DOI:
10.1039/D1MA01199E
(Review Article)
Mater. Adv., 2022,
3, 7445-7462
MXenes: promising 2D materials for wound dressing applications – a perspective review
Received
18th December 2021
, Accepted 24th July 2022
First published on 12th September 2022
Abstract
Two-dimensional (2D) materials have been studied extensively for the past 15 years, sparking a new wave of research on well-known 2D materials. Due to their specific configuration and noteworthy physiochemical features, intensive, multifaceted research attempts have been focused on the medical and clinical applications of 2D materials. In this context, 2D MXenes, a new class of ultra-thin atomic nanosheet materials produced from MAX phase ceramics, are gaining popularity as inorganic nanosystems, especially for biomedical applications. The 2D MXenes can meet the stringent biomedical standards due to their high conductivity, hydrophilicity, and other interesting physicochemical properties. Based on these characteristics, 2D MXenes have been used in wound dressing management and there are many studies on the development of nanofibers and nanosheets. Herein, we present an overview of MXenes, and their synthesis using various processes and properties. The review further focuses on the mechanism and importance of MXenes for wound dressing applications. Additionally, we summarize the toxicity and bio-safety issues of MXene-based materials. In the last section, we present the conclusions, challenges and future outlook.
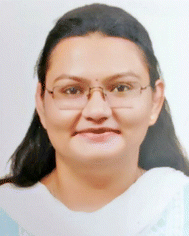
Vaishnavi Hada
| Ms Vaishnavi Hada is a Project Associate at the Council of Scientific and Industrial Research–Advanced Materials and Process Research Institute (CSIR–AMPRI). She is a postgraduate and her research is focused on developing smart and functional biomaterials such as shape-memory polymer composites for sutures and wound healing applications, nano-biomaterials, advanced radiation shielding materials, biomass utilization, and antimicrobial materials useful for versatile applications. |
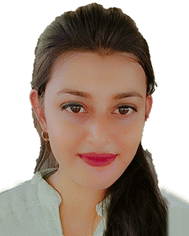
Deeksha Malvi
| Ms Deeksha Malvi is a post-graduate at the Council of Scientific and Industrial Research–Advanced Materials and Process Research Institute (CSIR–AMPRI). Her area of interest is biochemistry research. She mainly works on developing advanced materials via various chemical processes for a broad application. She is working on chitosan and lignin-based antimicrobial gels for wound healing applications, and is also interested in the scaling up of methods using alternative approaches. |
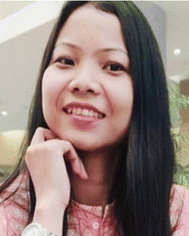
Medha Mili
| Mrs Medha Mili is working as a Scientist at the Council of Scientific And Industrial Research–Advanced Materials and Process Research Institute (CSIR–AMPRI). Her research areas mainly include polymer composites/nanocomposites, polymer processing, advanced bamboo-based polymeric composites for structural applications, advanced lignocellulosic materials based composites, and shape-memory polymer composites. |
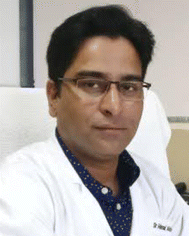
Manal M Khan
| Dr Manal Mohd Khan is presently an Additional Professor and Head, the Department of Burns and Plastic Surgery, All India Institute of Medical Sciences, Bhopal, Madhya Pradesh. He did his MS (Surgery) and MCh (Plastic & Reconstructive Surgery) at the prestigious J. N. M. C., Aligarh Muslim University, and Fellowship in Aesthetic & Laser Surgery. His clinical and research interests include microvascular surgery, onco-reconstruction, brachial plexus injury surgery, cranio-maxillo-facial surgery, and management of various burns and post-traumatic wounds. He has published multiple papers in national and international journals and contributed various book chapters. |
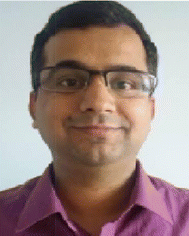
Gaurav Chaturvedi
| Dr Gaurav Chaturvedi is an Associate Professor in the Dept. of Burns & Plastic Surgery at All India Institute of Medical Sciences, Bhopal, Madhya Pradesh. He brings his clinical experience to his role at AIIMS, Bhopal, after training for over six years at Christian Medical College, Vellore, one of the premier Institutes in India. His clinical and research interest includes managing patients with brachial plexus injury, onco-reconstruction, and managing wounds and their coverage, especially in burns and trauma patients, with the help of microvascular surgery. He has published multiple research papers in the International and National Journal on Wounds Management. |
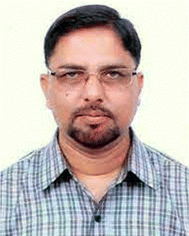
SAR Hashmi
| Dr SAR Hashmi is the Chief Scientist at the Council of Scientific and Industrial Research–Advanced Materials and Process Research Institute (CSIR–AMPRI). He specializes in the development of polymer composites such as bamboo-based polymeric composites and shape-memory polymers. He also works on the processing and rheology of polymers, blends and composites, design and development of components, products and experiments. |
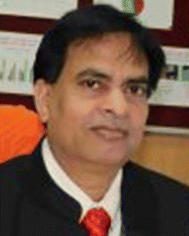
AK Srivastava
| Dr A. K. Srivastava is the Director of the Council of Scientific and Industrial Research–Advanced Materials and Processes Research Institute (CSIR–AMPRI). His prolific research applied to understanding the nucleation-growth mechanisms, phase transformations, microstructures and defects of various materials in the form of bulk and fascinating nanoscale is outstanding and known by peers worldwide. He has contributed significantly to multiple projects in the area of advanced materials about (i) biomaterials, (ii) rapidly solidified metallic systems, (iii) nanostructures, (iv) polymer composites, (v) solar energy, (vi) thermoelectrics, (viii) magnetic, (viiii) waste to wealth, and (ix) safety, health & environment. |
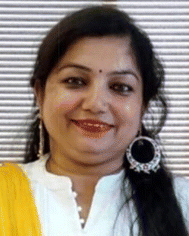
Sarika Verma
| Dr Sarika Verma is currently working as the Principal Scientist at Council Of Scientific and Industrial Research-Advanced Materials and Processes Research Institute (CSIR-AMPRI). Her areas of interdisciplinary research primarily include the design and development of advanced materials including materials for health care applications, radiation shielding materials, biomedical and energy materials, and polymeric composites such as bamboo-based polymeric composites and shape-memory polymers via novel techniques useful for a wide range of applications. She is also the recipient of various awards and fellowships. |
1. Introduction
Over the last few years, incredible potential and many intriguing opportunities have resulted in the development of commercial adaptable nano-materials for disease and patient-specific treatment.1,2 Among the various nanostructures, two-dimensional (2D) nanomaterials have become a research focus in the world of nanoscience. 2D nanomaterials have shown potential for wide application ranging from well-developed power storage to burgeoning medicinal chemistry. In the case of 2D materials, MXenes are emerging materials, which were discovered in 2011 by etching an MAX phase by chemical treatment.3 MXenes have the general formula of Mn+1XnTx, where M represents an early transition metal such as Ti. Meanwhile, X represents nitrogen and/or carbon, T represents a surface functional group such as O, OH, and F, x represents the number of functional groups, and n stands for a positive integer between 1 and 4.4 MXenes are a family of 2D crystalline solids with a substantial available diameter range. Further, their nanoscale density is distinguished by their endpoints or surface elements, which give them well-defined attributes.5 Owing to this, MXene research has progressed, with scientists attempting to comprehend their features and uses.6
Due to their unique tensile characteristics, physio-chemical characteristics (e.g., photonic, catalytic, magnetic, and electronic properties) and numerous other exciting features, MXenes have attracted significant interest from researcher.7 Because of the partially occupied d-shells of their transition metal atoms and their peculiar coordination with neighbouring atoms, most MXenes are metallic.8 Also, it should be highlighted that the surface modification of MXenes can enhance their electronic attributes. Moreover, because most MXenes are metallic, their electronic properties are primarily influenced by their M atoms. Surface modification can also have an impact on the magnetization of Mxenes.9 This can result in a surface-functionalized MXene with weaker magnetization, which is caused by the transition metal element that has unpaired surface electrons.10 Furthermore, magnetic MXenes with half-metallic functionalities have been studied. One spin channel is semi-conducting, while the other is metallic, giving rise to completely spin-polarized electrons at the Fermi level.11 Besides, the surface terminations of MXenes play a significant role in their mechanical characteristics. The proportion of atomic layers of MXenes, depending on their chemical formula, is another variable that affects their mechanical characteristics. MXene–polymer composites have been shown to have significantly greater compression strength and tensile properties, durability, and functionality than pure MXenes.12 It has been reported that the optical properties of MXenes, such as their refractive index, reflectivity, absorption, and transmittance, are essential in various applications. Some investigations on the effect of the surface functionalization of MXenes on their optical characteristics have been reported.13 Moreover, the visible and UV light absorption of MXenes is the keystone for their optoelectronic, photo-voltaic and photocatalytic properties, which are influenced by the presence of functional groups.14
In the recent clinical advancement, bioengineering and nanobiotechnology have sparked the development of various innovative synthetic nanomaterials. These innovative nanomaterials give alternative theranostic (therapeutic and diagnostic) methods as possible solutions to counter various diseases through synergistic treatment and multimodal imaging, particularly in cancer therapies.15–25 Their multiple features, such as porous structure and fascinating physio-chemical natures, enable them to meet the stringent requirements of theranostic nanomedicine, including drug administration, laser treatment, radiology, biosensors, and even wound healing.19,26–33 Bio-sensing, antimicrobial materials, biomedical imaging, photo-thermal therapy nanomedicine, cancer detection and therapy are a few applications besides wound dressing.34
A wound is an interruption in the cohesiveness of the epithelial lining of the skin or mucosa caused by physical or thermal impairment.35 Wound curing is a dynamic and complicated tissue proliferation and growth procedure, which occurs in four stages, as follows: (i) agglomeration and haemostasis process (instantly after damage); (ii) inflammation process (soon after tissue damage), after which swelling occurs; (iii) proliferation process, in which new skin cells and vasculature are developed; and (iv) maturation process, in which new skin cells are remodelled.36 In the 4 healthcare sectors, MXene-based materials have become the most promising and effective advanced materials in wound dressing applications. Wound dressings containing microbicidal compounds have emerged as excellent solutions for reducing wound infection, thus+ increasing the speed of the recovery process.37 MXene-based materials have demonstrated strong bactericidal and wound-healing potential, paving the way for the development of new wound healing dressings and antimicrobial techniques.38
The present review highlights the synthesis of MXene-based materials using various processes and their properties. Further, we focus on the mechanism and importance of MXenes for wound dressing applications. Additionally, we summarize the toxicity and bio-safety issues associated with MXene-based materials. In the last section, we present the conclusions, challenges and future outlook.
