DOI:
10.1039/D3QI00268C
(Research Article)
Inorg. Chem. Front., 2023,
10, 2708-2715
Atomically isolated and unsaturated Sb sites created on Sb2S3 for highly selective NO electroreduction to NH3†
Received
14th February 2023
, Accepted 28th March 2023
First published on 28th March 2023
Abstract
Sb2S3 comprising an atomically isolated and unsaturated Sb (SbAIU) site is demonstrated as a fascinating catalyst for highly selective electrochemical NO-to-NH3 conversion (NORR). Theoretical calculations reveal the crucial function of SbAIU sites to favor the adsorption and activation of NO, accelerate the protonation energetics of the NO-to-NH3 pathway and impede the coverage of H2O/H species, thereby boosting both NORR activity and selectivity. Consequently, the developed SbAIU-rich Sb2S3 catalyst exhibits an excellent NO-to-NH3 faradaic efficiency of 93.7% and a high NH3 yield rate of 168.6 μmol h−1 cm−2, representing the highest NORR selectivity among all reported NORR catalysts.
1. Introduction
Ammonia is a pivotal chemical that is widely applied in many aspects of social and economic development.1–3 Recently, N2 electrofixation emerged as a promising technology for green NH3 synthesis, whereas its efficiency is greatly limited by intractable issues of ultrastable N
N bonds.4–10 Alternatively, NO possesses a relatively low N
O bond energy and thus electrochemical NO-to-NH3 conversion (NORR) represents a more prospective approach than N2 electrofixation for NH3 electrosynthesis.11–14 Nevertheless, the NORR effectiveness is greatly retarded by the sophisticated five-electron reaction process and severe competition from the hydrogen evolution reaction (HER),1 and it is imperative to explore efficient NORR electrocatalysts capable of boosting the NO-to-NH3 pathway with high selectivity.15–20
Transition metal-based catalysts commonly exhibit high NORR activity owing to their partially occupied d-orbitals boosting NO adsorption.21–27 Nevertheless, d-orbitals also favor the formation of metal–H bonds to trigger the competitive HER, giving rise to low NORR selectivity.28 Promisingly, main group p-block metals (Sb, In, Bi, etc.) are catalytically inert in the HER because of their closed d-band shells.29 Meanwhile, the partially occupied p-orbitals in p-block metals are confirmed to be active for N
O bond dissociation, making p-block metal-based materials promising as a new class of NORR catalysts.30–34 P-block Sb-based catalysts are appealing NORR candidates owing to the great capability of Sb sites to impede the HER and activate the nitrogen-containing molecules.35 On the other hand, catalysts with atomically isolated sites are known to present outstanding catalytic performance because of their high atom utilization and optimal binding with intermediates and reactants.36–38 Besides, defect engineering by creating vacancies or unsaturated sites is considered an effective strategy to tailor the electronic structure of catalysts with enhanced catalytic activities.39–41 In view of the above, creating atomically isolated and unsaturated Sb sites is therefore an attractive strategy for designing high-efficiency NORR catalysts.
In this study, p-block Sb2S3 is designed as a fascinating catalyst for highly selective NORR, which exhibits an excellent NO-to-NH3 faradaic efficiency (FENH3) of 93.7% and a high NH3 yield rate of 168.6 μmol h−1 cm−2, representing the highest NORR selectivity among all reported NORR catalysts. Detailed structural characterization and theoretical computations reveal that atomically isolated and unsaturated Sb sites created on Sb2S3 play a crucial role in greatly enhancing the NORR activity and selectivity.
2. Results and discussion
