DOI:
10.1039/D3QI00865G
(Research Article)
Inorg. Chem. Front., 2023,
10, 3955-3962
Regulating the spin density of CoIII using boron-doped carbon dots for enhanced electrocatalytic nitrate reduction†
Received
9th May 2023
, Accepted 24th May 2023
First published on 24th May 2023
Abstract
Controlling the electron spin state on the catalyst surface can regulate the reaction activity, rate, and selectivity of surface reactions. However, there are still many challenges in exploring new strategies for electron spin regulation and mechanisms of the electron spin effect. Herein, we reported a method of using BCDs loading to change the electron spin density of magnetic Co3O4. Octahedral Co3+(Oh)–O with a t2g6eg0 configuration transformed into Co2+(Oh)–O with a t2g5eg2 configuration after BCDs loading showed excellent activity in the electrocatalytic nitrate reduction reaction, achieving a maximum NH4+ Faradaic efficiency of 94.6 ± 0.9% and high stability. Experimental results and theoretical calculations have found that high activity is attributed to the spin density regulation changing the rate-determining step and reducing the energy it needs to overcome. This work expands the spin density regulation methods and provides a new perspective for understanding the mechanisms of carbon dot materials in electrocatalytic reactions.
1. Introduction
In electrocatalytic reactions, changes in the electron spin can affect the chemical properties and reactivity of surface atoms or molecules on the catalyst, thereby affecting the rate and selectivity of the reaction.1,2 For example, on the metal surface, the change of electron spin will affect the electron cloud density and binding energy of surface atoms, thus affecting the interaction and reactivity between atoms and reactants.3 Secondly, the electron spin effect has an important impact on the reaction mechanism and reaction kinetics of catalysts. For example, in some catalytic reactions, electron spin can affect the generation and conversion of reaction intermediates, thereby affecting the rate and selectivity of the reaction.4 At the same time, electron spin can also affect the stability and activation energy of the transition state of the reaction, thus affecting the dynamic characteristics and thermodynamic stability of the reaction.5 Overall, there is a close relationship between electron spin and surface catalysis. By controlling the electron spin state on the catalyst surface, the reaction activity, rate, and selectivity of surface reactions can be regulated, providing new ideas and methods for preparing efficient and highly selective electrocatalysts.6,7
Researchers have proposed some electron spin regulation strategies, which can achieve high efficiency and selectivity of the catalyst by adjusting the state of electron spin in the catalyst.8–10 One strategy is to regulate electron spin through external electromagnetic fields.11 Researchers used external magnetic fields to regulate the state of electron spin in the catalyst, thereby controlling the catalytic efficiency and selectivity of the catalyst.12 Another strategy is to achieve the regulation of electron spin through metal doping or alloying.13 In this strategy, researchers doped other metal or semiconductor materials into the catalyst, thereby changing the state of electron spin in the catalyst and achieving the regulation of catalytic efficiency and selectivity.14 These electron spin control strategies can effectively influence the properties of catalysts and improve the performance in applications.15
Herein, we reported the discovery of a new method for altering the electron spin density using non-metallic composites. Spin density modulation of Co-based spinel oxide (Co3O4) can be achieved by loading B-doped carbon dots (BCDs), that is octahedral Co3+(Oh)–O with a t2g6eg0 electron configuration will be transformed into Co2+(Oh)–O with a high spin t2g5eg2 configuration. The spin density-regulated BCDs/Co3O4/CC catalyst exhibited excellent activity in the electrocatalytic nitrate reduction reaction (NO3RR) for ammonia synthesis,16,17 achieving a maximum NH4+ Faradaic efficiency (FE) of 94.6 ± 0.9% at −1.3 V vs. SCE and high stability. This work demonstrates the feasibility of adjusting the spin density using BCDs, displaying the ability of carbon dots to change the catalytic performance by affecting metal spin states and providing new strategies for expanding the application and universality of carbon dot materials.
2. Results and discussion
2.1. Morphology and structural characterization
Before preparing the precursor catalyst, BCDs were synthesized from C6H9BClNO2 using a hydrothermal method.18 The detailed synthesis steps are described in the ESI.† To verify the successful preparation of BCDs, we performed transmission electron microscopy (TEM) characterization. The TEM morphological image in Fig. S1† shows that BCDs have a typical shape of quantum dots with an average diameter of around 4.1 nm. X-ray diffraction (XRD) measurements in Fig. S2† displays an obvious broad diffraction peak centered at 22.8°, which corresponds to the (100) crystal plane of graphitized carbon in BCDs.19 Various related functional groups (C–C, C
C, C–N, B–O, and O–H) on the surface of BCDs were detected using Fourier transform infrared spectroscopy (FT-IR, Fig. S3†).20 Additionally, X-ray photoelectron spectroscopy (XPS) was used to further understand the chemical structure and elemental composition of BCDs. As shown in Fig. S4,† the survey spectrum displayed that the BCDs were mainly composed of C, N, O and B. In the high resolution C 1s, N 1s, O 1s and B 1s spectra, the assignment of different functional groups corresponds well with the FT-IR results.21 Based on the above results, we can confirm the successful preparation of BCDs.
