological conditions specific to the targeted application. To this end, graphene-based biomaterials have branched into more complex cell environments such as the realm of 3D interfaces.
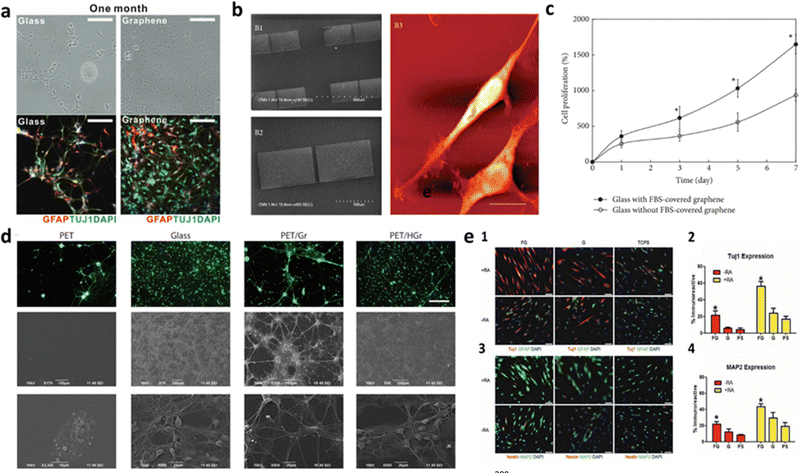 |
| Fig. 4 Graphene influence on the improvement of neurite outgrowth and cell adhesion, proliferation and differentiation in 2D cultures. (a) Bright field (top) and fluorescence (bottom) images of hNSCs differentiated both on glass and graphene. Graphene not only provided more hNSCs adhesion than glass, but also induced the differentiation of hNSCs more toward neurons than glial cells. Reproduced from ref. 83 with permission from Advanced Materials, copyright 2011. (b) PC-12 spreading with apparent neurite outgrowth on graphene-based biomimetic substrates and (c) evaluation of PC-12 cells proliferation with WST-8 assay on bare glass coverslips and glass coverslips with FBS-covered graphene. The cells proliferated more on the glass coverslip with FBS-covered graphene than on the bare glass coverslip (*p < 0.05). Reproduced from ref. 200 with permission from BioMed Research International, copyright 2014. (d) Potential of hydrogenated graphene in cell adhesion and viability. The Middle and Lower panel show SEM images of cultured neuronal networks, confirming no substantial affectation of cell morphology. Reproduced from ref. 209 with permission from Advanced Materials, copyright 2012. (e) Fluorescent images of immunostained MSCs for (1) Tuj1 and GFAP and (3) Nestin and MAP2 cultured on FG, G, and polystyrene (PS), as the control, in the presence (+RA, yellow) and in the absence (−RA, red) of retinoic acid. Neuronal markers Tuj1 and MAP2, are highly expressed in MSCs cultured on FG, conversely to Nestin and the glial-specific marker GFAP. This indicates a preference of morphologically induced differentiation towards the neuronal lineage over the glial lineage. (2) and (4) Show the percentage of immunoreactive MSCs on FG, G, and PS, confirming high neuronal differentiation of MSCs, further enhanced by the addition of RA. Reproduced from ref. 209 with permission from Advanced Materials, copyright 2012. | |
3.5. CVD graphene for scaffolds
The need to evolve cell scaffolds to the next level is more pronounced than ever before, as they are equipped with physical and chemical characteristics that more closely resemble the conditions of in vivo tissue. Increasing the dimensionality of the substrate that can interact with cells comes with reasonable arguments. When cells grow on substrates, they can only attach to the planar surface, forcing the culture to adopt a monolayer nature, whilst in vivo layering is regular.96 Interconnected 3D networks, especially in the nervous system, not only enable more complex cell–cell and cell–ECM interactions and exchanges, but also allow for a greater adhesion due to the increase in surface area.221 In this regard, a porous 3D scaffold has been shown to facilitate the transfer of nutrients and gases and promote cell growth, migration and proliferation, leading to the formation of larger and more complex tissue.51,222 The aromatic structure of graphene scaffolds increases the local concentrations of adhesion proteins in the matrix (e.g., laminin, collagen, fibronectin) by non-covalent binding.209 In the case of neurons, the interconnected available area for growth is useful for providing conductive pathways for charge transport and neural network formation.140
Graphene-based materials have been used to create porous scaffolds with neuron-affinity formats, including NGCs, membranes and fibers.223,224 However, to maintain the pristine state of monolayer graphene still remains the challenge in the fabrication of graphene scaffolds. One approach is to use CVD graphene coatings, by fabricating graphene foams (GFs). They can be described as three-dimensional counterparts to planar graphene, which possess several favorable physical and electrical traits (e.g., high pore volume, distinguishable thermal and electrical conductivity, controllable degradation rate),225 but are created as porous scaffolds, considerably augmenting the available surface area for cellular proliferation.226 Their good electrical conductivity is a key factor as neurons communicate via electrical impulse and the interconnected porous network facilitate the diffusion of nutrients and oxygen, which is crucial for neuron survival and growth. The most common example is synthesizing 3D-GFs by CVD, using a Ni foam as template (Fig. 5). Reports have shown how scaffolds based on GFs can enhance neural cell adhesion, proliferation and differentiation of neural cells.226–230 Specifically, a study demonstrated the feasibility of GF as a 3D scaffold for functional myotube growth, highlighting the functionality of the myotubes with electrical stimulation, which produced observable motion of the GF from the contraction of the cells.226 After 2 days in differentiation media, both the laminin-coated GF samples and the uncoated (bare GF) had observable cell growth demonstrating the feasibility of both scaffolds for C2C12 attachment. After day 6, both samples exhibited multinucleated myotube formation (Fig. 5d). In addition, a 3D-GF/polycaprolactone (PCL) caused the cells to exhibit more elongated cell shape than PCL scaffold itself, highlighted by yellow arrows (Fig. 5b).228 The proper mechanical properties of this structure enhanced PC12 cells proliferation. Finally, these graphene-based structures were shown to electrically stimulate cells differentiated from NSCs, confirming their efficiency as conductive platforms to mediate electrical stimulation for differentiated NSCs (Fig. 4c),229 provide the differentiation of NSCs into neural lineages (Fig. 5a),227 and exhibit substantially longer neurite extensions on GF-based scaffolds than on collagen scaffolds (Fig. 5e).230 In summary, adopting scaffolds for interfacing with cells seems to be the next logical step in in vitro studies. However, the fabrication of these materials relies on complex techniques and heavy expenditure. The possibility of 3D substrates becoming a common method of interfacing with cells must precede the development of new and more efficient fabrication techniques in microtechnology.
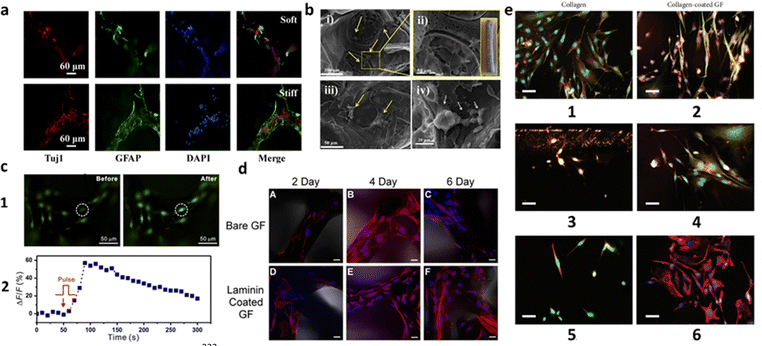 |
| Fig. 5 Review of CVD-grown 3D-GF scaffolds on Ni foam templates, considering their coatings, cells used and main results. (a) Immunofluorescence images of NSCs stained with Tuj1 (specific neuron marker), GFAP (intermediate filament protein in astrocytes) and DAPI (membrane viability) on 3D-GFs coated with ploy-L-ornithine (PLO) and laminin. NSCs are shown to differentiate into neural lineages. The 3D-GFs fabricated with the original Ni templates were defined as soft scaffold and with the extruded one (75%) as stiff scaffold. Reproduced from ref. 227 with permission from ACS Applied Materials & Interfaces, copyright 2016. (b) SEM images of PC12 cells on 3D-GF/PCL scaffolds after 1 week of culture. Higher proliferation was achieved as a result of good mechanical properties. Reproduced with permission from ref. 228. (c) Electrical stimulation of the cells differentiated from NSCs on laminin-coated 3D-GFs: (1) fluorescence imaging of the cells pre-incubated with Fluo-4 AM dye on 3D-GFs before (left) and after (right) electrical stimulation; (2) Plot of the relative fluorescence intensity change ΔF/F of the circled cell in panel (1) versus the stimulation time period. Reproduced from ref. 229 with permission from Scientific Reports, copyright 2013. (d) Confocal Z-stack images of C2C12 cells cultured on Bare GF and laminin-coated GF. Blue, nuclei; red, actin. Growth on bare GF after culture in differentiation media (DM) for (A) 2, (B) 4, and (C) 6 days. Laminin-coated GF after culture in DM for (D) 2, (E) 4, and (F) 6 days. Scale bars: 20 μm. Reproduced from ref. 226 with permission from ACS Biomaterials Science & Engineering, copyright 2016. (e) Comparison of differentiated DA neurons with mouse MSCs in contact with (1, 3, 5) collagen gels and in contact with (2, 4, 6) collagen-coated GF. (1, 2) NeuN (red) and β-III tubulin (green); (3,4) TH (red) and β-III tubulin (green); (5, 6) NeuN (red) and vimentin (green). In all instances, the differentiation of mouse MSCs into a neuronal phenotype resembling dopaminergic (DA) neurons was observed. GF-based scaffolds exhibited substantially longer neurite extensions compared to those cultured in contact with collagen. Scale bars: 30 μm. Reproduced from ref. 230 with permission from Stem Cells International, copyright 2018. | |
3.6. Brain-interfaces based on CVD graphene for biomedical applications
As discussed in the previous sections, CVD graphene is one optimal candidate to create 2D and 3D neural environments, by promoting cell adhesion and proliferation and electrical stimulation. Producing graphene through CVD allows for optical transparency, making it ideal for studying neural networks and cortical features, where optogenetics and calcium imaging provide complementary information.231 In this regard, CVD graphene can be used for revolutionary devices capable of interfacing directly with the brain. One example is represented by graphene-based flexible electrodes and field-effect transistors (GFETs) for recording neural signals in brain–computer interfaces, able to conform to the irregular surface of the brain and enable stable signal recording. Microelectrodes can be also used to neuro-stimulate the brain with electrical impulses that modulate neural activity. This brings relevance especially to the treatment of diseases such as the Parkinson's and epilepsy. Zhao et al. developed implantable neural electrodes by encapsulating CVD-grown graphene on Cu microwires.232 The graphene-encapsulated Cu microwires demonstrated significantly reduced toxicity to brain tissues, as evidenced by in vitro cell tests, in vivo histology, and MRI studies. The high MRI compatibility opens new opportunities for brain activity studies and clinical applications requiring continuous MRI and electrophysiological recordings. In addition to MRI applications, multilayer CVD graphene electrodes were shown to enable multimodal neural recording and electrical and optogenetic stimulation, while allowing artifact-free MRI studies.233 CVD graphene-based microelectrodes were also shown to provide simultaneous in vivo calcium imaging and electrocorticography (ECoG) recording of neural activity234 and the detection of changes in beating frequency.235 GFETs are another platform on which CVD graphene can and has been integrated. The intrinsic amplification effect of transistor configuration leads to a higher SNR than electrodes.236 Garrido's group fabricated solution-gated GFETs on polyimide substrates suitable for μ-ECoG recordings through studies of spontaneous slow-wave activity, sensory-evoked responses on the visual and auditory cortices, and synchronous activity in a rat model of epilepsy. The SNR of this technology was similar to that of Pt black electrodes for frequencies under 100 Hz.231 The same group used the same kind of devices to In vivo record and map brain electrophysiological signals. Specifically, the devices provided high-fidelity in vivo recording of cortical spreading depression signals at sub 0.1 Hz frequencies.237 Finally, the GFETs allowed for amplitude modulation of neural signals in situ, simplifying the technical complexity multiplexed neural probes.238