A solvothermal method was utilized to synthesize Sb2S3. The XRD pattern of the as-synthesized Sb2S3 (Fig. 1a) shows distinct peaks that are assigned to the pure orthorhombic Sb2S3 phase with a good crystallinity. The SEM image of Sb2S3 (Fig. 1b) shows a typical nanoflower morphology consisting of numerous vertically aligned nanosheets. The nanosheet feature can be further confirmed by the TEM image (Fig. 1c). As shown in the HRTEM image (Fig. 1c, inset), the lattice spacing of Sb2S3 nanosheets is determined to be 0.352 nm, corresponding to the (130) facet of orthorhombic Sb2S3, in line with the XRD result (Fig. 1a). The XPS Sb spectrum of Sb2S3 (Fig. 1d) can be split into Sb3+2p3/2 (539.3 eV) and Sb3+2p5/2 (529.8 eV), while the deconvolution of the S2p spectrum (Fig. S1†) shows two peaks of S2p1/2 (163.6 eV) and S2p3/2 (161.8 eV), in good accordance with those reported for Sb2S3.42–44
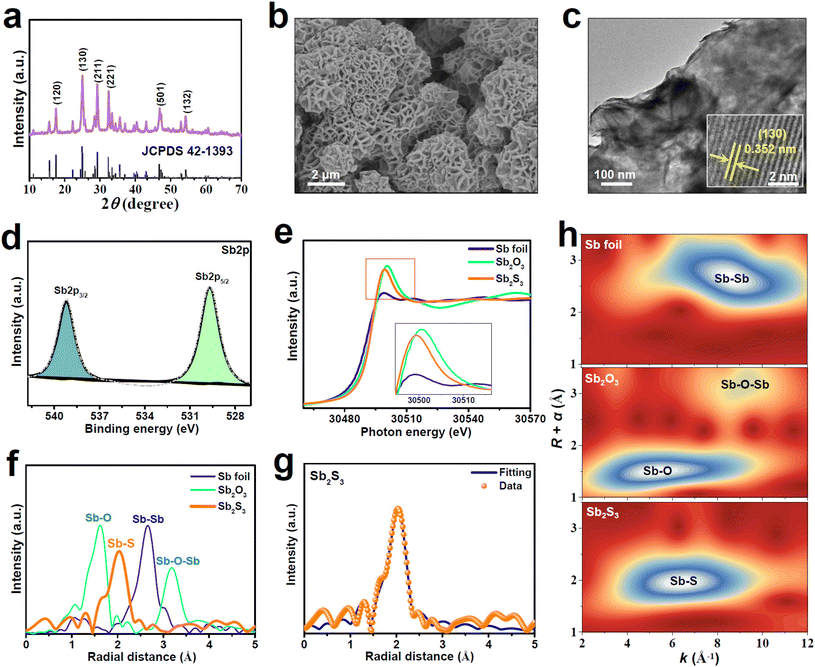 |
| Fig. 1 Characterization of Sb2S3: (a) XRD pattern, (b) SEM image, (c) TEM image and HRTEM image (inset), (d) XPS Sb2p spectrum, (e) Sb K-edge XANES spectra, (f) EXAFS spectra and (h) WT profiles of Sb2S3 and reference samples. (g) EXAFS fitting curve of Sb2S3. | |
We employ X-ray absorption near-edge structure (XANES) and extended X-ray absorption fine structure (EXAFS) characterizations to further examine the valence states and coordination structures of Sb2S3. The Sb K-edge XANES spectra (Fig. 1e) show that the white line of Sb2S3 is slightly lower than that of Sb2O3, suggesting the valence state of Sb to be smaller than the intrinsic Sb valence of Sb2S3 (+3), which is caused by the presence of coordinatively unsaturated Sb sites in Sb2S3. The Sb K-edge EXAFS spectra (Fig. 1f) show that Sb2S3 exhibits a dominant peak at 2.03 Å assignable to the Sb–S bond, which largely differs from those of Sb foil (Sb–Sb: 2.66 Å) and Sb2O3 (Sb–O: 1.62 Å, Sb–O–Sb: 3.18 Å), indicating that Sb2S3 comprises the isolated state of Sb and no oxidized Sb species are present on Sb2S3. Likewise, the corresponding wavelet transform (WT) contour plots (Fig. 1h) show only one intensity maximum at 6.3 Å−1 corresponding to the Sb–S coordination, suggesting the existence of atomically dispersed Sb atoms. The EXAFS fitting data (Fig. 1g and Table S1†) reveal the average coordination number (CN) of Sb2S3 to be 4.2, much smaller than the crystallographic value of Sb2S3 (CN = 5),45 corroborating the existence of plentiful unsaturated Sb sites in Sb2S3. These XAS results reveal that the prepared Sb2S3 naturally contains abundant atomically isolated and unsaturated Sb (SbAIU) sites, which are considered to be catalytically active towards NORR.
Electrocatalytic NORR measurements were carried out using a gas-tight H-type electrolytic cell containing 0.5 M Na2SO4 solution.46 Several colorimetric approaches (Fig. S2 and S3†) were performed to detect the liquid products, while the gaseous products were detected by gas chromatography. We conducted linear sweep voltammetry (LSV) measurement to initially assess the NORR activity of Sb2S3. It is displayed in Fig. 2a that Sb2S3 presents a noticeable current density (j) enhancement in the NO-saturated electrolyte relative to the Ar-saturated one, proving that Sb2S3 has a high NORR activity. We then quantitatively determined the NORR performance of Sb2S3 with the integration of chronoamperometry (Fig. 2b) and colorimetric tests at various potentials. As shown in Fig. 2c, with increasing the potential, both the NH3 yield rate and FENH3 of Sb2S3 exhibit a volcanic shape and reach their highest values of 168.6 μmol h−1 cm−2 and 93.7% at −0.7 V, respectively. Strikingly, as shown in Fig. 2d (see Table S2 for details†), the FENH3 of