The heterostructured BCDs/Co3O4/CC electrocatalyst was prepared by a two-step process as illustrated in Fig. 1a. First, a precursor catalyst BCDs/Co(OH)x/CC supported on carbon cloth was synthesized through a hydrothermal method. The scanning electron microscopy (SEM) image in Fig. 1b shows the morphology of nanowires grown on carbon cloth fibers. Then, the as-prepared precursor catalyst was annealed at 450 °C for 6 h under an Ar atmosphere to obtain the BCDs/Co3O4/CC electrocatalyst. The SEM image of BCDs/Co3O4/CC in Fig. 1c still displays the morphology of nanowires without significant surface changes, which is similar to the changes in the control sample of Co3O4/CC (Fig. S5†). In order to further analyze the surface morphology and structure of BCDs/Co3O4/CC, we conducted TEM characterization. As shown in Fig. 1d, the primary structure of the nanowire morphology is nanoparticles with a diameter of 50–100 nm, which is connected one by one. Such a morphology is conducive to exposing more active sites than smooth nanowires. The high-resolution TEM (HRTEM) image shows that the sample has two distinct regions (Fig. 1e). One is the inner region with a highly crystalline lattice. The lattice spacing is 0.24 nm, corresponding to the (311) crystal plane of Co3O4.22,23 The other region is a carbonized surface layer, which is formed by the BCDs at high annealing temperatures. In addition, we analyzed the composition and distribution of elements in the sample, and the results shows that Co, O, C, and B were evenly distributed on the catalyst (Fig. 1f).
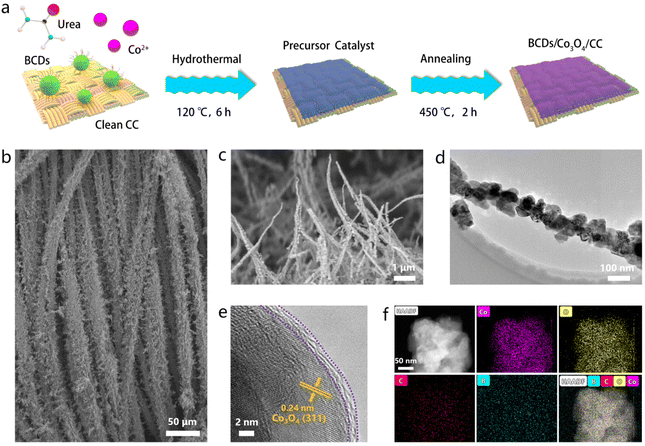 |
| Fig. 1 Synthesis and characterization of BCDs/Co3O4/CC. (a) Schematic illustration of the synthesis of BCDs/Co3O4/CC. (b) SEM image of the precursor catalyst. (c–e) SEM, TEM and HRTEM images of BCDs/Co3O4/CC. (f) Element mapping images of BCDs/Co3O4/CC. | |
As a comparison, we also prepared Co3O4/CC using the same method without adding BCDs as a reactant. The XRD patterns of BCDs/Co3O4/CC, Co3O4/CC and CC are shown in Fig. S6a.† The diffraction peaks centered at 31.3, 36.9, 59.5 and 65.3° correspond to the Co3O4 phase (JCPDS No. 73-1701),24 while the diffraction peak of graphitized carbon in the BCDs is covered by the broad diffraction peak of carbon cloth in the range of 20–30°. Raman tests were also used to analyze the structure of the three samples (Fig. S6b†). CC showed characteristic D and G peak signals at 1355 and 1601 cm−1, respectively. After loading Co3O4 on CC, additional signals caused by Co3O4 appear in the region of 400–700 cm−1.25 Furthermore, XPS was used to analyze the element composition and surface chemical states of the catalyst. The survey spectrum of BCDs/Co3O4/CC shows the presence of Co, C, O, and B in the catalyst (Fig. S7†). The high-resolution Co 2p XPS spectra of BCDs/Co3O4/CC and Co3O4/CC in Fig. 2a show two main peaks (denoted by grey) at 781.6 and 797.1 eV, which could be ascribed to the Co 2p3/2 and Co 2p1/2 of Co2+ species.26 The other two peaks denoted by red can be attributed to Co3+ species. In addition, the shake-up process of Co2+ species in high spin states leads to the appearance of two adjacent satellite peaks centered at 787.4 and 802.9 eV.14 The proportion of Co3+ and Co2+ species in Co3O4/CC was calculated to be 62.1% and 37.9% (Fig. 2b), respectively, while this proportion changed to 34.4% and 65.6% in BCDs/Co3O4/CC (Fig. 2c). This result indicates an increased proportion of Co2+ species as a function of BCDs loading. In addition, the O 1s XPS spectra display peaks at 533.1, 530.9 and 529.3 eV due to C