4. Conclusions, challenges, and future prospects
Substrates and scaffolds are made of biomaterials engineered to interact with biological systems with the goal of facilitating cell adhesion and proliferation. All this ultimately aims at promoting tissue regeneration, organ repair, or the study of cellular behaviors in controlled environments. Biomaterials can be natural, like gelatine, silk, and collagen, or synthetic, like polymers, and they are used in a wide range of medical applications, such as drug delivery, tissue engineering, and implantable devices. However, the development of new substrates and scaffolds and the advancement of existing ones is of utmost importance, especially to target particularly sensitive tissues and organs such as the brain and the central nervous system in general. Among all, biocompatibility is the primary characteristic that any cell interface must have. Therefore, cell substrates and scaffolds need to be bioinert, minimizing tissue reactivity, and bioactive, exploiting the interaction with a living tissue to positively influence the biological response. Recently, graphene-based scaffolds and substrates have been investigated and developed for both neural tissue engineering and treatment and diagnosis of neurological diseases, exploiting their electrically conductivity, versatility and flexibility. However, before graphene could be routinely used to engineer sophisticated bio-sensing interfaces adaptable to the central nervous system, a detailed comprehension of its behavior of in a biological context is mandatory. To address this point, reliable in vitro models are fundamental before moving to in vivo applications. In this review, we identified the crucial prerequisites for in vitro and in vivo neuroscience research on graphene-based substrates and scaffolds: (i) wetting properties; (ii) topography and roughness; (iii) electrical conductivity; and (iv) interactions with the extracellular matrix. We highlighted the need of hydrophilic surfaces (also possibly obtained by surface modification strategies) when designing substrates and scaffolds for biological applications to improve cell adhesion and growth. We underlined that topographical cues are extremely powerful tools to influence cell behavior in terms of adhesion, morphology, migration and differentiation. The geometrical features of substrates and scaffolds might even cooperate with biochemical compounds to synergistically control the biological response. Especially in central nervous system applications, the electrical conductivity provided by graphene might lead to beneficial effect on the neural cells. Finally, we explored the differences between substrates and scaffolds when coming to extracellular matrix interactions, highlighting how substrates could be better suited for in vitro studies, while scaffolds might be exploited in in vivo applications due to their 3D structure and porosity that better mimic the physiological conditions. In this scenario, a crucial point that future research will have to address is to transfer the large body of knowledge obtained from in vitro evidence to the in vivo context. A detailed elucidation of all these aspects will greatly improve our ability to engineer and select suitable interfaces for brain-tissue engineering and regenerative medicine applications. Although most studies agree that graphene displays a safe biocompatibility, when designing planar substrates and scaffolds its long-term interactions with cells are still relatively obscure. Further studies are required on graphene's long-term and in vivo biocompatibility and biodegradation to fully understand its effects on intracellular mechanisms and signaling protocols, to properly make the transition to in vivo neuro-applications. Moreover, molecular consequences and biological effects from the interaction of neuroimmune cells and graphene supports are still unknown. Similarly, there is the need of experiments to measure the expression of factors secreted by microglia and characterize its activation in the presence of graphene. Nonetheless, future functionalization techniques might as well push graphene compatibility with cells to new heights and facilitate its transition to more complex 3D environments.
Author contributions
All authors have reviewed and approved the final version of the manuscript. Conceptualization: A. C.; funding acquisition: A. C.; investigation: V. L., G. M.; visualization: V. L., G. M., A. C.; writing – original draft: V. L., G. M.; writing – review and editing: all authors; supervision: M. B., A. C.; project administration: A. C.
Conflicts of interest
There are no conflicts to declare.
Acknowledgements
The authors acknowledge the financial support of the project 2DM4EH with reference DRI/India/0664/2020, funded by FCT—Science and Technology Foundation. M. B. acknowledges the MICIN/AEI/10.13039/501100011033 and the European Social Found (FSE) El FSE invierte en tu futuro for Ramón y Cajal research contract (RYC-2019–027692-I).
References
- R. Tanamoto, Y. Shindo, N. Miki, Y. Matsumoto, K. Hotta and K. Oka, Electrical stimulation of cultured neurons using a simply patterned indium-tin-oxide (ITO) glass electrode, J. Neurosci. Methods, 2015, 253, 272–278 CrossRef CAS PubMed.
- S. Buccelli, Y. Bornat, I. Colombi, M. Ambroise, L. Martines, V. Pasquale, M. Bisio, J. Tessadori, P. Nowak, F. Grassia, A. Averna, M. Tedesco, P. Bonifazi, F. Difato, P. Massobrio, T. Levi and M. Chiappalone, A Neuromorphic Prosthesis to Restore Communication in Neuronal Networks, Science, 2019, 19, 402–414 Search PubMed.
- L. A. Struzyna, K. Katiyar and D. K. Cullen, Living scaffolds for neuroregeneration, Curr. Opin. Solid State Mater. Sci., 2014, 18(6), 308–318 CrossRef CAS PubMed.
- Y. D. Teng, E. B. Lavik, X. Qu, K. I. Park, J. Ourednik, D. Zurakowski, R. Langer and E. Y. Snyder, Functional recovery following traumatic spinal cord injury mediated by a unique polymer scaffold seeded with neural stem cells, Proc. Natl. Acad. Sci. U. S. A., 2002, 99(5), 3024–3029 CrossRef CAS PubMed.
- J. Czyz and A. M. Wobus, Embryonic stem cell differentiation: The role of extracellular factors, Differentiation, 2001, 68(4–5), 167–174 CrossRef CAS PubMed.
- J. Santos, M. Moschetta, J. Rodrigues, P. Alpuim and A. Capasso, Interactions Between 2D Materials and Living Matter: A Review on Graphene and Hexagonal Boron Nitride Coatings, Front. Bioeng. Biotechnol., 2021, 9, 612669 CrossRef.
- A. A. Khalili and M. R. Ahmad, A Review of cell adhesion studies for biomedical and biological applications, Int. J. Mol. Sci., 2015, 16(8), 18149–18184 CrossRef CAS.
- M. Moschetta, J. Y. Lee, J. Rodrigues, A. Podestà, O. Varvicchio, J. Son, Y. Lee, K. Kim, G. H. Lee, F. Benfenati, M. Bramini and A. Capasso, Hydrogenated Graphene Improves Neuronal Network Maturation and Excitatory Transmission, Adv. Biol., 2021, 5(1), 2000177 CrossRef CAS PubMed.
- S. Huang and D. E. Ingber, The structural and mechanical complexity of cell-growth control, Nat. Cell Biol., 1999, 1(5), E131–E138 CrossRef CAS PubMed.
- S. Hong, E. Ergezen, R. Lec and K. A. Barbee, Real-time analysis of cell-surface adhesive interactions using thickness shear mode resonator, Biomaterials, 2006, 27(34), 5813–5820 CrossRef CAS PubMed.
- B. Brenner and E. Eisenberg, The Mechanism of Muscle Contraction. Biochemical, Mechanical, and Structural Approaches to Elucidate Cross-Bridge Action in Muscle, Cardiac Energetics, 1987, 3–16 CAS.
- B. B. McIntosh and E. M. Ostap, Myosin-I molecular motors at a glance, J. Cell Sci., 2016, 129(14), 2689–2695 CAS.
- S. Hirohashi and Y. Kanai, Cell Adhesion System and Human Cancer Morphogenesis, Cancer Sci., 2003, 575–581 CrossRef CAS.
- S. Y. Proskuryakov, A. G. Konoplyannikov and V. L. Gabai, Necrosis: A specific form of programmed cell death?, Exp. Cell Res., 2003, 283(1), 1–16 CrossRef CAS.
- W. C. L. Kenry, K. P. Loh and C. T. Lim, When stem cells meet graphene: Opportunities and challenges in regenerative medicine, Biomaterials, 2018, 155, 236–250 CrossRef CAS.
- S. W. Crowder, V. Leonardo, T. Whittaker, P. Papathanasiou and M. M. Stevens, Material Cues as Potent Regulators of Epigenetics and Stem Cell Function, Cell Stem Cell, 2016, 18(1), 39–52 CrossRef CAS.
- C. J. Bettinger, R. Langer and J. T. Borenstein, Engineering Substrate Topography at the Micro- and Nanoscale to Control Cell Function, Angew. Chem., Int. Ed., 2009, 48(30), 5406–5415 CrossRef CAS PubMed.
- P. M. Mendes, Cellular nanotechnology: making biological interfaces smarter, Chem. Soc. Rev., 2013, 42(24), 9207–9218 RSC.
- L. Ghasemi-Mobarakeh, Structural properties of scaffolds: Crucial parameters towards stem cells differentiation, World J. Stem Cells, 2015, 7(4), 728 CrossRef.
- T. Okano, N. Yamada, H. Sakai and Y. Sakurai, A novel recovery system for cultured cells using plasma-treated polystyrene dishes grafted with poly(N-isopropylacrylamide), J. Biomed. Mater. Res., 1993, 27(10), 1243–1251 CrossRef CAS PubMed.