2. Synthesis and general properties of MXene-based materials
2.1 Synthesis of MXenes
MXene is the name given to a group of transition metal nitrides, carbides and carbonitrides assembled by molecular deformation of a MAX phase, which is 3D ternary (or quaternary) composition.39 The MAX phase is an MXene precursor with the stoichiometric ratio of Mn+1AXn, where “M” is a d-block transition metal, “A” is a group 13 or 14 component (e.g., Si, Sn, Ge and Al), and “X” is nitrogen, carbon, or both.40 The layers “M” and “A” are agglomerated in these portions, which have a hexagonal shape (space group P63/mmc). The “X” atoms occupy the octahedral positions created by the “M” atoms.41–45Fig. 1 illustrates the general elemental composition of MXenes.
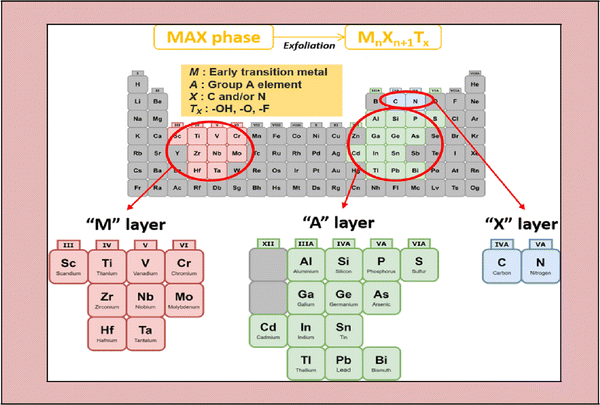 |
| Fig. 1 Composition of the general elements of MXenes and MAX phases, where M is an early transition metal, A is a group A element, X is N and/or C, and Tx is a surface functional group (adapted from ref. 46 under Creative Commons Attribution 4.0). | |
The bond stress of M–X and M–A is the fundamental MAX phase difference. Alternatively, M–A is weakly bonded and more fragile than the M–X bonds.47–49 The central concept for the synthesis of MXenes is removing the weakly linked A atoms layers to create carbides, nitrides, or both,50 which is the critical stage in their synthesis.51 This series of 2D metal nitrides and carbides created is termed MXenes due to the deletion of the A atom layer from the MAX phase.52 Because the layer-to-layer bonding in the MAX phase is substantially weaker than the intra-layer bonding, wet chemical etching of the atomic layers in a multilayered MAX phase is the most popular method for the preparation of MXenes. The MAX phase is initially immersed in acid, which dissolves the bonding between the transition metal and the A element.53
After synthesizing the MAX phase, the primary step is to etch the 3D MAX phase, such as Ti3AlC2, with a strong etchant, commonly hydrofluoride (HF).54
In comparison to M–X bonds, M–A bonds are more fragile, and hence the specific etching of the M–A bonds can be achievable.55 Given that F ions bond tightly to the A-element, etching the A-element from the typical MAX phase demands a higher accumulation of fluoride ions (F) (or Al).55 Furthermore, HF processing of the MAX phase produces surface terminations with –O, –F, and –OH molecules, as represented in Fig. 2.56
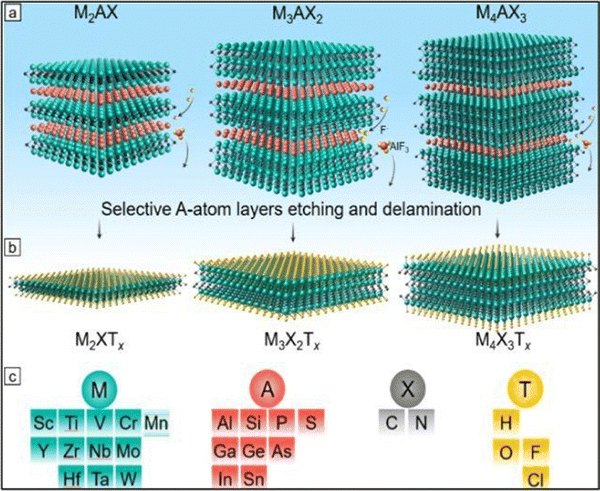 |
| Fig. 2 Precursors and synthesis of MXenes. (a) Three types of mono-M MAX phases: M2AX, M3AX2, and M4AX3 and the selective etching process of the group A layers (red atoms). (b) Formation of MXene after selective etching and the formation of surface terminations (yellow atoms) labeled as T. (c) Feasible elements for M, A, X, and T in MAX and MXene phases (adapted with permission from ref. 57 Copyright©2021). | |
The first etchant employed was hydrofluoric acid (HF), which is highly hazardous to the environment.58–60 This problem was solved using various processes in which the use of HF is not required. Consequently, the necessity for multiple etchants emerged. In 2014, a better combination of HCl (hydrochloric acid) and LiF (lithium fluoride) was introduced.61 The HF acid etching approach boosted the hydrophilic functional groups such as F, OH and O on the surface of MXenes, which define their augmented hydrophilic properties, resulting in enhanced biocompatibility.62
(i) Chemical vapour deposition.
Taking methane (CH4) as the source of carbon (C) and copper (Cu) foil above molybdenum (Mo) foil as the substrate, Xu et al. established a CVD (chemical vapour deposition) technique to create MXenes in 201563 at a temperature of more than 1085 °C. The breakdown of chemicals on the plane of the substrate throughout the CVD process resulted in the formation of material layers from the vapour phase. The Cu foil melted because of the elevated temperature, formed an Mo–Cu alloy. Upon interacting with the carbon atoms created via the combustion of methane, the Mo-atoms diffused into the surface of the Cu liquid, generating Mo2C-crystals. Despite its MXene-like structure, the created substance was a 2D transition metal carbide with a more extensive size than the previously developed nanosheets of up to 10 mm. Researchers were able to alter the diameter by adjusting the methane content. Once the MXene-like substance was exposed to air for several months, it was devoid of flaws, and its super-conductivity remained unchanged.
(ii) Urea glass route.
Using urea glass, Ma et al. (2015)64 produced Mo2C and Mo2N MXenes from the metal precursor MoCl5. They introduced ethanol to the precursor, which reacted, resulting in the formation of Mo-orthoesters. Subsequently, solid urea was added to the mixture and agitated until it was completely dissolved. After that, the gel-like product was calcined at 800 °C in an N2 gas atmosphere. They obtained silvery black particles and performed XRD (X-ray diffraction) analysis on the samples and found no other crystalline side phases, such as MoOx, indicating that the powder was pure.
(iii) Hydrothermal synthesis in an aqueous solution of NaOH.
In 2018, Li et al.65 published a method for the preparation of Ti3C2TxMXene from the Ti3AlC2 MAX phase using NaOH (sodium hydroxide). In this method, the (OH–OH–) hydroxide anions damaged the aluminium (Al) layers, causing the Al-atoms to be oxidized. The generated aluminium hydroxides, i.e., Al(OH)–4Al(OH)4 were subsequently dispersed in the alkali, with the exposed Ti (titanium) atoms being exchanged with O or OH. This procedure allows new aluminium hydroxides in the matrix of Ti layers, and therefore they react with OH–OH– again. The MXenes acquired by this hydrothermal process had more O and OH terminations than that obtained by HF etching, significantly improving their supercapacitor efficiency. Fig. 3 represents the formation of MXenes via hydrothermal synthesis using Ti3AlC2 (MAX phase) and NaOH solution.