O species, surface –OH groups and the lattice oxygen of Co–O in Co3O4, respectively (Fig. 2d).27 Notably, the characteristic peak of B–O centered at 531.8 eV in BCDs/Co3O4/CC can be observed, further indicating the successful loading of BCDs. Moreover, the B 1s XPS spectrum of BCDs/Co3O4/CC also confirm the above results (Fig. 2e).28
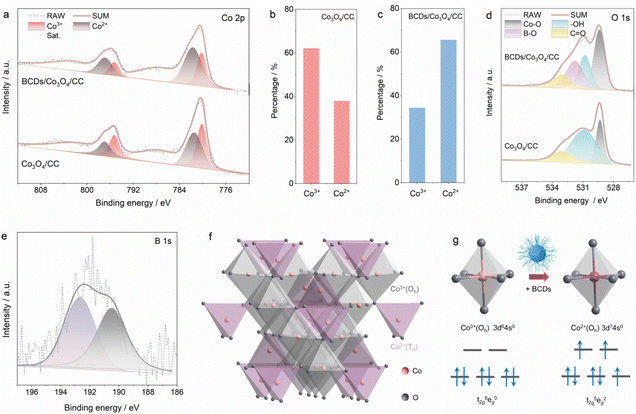 |
| Fig. 2 Surface property characterization. (a) XPS spectra of the Co 2p region of the Co3O4/CC and BCDs/Co3O4/CC. (b and c) Calculated ratio of Co3+ and Co2+ in Co3O4/CC and BCDs/Co3O4/CC, respectively. (d) O 1s region of Co3O4/CC and BCDs/Co3O4/CC. (e) B 1s region of BCDs/Co3O4/CC. (f) Crystal structure of Co3O4. (g) Scheme of the valence electron configurations and spin states of octahedral Co influenced by the BCDs. | |
On the basis of the above analysis, the valence electron configurations of Co with and without BCDs in Co3O4 are identified. Typically, as illustrated in Fig. 2f, Co3O4 has two types of Co–O species, that is, octahedral Co3+(Oh)–O coordinated by six oxygen atoms (denoted by grey) and tetrahedral Co2+(Td)–O coordinated by four oxygen atoms (denoted by purple).29 The theoretical proportion of Co2+ in Co3O4 inferred from the above crystal structure is 33.3%, which is close to the Co2+ proportion of 37.9% in Co3O4/CC. However, this proportion underwent a significant change to 65.6% after the loading of BCDs, indicating that the BCDs can act as an electron bridge to accelerate electron transfer from BCDs to Co3+(Oh), forming the Co2+(Oh) species. The valence electron configuration of Co3+(Oh) in Co3O4/CC is t2g6eg0, while Co2+(Oh) in BCDs/Co3O4/CC possesses a t2g5eg2 configuration with three unpaired electrons, as illustrated in Fig. 2g.30 Specifically, the π-symmetry t2g of Co3+(Oh) is fully occupied, which would result in electron repulsion between Co3+ and the reactant.7 As for the unpaired electron in Co2+(Oh), π-donation via O–Co–O would be strengthened by BCDs loading. In summary, the BCDs can alter the electronic structure of Co3O4 and regulate the spin state of Co3+(Oh) to active Co2+(Oh) species.
2.2. Electrocatalytic performances
The spin density-regulated BCDs/Co3O4/CC electrocatalyst was applied for nitrate reduction reaction measurements. We used a typical H-type three electrode reactor for performance testing, where 50 mL of 0.5 M K2SO4 and 0.5 M K2SO4 + 200 ppm NO3−–N electrolyte were added to the anode and cathode chambers, respectively.31–33 As shown in Fig. 3a, there is no significant difference between the linear sweep voltammetry (LSV) curves of CC before and after the addition of nitrate, which indicates that the influence and contribution of the substrate material to the reaction performance are basically zero. As for the BCDs/Co3O4/CC and Co3O4/CC electrocatalysts, the current density of the LSV curves significantly increased after the adding of nitrate. Meanwhile, BCDs/Co3O4/CC shows a higher current density compared to Co3O4/CC. Additionally, the catalytic performance of BCDs/CC was also tested (Fig. S8†), and the low current density indicated that the activity of single-component BCDs was weak in electrocatalytic nitrate-to-ammonia conversion. The above results revealed that nitrate participates in the reaction and the spin density of Co3O4 regulated by BCDs effectively improves the performance of the reaction.34 Then, we tested nitrate reduction at fixed potentials, and measured the concentration of each ion in the electrolyte after the reaction using ion chromatography (IC, Fig. S9–S11†).35,36 As displayed in Fig. 3b, with the increase of applied potentials, the FE of NH4+ exhibits a volcanic trend of first increasing and then decreasing, and can reach a maximum FE of 94.6 ± 0.9% at −1.3 V vs. SCE. Furthermore, the NH4+ generation rate gradually increases with the lowering of potentials, reaching 193.3 μmol h−1 cm−2 at −1.4 V vs. SCE (Fig. 3c).