- N. Yamada, T. Okano, H. Sakai, F. Karikusa, Y. Sawasaki and Y. Sakurai, Thermo-responsive polymeric surfaces; control of attachment and detachment of cultured cells, Makromol. Chem., Rapid Commun., 1990, 11(11), 571–576 CrossRef CAS.
- J. O. You, M. Rafat, D. Almeda, N. Maldonado, P. Guo, C. S. Nabzdyk, M. Chun, F. W. LoGerfo, J. W. Hutchinson, L. K. Pradhan-Nabzdyk and D. T. Auguste, pH-responsive scaffolds generate a pro-healing response, Biomaterials, 2015, 57, 22–32 CrossRef CAS PubMed.
- J. Geringer, B. Forest and P. Combrade, Fretting-corrosion of materials used as orthopaedic implants, Wear, 2005, 259(7–12), 943–951 CrossRef CAS.
- J. E. Dueber, G. C. Wu, G. R. Malmirchegini, T. S. Moon, C. J. Petzold, A. V. Ullal, K. L. J. Prather and J. D. Keasling, Synthetic protein scaffolds provide modular control over metabolic flux, Nat. Biotechnol., 2009, 27(8), 753–759 CrossRef CAS PubMed.
- B. A. Barres and Y. A. Barde, Neuronal and glial cell biology, Curr. Opin. Neurobiol., 2000, 642–648 CrossRef CAS PubMed.
- M. Kapałczyńska, T. Kolenda, W. Przybyła, M. Zajączkowska, A. Teresiak, V. Filas, M. Ibbs, R. Bliźniak, Ł. Łuczewski and K. Lamperska, 2D and 3D cell cultures – a comparison of different types of cancer cell cultures, Arch. Med. Sci., 2018, 14(4), 910–919 Search PubMed.
- L. G. Griffith and M. A. Swartz, Capturing complex 3D tissue physiology in vitro, Nat. Rev. Mol. Cell Biol., 2006, 7(3), 211–224 CrossRef CAS.
- D. Ohayon and S. Inal, Organic Bioelectronics: From Functional Materials to Next-Generation Devices and Power Sources, Adv. Mater., 2020, 32(36), 2001439 CrossRef CAS.
- G. Reina, J. M. González-Domínguez, A. Criado, E. Vázquez, A. Bianco and M. Prato, Promises, facts and challenges for graphene in biomedical applications, Chem. Soc. Rev., 2017, 46(15), 4400–4416 RSC.
- L. Ou, B. Song, H. Liang, J. Liu, X. Feng, B. Deng, T. Sun and L. Shao, Toxicity of graphene-family nanoparticles: A general review of the origins and mechanisms, Part. Fibre Toxicol., 2016, 13(1), 1–24 CrossRef.
- P. Roach, T. Parker, N. Gadegaard and M. R. Alexander, Surface strategies for control of neuronal cell adhesion: A review, Surf. Sci. Rep., 2010, 65(6), 145–173 CrossRef CAS.
- Y. Arima and H. Iwata, Effect of wettability and surface functional groups on protein adsorption and cell adhesion using well-defined mixed self-assembled monolayers, Biomaterials, 2007, 28(20), 3074–3082 CrossRef CAS PubMed.
- T. Akasaka, A. Yokoyama, M. Matsuoka, T. Hashimoto and F. Watari, Thin films of single-walled carbon nanotubes promote human osteoblastic cells (Saos-2) proliferation in low serum concentrations, Mater. Sci. Eng. C, 2010, 30(3), 391–399 CrossRef CAS.
- F. Grinnell and M. K. Feld, Fibronectin Adsorption on Hydrophilic and Hydrophobic Surfaces Detected by Antibody Binding and Analyzed during Cell Adhesion in Serum-Containing Medium, J. Biol. Chem., 1982, 4888–4893 CrossRef CAS.
- D. E. Macdonald, N. Deo, B. Markovic, M. Stranick and P. Somasundaran, Adsorption and Dissolution Behavior of Human Plasma Fibronectin on Thermally and Chemically Modified Titanium Dioxide Particles, Biomaterials, 2002, 1269–1279 CrossRef CAS PubMed.
- G. Altankov and T. H. Groth, Reorganization of substratum-bound fibronectin on hydrophilic and hydrophobic materials is related to biocompatibility, J. Mater. Sci., 1994, 732–737 CAS.
- F. Grinnell, Focal Adhesion Sites and the Removal of Substratum-bound Fibronectin, J. Cell Biol., 1986, 103, 2697–2706 CrossRef CAS.
- G. Altankov, F. Grinnell and T. Groth, Studies on the biocompatibility of materials: Fibroblast reorganization of substratum-bound fibronectin on surfaces varying in wettability, J. Biomed. Mater. Res., 1996, 30(3), 385–391 CrossRef CAS.
- E. A. Vogler, Thermodynamics of short-term cell adhesion in vitro, Biophys. J., 1988, 53(5), 759–769 CrossRef CAS PubMed.
- D. R. Absolom, C. Thomson, G. Kruzyk, W. Zingg and A. W. Neumann, Adhesion of hydrophilic particles (human erythrocytes) to polymer surfaces: Effect of pH and ionic strength, Colloids Surf., 1986, 21, 447–456 CrossRef CAS.
- D. Kelkar and A. Chattopadhyay, Membrane interfacial localization of aromatic amino acids and membrane protein function, J. Biosci., 2006, 31, 297–302 CrossRef CAS PubMed.
- J. Park, P. Kim, W. Helen, A. J. Engler, A. Levchenko and D.-H. Kim, Control of Stem Cell Fate and Function by Engineering Physical Microenvironments, Integr. Biol., 2012, 1008–1018 Search PubMed.
- G. Charras and E. Sahai, Physical influences of the extracellular environment on cell migration, Nat. Rev. Mol. Cell Biol., 2014, 15(12), 813–824 CrossRef CAS PubMed.
- R. G. Flemming, C. J. Murphy, G. A. Abrams, S. L. Goodman and P. F. Nealey, Effects of synthetic micro- and nano-structured surfaces on cell behavior, Biomaterials, 1999, 20(6), 573–588 CrossRef CAS PubMed.
- D. Dado and S. Levenberg, Cell–scaffold mechanical interplay within engineered tissue, Semin. Cell Dev. Biol., 2009, 20(6), 656–664 CrossRef CAS PubMed.
- L. Feld, L. Kellerman, A. Mukherjee, A. Livne, E. Bouchbinder and H. Wolfenson, Cellular contractile forces are nonmechanosensitive, Sci. Adv., 2023, 6(17), eaaz6997 CrossRef PubMed.
- T. M. Freyman, I. V. Yannas, R. Yokoo and L. J. Gibson, Fibroblast Contractile Force Is Independent of the Stiffness Which Resists the Contraction, Exp. Cell Res., 2002, 272(2), 153–162 CrossRef CAS PubMed.
- M. Levy-Mishali, J. Zoldan and S. Levenberg, Effect of Scaffold Stiffness on Myoblast Differentiation, Tissue Eng., Part A, 2008, 935–944 Search PubMed.
- C. S. Chen, M. Mrksich, S. Huang, G. M. Whitesides and D. E. Ingber, Geometric Control of Cell Life and Death, Science, 1997, 276(5317), 1425–1428 CrossRef CAS PubMed.
- C. M. Lo, H. B. Wang, M. Dembo and Y. L. Wang, Cell Movement Is Guided by the Rigidity of the Substrate, Biophys. J., 2000, 79(1), 144–152 CrossRef CAS PubMed.
- J. C. Courtenay, J. G. Filgueiras, E. R. deAzevedo, Y. Jin, K. J. Edler, R. I. Sharma and J. L. Scott, Mechanically robust cationic cellulose nanofibril 3D scaffolds with tuneable biomimetic porosity for cell culture, J. Mater. Chem. B, 2019, 7(1), 53–64 RSC.
- A. J. Engler, S. Sen, H. L. Sweeney and D. E. Discher, Matrix Elasticity Directs Stem Cell Lineage Specification, Cell, 2006, 126(4), 677–689 CrossRef CAS PubMed.
- J. R. Lange and B. Fabry, Cell and tissue mechanics in cell migration, Exp. Cell Res., 2013, 319(16), 2418–2423 CrossRef CAS PubMed.
- Y. Hou, L. Yu, W. Xie, L. C. Camacho, M. Zhang, Z. Chu, Q. Wei and R. Haag, Surface Roughness and Substrate Stiffness Synergize to Drive Cellular Mechanoresponse, Nano Lett., 2020, 20(1), 748–757 CrossRef CAS PubMed.
- A. M. Malek and S. Izumo, Mechanism of endothelial cell shape change and cytoskeletal remodeling in response to fluid shear stress, J. Cell Sci., 1996, 109(4), 713–726 CrossRef CAS PubMed.
- B. Majhy, P. Priyadarshini and A. K. Sen, Effect of surface energy and roughness on cell adhesion and growth – facile surface modification for enhanced cell culture, RSC Adv., 2021, 11(25), 15467–15476 RSC.
- M. Lampin, R. Warocquier-Clérout, C. Legris, M. Degrange and M. F. Sigot-Luizard, Correlation between substratum roughness and wettability, cell adhesion, and cell migration, J. Biomed. Mater. Res., 1997, 36(1), 99–108 CrossRef CAS PubMed.
- H. H. Huang, C. Te Ho, T. H. Lee, T. L. Lee, K. K. Liao and F. L. Chen, Effect of surface roughness of ground titanium on initial cell adhesion, Biomol. Eng., 2004, 21(3–5), 93–97 CrossRef CAS PubMed.
-
A. F. Von Recum, C. E. Shannon, C. E. Cannon, K. J. Long, T. G. Van Kooten and J. Meyle, Surface Roughness, Porosity, and Texture as Modifiers of Cellular Adhesion, Mary Ann Liebert, Inc., 1996 Search PubMed.
- K. Rechendorff, M. B. Hovgaard, M. Foss, V. P. Zhdanov and F. Besenbacher, Enhancement of protein adsorption induced by surface roughness, Langmuir, 2006, 22(26), 10885–10888 CrossRef CAS PubMed.
- D. D. Deligianni, N. Katsala, S. Ladas, D. Sotiropoulou, J. Amedee and Y. F. Missirlis, Effect of Surface Roughness of the Titanium Alloy Ti–6Al–4V on Human Bone Marrow Cell Response and on Protein Adsorption, Biomaterials, 2001, 1241–1251 CrossRef CAS PubMed.
- B. D. Bovan, T. W. Hummert, D. D. Dean and Z. Schwartz, Role of Material Surfaces in Regulating Bone and Cartilage Cell Response, Biomaterials, 1996, 137–146 Search PubMed.