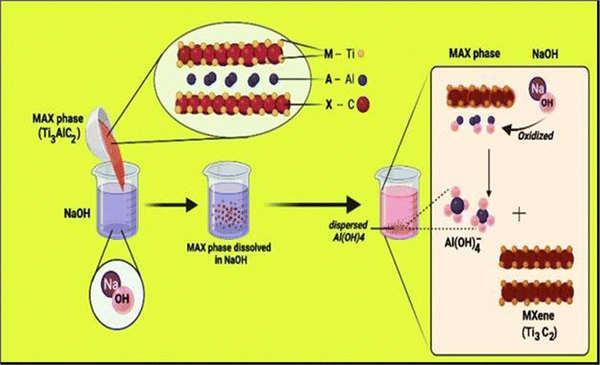 |
| Fig. 3 Formation of MXene via hydrothermal synthesis using Ti3AlC2 (MAX phase) and NaOH solution. | |
(iv) Electrical synthesis at room temperature.
Yang et al. (2018)66 reported the first electrochemical technique for debonding Ti3C2 in a dual electrolyte solution. Bulk Ti3AlC2 was used as the anode and cathode in a two-electrode arrangement; however, only the anode was etched, resulting in Ti3C2Tx.67 They employed a pH > 9 mixture of 1 M NH4Cl and 0.2 M tetramethylammonium hydroxide (TMAOH) to permit the electrolyte atoms to permeate a certain depth in the anode. The core anode was progressively deformed using a low voltage of +5 V. To make individual sheets of Ti3C2Tx, the residue of Ti3C2Tx was crushed and put into 25% w/w TMAOH. The electrical conductivity of the generated MXenes was comparable to that synthesized using HCl or HF/LiF. However, the electrochemical approach is more attractive given that in the overall etching productivity, almost 60% of the material mass can be converted into Ti3C2Tx.61
(v) Molten salt etching.
The approach of etching molten salt was first employed on MXenes in 2016. Urbankowski et al.68 heated Ti4AlN3 powder in a 1
:
1 mass ratio with fluoride salt for about 550 °C for 30 min. They discovered five distinct fluoride phases comprising aluminium, but no Ti-comprising fluorides, proving the etching sensitivity. They utilized dilute sulfuric acid (H2SO4) to disseminate the Al-containing fluorides, and the etching residues were eliminated by rinsing with deionized water, centrifugation, and decanting. The powder was further combined with tetrabutylammonium hydroxide to break down the layered Ti4N3Tx MXene (TBAOH). The small unlayered Ti4N3Tx flakes (T = OH, FT = OH, and F) were recovered after processing. Table 1 describes the different etching methods used to obtain MXenes.69 Some of the etchants include HF, HCl + LiF, NH4HF2 NH4Cl/TMAOH, LiF + NaF + KF, ZnCl2, NaBF4, HCl, and NaOH.
Table 1 Various methods employing etchants to obtain MXenes
S. no. |
Method |
Etchants |
Temperature |
Ref. |
1 |
— |
NaBF4, HCl |
180 °C |
6
|
2 |
Acid with and without fluorine |
HF |
Room temp. 55 °C |
54
|
3 |
— |
HCl + LiF |
35–55 °C |
61
|
4 |
Hydrothermal |
NaOH |
270 °C |
65
|
5 |
Electrochemical |
NH4Cl/TMAOH |
Room temp. |
66
|
6 |
— |
HCl |
Room temp. |
67
|
7 |
Molten salts |
LiF + NaF + KF |
550 °C |
68
|
8 |
— |
NH4HF2 |
Room temp. |
70
|
9 |
Lewis acid |
ZnCl2 |
550 °C |
71
|
2.1 General properties of MXenes
MXenes possess valuable essential characteristics (as shown in Fig. 4), including structural, electrical, optical, and biological properties.72 These characteristics make them suitable for extensive applications, with the latest being in healthcare. High specific surface area, availability of hydrophilic groups, high molecular weights (for certain transition metals), and magnetization properties are all hallmarks of MXenes.73–75 The latest innovations in biology and electronics using MXenes have realized a new era of technology in the field of bio-electronics for healthcare assistance and theranostics, medical observation, and wearable devices.76–78 Bioelectronic devices are invented for the safest association with human body tissues, offering a prototype-changing chance for monitoring brain activity, cardiac health, and muscle function. They can also assist human–machine interactions, such as myo-electric control of advanced prostheses79,80 and brain–computer interfaces (BCIs).81 Active bio-electronic components can also be applied by the smart approach depending on the electrical stimulation of excitable circuits for treating neurological diseases,82,83 heart arrhythmias84 and inflammatory disorders85,86 and used for rehabilitation therapies. The functional groups of MXenes also enhance their rigidity and elasticity, which are applicable for thin-film production in bio-electronic equipment.87
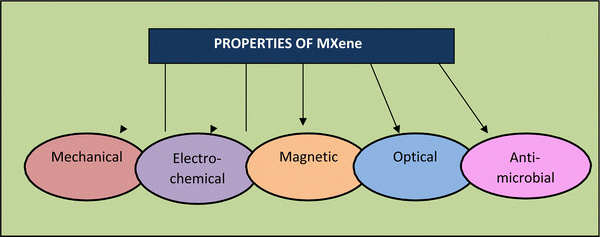 |
| Fig. 4 Properties of MXenes. | |
(i) Mechanical properties.
The mechanical characteristics of MXenes can vary significantly, depending on their surface terminations. Bai et al. (2016)88 discovered that the Ti atoms and O terminations interacted more strongly in Ti2C and Ti3C2 than in OH or F-terminated MXenes. Furthermore, O-terminated MXenes have very high strength and stiffness, signifying that the bond stability of Ti–O is superior compared to Ti–F and Ti–OH.
Magnuson et al. (2018)89 also discovered that the surface groups diminish–C bonds by removing their energy. They found that the Ti–C bond is more robust in Ti2CTx than Ti3C2Tx, which they believe may alter the elasticity of these materials. Scientists also proposed that modifying the binding strength can help improve the flexibility of MXenes. Furthermore, because of their higher structural rigidity, Zha et al. (2016)90 indicated that O-terminated MXenes should be the primary candidates as structural materials, supercapacitors, and other purposes.
In theory, Ti2CO2-based composite materials can withstand considerable strain under uniaxial and deformation tension. Regarding the strain endurance of MXenes, Chakraborty et al. (2017)91 demonstrated the doping of Ti2CO2 with boron (B), where boron replaces the carbon atoms, which could withstand excessive strain (almost 100%) compared to the undoped MXene. This is because of the weak bond between Ti–Bas and Ti–C bond. Furthermore, Yorulmaz et al. (2016)92 discovered that MXenes stiffen as the density of M, i.e., transition metal, increases for carbides, although this finding was not observed for nitrides.
(ii) Electronic properties.
The strong electronic conductivity of MXenes is their most vital electronic feature. The majority of uncoated MXenes and those with surface terminations have metallic characteristics. Although Ti3C2Tx was the first identified, the longest investigated, and the most active MXene, an endeavour has been undertaken to boost the metallic conductivity of MXenes in recent years.93 The focus is producing new Mn+1XnMn+1Xn compounds with regulated surface terminations to increase their resistivity. Unfortunately, there is no scientific confirmation for the research done in this area.94 The formation of cation or organic–molecule complexes is an alternative way to influence the resistivity of MXenes. An almost absolute scale can enhance the susceptibility of devices to layered substances.95,96
Zhang et al. (2018)97 found that OH-terminated MXenes had free electron energies outside the particle surface and parallel to the surface. They form clusters in areas with the most significant positive charge, providing nearly perfect electron transport routes. At the Fermi level, the transition metal has a reduced density of states (DOS) because of the electron transport from a transition metal to the electronegative surface terminations.98,99 No MXene semiconductors have been investigated to date, even though most are anticipated to have a band gap for all surface terminations.100
(iii) Magnetic properties.
The most prevalent MXenes, together with Ti3C2, are non-magnetic; however, various magnetic frameworks have been revealed. For example, Dong et al. (2017)101 reported that Ti2MnC2Tx MXenes in the ground state are ferromagnetic. Ti2MnC2Tx is associated with the new family of double ordered-transition-metal MXenes,102,103 which have a transition metal of one or two layers that joins with the other layers. Sun et al. (2018)104 investigated a central layer consisting of double-transition-metal MXenes with Ti atoms. It has also demonstrated that various terminations and cation arrangements result in magnetic characteristics and attributes distinct from the previously known single transition metal carbides.105,106
Previously, Zhu et al. (2015)107 discovered a conceptual in-plane lattice fixed standard for Nb2C, which agrees with the experimental one, and demonstrated that the thermodynamically favourable place for Li sorbent was situated on the upper end of the MXene. Bandyopadhyay et al.,108 explored the process of node deformation in MXenes in 2018. They discovered that a few deficient MXenes exhibit magnetism due to the delocalized electrons in their rotation-torn d-orbitals. Consequently, they proposed that inherent defects could be used to alter the magnetization of MXenes.