Sb2S3 shows the highest NORR selectivity among all the reported NORR catalysts, while its NH3 yield rate is also superior to those of most reported NORR catalysts. Meanwhile, Fig. 2e shows that the FEs of N-containing side products (N2O and N2H4) are rather low at all considered potentials, in good accordance with the partial current density data (Fig. S4†), signifying the outstanding NO-to-NH3 selectivity of Sb2S3. Regarding the NORR stability, the chronopotentiometric test presents a stable current density for at least 20 h of electrolysis (Fig. S5†), and the resulting FENH3 shows very small attenuations, indicating the good long-term stability of Sb2S3. Besides, no remarkable fluctuations in the NH3 yield rate and FENH3 occur during the seven electrolysis cycles (Fig. S6†), proving the favorable cycling stability of Sb2S3.47–50
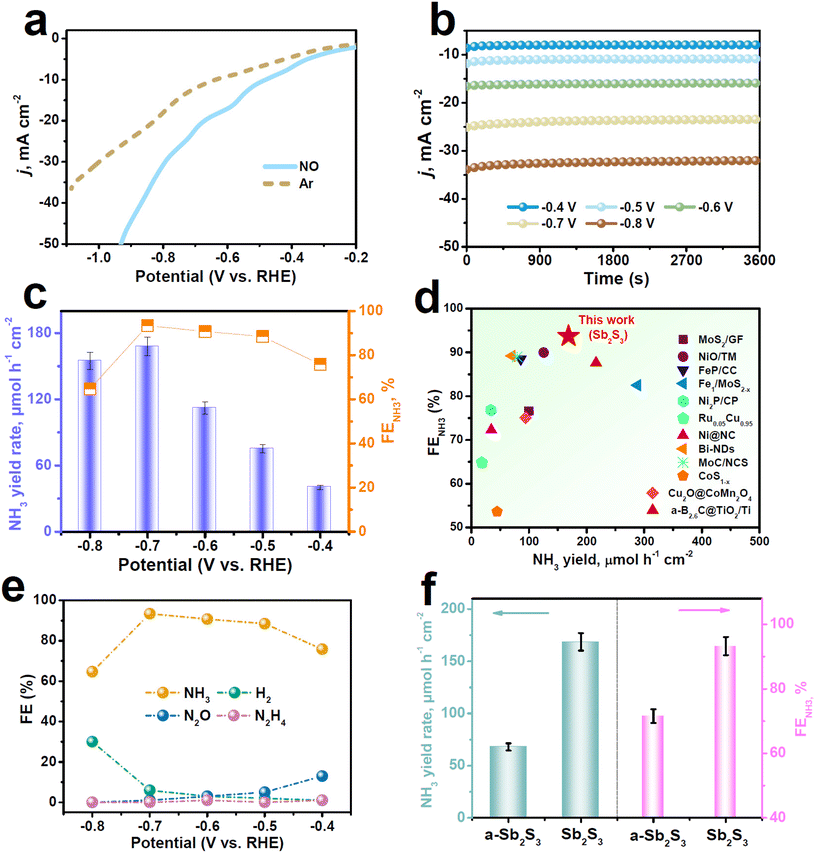 |
| Fig. 2 (a) LSV curves of Sb2S3 in Ar/NO-saturated 0.5 M Na2SO4. (b) Chronoamperometry test of Sb2S3 at various potentials, and the resulting (c) NH3 yield rates and FENH3. (d) Comparison of NH3 yield rates and FENH3 between Sb2S3 and the recently reported NORR catalysts. (e) FEs of different products on Sb2S3 after NORR electrolysis at various potentials. (f) NH3 yield rates and FENH3 of Sb2S3 and a-Sb2S3 at −0.7 V. | |
We conducted several experiments to verify the NH3 origin. First, NH3 is almost undetectable in the control colorimetric tests (Fig. S7†).32 In addition, upon feeding 15NO gas, the resulting 1H nuclear magnetic resonance (NMR, Fig. S8†) spectra reveal the characteristic 15NH4+ doublets, whereas feeding Ar gas leads to the absence of 15NH4+ doublets.51–53 Furthermore, the switching NO–Ar test (Fig. S9†) reveals significant NH3 production in NO cycles, whereas NH3 is nearly undetectable in Ar cycles. All these results validate that the produced NH3 stems from the electrochemical NORR process catalyzed by Sb2S3.
For comparison, we evaluated the NORR property of annealed Sb2S3 (a-Sb2S3) with much reduced SbAIU (Fig. S10 and Table S1†) under identical measurement conditions at −0.7 V. Impressively, Fig. 2f shows that the NORR performance of a-Sb2S3 is significantly poorer than that of the original Sb2S3, revealing that the SbAIU sites play a vital role in dramatically boosting the NORR property of Sb2S3. Electrochemical surface area (ECSA, Fig. S11 and S12†) measurements show that the ECSA-normalized NORR performances of the two catalysts (Fig. S13†) present the same trend as that shown in Fig. 2f. Besides, both catalysts have comparable charge transport kinetics (Fig. S14†).54–57 These findings demonstrate the intrinsic superior NORR property of Sb2S3.
Theoretical computations were carried out to shed light on the boosted NORR property of Sb2S3. To start, we evaluated the adsorption behaviors of the NO molecule on two sites of Sb2S3, namely the pristine Sb (SbP) site and the SbAIU site, as the NO adsorption is the initial critical step to trigger the NORR.46 Upon absorbing NO on the SbP site (Fig. 3a), *NO exhibits a rather small N
O elongation (1.163 Å, 1.159 Å for original NO) with negligible Sbp-to-*NO electron transfer (−0.02 |e|), which means poor NO adsorption on the SbP site. As a sharp comparison, *NO on the SbAIU site (Fig. 3b) presents dramatic N
O bond elongation (1.205 Å) and SbAIU-to-*NO electron transfer (−0.13 |e|), indicating largely improved NO adsorption on SbAIU. Additionally, the charge density difference (Fig. 3c) clearly shows strong *NO/SbAIU electronic interactions, where both remarkable positive and negative charge aggregations can be seen on *NO, proving that SbAIU enables powerful NO activation via a “donation–backdonation” mechanism.