- H. J. Schnittler, R. P. Franke, U. Akbay, C. Mrowietz and D. Drenckhahn, Improved in vitro rheological system for studying the effect of fluid shear stress on cultured cells, Am. J. Physiol. Cell Physiol., 1993, 265(1), C289–C298 CrossRef CAS PubMed.
- N. Paddillaya, A. Mishra, P. Kondaiah, P. Pullarkat, G. I. Menon and N. Gundiah, Biophysics of Cell–Substrate Interactions Under Shear, Front. Cell Dev. Biol., 2019, 7, 251 CrossRef PubMed.
- P. F. Davies, J. Zilberberg and B. P. Helmke, Spatial microstimuli in endothelial mechanosignaling, Circ. Res., 2003, 92(4), 359–370 CrossRef CAS PubMed.
- T. Ohashi and M. Sato, Remodeling of vascular endothelial cells exposed to fluid shear stress: experimental and numerical approach, Fluid Dyn. Res., 2005, 37(1–2), 40–59 CrossRef.
- C. G. Galbraith, R. Skalak and S. Chien, Shear stress induces spatial reorganization of the endothelial cell cytoskeleton, Cell Motil., 1998, 40(4), 317–330 CrossRef CAS.
- S. Hsu, R. Thakar, D. Liepmann and S. Li, Effects of shear stress on endothelial cell haptotaxis on micropatterned surfaces, Biochem. Biophys. Res. Commun., 2005, 337(1), 401–409 CrossRef CAS PubMed.
- R. E. Mott and B. P. Helmke, Mapping the dynamics of shear stress-induced structural changes in endothelial cells, Am. J. Physiol.: Cell Physiol., 2007, 293, 1616–1626 CrossRef PubMed.
- S. Pothapragada, P. Zhang, J. Sheriff, M. Livelli, M. J. Slepian, Y. Deng and D. Bluestein, A phenomenological particle-based platelet model for simulating filopodia formation during early activation, Int. J. Numer. Methods Biomed. Eng., 2015, 31(3), e02702 CrossRef PubMed.
- E. N. Olson and A. Nordheim, Linking actin dynamics and gene transcription to drive cellular motile functions, Nat. Rev. Mol. Cell Biol., 2010, 11(5), 353–365 CrossRef CAS PubMed.
- J. Lee, A. A. Abdeen and K. A. Kilian, Rewiring mesenchymal stem cell lineage specification by switching the biophysical microenvironment, Sci. Rep., 2014, 4, 5188 CrossRef CAS PubMed.
- R. Das, F. Moradi and H. Heidari, Biointegrated and Wirelessly Powered Implantable Brain Devices: A Review, IEEE Trans. Biomed. Circuits Syst., 2020, 14(2), 343–358 Search PubMed.
- L. A. Flanagan, Y.-E. Ju, B. Marg, M. Oster¢eld and P. A. Janmey, Neurite branching on deformable substrates, Neuroreport, 2002, 2411–2415 CrossRef.
- H. G. Sundararaghavan, G. A. Monteiro, B. L. Firestein and D. I. Shreiber, Neurite growth in 3D collagen gels with gradients of mechanical properties, Biotechnol. Bioeng., 2009, 102(2), 632–643 CrossRef CAS PubMed.
- S. P. Khan, G. G. Auner and G. M. Newaz, Influence of nanoscale surface roughness on neural cell attachment on silicon, Nanomedicine, 2005, 1(2), 125–129 CrossRef CAS PubMed.
- K. J. Jeon, S. H. Park, J. W. Shin, Y. G. Kang, J. S. Hyun, M. J. Oh, S. Y. Kim and J. W. Shin, Combined effects of flow-induced shear stress and micropatterned surface morphology on neuronal differentiation of human mesenchymal stemcells, J. Biosci. Bioeng., 2014, 117(2), 242–247 CrossRef CAS PubMed.
- D. Chafik, D. Bear, P. Bui, A. Patel, N. F. Jones, B. T. Kim, C. T. Hung and R. Gupta, Optimization of Schwann Cell Adhesion in Response to Shear Stress in an in Vitro Model for Peripheral Nerve Tissue Engineering, Tissue Eng., 2003, 233–241 CrossRef CAS PubMed.
-
I. B. Levitan and L. B. Kaczmarek, The Neuron: Cell and Molecular Biology, Oxford University Press, 3rd edn, 2002 Search PubMed.
- L. Sheng, I. Leshchyns’ka and V. Sytnyk, Cell Adhesion and Intracellular Calcium Signaling in Neurons, Cell Commun. Signaling, 2013, 94 CrossRef PubMed.
- R. S. Bedlack, M. Wei and M. Loew, Localized Membrane Depolarizations and Localized Calcium Influx during Ekctric Field-Guided Neurite Growth, Neuron, 1992, 393–403 CrossRef CAS PubMed.
- J. Henley and M. M. Poo, Guiding neuronal growth cones using Ca2+ signals, Trends Cell Biol., 2004, 14(6), 320–330 CrossRef CAS PubMed.
- S. Y. Park, J. Park, S. H. Sim, M. G. Sung, K. S. Kim, B. H. Hong and S. Hong, Enhanced Differentiation of Human Neural Stem Cells into Neurons on Graphene, Adv. Mater., 2011, 23(36), H263–H267 CrossRef CAS PubMed.
- L. F. Jaffe and M.-M. Poo, Neurites Grow Faster towards the Cathode than the Anode in a Steady Field, J. Exp. Zool., 1979, 115–127 CrossRef CAS PubMed.
- L. M. Y. Yu, N. D. Leipzig and M. S. Shoichet, Promoting Neuron Adhesion and Growth, Mater. Today, 2008, 36–43 CrossRef CAS.
- Y. Sun, Z. Huang, W. Liu, K. Yang, K. Sun, S. Xing, D. Wang, W. Zhang and X. Jiang, Surface coating as a key parameter in engineering neuronal network structures in vitro, Biointerphases, 2012, 7(1–4), 1–14 Search PubMed.
- Y. Sun, Z. Huang, W. Liu, K. Yang, K. Sun, S. Xing, D. Wang, W. Zhang and X. Jiang, Surface coating as a key parameter in engineering neuronal network structures in vitro, Biointerphases, 2012, 7(1–4), 1–14 Search PubMed.
- F. Veliev, A. Briançon-Marjollet, V. Bouchiat and C. Delacour, Impact of crystalline quality on neuronal affinity of pristine graphene, Biomaterials, 2016, 86, 33–41 CrossRef CAS PubMed.
- F. S. Walsh, K. F. Meiri and P. Doherty, Cell signalling and CAM-mediated neurite outgrowth, Soc. Gen. Physiol. Ser., 1997, 52, 221–226 CAS.
- W. Ma, T. Tavakoli, E. Derby, Y. Serebryakova, M. S. Rao and M. P. Mattson, Cell-extracellular matrix interactions regulate neural differentiation of human embryonic stem cells, BMC Dev. Biol., 2008, 8(1), 90 CrossRef PubMed.
- S. Goodman, R. Deutzmann and K. Mark, Two distinct cell-binding domains in laminin can independently promote non-neuronal cell adhesion and spreading, J. Cell Biol., 1987, 105, 589–598 CrossRef CAS PubMed.
- E. C. Qin, M. E. Kandel, E. Liamas, T. B. Shah, C. Kim, C. D. Kaufman, Z. J. Zhang, G. Popescu, M. U. Gillette, D. E. Leckband and H. Kong, Graphene oxide substrates with N-cadherin stimulates neuronal growth and intracellular transport, Acta Biomater., 2019, 90, 412–423 CrossRef CAS PubMed.
- Y. Sun, Z. Huang, W. Liu, K. Yang, K. Sun, S. Xing, D. Wang, W. Zhang and X. Jiang, Surface Coating as a Key Parameter in Engineering Neuronal Network Structures In Vitro, Biointerphases, 2012, 7(1), 29 CrossRef CAS PubMed.
- C. Miller, S. Jeftinija and S. Mallapragada, Synergistic Effects of Physical and Chemical Guidance Cues on Neurite Alignment and Outgrowth on Biodegradable Polymer Substrates, Tissue Eng., 2002, 8(3), 367–378 CrossRef CAS PubMed.
- T. Nakayama, T. Momoki-Soga and N. Inoue, Astrocyte-derived factors instruct differentiation of embryonic stem cells into neurons, Neurosci. Res., 2003, 46(2), 241–249 CrossRef CAS PubMed.
- D. Anton, H. Burckel, E. Josset and G. Noel, Three-dimensional cell culture: A breakthrough in vivo, Int. J. Mol. Sci., 2015, 16(3), 5517–5527 CrossRef PubMed.
- M. Srikanth and J. A. Kessler, Nanotechnology—novel therapeutics for CNS disorders, Nat. Rev. Neurol., 2012, 8(6), 307–318 CrossRef CAS PubMed.
- D. Yur, R. M. Lieser, M. O. Sullivan and W. Chen, Engineering bionanoparticles for improved biosensing and bioimaging, Curr. Opin. Biotechnol, 2021, 71, 41–48 CrossRef CAS PubMed.
- M. Biondi, F. Ungaro, F. Quaglia and P. A. Netti, Controlled drug delivery in tissue engineering, Adv. Drug Delivery Rev., 2008, 60(2), 229–242 CrossRef CAS PubMed.
- T. K. Garg, O. N. Singh, S. Arora and R. S. R. Murthy, Scaffold: a novel carrier for cell and drug delivery, Crit. Rev. Ther. Drug Carrier Syst., 2012, 29(1), 1–63 CrossRef CAS PubMed.
- B. P. Chan and K. W. Leong, Eur. Spine J., 2008, 467–479 CrossRef CAS PubMed.
- F. Rosso, G. Marino, A. Giordano, M. Barbarisi, D. Parmeggiani and A. Barbarisi, Smart materials as scaffolds for tissue engineering, J. Cell. Physiol., 2005, 203(3), 465–470 CrossRef CAS PubMed.
- M. Spector, Biomaterials-based tissue engineering and regenerative medicine solutions to musculoskeletal problems, Swiss Med. Wkly., 2006, 136(1920), 293–301 CAS.
- M. I. Echeverria Molina, K. G. Malollari and K. Komvopoulos, Design Challenges in Polymeric Scaffolds for Tissue Engineering, Front. Bioeng. Biotechnol., 2021, 9, 617141 CrossRef PubMed.