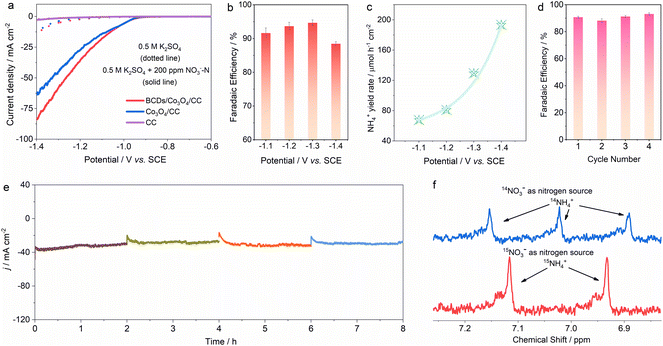 |
| Fig. 3 Electrocatalytic nitrate reduction performances. (a) LSV curves of BCDs/Co3O4/CC, Co3O4/CC, and CC. (b and c) NH4+ FEs and NH4+ yield rates of BCDs/Co3O4/CC at different applied potentials. (d and e) Stability test (repeated for four times) and the corresponding NH4+ FEs of BCDs/Co3O4/CC at a potential of −1.3 V vs. SCE. (f) 1H NMR spectra of electrocatalytic nitrate-to-ammonia conversion on BCDs/Co3O4/CC at −1.3 V vs. SCE by using 14NO3− and 15NO3− as the nitrogen source. | |
In order to determine whether the spin density-regulated BCDs/Co3O4/CC electrocatalyst can work effectively for a long time, we conducted stability tests at −1.3 V vs. SCE.37 As shown in Fig. 3d and e, under four consecutive cyclic tests, the FE of NH4+ and the current density remained basically unchanged, indicating that the BCDs/Co3O4/CC electrocatalyst can maintain good stability during the electrocatalytic process. In addition, to confirm that the ammonia generated during the reaction process comes from the reduction of nitrate, we used isotope labeling experiments to detect the source of nitrogen in the ammonia.38–40 Under the same reaction conditions, 15NO3− was used to replace the original 14NO3− as the reactant, and the ammonia was generated by a continuous reaction at −1.3 V vs. SCE. When 14NO3− was used, the 1H nuclear magnetic resonance (NMR, 600 MHz) spectrum showed a typical triple peaks for 14NH4+. When 15NO3− was used, the 1H NMR spectrum displayed a double peaks for 15NH4+ (Fig. 3f).41 Therefore, we determined that the NH4+ obtained by the reaction was reduced from the added NO3−, excluding the interference of the reaction system or other nitrogen-containing compounds.
2.3. Density functional theory (DFT) calculations
DFT calculations were utilized to further understand the activity of BCDs/Co3O4/CC for the electrocatalytic NO3RR.42 First, we calculated the direction and amount of charge transfer on the composite catalyst using Bader charge analysis. As shown in Fig. 4a, the B atoms will transfer 1.76 and 1.82 electrons to the adjacent metal Co on the BCDs/Co3O4/CC catalyst. Therefore, BCDs can serve as a bridge for electron transfer from B atoms to the octahedral Co3+(Oh), forming active high-spin Co2+(Oh) with a t2g5eg2 configuration.43 Furthermore, thecompetitive hydrogen evolution reaction is the main reason for the decrease in the FE of ammonia in the electrocatalytic NO3RR.44 Therefore, we also calculated the adsorption energy for the interaction between nitrate and H+ on the catalyst surface. As displayed in Fig. 4b, the adsorption energies for nitrate on BCDs/Co3O4/CC and Co3O4/CC are 3.00 and 2.47 eV, respectively, indicating that BCDs/Co3O4/CC has a stronger adsorption effect on nitrate. This is beneficial for the adsorption and occupation of nitrate on the catalyst surface and improves the catalytic reaction performance. Meanwhile, the adsorption energies for H+ on BCDs/Co3O4/CC and Co3O4/CC were 0.32 and 0.19 eV, respectively, much lower than the adsorption energies of nitrate. This indicates that the hydrogen evolution reaction can be effectively suppressed on the surfaces of these two catalysts, which is consistent with the high FE of the test results.