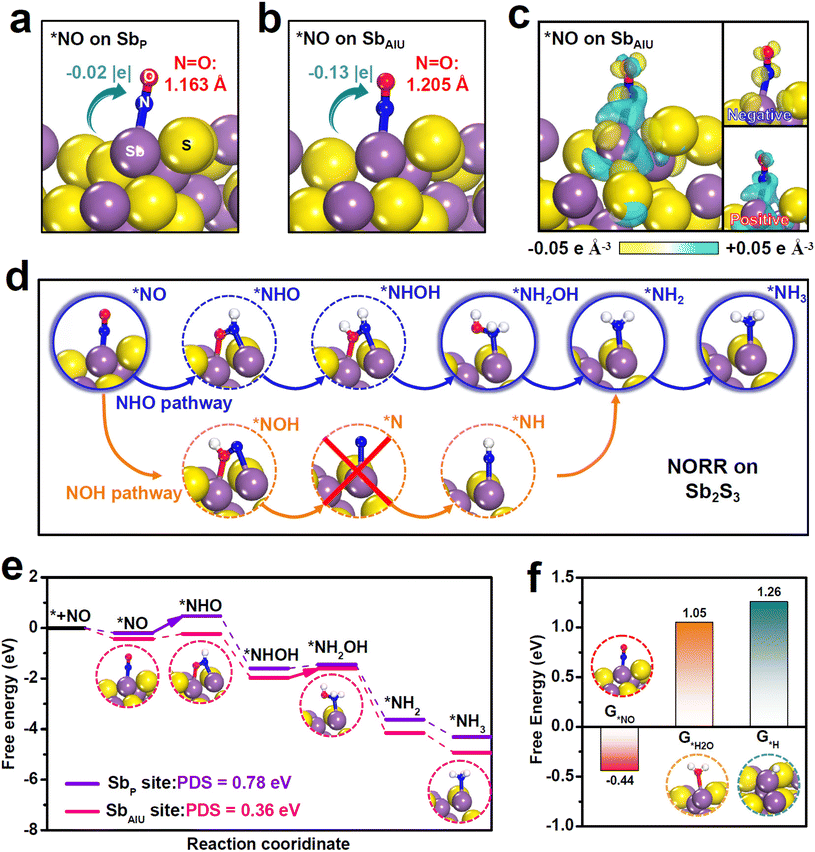 |
| Fig. 3 (a and b) Atomic structures of absorbed NO on (a) the SbP site and (b) the SbAIU site of Sb2S3. (c) Charge density difference of *NO on the SbAIU site (yellow: accumulation; cyan: depletion). (d) Schematic of two NORR pathways (NHO and NOH) on Sb2S3. (e) Free energy profiles of the NORR process (NHO pathway) on SbP and SbAIU. (f) Binding free energies of *H2O, *H and *NO on SbAIU. | |
To investigate the entire NORR process, we initially conducted online differential electrochemical mass spectrometry (DEMS) measurements to experimentally probe the reaction intermediates formed on Sb2S3 during the NORR electrolysis. The online DEMS spectra (Fig. S15†) reveal the generation of distinct NH3 (m/z = 17) and NH2OH (m/z = 33) signals. Specifically, N (m/z = 14), which is the key intermediate involved in the NOH pathway, is absent in the NORR electrolysis, demonstrating that Sb2S3 preferentially undergoes the NHO pathway to drive the NORR process,58 as illustrated in Fig. 3d. As displayed in the free energy profiles of the energetic-preferred NHO pathway (Fig. 3e and Fig. S16†), the Sbp site exhibits a large energy barrier of 0.78 eV to drive the first protonation step of *NO → *NOH as the potential-determining step (PDS). In stark contrast, by virtue of powerful NO activation, the SbAIU site presents a largely reduced barrier of 0.21 eV for the same *NO → *NOH, suggesting that the initial protonation step can be greatly boosted on the SbAIU site. The PDS of SbAIU is changed to *NHOH → *NH2OH with only 0.36 eV uphill, corroborating the significantly enhanced NORR energetics over the SbAIU site that renders a high NORR activity of Sb2S3. We then investigated the catalytic behavior of the SbAIU site towards the HER, which is the main competitive reaction for NORR.24 The calculated binding free energies (G) of various species (Fig. 3f) show that G*NO (−0.44 eV) is much more negative than G*H2O (1.05 eV) and G*H (1.26 eV), demonstrating that the SbAIU site preferentially absorbs NO over H2O/H species to impede the competing HER.
Molecular dynamics (MD) simulations were conducted to further examine the competitive adsorption of NO and H2O/H on SbAIU. After simulation, the snapshots (Fig. 4a) show prominent NO aggregation on SbAIU together with an enhanced SbAIU–*NO interaction over SbAIU–*H2O and SbAIU–*H interactions, as displayed in the radial distribution function (RDF, Fig. 4b) curves and the corresponding integrated RDF curves (Fig. 4c),58–62 proving a high tendency of SbAIU for the adsorption and coverage of NO over H2O/H, which is greatly favorable for HER suppression to obtain a high NORR selectivity.