- T. T. Tran, Z. A. Hamid and K. Y. Cheong, A Review of Mechanical Properties of Scaffold in Tissue Engineering: Aloe Vera Composites, J. Phys.: Conf. Ser., 2018, 1082(1), 12080 CrossRef.
- E. Toloue, S. Karbasi, H. Salehi and M. Rafienia, Evaluation of mechanical properties and cell viability of poly (3-hydroxybutyrate)-chitosan/Al2O3 nanocomposite scaffold for cartilage tissue engineering, J. Med. Signals Sens., 2019, 9, 111 CrossRef PubMed.
- A. Samourides, L. Browning, V. Hearnden and B. Chen, The effect of porous structure on the cell proliferation, tissue ingrowth and angiogenic properties of poly(glycerol sebacate urethane) scaffolds, Mater. Sci. Eng. C, 2020, 108, 110384 CrossRef CAS PubMed.
- Z. Abpeikar, P. B. Milan, L. Moradi, M. Anjomshoa and S. Asadpour, Influence of pore sizes in 3D-scaffolds on mechanical properties of scaffolds and survival, distribution, and proliferation of human chondrocytes, Mech. Adv. Mater. Struct., 2022, 29(26), 4911–4922 CrossRef CAS.
- F. J. O’Brien, Biomaterials & scaffolds for tissue engineering, Mater. Today, 2011, 14(3), 88–95 CrossRef.
-
V. Tran and X. Wen, Rapid prototyping technologies for tissue regeneration, Rapid Prototyping of Biomaterials: Principles and Applications, 2014, pp. 97–155, ISBN: 978-0-85709-599-2.
- Optimal Properties of the Scaffold.
- P. Deb, A. B. Deoghare, A. Borah, E. Barua and S. das Lala, Scaffold Development Using Biomaterials: A Review, Mater. Today: Proc., 2018, 5(5), 12909–12919 CAS.
- Q. Zhang, H. Lu, N. Kawazoe and G. Chen, Pore size effect of collagen scaffolds on cartilage regeneration, Acta Biomater., 2014, 10(5), 2005–2013 CrossRef CAS PubMed.
- M. S. Shoichet, Polymer Scaffolds for Biomaterials Applications, Macromolecules, 2010, 43(2), 581–591 CrossRef CAS.
- S. K. L. Levengood and M. Zhang, Chitosan-based scaffolds for bone tissue engineering, J. Mater. Chem. B, 2014, 2(21), 3161–3184 RSC.
- J. Sun and H. Tan, Alginate-based biomaterials for regenerative medicine applications, Materials, 2013, 6(4), 1285–1309 CrossRef CAS PubMed.
- J. H. Cherng, S. C. Chou, C. L. Chen, Y. W. Wang, S. J. Chang, G. Y. Fan, F. S. Leung and E. Meng, Bacterial cellulose as a potential bio-scaffold for effective re-epithelialization therapy, Pharmaceutics, 2021, 13(10), 1592 CrossRef CAS PubMed.
- R. Dwivedi, S. Kumar, R. Pandey, A. Mahajan, D. Nandana, D. S. Katti and D. Mehrotra, Polycaprolactone as biomaterial for bone scaffolds: Review of literature, J. Oral Biol. Craniofac. Res., 2020, 10(1), 381–388 CrossRef PubMed.
- Z. Pan and J. Ding, Poly(lactide-co-glycolide) porous scaffolds for tissue engineering and regenerative medicine, Interface Focus, 2012, 2(3), 366–377 CrossRef PubMed.
- A. Escudero-Castellanos, B. E. Ocampo-García, M. V. Domínguez-García, J. Flores-Estrada and M. V. Flores-Merino, Hydrogels based on poly(ethylene glycol) as scaffolds for tissue engineering application: biocompatibility assessment and effect of the sterilization process, J. Mater. Sci.: Mater. Med., 2016, 27(12), 176 CrossRef PubMed.
- L. Suamte, A. Tirkey, J. Barman and P. Jayasekhar Babu, Various manufacturing methods and ideal properties of scaffolds for tissue engineering applications, Smart Mater. Manufacturing, 2023, 1, 100011 Search PubMed.
- A. H. Yusop, A. A. Bakir, N. A. Shaharom, M. R. Abdul Kadir and H. Hermawan, Porous biodegradable metals for hard tissue scaffolds: A review, Int. J. Biomater., 2012, 641430 CAS.
- X. Du, S. Fu and Y. Zhu, 3D printing of ceramic-based scaffolds for bone tissue engineering: an overview, J. Mater. Chem. B, 2018, 6(27), 4397–4412 RSC.
- C. Vyas, G. Poologasundarampillai, J. Hoyland and P. Bartolo, 3D printing of biocomposites for osteochondral tissue engineering, Biomed. Compos., 2017, 261–302 CAS.
- P. Zarrintaj, E. Zangene, S. Manouchehri, L. M. Amirabad, N. Baheiraei, M. R. Hadjighasem, M. Farokhi, M. R. Ganjali, B. W. Walker, M. R. Saeb, M. Mozafari, S. Thomas and N. Annabi, Conductive biomaterials as nerve conduits: Recent advances and future challenges, Appl Mater. Today, 2020, 20, 100784 CrossRef.
- L. R. Doblado, C. Martínez-Ramos and M. M. Pradas, Biomaterials for Neural Tissue Engineering, Front. Nanotechnol., 2021, 3, 643507 CrossRef.
- C. Raza, H. A. Riaz, R. Anjum and N. ul A. Shakeel, Repair strategies for injured peripheral nerve: Review, Life Sci., 2020, 243, 117308 CrossRef CAS PubMed.
- A. Subramanian, U. M. Krishnan and S. Sethuraman, Development of biomaterial scaffold for nerve tissue engineering: Biomaterial mediated neural regeneration, J. Biomed. Sci., 2009, 16, 108 CrossRef PubMed.
- X. Zhou, A. Yang, Z. Huang, G. Yin, X. Pu and J. Jin, Enhancement of neurite adhesion, alignment and elongation on conductive polypyrrole-poly(lactide acid) fibers with cell-derived extracellular matrix, Colloids Surf., B, 2017, 149, 217–225 CrossRef CAS PubMed.
- R. Yang, C. Xu, T. Wang, Y. Wang, J. Wang, D. Quan and D. Y. B. Deng, PTMAc-PEG-PTMAc hydrogel modified by RGDC and hyaluronic acid promotes neural stem cells’ survival and differentiation: In vitro, RSC Adv., 2017, 7(65), 41098–41104 RSC.
- M. Alrashdan, J.-C. Park, M.-A. Sung, S. Yoo, J. Jahng, T. Lee, S.-J. Kim and J. Lee, Thirty minutes of low intensity electrical stimulation promotes nerve regeneration after sciatic nerve crush injury in a rat model, Acta Neurol. Belg., 2010, 110, 168–179 Search PubMed.
- V. C. Sanchez, A. Jachak, R. H. Hurt and A. B. Kane, Biological interactions of graphene-family nanomaterials: An interdisciplinary review, Chem. Res. Toxicol., 2012, 25(1), 15–34 Search PubMed.
- F. Menaa, A. Abdelghani and B. Menaa, Graphene nanomaterials as biocompatible and conductive scaffolds for stem cells: Impact for tissue engineering and regenerative medicine, J. Tissue Eng. Regener. Med., 2015, 9(12), 1321–1338 Search PubMed.
- D. Sahni, A. Jea, J. A. Mata, D. C. Marcano, A. Sivaganesan, J. M. Berlin, C. E. Tatsui, Z. Sun, T. G. Luerssen, S. Meng, T. A. Kent and J. M. Tour, Biocompatibility of pristine graphene for neuronal interface: Laboratory investigation, J. Neurosurg. Pediatr., 2013, 11(5), 575–583 Search PubMed.
- A. Bolotsky, D. Butler, C. Dong, K. Gerace, N. R. Glavin, C. Muratore, J. A. Robinson and A. Ebrahimi, Two-Dimensional Materials in Biosensing and Healthcare: From in Vitro Diagnostics to Optogenetics and beyond, ACS Nano, 2019, 13(9), 9781–9810 CrossRef CAS PubMed.
- M. Bramini, S. Sacchetti, A. Armirotti, A. Rocchi, E. Vázquez, V. León Castellanos, T. Bandiera, F. Cesca and F. Benfenati, Graphene Oxide Nanosheets Disrupt Lipid Composition, Ca2+ Homeostasis, and Synaptic Transmission in Primary Cortical Neurons, ACS Nano, 2016, 10(7), 7154–7171 CrossRef CAS PubMed.
- M. Bramini, M. Chiacchiaretta, A. Armirotti, A. Rocchi, D. D. Kale, C. Martin, E. Vázquez, T. Bandiera, S. Ferroni, F. Cesca and F. Benfenati, An Increase in Membrane Cholesterol by Graphene Oxide Disrupts Calcium Homeostasis in Primary Astrocytes, Small, 2019, 15(15), 1900147 CrossRef PubMed.
- V. Castagnola, L. Deleye, A. Podestà, E. Jaho, F. Loiacono, D. Debellis, M. Trevisani, D. Z. Ciobanu, A. Armirotti, F. Pisani, E. Flahaut, E. Vazquez, M. Bramini, F. Cesca and F. Benfenati, Interactions of Graphene Oxide and Few-Layer Graphene with the Blood–Brain Barrier, Nano Lett., 2023, 23(7), 2981–2990 CrossRef CAS PubMed.
- B. Fadeel, C. Bussy, S. Merino, E. Vázquez, E. Flahaut, F. Mouchet, L. Evariste, L. Gauthier, A. J. Koivisto, U. Vogel, C. Martín, L. G. Delogu, T. Buerki-Thurnherr, P. Wick, D. Beloin-Saint-Pierre, R. Hischier, M. Pelin, F. Candotto Carniel, M. Tretiach, F. Cesca, F. Benfenati, D. Scaini, L. Ballerini, K. Kostarelos, M. Prato and A. Bianco, Safety Assessment of Graphene-Based Materials: Focus on Human Health and the Environment, ACS Nano, 2018, 12(11), 10582–10620 CrossRef CAS PubMed.
- M. Bramini, G. Alberini, E. Colombo, M. Chiacchiaretta, M. L. DiFrancesco, J. F. Maya-Vetencourt, L. Maragliano, F. Benfenati and F. Cesca, Interfacing Graphene-Based Materials With Neural Cells, Front. Syst. Neurosci., 2018, 12, 12 CrossRef PubMed.
- T. Kuila, S. Bose, P. Khanra, A. K. Mishra, N. H. Kim and J. H. Lee, Recent advances in graphene-based biosensors, Biosens. Bioelectron., 2011, 26(12), 4637–4648 CrossRef CAS PubMed.