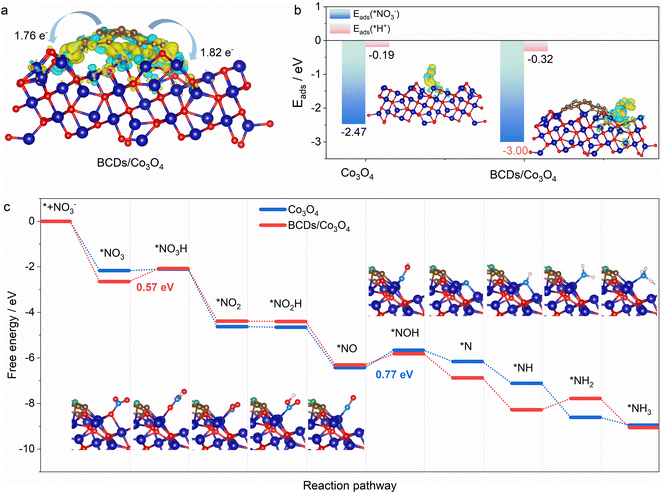 |
| Fig. 4 Mechanism investigation. (a) Charge density difference and Bader charge of BCDs/Co3O4. The yellow and blue electronic clouds indicate charge accumulation and depletion. (b) The adsorption energy for NO3− and H+ on Co3O4 and BCDs/Co3O4. Insets show the charge-density difference for NO3− adsorption. (c) Free-energy diagrams of nitrate reduction to ammonia on the surface of Co3O4 and BCDs/Co3O4. Insets show the optimized configurations for adsorbates on the surface of BCDs/Co3O4. | |
Based on our experimental testing and computational findings, we have deduced a possible reaction pathway for nitrate reduction to generate ammonia (Fig. 4c).45 The most favorable pathway is as follows:
For the Co3O4/CC catalyst without spin regulation, the rate-determining step of the reaction is the conversion of NO* to NOH*, while for the BCDs/Co3O4/CC catalyst with spin regulation, the rate-determining step of the reaction is the hydrogenation of NO3*. Therefore, for BCDs/Co3O4/CC, the rate-determining step has changed and the energy to be overcome in this step has also decreased, resulting in a significantly improved electrocatalytic performance.
3. Conclusion
In summary, we report a method of using BCDs loading to alter the electron spin density of magnetic metal oxides. Octahedral Co3+(Oh)–O with a t2g6eg0 electronic configuration transforms into octahedral Co2+(Oh)–O with a t2g5eg2 configuration after BCDs loading. The BCDs/Co3O4/CC catalyst with spin density regulation showed excellent activity in the electrocatalytic NO3RR for ammonia synthesis, achieving a maximum NH4+ FE of 94.6 ± 0.9% and high stability at −1.3 V vs. SCE. The high activity is attributed to the spin density regulation changing the rate-determining step and reducing the energy it needs to overcome. This work expands the methods of spin density regulation and provides a new perspective on the mechanism of carbon dot materials in catalytic reactions.
Author contributions
Conceptualization: J.C. and S.L.; methodology: J.Y. and A.C.; investigation: J.H., X.L., H.S., and Y.W.; writing – original draft: J.H. and X.L.; writing – review and editing: J.C., S.Q.Z., and S.L.; funding acquisition: J.C., S.L., and S.Q.Z.; supervision: S.L.
Conflicts of interest
The authors declare no competing financial interest.
Acknowledgements
This work was supported by the National Natural Science Foundation of China (No. 22205213), the China Postdoctoral Science Foundation (2020M682332, 2021T140614), the Natural Science Foundation of Henan Province (222300420287), and the Henan Postdoctoral Foundation (202003015).
References
- J. Suntivich, K. J. May, H. A. Gasteiger, J. B. Goodenough and Y. Shao-Horn, A Perovskite Oxide Optimized for Oxygen Evolution Catalysis from Molecular Orbital Principles, Science, 2011, 334, 1383–1385 CrossRef CAS PubMed.
- A. L. Buchachenko and V. L. Berdinsky, Electron Spin Catalysis, Chem. Rev., 2002, 102, 603–612 CrossRef CAS PubMed.
- J. Lv, P. Liu, R. Li, L. Wang, K. Zhang, P. Zhou, X. Huang and G. Wang, Constructing accelerated charge transfer channels along V-Co-Fe via introduction of V into CoFe-layered double hydroxides for overall water splitting, Appl. Catal., B, 2021, 298, 120587 CrossRef CAS.
- A. Cao and J. K. Nørskov, Spin Effects in Chemisorption and Catalysis, ACS Catal., 2023, 13, 3456–3462 CrossRef CAS.
- C. Y. Zhang, C. Zhang, G. W. Sun, J. L. Pan, L. Gong, G. Z. Sun, J. J. Biendicho, L. Balcells, X. L. Fan, J. R. Morante, J. Y. Zhou and A. Cabot, Spin Effect to Promote Reaction Kinetics and Overall Performance of Lithium-Sulfur Batteries under External Magnetic Field, Angew. Chem., Int. Ed., 2022, 61, e202211570 CAS.