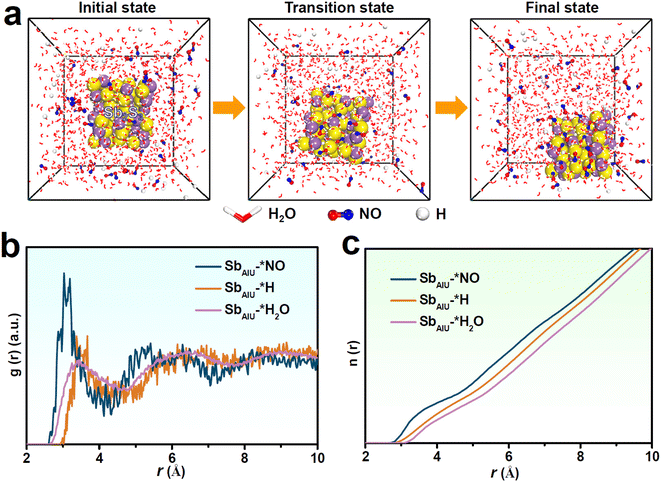 |
| Fig. 4 (a) Initial, transition and final simulated states of the dynamic adsorption process of *H2O, *H and *NO on SbAIU, and the corresponding (b) RDF and (c) integrated RDF curves of the interactions between SbAIU and *NO, *H and *H2O. | |
3. Conclusion
In summary, SbAIU-rich Sb2S3 has been corroborated as a high-performing p-block metal catalyst for NORR. Theoretical computations reveal the critical role of SbAIU sites in promoting the activation and protonation of NO, while concurrently prohibiting the coverage of H2O/H species. This work not only highlights the critical design of atomically isolated and unsaturated sites to dramatically enhance the catalytic NORR activity and selectivity, but also demonstrates the promising prospects of p-block metal elements in the design of high-efficiency NORR electrocatalysts.
Conflicts of interest
There are no conflicts of interest to declare.
Acknowledgements
This work was supported by the Longyuan Youth Innovative and Entrepreneurial Talents Project ([2021]17).
References
- J. Liang, Q. Liu, A. A. Alshehri and X. Sun, Recent advances in nanostructured heterogeneous catalysts for N-cycle electrocatalysis, Nano Res. Energy, 2022, 1, e9120010 CrossRef
.
- G. Wang, P. Shen, Y. Luo, X. Li, X. Li and K. Chu, A vacancy engineered MnO2-x electrocatalyst promotes electroreduction of nitrate to ammonia, Dalton Trans., 2022, 51, 9206–9212 RSC
.
- X. Zhao, G. Hu, G. F. Chen, H. Zhang, S. Zhang and H. Wang, Comprehensive understanding of the thriving ambient electrochemical nitrogen reduction reaction, Adv. Mater., 2021, 33, 2007650 CrossRef CAS PubMed
.
- Y. Luo, P. Shen, X. Li, Y. Guo and K. Chu, Sulfur-deficient Bi2S3−x synergistically coupling Ti3C2Tx-MXene for boosting electrocatalytic N2 reduction, Nano Res., 2022, 15, 3991–3999 CrossRef CAS
.
- Y. Luo, Q. Li, Y. Tian, Y. Liu and K. Chu, Amorphization engineered VSe2−x nanosheets with abundant Se-vacancies for enhanced N2 electroreduction, J. Mater. Chem. A, 2022, 10, 1742–1749 RSC
.
- K. Chu, Y. Luo, P. Shen, X. Li, Q. Li and Y. Guo, Unveiling the synergy of O-vacancy and heterostructure over MoO3-x/MXene for N2 electroreduction to NH3, Adv. Energy Mater., 2022, 12, 2103022 CrossRef CAS
.
- Y. Cheng, X. Li, P. Shen, Y. Guo and K. Chu, MXene quantum dots/copper heterostructure for synergistically enhanced N2 electroreduction, Energy Environ. Mater., 2023, 6, e12268 CAS
.
- Z. Yan, M. Ji, J. Xia and H. Zhu, Recent advanced materials for electrochemical and photoelectrochemical synthesis of ammonia from dinitrogen: One step closer to a sustainable energy future, Adv. Energy Mater., 2020, 10, 1902020 CrossRef CAS
.
- K. Tanifuji and Y. Ohki, Metal–sulfur compounds in N2 reduction and nitrogenase-related chemistry, Chem. Rev., 2020, 120, 5194–5251 CrossRef CAS PubMed
.
- G. Qing, R. Ghazfar, S. T. Jackowski, F. Habibzadeh, M. M. Ashtiani, C.-P. Chen, M. R. Smith and T. W. Hamann, Recent advances and challenges of electrocatalytic N2 reduction to ammonia, Chem. Rev., 2020, 120, 5437–5516 CrossRef CAS PubMed
.
- B. H. Ko, B. Hasa, H. Shin, Y. Zhao and F. Jiao, Electrochemical reduction of gaseous nitrogen oxides on transition metals at ambient conditions, J. Am. Chem. Soc., 2022, 144, 1258–1266 CrossRef CAS PubMed
.