- X. Sun, Z. Liu, K. Welsher, J. T. Robinson, A. Goodwin, S. Zaric and H. Dai, Nano-graphene oxide for cellular imaging and drug delivery, Nano Res., 2008, 1(3), 203–212 CrossRef CAS PubMed.
- J. Liu, L. Cui and D. Losic, Graphene and graphene oxide as new nanocarriers for drug delivery applications, Acta Biomater., 2013, 9(12), 9243–9257 CrossRef CAS PubMed.
- P. Kumar, P. Huo, R. Zhang and B. Liu, Antibacterial properties of graphene-based nanomaterials, Nanomaterials, 2019, 9(5), 737 CrossRef CAS PubMed.
- H.-W. Yang, C.-Y. Huang, C.-W. Lin, H.-L. Liu, C.-W. Huang, S.-S. Liao, P.-Y. Chen, Y.-J. Lu, K.-C. Wei and C.-C. M. Ma, Gadolinium-functionalized nanographene oxide for combined drug and microRNA delivery and magnetic resonance imaging, Biomaterials, 2014, 35(24), 6534–6542 CrossRef CAS PubMed.
-
K. S. Novoselov, A. K. Geim, S. V. Morozov, D. Jiang, Y. Zhang, S. V. Dubonos, I. V. Grigorieva and A. A. Firsov, Electric Field Effect in Atomically Thin Carbon Films, Kluwer, 2004 Search PubMed.
- S. K. Tiwari, S. Sahoo, N. Wang and A. Huczko, Graphene research and their outputs: Status and prospect, J. Sci.: Adv. Mater. Devices., 2020, 5(1), 10–29 Search PubMed.
- D. G. Papageorgiou, I. A. Kinloch and R. J. Young, Mechanical properties of graphene and graphene-based nanocomposites, Prog. Mater. Sci., 2017, 90, 75–127 CrossRef CAS.
- C. Liao, Y. Li and S. C. Tjong, Graphene nanomaterials: Synthesis, biocompatibility, and cytotoxicity, Int. J. Mol. Sci., 2018, 19(11), 3564 CrossRef PubMed.
- S. V. Morozov, K. S. Novoselov, M. I. Katsnelson, F. Schedin, D. C. Elias, J. A. Jaszczak and A. K. Geim, Giant Intrinsic Carrier Mobilities in Graphene and Its Bilayer, Phys. Rev. Lett., 2007, 016602 Search PubMed.
- K. J. Lampe, A. L. Antaris and S. C. Heilshorn, Design of three-dimensional engineered protein hydrogels for tailored control of neurite growth, Acta Biomater., 2013, 9(3), 5590–5599 CrossRef CAS PubMed.
- R. R. Nair, P. Blake, A. N. Grigorenko, K. S. Novoselov, T. J. Booth, T. Stauber, N. M. R. Peres and A. K. Geim, Fine structure constant defines visual transparency of graphene, Science, 2008, 320(5881), 1308 CrossRef CAS PubMed.
- A. C. Ferrari, F. Bonaccorso, V. Fal’ko, K. S. Novoselov, S. Roche, P. Bøggild, S. Borini, F. H. L. Koppens, V. Palermo, N. Pugno, J. A. Garrido, R. Sordan, A. Bianco, L. Ballerini, M. Prato, E. Lidorikis, J. Kivioja, C. Marinelli, T. Ryhänen, A. Morpurgo, J. N. Coleman, V. Nicolosi, L. Colombo, A. Fert, M. Garcia-Hernandez, A. Bachtold, G. F. Schneider, F. Guinea, C. Dekker, M. Barbone, Z. Sun, C. Galiotis, A. N. Grigorenko, G. Konstantatos, A. Kis, M. Katsnelson, L. Vandersypen, A. Loiseau, V. Morandi, D. Neumaier, E. Treossi, V. Pellegrini, M. Polini, A. Tredicucci, G. M. Williams, B. Hee Hong, J. H. Ahn, J. Min Kim, H. Zirath, B. J. van Wees, H. van der Zant, L. Occhipinti, A. di Matteo, I. A. Kinloch, T. Seyller, E. Quesnel, X. Feng, K. Teo, N. Rupesinghe, P. Hakonen, S. R. T. Neil, Q. Tannock, T. Löfwander and J. Kinaret, Science and technology roadmap for graphene, related two-dimensional crystals, and hybrid systems, Nanoscale, 2015, 7(11), 4598–4810 RSC.
- S. Gan, L. Zhong, D. Han, L. Niu and Q. Chi, Probing Bio-Nano Interactions between Blood Proteins and Monolayer-Stabilized Graphene Sheets, Small, 2015, 11(43), 5814–5825 CrossRef CAS PubMed.
- A. T. Smith, A. M. LaChance, S. Zeng, B. Liu and L. Sun, Synthesis, properties, and applications of graphene oxide/reduced graphene oxide and their nanocomposites, Nano Mater. Sci., 2019, 1(1), 31–47 CrossRef.
- A. Sasidharan, L. S. Panchakarla, P. Chandran, D. Menon, S. Nair, C. N. R. Rao and M. Koyakutty, Differential nano-bio interactions and toxicity effects of pristine versus functionalized graphene, Nanoscale, 2011, 3(6), 2461–2464 RSC.
- Z. Guo, S. Chakraborty, F. A. Monikh, D.-D. Varsou, A. J. Chetwynd, A. Afantitis, I. Lynch and P. Zhang, Surface Functionalization of Graphene-Based Materials: Biological Behavior, Toxicology, and Safe-By-Design Aspects, Adv. Biol., 2021, 5(9), 2100637 CrossRef CAS PubMed.
- C. Cheng, S. Li, A. Thomas, N. A. Kotov and R. Haag, Functional Graphene Nanomaterials Based Architectures: Biointeractions, Fabrications, and Emerging Biological Applications, Chem. Rev., 2017, 117(3), 1826–1914 CrossRef CAS PubMed.
- L. Yan, Y. B. Zheng, F. Zhao, S. Li, X. Gao, B. Xu, P. S. Weiss and Y. Zhao, Chemistry and physics of a single atomic layer: strategies and challenges for functionalization of graphene and graphene-based materials, Chem. Soc. Rev., 2012, 41(1), 97–114 RSC.
- A. Eftekhari and H. Garcia, The necessity of structural irregularities for the chemical applications of graphene, Mater. Today Chem., 2017, 4, 1–16 CrossRef.
- A. A. John, A. P. Subramanian, M. V. Vellayappan, A. Balaji, H. Mohandas and S. K. Jaganathan, Carbon nanotubes and graphene as emerging candidates in neuroregeneration and neurodrug delivery, Int. J. Nanomed., 2015, 10, 4267–4277 CAS.
- T. A. Mattei and A. A. Rehman, Technological Developments and Future Perspectives on Graphene-Based Metamaterials: A Primer for Neurosurgeons, Neurosurgery, 2014, 74(5), 499–516 CrossRef PubMed.
- X. Y. Wang, A. Narita and K. Müllen, Precision synthesis versus bulk-scale fabrication of graphenes, Nat. Rev. Chem., 2018, 2(1), 0100 CrossRef.
- L. Li, M. Zhou, L. Jin, L. Liu, Y. Mo, X. Li, Z. Mo, Z. Liu, S. You and H. Zhu, Research Progress of the Liquid-Phase Exfoliation and Stable Dispersion Mechanism and Method of Graphene, Front. Mater., 2019, 6, 325 CrossRef.
- X. Gu, Y. Zhao, K. Sun, C. L. Z. Vieira, Z. Jia, C. Cui, Z. Wang, A. Walsh and S. Huang, Method of ultrasound-assisted liquid-phase exfoliation to prepare graphene, Ultrason. Sonochem., 2019, 58, 104630 CrossRef CAS PubMed.
- S. Lund, J. Kauppila, S. Sirkiä, J. Palosaari, O. Eklund, R.-M. Latonen, J.-H. Smått, J. Peltonen and T. Lindfors, Fast high-shear exfoliation of natural flake graphite with temperature control and high yield, Carbon, 2021, 174, 123–131 CrossRef CAS.
- L. Sun, G. Yuan, L. Gao, J. Yang, M. Chhowalla, M. Heydari Gharahcheshmeh, K. Gleason, Y. Choi, B. Hong and Z. Liu, Chem. Vap. Depos., 2021, 1, 5 CAS.
- V. B. Mbayachi, E. Ndayiragije, T. Sammani, S. Taj, E. R. Mbuta and A. Ullah Khan, Graphene synthesis, characterization and its applications: A review, Results Chem., 2021, 3, 4347–4379 Search PubMed.
- X. Li, W. Cai, J. An, S. Kim, J. Nah, D. Yang, R. Piner, A. Velamakanni, I. Jung, E. Tutuc, S. K. Banerjee, L. Colombo and R. S. Ruoff, Large-Area Synthesis of High-Quality and Uniform Graphene Films on Copper Foils, Science, 2009, 324(5932), 1312–1314 CrossRef CAS PubMed.
- D. R. Cooper, B. D’Anjou, N. Ghattamaneni, B. Harack, M. Hilke, A. Horth, N. Majlis, M. Massicotte, L. Vandsburger, E. Whiteway and V. Yu, Experimental Review of Graphene, ISRN Condens. Matter Phys., 2012, 1–56 Search PubMed.
- Q. Yu, J. Lian, S. Siriponglert, H. Li, Y. P. Chen and S. Pei, Graphene segregated on Ni surfaces and transferred to insulators, Appl. Phys. Lett., 2008, 93, 113103 CrossRef.
- N. Lisi, T. Dikonimos, F. Buonocore, M. Pittori, R. Mazzaro, R. Rizzoli, S. Marras and A. Capasso, Contamination-free graphene by chemical vapor deposition in quartz furnaces, Sci. Rep., 2017, 7(1), 9927 CrossRef PubMed.
- B. Deng, Z. Liu and H. Peng, Toward Mass Production of CVD Graphene Films, Adv. Mater., 2019, 31(9), 1800996 CrossRef PubMed.
- A. Aryaei, A. Jayatissa and A. Jayasuriya, The effect of graphene substrate on osteoblast cell adhesion and proliferation, J. Biomed. Mater. Res., Part A, 2014, 102, 3282–3290 CrossRef PubMed.
- R. Podila, T. Moore, F. Alexis and A. M. Rao, Graphene coatings for enhanced hemo-compatibility of nitinol stents, RSC Adv., 2013, 3(6), 1660–1665 RSC.
- B. Zhang, P. Wei, Z. Zhou and T. Wei, Interactions of graphene with mammalian cells: Molecular mechanisms and biomedical insights, Adv. Drug Delivery Rev., 2016, 105, 145–162 CrossRef CAS PubMed.