- J. Xu, X. Zhong, X. Wu, Y. Wang and S. Feng, Optimizing the electronic spin state and delocalized electron of NiCo2(OH)x/MXene composite by interface engineering and plasma boosting oxygen evolution reaction, J. Energy Chem., 2022, 71, 129–140 CrossRef CAS.
- Z. Sun, L. Lin, J. He, D. Ding, T. Wang, J. Li, M. Li, Y. Liu, Y. Li, M. Yuan, B. Huang, H. Li and G. Sun, Regulating the Spin State of FeIII Enhances the Magnetic Effect of the Molecular Catalysis Mechanism, J. Am. Chem. Soc., 2022, 144, 8204–8213 CrossRef CAS PubMed.
- A. Cao, V. J. Bukas, V. Shadravan, Z. Wang, H. Li, J. Kibsgaard, I. Chorkendorff and J. K. Nørskov, A spin promotion effect in catalytic ammonia synthesis, Nat. Commun., 2022, 13, 2382 CrossRef CAS PubMed.
- Y. C. Liang, M. Lihter and M. Lingenfelder, Spin-Control in Electrocatalysis for Clean Energy, Isr. J. Chem., 2022, 62, e202200052 CrossRef CAS.
- Z. Li, R. Ma, Q. Ju, Q. Liu, L. Liu, Y. Zhu, M. Yang and J. Wang, Spin engineering of single-site metal catalysts, Innovation, 2022, 3, 100268 CAS.
- S. Luo, K. Elouarzaki and Z. J. Xu, Electrochemistry in Magnetic Fields, Angew. Chem., Int. Ed., 2022, 61, e202203564 CAS.
- X. Ren, T. Wu, Y. Sun, Y. Li, G. Xian, X. Liu, C. Shen, J. Gracia, H.-J. Gao, H. Yang and Z. J. Xu, Spin-polarized oxygen evolution reaction under magnetic field, Nat. Commun., 2021, 12, 2608 CrossRef CAS PubMed.
- C.-C. Lin, T.-R. Liu, S.-R. Lin, K. M. Boopathi, C.-H. Chiang, W.-Y. Tzeng, W.-H. C. Chien, H.-S. Hsu, C.-W. Luo, H.-Y. Tsai, H.-A. Chen, P.-C. Kuo, J. Shiue, J.-W. Chiou, W.-F. Pong, C.-C. Chen and C.-W. Chen, Spin-Polarized Photocatalytic CO2 Reduction of Mn-Doped Perovskite Nanoplates, J. Am. Chem. Soc., 2022, 144, 15718–15726 CrossRef CAS PubMed.
- Z. Li, Z. Wang, S. Xi, X. Zhao, T. Sun, J. Li, W. Yu, H. Xu, T. S. Herng, X. Hai, P. Lyu, M. Zhao, S. J. Pennycook, J. Ding, H. Xiao and J. Lu, Tuning the Spin Density of Cobalt Single-Atom Catalysts for Efficient Oxygen Evolution, ACS Nano, 2021, 15, 7105–7113 CrossRef CAS PubMed.
- Y. Sun, S. Sun, H. Yang, S. Xi, J. Gracia and Z. J. Xu, Spin-Related Electron Transfer and Orbital Interactions in Oxygen Electrocatalysis, Adv. Mater., 2020, 32, 2003297 CrossRef CAS PubMed.
- X. Lu, H. Song, J. Cai and S. Lu, Recent development of electrochemical nitrate reduction to ammonia: A mini review, Electrochem. Commun., 2021, 129, 107094 CrossRef CAS.
- H. Wang, J. Huang, J. Cai, Y. Wei, A. Cao, B. Liu and S. Lu, In Situ/Operando Methods for Understanding Electrocatalytic Nitrate Reduction Reaction, Small Methods, 2023, 2300169 CrossRef PubMed.
- X. Lu, J. Yu, J. Cai, Q. Zhang, S. Yang, L. Gu, G. I. N. Waterhouse, S.-Q. Zang, B. Yang and S. Lu, Exclusive nitrate to ammonia conversion via boron-doped carbon dots induced surface Lewis acid sites, Cell Rep. Phys. Sci., 2022, 3, 100961 CrossRef CAS.
- X. Miao, D. Qu, D. Yang, B. Nie, Y. Zhao, H. Fan and Z. Sun, Synthesis of Carbon Dots with Multiple Color Emission by Controlled Graphitization and Surface Functionalization, Adv. Mater., 2018, 30, 1704740 CrossRef PubMed.
- Q. Zhang, R. Wang, B. Feng, X. Zhong and K. Ostrikov, Photoluminescence mechanism of carbon dots: triggering high-color-purity red fluorescence emission through edge amino protonation, Nat. Commun., 2021, 12, 6856 CrossRef CAS PubMed.