(iv) Optical property.
Various intriguing optical properties of MXenes have been documented in recent years, including plasmonic behaviour, optical transparency, and photothermal conversion efficiency. The potential of MXenes to interact with light in several ways has had a massive effect on the scientific community.109 Specifically, the surface terminations play a significant role in determining their optical properties. Halim et al., 2019110 studied Ti2CTx and Nb2CTx films and demonstrated that changing the transition metal is a novel method of controlling the reflectivity of MXenes. They discovered that the permeability of the Nb-based films increased, as shown by their infrared spectra. These actions varied greatly for the correlating MAX sequence Nb2AlC, which was not detected for the titanium-based films. Halim et al.55 recommended that the discrepancy in optical characteristics was due to the electron pairs of the transition metals (titanium in group 4 of the periodic table, while Nb is in group 5), their bond formation with carbon atoms and surface termination.
Li et al. (2017)65 demonstrated that in comparison with carbon nanotubes (CNTs), MXenes accumulate more light. Furthermore, Jiang et al. (2018)111 investigated the optical nonlinearity of Ti3C2Tx using oscillation sources with different wavelengths (1800 nm, 1550 nm, 1064 nm, and 800 nm). They discovered that the permeability reaction was two orders of magnitude greater than several other mechanisms, but the refractive index of graphene was similar. Finally, for Ti2N, Ti3N2, Ti2C, and Ti3C2, their reflectivity reached 100%, indicating these materials can transmit electromagnetic waves.112
(v) Antimicrobial property.
Before MXenes, nanomaterials based on graphene were used in biomedical applications to produce reactive oxygen species (ROS) and directly interact with the cell wall of microbes.1,113–116 The first Ti3C2MXene nanoparticle solution demanded a significant inhibition dose of 200 g to provide favorable suppression.117,118 Nevertheless, the modification of Ti3C2 with PVDF (polyvinylidene fluoride) significantly improved its antimicrobial activity.117 One study proposed that due to the unique characteristics of MXene nanostructures such as their sharp edges and compact size, they can pierce the bacterial cell membrane, resulting in the release of bacterial DNA and ultimately bacterial dispersion.119 The only report on the anti-fungal properties of MXene was investigated using Ti3C2 MXene against T. reesei (Trichoderma reesei).120 The influence of delaminated MXene (d-Ti3C2) inhibited the development of hyphae in T. reesei, which yielded similar findings to the anti-fungal medicine amphotericin-B. Because of their sharp lamellar periphery, MXene nanosheets may also impede sporulation. Consequently, this study demonstrated that Ti3C2MXene may destabilize the fungal life cycle and indicated the tremendous capacity of MXenes as convincing anti-fungal agents. Because of their significant microbicidal actions, the chemistry of MXenes enables future applications in previously unexplored areas such as coating materials for medical catheters, masks, and gloves.46
3. Why and how are MXene-based materials helpful for wound dressing applications?
Wounds can occur due to severe trauma, diabetes, and burns as a disorder profoundly influencing human health and causing tough challenges in the field of healthcare.121–125 Although several remedies for wound healing, such as drug therapies,126 skin grafting,127 and cellular treatment,128 are used in medical care, they have some restrictions that limit their therapeutic effects. Because of their distinctive attributes, MXenes have intrigued many researchers in the biomedical field,38,123,129,130 such as their excellent electrical performance,1 high photo-thermal conversion efficiency,131,132 and large specific surface area.125 MXenes have fluorescence imaging properties and photo-thermal and antibacterial features, which are helpful in various biomedical applications.133 This is due to the capability of MXene nanosheets to rapidly decompose upon interaction with water and oxygen. Consequently, it is possible to use these distinct photo-thermal characteristics and bio-based effectiveness for wound healing.134 Many researchers have looked into the significance of MXenes in wound dressing applications. Some recent studies highlighted the importance of MXene-based composites for wound dressing applications. MXenes have anti-bacterial, photo-thermal, and luminescent attributes, making them suitable for a wide range of medical appliactions.135,136 MXenes must be developed with various performance parameters without modifying their inherent properties to be used in medical applications. Notably, the influence of water and oxygen can deteriorate MXene nanosheets. Hence, employing these peculiar photo-thermal and bio-degradable activities for wound healing application is viable.137
MXenes have many elements, but titanium carbide (Ti3C2Tx) is mainly used for wound healing. According to the reported studies, titanium shows the greatest potential in the biomedical field given that it is an antimicrobial agent, shows cytotoxicity and has ROS (reactive oxygen species)-dependent characteristics, which ultimately enhance the cell viability.138 ROS is essential in cell metabolic activities and viability and the anti-proliferative pathways of distinct classifications of carbon-based nanomaterials.139 Hence, Ti3C2Tx MXenes are the most promising wound-healing materials given that they offer prospective benefits in the wound healing process through fibroblast proliferation. The increasing speed in wound repair is associated with previous wound contraction and stability of the impaired region reorganization of granulation tissue and collagen fibers.140
Lin Mao et al. (2020)141 proposed that electrical stimulation can be applied to accelerate skin wound healing. However, electrical stimulation (ES) is rarely used to promote effective wound healing by encouraging skin cells behaviour. This study described the first sequence of multipurpose hydrogels made of regenerative Ti3C2Tx and bacterial cellulose (rBC), which can electrically control cell behaviour for effective wound repair with extrinsic ES. An amalgam hydrogel containing MXene of 2 wt% (rBC/MXene2%) had the optimum electrical conductivity, biocompatibility, mechanical characteristics, flexibility, and bio-degradability. According to an in vivo investigation on a rat with a whole thickness damage specimen, this rBC/MXene hydrogel demonstrated superior healing effects compared to the conventional Tegaderm film. Mainly, the in vivo and in vitro findings showed that the hydrogel could remarkably boost NIH3T3 cell multiplication and speed up wound healing compared to the non-ES controls combined with ES. According to this report, the degradable and electro-active rBC/MXene hydrogel is a fascinating option for wound repairing on the skin. It also provides a successful synergistic treatment method for speeding wound healing by combining ES with hydrogel dressings. Fig. 5 represents the fabrication and synthesis of the rBC/MXene hydrogel.141
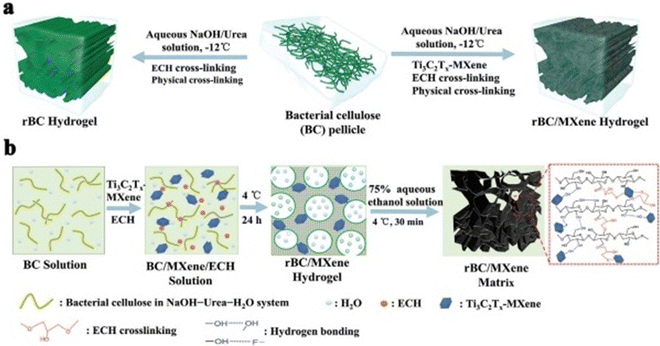 |
| Fig. 5 (a) Schematic outlining the fabrication of rBC-based hydrogels. (b) Diagrammatic representation of the process for developing rBC/MXene composite hydrogels. (Adapted with permission from ref. 141 Copyright©2021.) | |
Similar research was done by Lin Jin et al. (2020),142 wherein they proposed the synthesis of MXene nanobelts that respond to infrared light for wound healing applications. They offered temperature-responsive MXene nanobelt fibres (T-RMFs) with a controlled supply of vitamin E for wound healing applications. MXene nanosheets were dispersed over polyvinyl pyrrolidone and polyacrylonitrile composite nanobelts with a thermosensitive P(AAm-co-ANCO-VIm) (PAAV) coating layer to make these T-RMFs. The T-RMFs possessed outstanding photothermal characteristics because the MXene nanosheets had a high surface area and relatively high density. The temperature could be easily adjusted by NIR (near-infrared irradiation). The thermo-responsive polymer coating layer softened the bonds, allowing vitamin E to disintegrate and be released. The T-RMFs displayed remarkable bio-compatibility and wound healing activities in cellular and animal testing. These T-RMFs show that nanobelts have tremendous potential for wound healing, tissue regeneration, and a broad range of other applications. This is due to the simple technique, constant mass lifting, large surface area, outstanding wound curing activities, exciting nanosheet/nanobelt structure, enormous-scale productivity, and NIR-responsive features. This simple method for creating nanosheets and nanobelts opens up new possibilities for the manufacturing and applications of nanomaterial. Fig. 6 describes the fabrication and coating of T-RMFs and Fig. 7 represents the healing of skin wounds with various groups.