- F. Lin, F. Du, J. Huang, A. Chau, Y. Zhou, H. Duan, J. Wang and C. Xiong, Substrate effect modulates adhesion and proliferation of fibroblast on graphene layer, Colloids Surf., B, 2016, 146, 785–793 CrossRef CAS PubMed.
- T. R. Nayak, H. Andersen, V. S. Makam, C. Khaw, S. Bae, X. Xu, P. L. R. Ee, J. H. Ahn, B. H. Hong, G. Pastorin and B. Özyilmaz, Graphene for controlled and accelerated osteogenic differentiation of human mesenchymal stem cells, ACS Nano, 2011, 5(6), 4670–4678 CrossRef CAS PubMed.
- M. Kalbacova, A. Broz, J. Kong and M. Kalbac, Graphene substrates promote adherence of human osteoblasts and mesenchymal stromal cells, Carbon, 2010, 48(15), 4323–4329 CrossRef CAS.
- B. Fadeel, C. Bussy, S. Merino, E. Vázquez, E. Flahaut, F. Mouchet, L. Evariste, L. Gauthier, A. J. Koivisto, U. Vogel, C. Martín, L. G. Delogu, T. Buerki-Thurnherr, P. Wick, D. Beloin-Saint-Pierre, R. Hischier, M. Pelin, F. Candotto Carniel, M. Tretiach, F. Cesca, F. Benfenati, D. Scaini, L. Ballerini, K. Kostarelos, M. Prato and A. Bianco, Safety Assessment of Graphene-Based Materials: Focus on Human Health and the Environment, ACS Nano, 2018, 12(11), 10582–10620 CrossRef CAS PubMed.
- A. Bianco, Graphene: Safe or toxic? the two faces of the medal, Angew. Chem., Int. Ed., 2013, 52(19), 4986–4997 CrossRef CAS PubMed.
- C. Martín, K. Kostarelos, M. Prato and A. Bianco, Biocompatibility and biodegradability of 2D materials: Graphene and beyond, Chem. Commun., 2019, 55(39), 5540–5546 RSC.
- C. J. Bullock and C. Bussy, Biocompatibility Considerations in the Design of Graphene Biomedical Materials, Adv. Mater. Interfaces, 2019, 6(11), 1900229 CrossRef.
- P. Wick, A. E. Louw-Gaume, M. Kucki, H. F. Krug, K. Kostarelos, B. Fadeel, K. A. Dawson, A. Salvati, E. Vázquez, L. Ballerini, M. Tretiach, F. Benfenati, E. Flahaut, L. Gauthier, M. Prato and A. Bianco, Classification framework for graphene-based materials, Angew. Chem., Int. Ed., 2014, 53(30), 7714–7718 CrossRef CAS PubMed.
- M. Bramini, G. Alberini, E. Colombo, M. Chiacchiaretta, M. L. DiFrancesco, J. F. Maya-Vetencourt, L. Maragliano, F. Benfenati and F. Cesca, Interfacing graphene-based materials with neural cells, Front. Syst. Neurosci., 2018, 12, 12 CrossRef PubMed.
- V. Castagnola, L. Deleye, A. Podestà, E. Jaho, F. Loiacono, D. Debellis, M. Trevisani, D. Z. Ciobanu, A. Armirotti, F. Pisani, E. Flahaut, E. Vazquez, M. Bramini, F. Cesca and F. Benfenati, Interactions of Graphene Oxide and Few-Layer Graphene with the Blood-Brain Barrier, Nano Lett., 2023, 23(7), 2981–2990 CrossRef CAS PubMed.
- M. Bramini, S. Sacchetti, A. Armirotti, A. Rocchi, E. Vázquez, V. León Castellanos, T. Bandiera, F. Cesca and F. Benfenati, Graphene Oxide Nanosheets Disrupt Lipid Composition, Ca2+ Homeostasis, and Synaptic Transmission in Primary Cortical Neurons, ACS Nano, 2016, 10(7), 7154–7171 CrossRef CAS PubMed.
- R. Rauti, M. Medelin, L. Newman, S. Vranic, G. Reina, A. Bianco, M. Prato, K. Kostarelos and L. Ballerini, Graphene Oxide Flakes Tune Excitatory Neurotransmission in Vivo by Targeting Hippocampal Synapses, Nano Lett., 2019, 19(5), 2858–2870 CrossRef CAS PubMed.
- M. Chiacchiaretta, M. Bramini, A. Rocchi, A. Armirotti, E. Giordano, E. Vázquez, T. Bandiera, S. Ferroni, F. Cesca and F. Benfenati, Graphene Oxide Upregulates the Homeostatic Functions of Primary Astrocytes and Modulates Astrocyte-to-Neuron Communication, Nano Lett., 2018, 18(9), 5827–5838 CrossRef CAS PubMed.
- M. Bramini, M. Chiacchiaretta, A. Armirotti, A. Rocchi, D. D. Kale, C. Martin, E. Vázquez, T. Bandiera, S. Ferroni, F. Cesca and F. Benfenati, An Increase in Membrane Cholesterol by Graphene Oxide Disrupts Calcium Homeostasis in Primary Astrocytes, Small, 2019, 15(15), 1900147 CrossRef PubMed.
- M. C. P. Mendonça, E. S. Soares, M. B. De Jesus, H. J. Ceragioli, Â. G. Batista, Á. Nyúl-Tóth, J. Molnár, I. Wilhelm, M. R. Maróstica, I. Krizbai and M. A. Da Cruz-Höfling, PEGylation of reduced graphene oxide induces toxicity in cells of the blood-brain barrier: An in vitro and in vivo study, Mol. Pharmaceutics, 2016, 13(11), 3913–3924 CrossRef PubMed.
- G. Di Mauro, R. Rauti, R. Casani, G. Chimowa, A. M. Galibert, E. Flahaut, G. Cellot and L. Ballerini, Tuning the reduction of graphene oxide nanoflakes differently affects neuronal networks in the zebrafish, Nanomaterials, 2021, 11(9), 2161 CrossRef CAS PubMed.
- M. Moschetta, J. Y. Lee, J. Rodrigues, A. Podestà, O. Varvicchio, J. Son, Y. Lee, K. Kim, G. H. Lee, F. Benfenati, M. Bramini and A. Capasso, Hydrogenated Graphene Improves Neuronal Network Maturation and Excitatory Transmission, Adv. Biol., 2021, 5(1), 2000177 CrossRef CAS PubMed.
- N. P. Pampaloni, M. Lottner, M. Giugliano, A. Matruglio, F. D’Amico, M. Prato, J. A. Garrido, L. Ballerini and D. Scaini, Single-layer graphene modulates neuronal communication and augments membrane ion currents, Nat. Nanotechnol., 2018, 13(8), 755–764 CrossRef CAS PubMed.
- K. E. Kitko, T. Hong, R. M. Lazarenko, D. Ying, Y. Q. Xu and Q. Zhang, Membrane cholesterol mediates the cellular effects of monolayer graphene substrates, Nat. Commun., 2018, 9(1), 796 CrossRef PubMed.
- B. C. Thompson, E. Murray and G. G. Wallace, Graphite Oxide to Graphene. Biomaterials to Bionics, Adv. Mater., 2015, 27, 7563–7582 CrossRef CAS PubMed.
- S. K. Rastogi, G. Raghavan, G. Yang and T. Cohen-Karni, Effect of Graphene on Nonneuronal and Neuronal Cell Viability and Stress, Nano Lett., 2017, 17(5), 3297–3301 CrossRef CAS PubMed.
- M. Devi, M. Vomero, E. Fuhrer, E. Castagnola, C. Gueli, S. Nimbalkar, M. Hirabayashi, S. Kassegne, T. Stieglitz and S. Sharma, Carbon-based neural electrodes: Promises and challenges, J. Neural Eng., 2021, 18(4), 041007 CrossRef PubMed.
- C. Heo, J. Yoo, S. Lee, A. Jo, S. Jung, H. Yoo, Y. H. Lee and M. Suh, The control of neural cell-to-cell interactions through non-contact electrical field stimulation using graphene electrodes, Biomaterials, 2011, 32(1), 19–27 CrossRef CAS PubMed.
- S. W. Hong, J. H. Lee, S. H. Kang, E. Y. Hwang, Y. S. Hwang, M. H. Lee, D. W. Han and J. C. Park, Enhanced neural cell adhesion and neurite outgrowth
on graphene-based biomimetic substrates, Biomed Res. Int., 2014, 212149 Search PubMed.
- B. Li, Y. Ma, S. Wang and P. M. Moran, Influence of carboxyl group density on neuron cell attachment and differentiation behavior: Gradient-guided neurite outgrowth, Biomaterials, 2005, 26(24), 4956–4963 CrossRef CAS PubMed.
- D. R. Nisbet, S. Pattanawong, J. Nunan, W. Shen, M. K. Horne, D. I. Finkelstein and J. S. Forsythe, The effect of surface hydrophilicity on the behavior of embryonic cortical neurons, J. Colloid Interface Sci., 2006, 299(2), 647–655 CrossRef CAS PubMed.
- J. Rafiee, X. Mi, H. Gullapalli, A. V. Thomas, F. Yavari, Y. Shi, P. M. Ajayan and N. A. Koratkar, Wetting transparency of graphene, Nat. Mater., 2012, 11(3), 217–222 CrossRef CAS PubMed.
- R. Rauti, N. Lozano, V. León, D. Scaini, M. Musto, I. Rago, F. P. Ulloa Severino, A. Fabbro, L. Casalis, E. Vázquez, K. Kostarelos, M. Prato and L. Ballerini, Graphene Oxide Nanosheets Reshape Synaptic Function in Cultured Brain Networks, ACS Nano, 2016, 10(4), 4459–4471 CrossRef CAS PubMed.
- M. Chiacchiaretta, M. Bramini, A. Rocchi, A. Armirotti, E. Giordano, E. Vázquez, T. Bandiera, S. Ferroni, F. Cesca and F. Benfenati, Graphene Oxide Upregulates the Homeostatic Functions of Primary Astrocytes and Modulates Astrocyte-to-Neuron Communication, Nano Lett., 2018, 18(9), 5827–5838 CrossRef CAS PubMed.
- J. Son, N. Buzov, S. Chen, D. Sung, H. Ryu, J. Kwon, S. Kim, S. Namiki, J. Xu, S. Hong, K. Watanabe, T. Taniguchi, W. P. King, G.-H. Lee and A. M. van der Zande, Tailoring Surface Properties via Functionalized Hydrofluorinated Graphene Compounds, Adv. Mater., 2019, 31(39), 1903424 CrossRef PubMed.