- Y.-X. Wang, M. Rinawati, W.-H. Huang, Y.-S. Cheng, P.-H. Lin, K.-J. Chen, L.-Y. Chang, K.-C. Ho, W.-N. Su and M.-H. Yeh, Surface-engineered N-doped carbon nanotubes with B-doped graphene quantum dots: Strategies to develop highly-efficient noble metal-free electrocatalyst for online-monitoring dissolved oxygen biosensor, Carbon, 2022, 186, 406–415 CrossRef CAS.
- Y. Wang, C. Zhu, G. Zuo, Y. Guo, W. Xiao, Y. Dai, J. Kong, X. Xu, Y. Zhou, A. Xie, C. Sun and Q. Xian, 0D/2D Co3O4/TiO2 Z-Scheme heterojunction for boosted photocatalytic degradation and mechanism investigation, Appl. Catal., B, 2020, 278, 119298 CrossRef CAS.
- L. Lai, J. Zhu, Z. Li, D. Y. W. Yu, S. Jiang, X. Cai, Q. Yan, Y. M. Lam, Z. Shen and J. Lin, Co3O4/nitrogen modified graphene electrode as Li-ion battery anode with high reversible capacity and improved initial cycle performance, Nano Energy, 2014, 3, 134–143 CrossRef CAS.
- S. H. Hsu, S. F. Hung, H. Y. Wang, F. X. Xiao, L. P. Zhang, H. B. Yang, H. M. Chen, J. M. Lee and B. Liu, Tuning the Electronic Spin State of Catalysts by Strain Control for Highly Efficient Water Electrolysis, Small Methods, 2018, 2, 1800001 CrossRef.
- M. Rashad, M. Rusing, G. Berth, K. Lischka and A. Pawlis, CuO and Co3O4 Nanoparticles: Synthesis, Characterizations, and Raman Spectroscopy, J. Nanomater., 2013, 82 Search PubMed.
- W. Chu, P. A. Chernavskii, L. Gengembre, G. A. Pankina, P. Fongarland and A. Y. Khodakov, Cobalt species in promoted cobalt alumina-supported Fischer–Tropsch catalysts, J. Catal., 2007, 252, 215–230 CrossRef CAS.
- H. Wu, M. Wu, B. Wang, X. Yong, Y. Liu, B. Li, B. Liu and S. Lu, Interface electron collaborative migration of Co–Co3O4/carbon dots: Boosting the hydrolytic dehydrogenation of ammonia borane, J. Energy Chem., 2020, 48, 43–53 CrossRef.
- Y.-X. Wang, M. Rinawati, J.-D. Zhan, K.-Y. Lin, C.-J. Huang, K.-J. Chen, H. Mizuguchi, J.-C. Jiang, B.-J. Hwang and M.-H. Yeh, Boron-Doped Graphene Quantum Dots Anchored to Carbon Nanotubes as Noble Metal-Free Electrocatalysts of Uric Acid for a Wearable Sweat Sensor, ACS Appl. Nano Mater., 2022, 5, 11100–11110 CrossRef CAS.
- Z. Xiao, Y. Wang, Y.-C. Huang, Z. Wei, C.-L. Dong, J. Ma, S. Shen, Y. Li and S. Wang, Filling the oxygen vacancies in Co3O4 with phosphorus: an ultra-efficient electrocatalyst for overall water splitting, Energy Environ. Sci., 2017, 10, 2563–2569 RSC.
- C. Mu, J. Mao, J. Guo, Q. Guo, Z. Li, W. Qin, Z. Hu, K. Davey, T. Ling and S. Z. Qiao, Rational Design of Spinel Cobalt Vanadate Oxide Co2VO4 for Superior Electrocatalysis, Adv. Mater., 2020, 32, 1907168 CrossRef CAS PubMed.
- D. Qi, F. Lv, T. Wei, M. Jin, G. Meng, S. Zhang, Q. Liu, W. Liu, D. Ma, M. S. Hamdy, J. Luo and X. Liu, High-efficiency electrocatalytic NO reduction to NH3 by nanoporous VN, Nano Res. Energy, 2022, 1, e9120022 CrossRef.
- J. Cai, Y. Wei, A. Cao, J. Huang, Z. Jiang, S. Lu and S.-Q. Zang, Electrocatalytic nitrate-to-ammonia conversion with ∼100% Faradaic efficiency via single-atom alloying, Appl. Catal., B, 2022, 316, 121683 CrossRef CAS.
- Y. M. Wang, J. Cai, Q. Y. Wang, Y. Li, Z. Han, S. Li, C. H. Gong, S. Wang, S. Q. Zang and T. C. W. Mak, Electropolymerization of metal clusters establishing a versatile platform for enhanced catalysis performance, Angew. Chem., Int. Ed., 2022, 61, e202114538 CAS.
- Y. Wang, W. Zhou, R. Jia, Y. Yu and B. Zhang, Unveiling the activity origin of a copper–based electrocatalyst for selective nitrate reduction to ammonia, Angew. Chem., Int. Ed., 2020, 59, 5350–5354 CrossRef CAS PubMed.