- J. Long, S. Chen, Y. Zhang, C. Guo, X. Fu, D. Deng and J. Xiao, Direct electrochemical ammonia synthesis from nitric oxide, Angew. Chem., Int. Ed., 2020, 59, 9711–9718 CrossRef CAS PubMed
.
- J. Choi, H.-L. Du, C. K. Nguyen, B. H. R. Suryanto, A. N. Simonov and D. R. MacFarlane, Electroreduction of nitrates, nitrites, and gaseous nitrogen oxides: A potential source of ammonia in dinitrogen reduction studies, ACS Energy Lett., 2020, 5, 2095–2097 CrossRef CAS
.
- Y. Xiong, Y. Li, S. Wan, Y. Yu, S. Zhang and Q. Zhong, Ferrous-based electrolyte for simultaneous NO absorption and electroreduction to NH3 using Au/rGO electrode, J. Hazard. Mater., 2022, 430, 128451 CrossRef CAS PubMed
.
- W. Zhang, X. Qin, T. Wei, Q. Liu, J. Luo and X. Liu, Single atomic cerium sites anchored on nitrogen-doped hollow carbon spheres for highly selective electroreduction of nitric oxide to ammonia, J. Colloid Interface Sci., 2023, 638, 650–657 CrossRef CAS PubMed
.
- J. Liang, P. Liu, Q. Li, T. Li, L. Yue, Y. Luo, Q. Liu, N. Li, B. Tang, A. A. Alshehri, I. Shakir, P. O. Agboola, C. Sun and X. Sun, Amorphous boron carbide on titanium dioxide nanobelt arrays for high-efficiency electrocatalytic NO reduction to NH3, Angew. Chem., Int. Ed., 2022, 61, e202202087 CAS
.
- Q. Wu, H. Wang, S. Shen, B. Huang, Y. Dai and Y. Ma, Efficient nitric oxide reduction to ammonia on a metal-free electrocatalyst, J. Mater. Chem. A, 2021, 9, 5434–5441 RSC
.
- J. Long, C. Guo, X. Fu, H. Jing, G. Qin, H. Li and J. Xiao, Unveiling potential dependence in NO electroreduction to ammonia, J. Phys. Chem. Lett., 2021, 12, 6988–6995 CrossRef CAS PubMed
.
- S. Cheon, W. J. Kim, D. Y. Kim, Y. Kwon and J.-I. Han, Electro-synthesis of ammonia from dilute nitric oxide on a gas diffusion electrode, ACS Energy Lett., 2022, 7, 958–965 CrossRef CAS
.
- Y. Xiao and C. Shen, Transition-metal borides (MBenes) as new high-efficiency catalysts for nitric oxide electroreduction to ammonia by a high-throughput approach, Small, 2021, 17, 2100776 CrossRef CAS PubMed
.
- T. Wei, H. Bao, X. Wang, S. Zhang, Q. Liu, J. Luo and X. Liu, Ionic liquid-assisted electrocatalytic NO reduction to NH3 by P-doped MoS2, ChemCatChem, 2023, 15, e202201411 CrossRef CAS
.
- D. Qi, F. Lv, T. Wei, M. Jin, G. Meng, S. Zhang, Q. Liu, W. Liu, D. Ma, M. S. Hamdy, J. Luo and X. Liu, High-efficiency electrocatalytic NO reduction to NH3 by nanoporous VN, Nano Res. Energy, 2022, 1, e9120022 CrossRef
.
- J. Liang, Q. Zhou, T. Mou, H. Chen, L. Yue, Y. Luo, Q. Liu, M. S. Hamdy, A. A. Alshehri, F. Gong and X. Sun, FeP nanorod array: A high-efficiency catalyst for electroreduction of NO to NH3 under ambient conditions, Nano Res., 2022, 15, 4008–4013 CrossRef CAS
.
- K. Chen, G. Zhang, X. Li, X. Zhao and K. Chu, Electrochemical NO reduction to NH3 on Cu single atom catalyst, Nano Res., 2023 DOI:10.1007/s12274-023-5384-9
.
- K. Chen, J. Wang, H. Zhang, D. Ma and K. Chu, Self-tandem electrocatalytic NO reduction to NH3 on a W single-atom catalyst, Nano Lett., 2023, 23, 1735–1742 CrossRef CAS PubMed
.
- K. Chen, Y. Tian, Y. Li, Y.-P. Liu and K. Chu, Amorphous NiB2 for electroreduction of NO to NH3, J. Mater. Chem. A, 2023, 11, 7409–7414 RSC
.
- K. Chen, P. Shen, N. Zhang, D. Ma and K. Chu, Electrocatalytic NO reduction to NH3 on Mo2C nanosheets, Inorg. Chem., 2023, 62, 653–658 CrossRef CAS PubMed
.
- Y. Sun, Y. Wang, H. Li, W. Zhang, X.-M. Song, D.-M. Feng, X. Sun, B. Jia, H. Mao and T. Ma, Main group metal elements for ambient-condition electrochemical nitrogen reduction, J. Energy Chem., 2021, 62, 51–70 CrossRef CAS
.