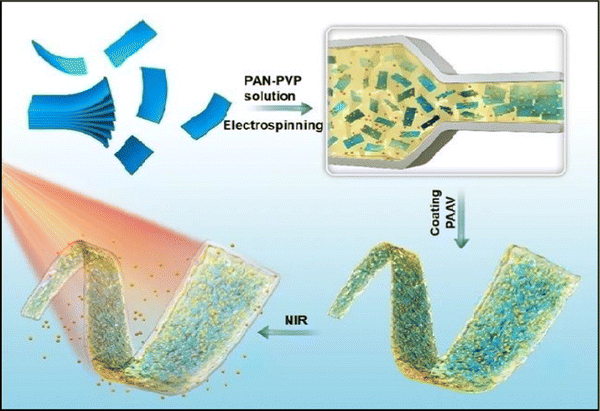 |
| Fig. 6 Design of T-RMF nanobelt surface coating and fabrication (adapted from ref. 142 under Creative Commons License 4.0). | |
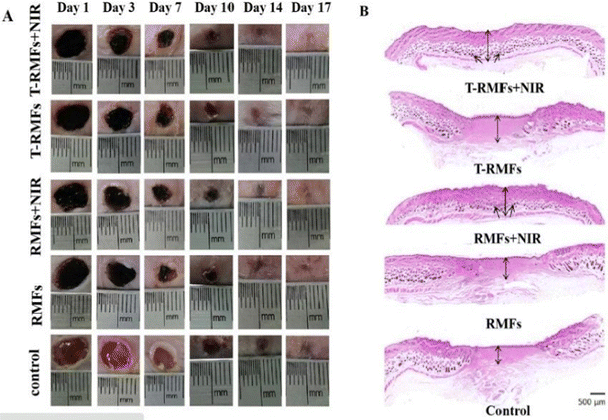 |
| Fig. 7 Pictures of the skin wounds in different groups (T-RMF + NIR group, T-RMF group, RMF + NIR group, RMF group, and control group) on days 1, 3, 7, 10, 14, and 17 (adapted from ref. 142 under Creative Commons License 4.0). | |
Xu et al. (2021)38 investigated a multimodal antimicrobial material based on an MXene to treat wound infections. They investigated electrostatic spinning nanofibrous membranes with a three-dimensional framework, which have been widely researched as potential wound-healing materials to preserve the water-absorbing balance in lesions and heal wounds. An MXene, polyvinyl alcohol (PVA), and amoxicillin (AMX) were electrospun into an antimicrobial nanofibrous membrane (MXene-PVA-AMX nanofibrous-membrane). The PVA matrix in the nanocomposite membrane may stimulate the dissemination of AMX to fight against pathogens. Simultaneously, the MXene may convert near-infrared irradiation to heat, causing local hyperthermia and promoting the release of AMX. The bacteriostatic activity and wound healing capability of the composite nanofibrous membrane were tested in vitro on S. aureus- and S. aureus-infected mice as defected models. This film served as a physical block for loading the MXene and AMX. It demonstrated significant antimicrobial and rapid wound healing capabilities, paving the way for the development of new wound-healing dressings and antibacterial techniques.38
Interestingly, Mayerberger et al. (2018)143 proposed the development of stretchable bandage composites with antibacterial properties by functionalizing electrospun CS nanofiber mats with Ti3C2Tz particles. Using an electrospinning method, they demonstrated how to develop embedded Ti3C2Tz (MXene) particles in chitosan nanofibers for inactive antimicrobial wound healing prospects. Ti3C2Tz particles were effectively integrated in the chitosan nanofibers without altering the strength of the nanofibers, as shown by SEM (Fig. 8(a) and TEM Fig. 8(b)). The Gram −ve E. coli had a 95% reduction in colony-forming units, while the Gram +ve S. aureus had a 62% reduction in colony forming units after in vitro antibacterial investigations on crosslinked Ti3C2Tz/chitosan composite fibres. The bactericidal MXene/chitosan nanofibers were shown to be non-toxic after the cytotoxicity tests, proving their biocompatibility. The findings revealed that Ti3C2Tz/CS nanofiber mats have high antibacterial activity and may be effective wound dressing materials.143
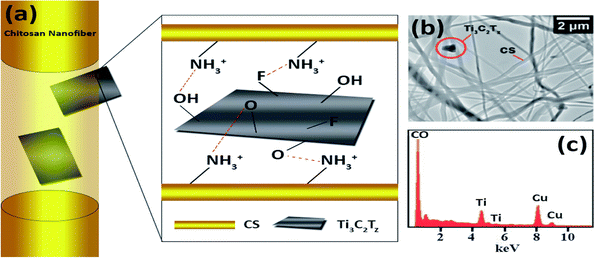 |
| Fig. 8 (a) Schematic representations of the observed orientations of Ti3C2Tz flakes inside chitosan nanofibers. (b) Transmission electron microscopy image of the Ti3C2Tz/CS nano-fibers and (c) elemental examination (presence of copper is attributed to the TEM grid). (Adapted from ref. 143 under Creative Commons License 3.0, Copyright©2018, The Royal Society of Chemistry.) | |
Zhou et al. (2021)144 created multi-functional scaffolds based on Ti3C2Tx MXene nanosheets, which exhibited an antibacterial hemostatic function for improving multidrug resistance bacteria-infected wound healing. In this study, a 2D (two-dimensional) Ti3C2TxMXene was employed to develop a multipurpose scaffold (HPEM) for wound healing with multidrug-resistant Staphylococcus aureus (MRSA). The HPEM scaffolds were created by combining poly(glycerol-ethylenimine), Ti3C2TxMXene@polydopamine (MXene@PDA) nanosheets and oxidized hyaluronic acid (HCHO). Consequently, self-healing, electrical conductivity, tissue adhesion, antimicrobial activities, particularly for MRSA resistant to multiple commonly used medicines with 99.03% bactericidal effectiveness, and the ability to stop bleeding were all demonstrated in the HPEM scaffolds. Also, the HPEM scaffolds boosted natural skin cell proliferation, while offering low danger of damage.144
Moreover, the HPEM scaffolds stimulated the recovery of MRSA-infected wounds (ratio of wound closure of about 96.31%) by increasing collagen deposition, granulation tissue development, angiogenesis, vascular endothelial differentiation, and cell growth angiogenic process. This study demonstrated the usefulness of multi-functional 2D MXene@PDA nanosheets in the closure of infected wounds. MRSA-infected wounds and skin regeneration could be treated with the HPEM scaffolds with versatile characteristics. According to this study, multifunctional conductive HPEM scaffolds can expedite wound curing and skin repair by encouraging early angiogenesis in infected wounds. Fig. 9 describes the healing of wounds infected by bacteria by using an HPEM scaffold.144
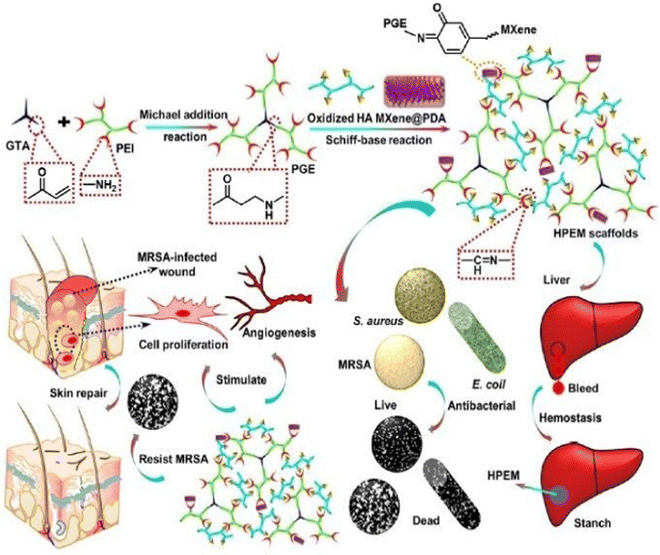 |
| Fig. 9 Representation of the application and fabrication of HPEM scaffolds in multidrug-resistant bacteria-infected wound healing (adapted with permission from ref. 144 Copyright© 2021, the American Chemical Society). | |
Sun et al. (2017)145 prepared microneedle patches integrated with an MXene and encapsulated innate molecule for wound healing. A PBA (3-(acrylamido)phenylboronic acid) integrated with PEGDA (polyethylene glycol diacrylate)hydrogel was used as the host material of the microneedle patches (Fig. 10). This is because of the potential covalent interaction of boronate molecules with adenosine. The release of loaded adenosine may be enhanced under NIR irradiation due to the photo-thermal conversion capacity of the MXene to maintain the stimulation signal at the injury site. The MXene-integrated microneedle covered with encapsulated adenosine enhanced the angiogenesis in vitro cell studies. This study concluded that microneedle patches proliferated effectively when used to treat animal models.