- Y. Zhao, F. Wu, Y. Miao, C. Zhou, N. Xu, R. Shi, L. Z. Wu, J. Tang and T. Zhang, Revealing Ammonia Quantification Minefield in Photo/Electrocatalysis, Angew. Chem., Int. Ed., 2021, 60, 21728–21731 CrossRef CAS PubMed.
- Y. Zhao, R. Shi, X. Bian, C. Zhou, Y. Zhao, S. Zhang, F. Wu, G. I. N. Waterhouse, L. Z. Wu, C. H. Tung and T. Zhang, Ammonia Detection Methods in Photocatalytic and Electrocatalytic Experiments: How to Improve the Reliability of NH3 Production Rates?, Adv. Sci., 2019, 6, 1802109 CrossRef PubMed.
- F.-Y. Chen, Z.-Y. Wu, S. Gupta, D. J. Rivera, S. V. Lambeets, S. Pecaut, J. Y. T. Kim, P. Zhu, Y. Z. Finfrock, D. M. Meira, G. King, G. Gao, W. Xu, D. A. Cullen, H. Zhou, Y. Han, D. E. Perea, C. L. Muhich and H. Wang, Efficient conversion of low-concentration nitrate sources into ammonia on a Ru-dispersed Cu nanowire electrocatalyst, Nat. Nanotechnol., 2022, 17, 759–767 CrossRef CAS PubMed.
- Y. Zhang, X. Chen, W. Wang, L. Yin and J. C. Crittenden, Electrocatalytic nitrate reduction to ammonia on defective Au1Cu(111) single-atom alloys, Appl. Catal., B, 2022, 310, 121346 CrossRef CAS.
- Q. Liu, L. Xie, J. Liang, Y. Ren, Y. Wang, L. Zhang, L. Yue, T. Li, Y. Luo, N. Li, B. Tang, Y. Liu, S. Gao, A. A. Alshehri, I. Shakir, P. O. Agboola, Q. Kong, Q. Wang, D. Ma and X. Sun, Ambient Ammonia Synthesis via Electrochemical Reduction of Nitrate Enabled by NiCo2O4 Nanowire Array, Small, 2022, 18, 2106961 CrossRef CAS PubMed.
- H. Liu, X. Lang, C. Zhu, J. Timoshenko, M. Ruscher, L. Bai, N. Guijarro, H. Yin, Y. Peng, J. Li, Z. Liu, W. Wang, B. R. Cuenya and J. Luo, Efficient Electrochemical Nitrate Reduction to Ammonia with Copper-Supported Rhodium Cluster and Single-Atom Catalysts, Angew. Chem., Int. Ed., 2022, 61, e202202556 CAS.
- Q. Gao, B. Yao, H. S. Pillai, W. Zang, X. Han, Y. Liu, S.-W. Yu, Z. Yan, B. Min, S. Zhang, H. Zhou, L. Ma, H. Xin, Q. He and H. Zhu, Synthesis of core/shell nanocrystals with ordered intermetallic single-atom alloy layers for nitrate electroreduction to ammonia, Nat. Synth., 2023 DOI:10.1038/s44160-023-00258-x.
- H. Niu, Z. Zhang, X. Wang, X. Wan, C. Shao and Y. Guo, Theoretical Insights into the Mechanism of Selective Nitrate–to–Ammonia Electroreduction on Single–Atom Catalysts, Adv. Funct. Mater., 2020, 31, 2008533 CrossRef.
- R. R. Chen, Y. Sun, S. J. H. Ong, S. Xi, Y. Du, C. Liu, O. Lev and Z. J. Xu, Antiferromagnetic Inverse Spinel Oxide LiCoVO4 with Spin-Polarized Channels for Water Oxidation, Adv. Mater., 2020, 32, 1907976 CrossRef CAS PubMed.
- Y. Wang, A. Xu, Z. Wang, L. Huang, J. Li, F. Li, J. Wicks, M. Luo, D.-H. Nam, C.-S. Tan, Y. Ding, J. Wu, Y. Lum, C.-T. Dinh, D. Sinton, G. Zheng and E. H. Sargent, Enhanced nitrate-to-ammonia activity on copper–nickel alloys via tuning of intermediate adsorption, J. Am. Chem. Soc., 2020, 142, 5702–5708 CrossRef CAS PubMed.
- J. Cai, J. Huang, A. Cao, Y. Wei, H. Wang, X. Li, Z. Jiang, G. I. N. Waterhouse, S. Lu and S.-Q. Zang, Interfacial hydrogen bonding-involved electrocatalytic ammonia synthesis on OH-terminated MXene, Appl. Catal., B, 2023, 328, 122473 CrossRef CAS.
|
This journal is © the Partner Organisations 2023 |
Click here to see how this site uses Cookies. View our privacy policy here.