- J. Son, J.-Y. Lee, N. Han, J. Cha, J. Choi, J. Kwon, S. Nam, K.-H. Yoo, G.-H. Lee and J. Hong, Tunable Wettability of Graphene through Nondestructive Hydrogenation and Wettability-Based Patterning for Bioapplications, Nano Lett., 2020, 20(8), 5625–5631 CrossRef CAS PubMed.
- H. G. Oh, H. G. Nam, D. H. Kim, M. H. Kim, K. H. Jhee and K. S. Song, Neuroblastoma cells grown on fluorine or oxygen treated graphene sheets, Mater. Lett., 2014, 131, 328–331 CrossRef CAS.
- Y. Wang, W. C. Lee, K. K. Manga, P. K. Ang, J. Lu, Y. P. Liu, C. T. Lim and K. P. Loh, Fluorinated Graphene for Promoting Neuro-Induction of Stem Cells, Adv. Mater., 2012, 24(31), 4285–4290 CrossRef CAS PubMed.
- J. K. Chilton, Molecular mechanisms of axon guidance, Dev. Biol., 2006, 292(1), 13–24 CrossRef CAS PubMed.
- M. J. Jang and Y. Nam, Geometric effect of cell adhesive polygonal micropatterns on neuritogenesis and axon guidance, J. Neural Eng., 2012, 9(4), 046019 CrossRef PubMed.
- A. Solanki, S. Shah, K. A. Memoli, S. Y. Park, S. Hong and K. B. Lee, Controlling differentiation of neural stem cells using extracellular matrix protein patterns, Small, 2010, 6(22), 2509–2513 CrossRef CAS PubMed.
- X. Jiang, D. A. Bruzewicz, A. P. Wong, M. Piel and G. M. Whitesides, Directing Cell Migration with Asymmetric Micropatterns, PNAS, 2004, 975–978 Search PubMed.
- J. S. Da Silva and C. G. Dotti, Breaking the neuronal sphere:
regulation of the actin cytoskeleton in neuritogenesis, Nat. Rev. Neurosci., 2002, 3(9), 694–704 CrossRef CAS PubMed.
- G. S. Withers, C. D. James, C. E. Kingman, H. G. Craighead and G. A. Banker, Effects of substrate geometry on growth cone behavior and axon branching, J. Neurobiol., 2006, 66(11), 1183–1194 CrossRef PubMed.
- S. Y. Park, D. S. Choi, H. J. Jin, J. Park, K. E. Byun, K. B. Lee and S. Hong, Polarization-controlled differentiation of human neural stem cells using synergistic cues from the patterns of carbon nanotube monolayer coating, ACS Nano, 2011, 5(6), 4704–4711 CrossRef CAS PubMed.
- T. Wei, L. Bao, F. Hauke and A. Hirsch, Recent Advances in Graphene Patterning, ChemPlusChem, 2020, 85(8), 1655–1668 CrossRef CAS PubMed.
- D. A. Balikov, B. Fang, Y. W. Chun, S. W. Crowder, D. Prasai, J. B. Lee, K. I. Bolotin and H.-J. Sung, Directing lineage specification of human mesenchymal stem cells by decoupling electrical stimulation and physical patterning on unmodified graphene, Nanoscale, 2016, 8(28), 13730–13739 RSC.
- M. Lorenzoni, F. Brandi, S. Dante, A. Giugni and B. Torre, Simple and effective graphene laser processing for neuron patterning application, Sci. Rep., 2013, 3, 1954 CrossRef PubMed.
- S. Scalisi, F. Pennacchietti, S. Keshavan, N. D. Derr, A. Diaspro, D. Pisignano, A. Pierzynska-Mach, S. Dante and F. Cella Zanacchi, Quantitative super-resolution microscopy to assess adhesion of neuronal cells on single-layer graphene substrates, Membranes, 2021, 11(11), 878 CrossRef CAS PubMed.
- F. A. Saleh and P. G. Genever, Turning round: Multipotent stromal cells, a three-dimensional revolution?, Cytotherapy, 2011, 13(8), 903–912 CrossRef PubMed.
- B. A. Harley, J. H. Leung, E. C. C. M. Silva and L. J. Gibson, Mechanical characterization of collagen-glycosaminoglycan scaffolds, Acta Biomater., 2007, 3(4), 463–474 CrossRef CAS PubMed.
- Y. Qian, X. Zhao, Q. Han, W. Chen, H. Li and W. Yuan, An integrated multi-layer 3D-fabrication of PDA/RGD coated graphene loaded PCL nanoscaffold for peripheral nerve restoration, Nat. Commun., 2018, 9(1), 323 CrossRef PubMed.
- Y. Qian, J. Song, X. Zhao, W. Chen, Y. Ouyang, W. Yuan and C. Fan, 3D Fabrication with Integration Molding of a Graphene Oxide/Polycaprolactone Nanoscaffold for Neurite Regeneration and Angiogenesis, Adv. Sci., 2018, 5(4), 1700499 CrossRef PubMed.
- H. Amani, E. Mostafavi, H. Arzaghi, S. Davaran, A. Akbarzadeh, O. Akhavan, H. Pazoki-Toroudi and T. J. Webster, Three-Dimensional Graphene Foams: Synthesis, Properties, Biocompatibility, Biodegradability, and Applications in Tissue Engineering, ACS Biomater. Sci. Eng., 2019, 5(1), 193–214 CrossRef CAS PubMed.
- E. Krueger, A. N. Chang, D. Brown, J. Eixenberger, R. Brown, S. Rastegar, K. M. Yocham, K. D. Cantley and D. Estrada, Graphene Foam as a Three-Dimensional Platform for Myotube Growth, ACS Biomater. Sci. Eng., 2016, 2(8), 1234–1241 CrossRef CAS PubMed.
- Q. Ma, L. Yang, Z. Jiang, Q. Song, M. Xiao, D. Zhang, X. Ma, T. Wen and G. Cheng, Three-Dimensional Stiff Graphene Scaffold on Neural Stem Cells Behavior, ACS Appl. Mater. Interfaces, 2016, 8(50), 34227–34233 CrossRef CAS PubMed.
- N. Bahremandi Tolou, H. Salimijazi, M. Kharaziha, G. Faggio, R. Chierchia and N. Lisi, A three-dimensional nerve guide conduit based on graphene foam/polycaprolactone, Mater. Sci. Eng. C, 2021, 126, 112110 CrossRef CAS PubMed.
- N. Li, Q. Zhang, S. Gao, Q. Song, R. Huang, L. Wang, L. Liu, J. Dai, M. Tang and G. Cheng, Three-dimensional graphene foam as a biocompatible and conductive scaffold for neural stem cells, Sci. Rep., 2013, 3, 1604 CrossRef PubMed.
- N. Tasnim, V. Thakur, M. Chattopadhyay and B. Joddar, The efficacy of graphene foams for culturing mesenchymal stem cells and their differentiation into dopaminergic neurons, Stem Cells Int., 2018, 3410168 Search PubMed.
- C. Hébert, E. Masvidal-Codina, A. Suarez-Perez, A. B. Calia, G. Piret, R. Garcia-Cortadella, X. Illa, E. Del Corro Garcia, J. M. De la Cruz Sanchez, D. V. Casals, E. Prats-Alfonso, J. Bousquet, P. Godignon, B. Yvert, R. Villa, M. V. Sanchez-Vives, A. Guimerà-Brunet and J. A. Garrido, Flexible Graphene Solution-Gated Field-Effect Transistors: Efficient Transducers for Micro-Electrocorticography, Adv. Funct. Mater., 2018, 28(12), 1703976 CrossRef.
- S. Zhao, X. Liu, Z. Xu, H. Ren, B. Deng, M. Tang, L. Lu, X. Fu, H. Peng, Z. Liu and X. Duan, Graphene Encapsulated Copper Microwires as Highly MRI Compatible Neural Electrodes, Nano Lett., 2016, 16(12), 7731–7738 CrossRef CAS PubMed.
- N. Bakhshaee Babaroud, M. Palmar, A. I. Velea, C. Coletti, S. Weingärtner, F. Vos, W. A. Serdijn, S. Vollebregt and V. Giagka, Multilayer CVD graphene electrodes using a transfer-free process for the next generation of optically transparent and MRI-compatible neural interfaces, Microsyst. Nanoeng., 2022, 8(1), 107 CrossRef CAS PubMed.
- Y. Lu, X. Liu, R. Hattori, C. Ren, X. Zhang, T. Komiyama and D. Kuzum, Ultralow Impedance Graphene Microelectrodes with High Optical Transparency for Simultaneous Deep Two-Photon Imaging in Transgenic Mice, Adv. Funct. Mater., 2018, 28(31), 1800002 CrossRef PubMed.
- S. K. Rastogi, J. Bliley, D. J. Shiwarski, G. Raghavan, A. W. Feinberg and T. Cohen-Karni, Graphene Microelectrode Arrays for Electrical and Optical Measurements of Human Stem Cell-Derived Cardiomyocytes, Cell. Mol. Bioeng., 2018, 11(5), 407–418 CrossRef CAS PubMed.
- Y. Lu, X. Liu and D. Kuzum, Graphene-based neurotechnologies for advanced neural interfaces, Curr. Opin. Biomed. Eng., 2018, 6, 138–147 CrossRef.
- E. Masvidal-Codina, X. Illa, M. Dasilva, A. B. Calia, T. Dragojević, E. E. Vidal-Rosas, E. Prats-Alfonso, J. Martínez-Aguilar, J. M. De la Cruz, R. Garcia-Cortadella, P. Godignon, G. Rius, A. Camassa, E. Del Corro, J. Bousquet, C. Hébert, T. Durduran, R. Villa, M. V. Sanchez-Vives, J. A. Garrido and A. Guimerà-Brunet, High-resolution mapping of infraslow cortical brain activity enabled by graphene microtransistors, Nat. Mater., 2019, 18(3), 280–288 CrossRef CAS PubMed.
- R. Garcia-Cortadella, N. Schäfer, J. Cisneros-Fernandez, L. Ré, X. Illa, G. Schwesig, A. Moya, S. Santiago, G. Guirado, R. Villa, A. Sirota, F. Serra-Graells, J. A. Garrido and A. Guimerà-Brunet, Switchless Multiplexing of Graphene Active Sensor Arrays for Brain Mapping, Nano Lett., 2020, 20(5), 3528–3537 CrossRef CAS PubMed.
|
This journal is © The Royal Society of Chemistry 2024 |
Click here to see how this site uses Cookies. View our privacy policy here.