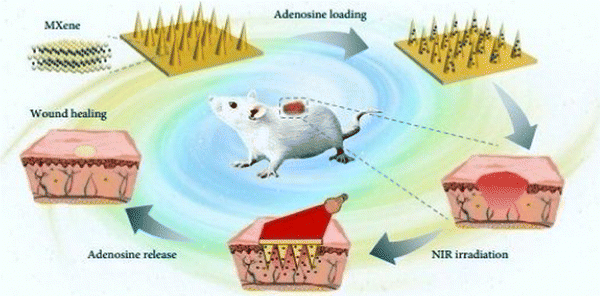 |
| Fig. 10 Schematic illustration of the mechanism of MXene-integrated microneedle patches with the encapsulation of adenosine for healing wound. (Adapted from ref. 145 under Creative Commons License 4.0 Copyright © 2021.) | |
Table 2 summarizes all the reported methods used for wound healing applications employing MXenes. This table overviews the work reported by various researchers.38,141–145 Different raw materials such as regenerative bacterial cellulose (rBC), Ti3C2Tx, polyacrylonitrile, polyvinyl pyrrolidone composite nanobelts, amoxicillin (AMX), MXene, polyvinyl alcohol, CS nanofiber mats with Ti3C2Tz particles, poly(glycerol-ethylenimine), Ti3C2TxMXene@polydopamine (MXene@PDA) nanosheets, oxidized hyaluronic acid (HCHO), and PBA-(3-(acrylamido)phenyl boronic acid)-integrated PEGDA (polyethylene glycol diacrylate) hydrogel were used by various researchers. These materials were obtained as hydrogels, temperature-responsive MXene nanobelt fibres (T-RMFs), stretchable bandages, microneedle patches, nanofibrous membranes, etc. The main methods for the synthesis of these materials include electrical stimulation, electrospinning, and NIR irradiation for developing MXene-based materials for wound applications.
Table 2 Summary of several types of MXenes for wound healing applications
S. no. |
Raw materials |
Method |
MXene-based developed material |
Ref. |
1 |
Regenerative bacterial cellulose (rBC) and Ti3C2Tx |
Electrical stimulation |
|
141
|
2 |
Polyvinylpyrrolidone and polyacrylonitrile composite nanobelts with a thermosensitive coating layer of PAAV |
NIR irradiation |
|
142
|
3 |
Amoxicillin (AMX), MXene, and polyvinyl alcohol (PVA) |
Electro-spinning |
|
38
|
4 |
Electrospun CS nanofiber mats with Ti3C2Tz particles |
Electro-spinning |
|
143
|
5 |
Poly(glycerol-ethylenimine), Ti3C2Tx MXene@polydopamine (MXene@PDA) nanosheets, and oxidised hyaluronic acid (HCHO) |
Fabrication and synthesis |
|
144
|
6 |
PBA(3-(acrylamido)phenylboronic acid) integrated PEGDA (polyethylene glycol diacrylate) hydrogel |
NIR irradiation |
|
145
|
4. Toxicity and biosafety issues
Due to its high efficiency, easy implementation and versatility in management, a wound treating method based on the conventional nature of the human skin has emerged in the last ten years and gained significant interest.146 Conventional organic materials with superior cytocompatibility have been reported for various biological applications; however, their poor chemical/thermal stability and single capabilities are the main disadvantages impacting their progress in healthcare.147 In contrast, inorganic 2D MXene-based nanomaterials have considerably large diagnostic interpretation prospects due to their intrinsic properties,148 including easy bioconjugation, controllable morphological characteristics, favourable bio-compatibility, precise microbial degradation, multi-functionalism and comparatively high physiological consistency.149 These characteristics are typically challenging to attain in most organic units. Even though MXenes have unique biomedicine practices, the bio-safety of the emerging 2D MXene family helps determine their ability in upcoming medical applications. In contrast to the well-researched carbon nanotubes, graphene oxide, and gold nano-systems, 2D MXenes and their compounds have recently become novel therapeutic nano-platforms. Consequently, investigation of their therapeutic and diagnostic effectiveness, biological impacts, and toxicology is still in its early stages.150 The toxicity analysis of different 2D nanomaterials is under study; however, the medicinal chemistry effects of 2D nanomaterials, such as their bioavailability, water-solubility, long-term toxic effects, and microbial degradation, remain unclear.25,151
According to an investigation, MXenes and MXene-based composites have low cytotoxicity effects against cells. The probable bio-safety and bio-compatibility of surface-modified MXene nanosheets against mice were also assessed in vivo.152 It was discovered that there was no difference in mouse behavior among the three treatment groups (exposed to NIR-I, NIR-II, and direct sunlight) and the control subjects. Also, no difference in mouse body weights was noticed over three durations. Haematoxylin and eosin (H&E) immune-histochemical assays were performed for significant organs (liver, heart, kidney, lung, and spleen) after a 28 day feed intake, with no apparent acute or chronic symptoms histopathologic toxic effects detected after comparison with the control and treatment groups. The blood tests were within normal parameters including haematological and cell biology factors. It is significant to note that Nb-based varieties produced by the bio-degradation of Nb2C MXene can foster the neogenesis and migration of blood vessels in the injured area, which can export more oxygen, vitamins, and energy around the surrounding bone during the repairment process, and also assemble more immune cells all over the damaged site to speed up the deterioration of the developed composite.153 These findings suggest that Nb2C-PVP is bio-compatible for future in vivo tumour theranostic modalities. The same evaluations were performed on Ti3C2 and Ta4C3 MXenes134 with marginal cytotoxic effects and sound in vivo biocompatibility. Moreover, regarding the present findings, more research and improvement of in vitro and in vivo toxicological evaluations, such as mutagenicity152 and reproductive toxicity154 are critical to fully utilize the ability of MXene-based theranostic nano-platforms for potent biomedical applications.
5. Challenges and future outlook
Current research on MXenes and MXene-based materials emphasizes the significance of controlling their surface morphology for potentially innovative bioengineering and nanomedicine applications.155 MXene-based nano-platforms must be biocompatible and stable in all physiological circumstances, with elevated targeting abilities, specificity and sustained release behavioral patterns.156 MXenes have undoubtedly proven to possess numerous biological potencies, particularly in biological imaging, biosensors, cancer treatment, antimicrobial activities, drug tissue regeneration, and cell therapy, due to their unique physico-chemical characteristics.2,132 These 2D materials can be efficiently designed and synthesized using cost-effective and straightforward techniques for complex healthcare gadgets.157 By modifying their synthetic features and implementing optimum circumstances, their physical characteristics can be adjusted for particular uses such as wound dressings, power storage, imaging, biosensors, and water filtering. Also, MXenes have low toxicity and can be employed to treat cancer, germs and modulate the immune system.158
The marvellous electro-optic attributes of MXenes make them perfect representatives for detecting a broad spectrum of bio-electronic signals. Future studies should focus on functional scaffolds (both electrical and mechanical) for wound dressings, partition membranes for organ transplants, intracellular fluorescent probes and the transplantation of bio-electronic peripheral areas for identifying neuro-transmitter and bio-electronic signals. Furthermore, novel sustainability and eco-friendly synthetic approaches for the environmental-friendly production of MXenes and their multifunctional modifications for novel wound dressing applications should be investigated.159 The size and shape effects of MXenes on their characteristics and the interrelations between MXenes and drugs or cells must be thoroughly and technically evaluated. Also, their toxic effects, bio-compatibility, cytocompatibility, histological, and bio-safety concerns must also be systematically investigated, as shown in Fig. 11.
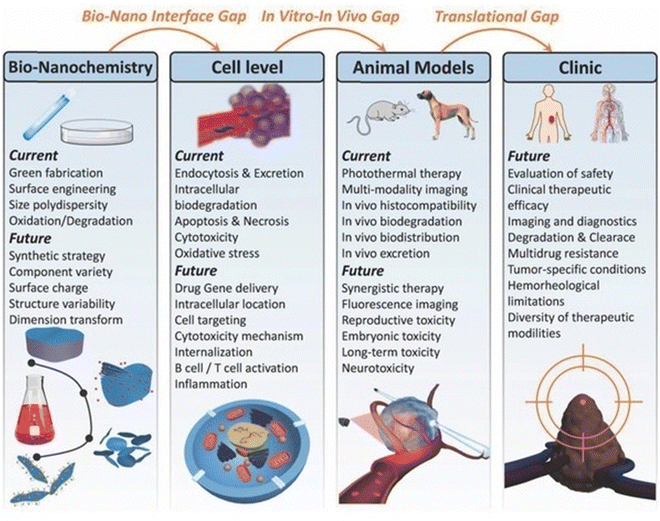 |
| Fig. 11 Use of 2D ultrathin MXenes in the area of nanomedicine. (Adapted from ref. 160 under Creative Commons License 4.0 Copyright©2018.) | |
Bio-functionalization and biochemical surface changes can enhance numerous features, such as efficiency, durability, bio-availability, biocompatibility, and cytocompatibility, and minimize adverse effects/immune system responses and improve targeted properties.158,159,161 Because of their high antibacterial action, the chemistry of MXenes enable their use in previously unexplored areas such as surface treatment of medical catheters, gloves, and masks.162 However, research on bioactive MXene-based composites is still restricted. Greater emphasis should be paid to the systematic evaluation and modification of the cytotoxicity of MXene compounds.163,164 The cell absorption characteristics, cytotoxic mechanisms, and in vivo and in vitro properties of MXenes, for example, should be thoroughly explored. Consequently, the physiological effects of MXenes must be entirely understood, given that MXene-based substances may accumulate in the body over time, potentially causing toxic effects. There have been no studies on the interactions of MXenes with the biological mechanism to date.46
6. Conclusion
Due to their ultrathin structural features, unique morphological structures, and specialized physicochemical, electrical, optical, and biological properties, MXene-based materials have shown great potential in healthcare applications, particularly wound dressing applications. These materials can be fabricated using facile chemical processes such as electrical stimulation, electrospinning, and NIR irradiation to develop MXene-based materials, which can be helpful for applications in the healthcare sector. Further, the productivity of the samples can be improved by enhancing their chemical composition and the process parameters during their synthetic process. Especially for wound dressing applications, these MXene-based materials can be obtained in various forms such as hydrogen temperature-responsive MXene nanobelt fibres (TRMFs), stretchable bandages, microneedle patches, and nanofibrous membranes.