- L. Li, C. Tang, H. Jin, K. Davey and S.-Z. Qiao, Main-group elements boost electrochemical nitrogen fixation, Chem, 2021, 7, 3232–3255 CAS
.
- C. Lv, J. Liu, C. Lee, Q. Zhu, J. Xu, H. Pan, C. Xue and Q. Yan, Emerging p-block-element-based electrocatalysts for sustainable nitrogen conversion, ACS Nano, 2022, 16, 15512–15527 CrossRef CAS PubMed
.
- X. Li, G. Zhang, P. Shen, X. Zhao and K. Chu, A defect engineered p-block SnS2−x catalyst for efficient electrocatalytic NO reduction to NH3, Inorg. Chem. Front., 2023, 10, 280–287 RSC
.
- K. Chen, Y. Zhang, J. Xiang, X. Zhao, X. Li and K. Chu, p-block antimony single-atom catalysts for nitric oxide electroreduction to ammonia, ACS Energy Lett., 2023, 8, 1281–1288 CrossRef CAS
.
- K. Chen, G. Wang, Y. Guo, D. Ma and K. Chu, Iridium single-atom catalyst for highly efficient NO electroreduction to NH3, Nano Res., 2023 DOI:10.1007/s12274-023-5556-7
.
- K. Chen, N. Zhang, F. Wang, J. Kang and K. Chu, Main-group indium single-atom catalysts for efficient electrocatalytic NO reduction to NH3, J. Mater. Chem. A, 2023, 11, 6814–6819 RSC
.
- X. Liu, H. Jang, P. Li, J. Wang, Q. Qin, M. G. Kim, G. Li and J. Cho, Antimony-based composites loaded on phosphorus-doped carbon for boosting Faradaic efficiency of the electrochemical nitrogen reduction reaction, Angew. Chem., Int. Ed., 2019, 58, 13329–13334 CrossRef CAS PubMed
.
- S. K. Kaiser, Z. Chen, D. Faust Akl, S. Mitchell and J. Pérez-Ramírez, Single-atom catalysts across the periodic table, Chem. Rev., 2020, 120, 11703–11809 CrossRef CAS PubMed
.
- C. Gao, J. Low, R. Long, T. Kong, J. Zhu and Y. Xiong, Heterogeneous single-atom photocatalysts: fundamentals and applications, Chem. Rev., 2020, 120, 12175–12216 CrossRef CAS PubMed
.
- L. Zhang, M. Zhou, A. Wang and T. Zhang, Selective hydrogenation over supported metal catalysts: from nanoparticles to single atoms, Chem. Rev., 2019, 120, 683–733 CrossRef PubMed
.
- Q. Li, Y. Guo, Y. Tian, W. Liu and K. Chu, Activating VS2 basal planes for enhanced NRR electrocatalysis: the synergistic role of S-vacancies and B dopants, J. Mater. Chem. A, 2020, 8, 16195–16202 RSC
.
- K. Chu, J. Wang, Y. Liu, Q. Li and Y. Guo, Mo-doped SnS2 with rich S-vacancies for highly efficient electrocatalytic N2 reduction: the critical role of Mo-Sn-Sn trimer, J. Mater. Chem. A, 2020, 8, 7117–7124 RSC
.
- H. Du, H. Guo, K. Wang, X. Du, B. A. Beshiwork, S. Sun, Y. Luo, Q. Liu, T. Li and X. Sun, Durable electrocatalytic reduction of nitrate to ammonia over defective pseudobrookite Fe2TiO5 nanofibers with abundant oxygen vacancies, Angew. Chem., Int. Ed., 2023, 135, e202215782 Search PubMed
.
- A. Maiti and S. K. Srivastava, N, Ru codoped pellet drum bundle-like Sb2S3: An efficient hydrogen evolution reaction and hydrogen oxidation reaction electrocatalyst in alkaline medium, ACS Appl. Mater. Interfaces, 2020, 12, 7057–7070 CrossRef CAS PubMed
.
- X. Chen, X. Li, P. Wei, X. Ma, Q. Yu and L. Liu, Selective synthesis of Sb2S3 nanostructures with different morphologies for high performance in dye-sensitized solar cells, Chin. J. Catal., 2020, 41, 435–441 CrossRef CAS
.
- S. Yao, J. Cui, Y. Deng, W. G. Chong, J. Wu, M. Ihsan-Ul-Haq, Y.-W. Mai and J.-K. Kim, Ultrathin Sb2S3 nanosheet anodes for exceptional pseudocapacitive contribution to multi-battery charge storage, Energy Storage Mater., 2019, 20, 36–45 CrossRef
.
- Y. S. Park, X. Jin, J. Tan, H. Lee, J. Yun, S. Ma, G. Jang, T. Kim, S. G. Shim and K. Kim, High-performance Sb2S3 photoanode enabling iodide oxidation reaction for unbiased photoelectrochemical solar fuel production, Energy Environ. Sci., 2022, 15, 4725–4737 RSC
.