Statement of ethical approval, ethical standards
The authors declare no involvement of animal studies or human participants in the study.
Funding statement
This research received no specific grant from any funding agency.
Conflicts of interest
The authors declare no conflict of interest.
References
- J. Li, H. Zhu, M. Zhang, X. Zheng, Z. Di, X. Liu and X. Wang, Sci. Rep., 2014, 4, 4359 CrossRef PubMed.
- H. Huang, R. Jiang, Y. Feng, H. Ouyang, N. Zhou, X. Zhang and Y. Wei, Nanoscale, 2020, 1325–1338 RSC.
- S. Verma, U. Dwivedi, K. Chaturvedi, N. Kumari, M. Dhangar, S. A. Hashmi, R. Singhal and A. K. Srivastava, Synth. Met., 2022, 287, 117095 CrossRef CAS.
- H. Riazi, K. Nemani, M. Grady, B. Anasori and M. Soroush, J. Mater. Chem. A, 2021, 9, 8051–8098 RSC.
- C. Zhang, L. Cui, S. Abdolhossein Zadeh and J. Heier, InfoMat, 2020, 4, 613–638 CrossRef.
- C. Peng, P. Wei, X. Chen, Y. Zhang, F. Zhu, Y. Cao, H. Wang, H. Yu and F. Peng, Ceram. Int., 2018, 44, 18886–18893 CrossRef CAS.
- H. Lin, S. Gao, C. Dai, Y. Chen and J. Shi, J. Am. Chem. Soc., 2017, 139, 16235–16247 CrossRef CAS PubMed.
- V. Shukla, Mater. Adv., 2020, 9, 3104–3121 RSC.
-
Z. Xiao, S. Ruan, L. B. Kong, W. Que, K. Zhou, Y. Liu and T. Zhang, MXenes and MXenes-based Composites, Springer Nature, 2020 Search PubMed.
- X. Zhan, C. Si, J. Zhou and Z. Sun, Nanoscale Horizon., 2020, 5, 235–258 RSC.
- M. Mozafari and M. Soroush, Mater. Adv., 2021, 7277–7307 RSC.
- N. Baig and I. Kammakakam, Mater. Adv., 2021, 2, 1821–1871 RSC.
- K. Hantanasirisakul, M. Q. Zhao, P. Urbankowski, J. Halim, B. Anasori, S. Kota, C. E. Ren, M. W. Barsoum and Y. Gogotsi, Adv. Electron. Mater., 2016, 2 Search PubMed.
- R. Sun, H. B. Zhang, J. Liu, X. Xie, R. Yang, Y. Li, S. Hong and Z. Z. Yu, Adv. Funct. Mater., 2017, 27, 1702807 CrossRef.
- C. Ashley, E. Carnes, G. Phillips, D. Padilla, P. Durfee, P. Brown, T. Hanna, J. Liu, B. Phillips, M. Carter, N. Carroll, X. Jiang, D. Dunphy, C. Willman, D. Petsev, D. Evans, A. Parikh, B. Chackerian, W. Wharton and C. Brinker, Naturematerials, 2011, 10, 476 CrossRef CAS.
- G. Hong, J. Lee, J. Robinson, U. Raaz, L. Xie, N. Huang, J. Cooke and H. Dai, Nat. Med., 2012, 18, 1841–1846 CrossRef CAS PubMed.
- N. Kotagiri, G. Sudlow, W. Akers and S. Achilefu, Nat. Nanotechnol., 2015, 10, 370–379 CrossRef CAS PubMed.
- S. Mura, J. Nicolas and P. Couvreur, Nat. Mater., 2013, 12, 991–1003 CrossRef CAS PubMed.
- H. Chen, W. Zhang, G. Zhu, J. Xie and X. Chen, Nat. Rev. Mater., 2017, 2, 17024 CrossRef CAS PubMed.
- M. Ferrari, Nat. Rev. Cancer, 2005, 5, 161–171 CrossRef CAS PubMed.
- K. Shin, J. Choi, G. Ko, S. Baik, D. Kim, O. K. Park, K. Lee, H. R. Cho, S. Han, S. H. Lee, D. Lee, N. Lee, H. C. Kim and T. Hyeon, Nat. Commun., 2017, 8, 15807 CrossRef CAS.
- C. Liang, L. Xu, G. Song and Z. Liu, Chem. Soc. Rev., 2016, 45, 6250–6269 RSC.
- Y. Lu, A. Aimetti, R. Langer and Z. Gu, Nat. Rev. Mater., 2016, 1, 16075 Search PubMed.
- D. Ni, W. Bu, E. Ehlerding, W. Cai and J. Shi, Chem. Soc. Rev., 2017, 46, 7438–7468 RSC.
- H. Lin, Y. Chen and J. Shi, Chem. Soc. Rev., 2018, 47, 1938–1958 RSC.
- K. Yang, L. Feng, X. Shi and Z. Liu, Chem. Soc. Rev., 2012, 42, 530–547 RSC.
- G. Reina, J. González-Domínguez, A. CriadoFernández, E. Vázquez, A. Bianco and M. Prato, Chem. Soc. Rev., 2017, 46, 4400–4416 RSC.
- X. Qian, Z. Gu and Y. Chen, Mater. Horiz., 2017, 4, 800–816 RSC.
- H. Mao, S. Laurent, W. Chen, O. Akhavan, M. Imani, A. A. Ashkarran and M. Mahmoudi, Chem. Rev., 2013, 113, 3407–3424 CrossRef CAS PubMed.
- R. Kurapati, K. Kostarelos, M. Prato and A. Bianco, Adv. Mater., 2016, 28, 6052–6074 CrossRef CAS PubMed.
- L. Feng, L. Wu and X. Qu, Adv. Mater., 2013, 25, 168–186 CrossRef CAS PubMed.
- D. Chimene, D. Alge and A. Gaharwar, Adv. Mater., 2015, 27, 7261–7284 CrossRef CAS.
- Y. Chen, C. L. Tan and H. Zhang, Catal. Commun., 2015, 44, 2681–2701 CAS.
- A. Naskar and K. Kim, Pharmaceutics, 2020, 12, 499 CrossRef CAS PubMed.
- S. Dhivya, V. Padma and S. Elango, BioMedicine, 2015, 5, 1–5 CrossRef PubMed.
- S. Baranoski and E. Ayello, Adv. Skin Wound Care, 2012, 25, 87–92 CrossRef PubMed ; quiz 92.
- D. Simões, S. Miguel, M. Ribeiro, P. Coutinho, A. Mendonça and I. Correia, Eur. J. Pharm. Biopharm., 2018, 127, 130–141 CrossRef.
- X. Xu, S. Wang, H. Wu, Y. Liu, F. Xu and J. Zhao, Colloids Surf., B, 2021, 207, 111979 CrossRef CAS PubMed.
- R. Ronchi, J. Arantes and S. Santos, Ceram. Int., 2019, 45, 18167–18188 CrossRef CAS.
- L. Fu, Adv. Eng. Mater., 2020, 23, 2001191 CrossRef.
- P. Eklund, M. Beckers, U. Jansson, H. Högberg and L. Hultman, Thin Solid Films, 2010, 518, 1851–1878 CrossRef CAS.
-
M. Barsoum and P. Eklund, The Mn+1AXn Phases:
The Precursors for MXenes, 2019, pp. 15–35.
-
M. Barsoum, A New Class of Solids; Thermodynamically Stable Nanolaminates, 2000, vol. 28, pp. 28–201 Search PubMed.
-
M. Barsoum, MAX Phases: Properties of Machinable Ternary Carbides and Nitrides, 2013 Search PubMed.
-
I. Low, Advances in Science & Technology of MAX Phases, 2012 Search PubMed.
- A. Zamhuri, G. Lim, N. L. Ma, K. Tee and C. Soon, BioMed. Eng. OnLine, 2021, 20, 1–24 CrossRef PubMed.
- M. Naguib, V. Mochalin, M. Barsoum and Y. Gogotsi, Adv. Mater., 2014, 26, 982 CrossRef.