- K. Chen, J. Wang, J. Kang, X. Lu, X. Zhao and K. Chu, Atomically Fe-doped MoS2−x with Fe-Mo dual sites for efficient electrocatalytic NO reduction to NH3, Appl. Catal., B, 2023, 324, 122241 CrossRef CAS
.
- L. Zhang, J. Zhang, A. Xu, Z. Lin, Z. Wang, W. Zhong, S. Shen and G. Wu, Charge redistribution of Co9S8/MoS2 heterojunction microsphere enhances electrocatalytic hydrogen evolution, Biomimetics, 2023, 8, 104 CrossRef CAS PubMed
.
- L. Zhang, Z. Wang, J. Zhang, Z. Lin, Q. Zhang, W. Zhong and G. Wu, High activity and stability in Ni2P/(Co,Ni)OOH heterointerface with a multiple-hierarchy structure for alkaline hydrogen evolution reaction, Nano Res., 2023 DOI:10.1007/s12274-022-5322-2
.
- X. Wang, J. Zhang, Z. Wang, Z. Lin, S. Shen and W. Zhong, Fabricating Ru single atoms and clusters on CoP for boosted hydrogen evolution reaction, Chin. J. Struct. Chem., 2023 DOI:10.1016/j.cjsc.2023.100035
.
- Y. Huang, Z. Hu, L.-a. Huang, Z. Wang, Z. Lin, S. Shen, W. Zhong and J. Pan, Phosphorus-modified cobalt single-atom catalysts loaded on crosslinked carbon nanosheets for efficient alkaline hydrogen evolution reaction, Nanoscale, 2023, 15, 3550–3559 RSC
.
- N. Zhang, G. Zhang, P. Shen, H. Zhang, D. Ma and K. Chu, Lewis acid Fe–V pairs promote nitrate electroreduction to ammonia, Adv. Funct. Mater., 2023, 33, 2211537 CrossRef CAS
.
- G. Wang, P. Shen, K. Chen, Y.-L. Guo, X. Zhao and K. Chu, Rare-earth La-doped VS2−x for electrochemical nitrate reduction to ammonia, Inorg. Chem. Front., 2023, 10, 2014–2021 RSC
.
- K. Chen, Z. Ma, X. Li, J. Kang, D. Ma and K. Chu, Single-atom Bi alloyed Pd metallene for nitrate electroreduction to ammonia, Adv. Funct. Mater., 2023, 33, 2209890 CrossRef CAS
.
- W. Zhang, M. Jiang, S. Yang, Y. Hu, B. Mu, Z. Tie and Z. Jin,
In situ grown CuOx nanowire forest on copper foam: A 3D hierarchical and freestanding electrocatalyst with enhanced carbonaceous product selectivity in CO2 reduction, Nano Res. Energy, 2022, 1, e9120033 CrossRef
.
- N. Zhang, G. Zhang, Y. Tian, Y. Guo and K. Chu, Boron phosphide as an efficient metal-free catalyst for nitrate electroreduction to ammonia, Nano Res. Energy, 2023, 52, 4290–4295 CAS
.
- F. Guo, M. Zhang, S. Yi, X. Li, R. Xin, M. Yang, B. Liu, H. Chen, H. Li and Y. Liu, Metal-coordinated porous polydopamine nanospheres derived Fe3N–FeCo encapsulated N-doped carbon as a highly efficient electrocatalyst for oxygen reduction reaction, Nano Res. Energy, 2022, 1, e9120027 CrossRef
.
- Y. Cheng, P. Shen, X. Li, X. Li, K. Chu and Y. Guo, Synergistically enhanced peroxidase-like activity of Fe3O4/Ti3C2 MXene quantum dots and its application in colorimetric determination of Cr(VI), Sens. Actuators, B, 2023, 376, 132979 CrossRef CAS
.
- X. Li, K. Chen, X. Lu, D. Ma and K. Chu, Atomically dispersed Co catalyst for electrocatalytic NO reduction to NH3, Chem. Eng. J., 2023, 454, 140333 CrossRef CAS
.
- P. Shen, X. Li, Y. Luo, Y. Guo, X. Zhao and K. Chu, High-efficiency N2 electroreduction enabled by Se-vacancy-rich WSe2−x in water-in-salt electrolytes, ACS Nano, 2022, 16, 7915–7925 CrossRef CAS PubMed
.
- G. Zhang, X. Li, K. Chen, Y. Guo, D. Ma and K. Chu, Tandem electrocatalytic nitrate reduction to ammonia on MBenes, Angew. Chem., Int. Ed., 2023, 62, e202300054 CAS
.
- Y. Luo, K. Chen, G. Wang, G. Zhang, N. Zhang and K. Chu, Ce-doped MoS2−x nanoflower arrays for electrocatalytic nitrate reduction to ammonia, Inorg. Chem. Front., 2023, 10, 1543–1551 RSC
.
- X. Li, P. Shen, X. Li, D. Ma and K. Chu, Sub-nm RuOx clusters on Pd metallene for synergistically enhanced nitrate electroreduction to ammonia, ACS Nano, 2023, 17, 1081–1090 CAS
.
|
This journal is © the Partner Organisations 2023 |
Click here to see how this site uses Cookies. View our privacy policy here.