- M. Khazaei, M. Arai, T. Sasaki, C.-Y. Chung, V. Natarajan Sathiyamoorthy, M. Estili, Y. Sakka and Y. Kawazoe, Adv. Funct. Mater., 2013, 23, 2185–2192 CrossRef CAS.
- J. Zhou, X.-H. Zha, Z. Xiaobing, F. Chen, G. Gao, S. Wang, C. Shen, T. Chen, C. Zhi, P. Eklund, S. Du, J. Xue, W. Shi, Z. Chai and Q. Huang, ACS Nano, 2017, 11, 3841–3850 CrossRef CAS PubMed.
- A. Enyashin and A. Ivanovskii, J. Solid State Chem., 2013, 207, 42–48 CrossRef CAS.
- N. Chaudhari, H. Jin, B. Kim, D. S. Baek, S. Joo and K. Lee, J. Mater. Chem. A, 2018, 6, 24564–24579 RSC.
- M. Naguib, O. Mashtalir, J. Carle, V. Presser, J. Lu, L. Hultman, Y. Gogotsi and M. Barsoum, ACS Nano, 2012, 6, 1322–1331 CrossRef CAS.
- K. Papadopoulou, A. Chroneos, D. Parfitt and S.-R. G. Christopoulos, J. Appl. Phys., 2020, 128, 170902 CrossRef CAS.
- M. Naguib, M. Kurtoglu, V. Presser, J. Lu, J. Niu, M. Heon, L. Hultman, Y. Gogotsi and M. Barsoum, Adv. Mater., 2011, 23, 4207 CrossRef.
- J. Halim, M. Lukatskaya, K. Cook, J. Lu, C. Smith, L.-Å. Näslund, S. May, L. Hultman, Y. Gogotsi, P. Eklund and M. Barsoum, Chem. Mater., 2014, 26, 2374–2381 CrossRef CAS PubMed.
- J. Pang, R. Mendes, A. Bachmatiuk, L. Zhao, H. Ta, T. Gemming, H. Liu, Z. Liu and M. Rümmeli, Chem. Soc. Rev., 2018, 48, 72–133 RSC.
- B. Wyatt, A. Rosenkranz and B. Anasori, Adv. Mater., 2021, 33, 2007973 CrossRef CAS PubMed.
- L. Verger, V. Natu, M. Carey and M. Barsoum, Trends Chem., 2019, 1, 656–669 CrossRef CAS.
- J. Zhou, S. Gao, Z. Guo and Z. Sun, Ceram. Int., 2017, 43, 11450–11454 CrossRef CAS.
- M. Tran, T. Schäfer, A. Shahraei, M. Duerrschnabel, L. Molina-Luna, U. Kramm and C. Birkel, ACS Appl. Energy Mater., 2018, 1, 3908–3914 CrossRef CAS.
- M. Ghidiu, M. Lukatskaya, M.-Q. Zhao, Y. Gogotsi and M. Barsoum, Nature, 2014, 78–81 CrossRef CAS PubMed.
- J. Yang, W. Bao, P. Jaumaux, S. Zhang, C. Wang and G. Wang, Adv. Mater. Interfaces, 2019, 6, 1802004 CrossRef.
- Y. Gogotsi, Nat. Mater., 2015, 14, 1079–1080 CrossRef CAS PubMed.
- L. Ma, L. Ting, V. Molinari, C. Giordano and B. Yeo, J. Mater. Chem. A, 2015, 3, 8361–8368 RSC.
- T. Li, L. Yao, Q. Liu, J. Gu, R. Luo, J. Li, X. Yan, W. Wang, P. Liu and B. Chen, Angew. Chem., Int. Ed., 2018, 57, 6115–6119 CrossRef CAS PubMed.
- S. Yang, P. Zhang, F. Wang, A. G. Ricciardulli, M. Lohe, P. Blom and X. Feng, Angew. Chem., Int. Ed., 2018, 57, 15491–15495 CrossRef CAS PubMed.
- W. Sun, S. Shah, Y. Chen, Z. Tan, H. Gao, T. Habib, M. Radovic and M. Green, J. Mater. Chem. A, 2017, 5, 21663–21668 RSC.
- P. Urbankowski, B. Anasori, T. Makaryan, D. Er, S. Kota, P. Walsh, M. Zhao, V. Shenoy, M. Barsoum and Y. Gogotsi, Nanoscale, 2016, 8, 11385–11391 RSC.
- L. Verger, C. Xu, V. Natu, H. M. Cheng, W. Ren and M. Barsoum, Curr. Opin. Solid State Mater. Sci., 2019, 23, 149–163 CrossRef CAS.
- L. Karlsson, J. Birch, J. Halim, M. Barsoum and P. Persson, Nano Lett., 2015, 15, 4955–4960 CrossRef CAS.
-
M. Li, J. Lu, K. Luo, Y. Li, K. Chang, K. Chen, J. Zhou, J. Rosén, L. Hultman, P. Eklund, P. Persson, S. Du, Z. Chai, Z. Huang and Q. Huang, Element Replacement Approach by Reaction with Lewis Acidic Molten Salts to Synthesize Nanolaminated MAXPhases and MXenes, 2019 Search PubMed.
- A. Sundaram, J. Ponraj, C. Wang, W. K. Peng, M. Kumar, S. Dhanabalan and J. Gaspar, J. Mater. Chem. B, 2020, 8, 4990–5013 RSC.
- H. Huang, W. Feng, Y. Chen and J. Shi, Nano Today, 2020, 35, 100972 CrossRef CAS.
- S. Wang, H. Zheng, L. Zhou, F. Cheng, C. K. Liu, H. Zhang, L. Wang and Q. Zhang, Nano Lett., 2020, 5149–5158 CrossRef CAS PubMed.
- K. Huang, Z. Li, J. Lin, G. Han and P. Huang, Chem. Soc. Rev., 2018, 47, 5109–5124 RSC.
- J. Heikenfeld, A. Jajack, J. Rogers, P. Gutruf, L. Tian, T. Pan, R. Li, M. Khine, J. Kim and J. Wang, Lab Chip, 2018, 2, 217–248 RSC.
- Y. Liu, M. Pharr and G. A. Salvatore, ACS Nano, 2017, 10, 9614–9635 CrossRef PubMed.
- F. Vitale and B. Litt, Bioelectron. Med., 2018, 1, 3–7 CrossRef.
- J. J. Norton, D. S. Lee, J. W. Lee, W. Lee, O. Kwon, P. Won, S. Y. Jung, H. Cheng, J. W. Jeong, A. Akce and S. Umunna, Proc. Natl. Acad. Sci. U. S. A., 2015, 13, 3920–3925 CrossRef PubMed.
- B. Xu, A. Akhtar, Y. Liu, H. Chen, W. H. Yeo, S. I. Park, B. Boyce, H. Kim, J. Yu, H. Y. Lai and S. Jung, Adv. Mater., 2016, 22, 4462–4471 CrossRef PubMed.
- D. McFarland and J. R. Wolpaw, Curr. Opin. Biomed. Eng., 2017, 4, 194–200 CrossRef CAS.
- S. Lee, W. Y. Peh, J. Wang, F. Yang, J. S. Ho, N. V. Thakor, S. C. Yen and C. Lee, Adv. Sci., 2017, 11, 1700149 CrossRef.
- M. R. DeLong and T. Wichmann, JAMA Neurol., 2015, 11, 1354–1360 CrossRef.
- G. T. Hwang, H. Park, J. H. Lee, S. Oh, K. I. Park, M. Byun, H. Park, G. Ahn, C. K. Jeong, K. No and H. Kwon, Adv. Mater., 2014, 28, 4754 CrossRef.
- L. Ulloa, S. Quiroz-Gonzalez and R. Torres-Rosas, Trends Mol. Med., 2017, 12, 1103–1120 CrossRef.
- J. Cheng, H. Shen, R. Chowdhury, T. Abdi, F. Selaru and J. D. Chen, Inflamm. Bowel Dis., 2020, 8, 1119–1130 CrossRef.
- N. Driscoll, B. Erickson, B. B. Murphy, A. G. Richardson, G. Robbins, N. V. Apollo, G. Mentzelopoulos, T. Mathis, K. Hantanasirisakul, P. Bagga and S. E. Gullbrand, Sci. Transl. Med., 2021, 612, eabf8629 CrossRef PubMed.
- Y. Bai, K. Zhou, S. Narasimalu, J. Pang, X. He and R. Wang, RSC Adv., 2016, 6, 35731–35739 RSC.
- M. Magnuson, J. Halim and L.-Å. Näslund, J. Electron Spectrosc. Relat. Phenom., 2018, 224, 27–32 CrossRef CAS.
- X.-H. Zha, J. Yin, Y. Zhou, Q. Huang, K. Luo, J. Lang, J. Francisco, J. He and S. Du, J. Phys. Chem. C, 2016, 120, 15082–15088 CrossRef CAS.
- P. Chakraborty, T. Das, D. Nafday, L. Boeri and T. Saha-Dasgupta, Phys. Rev. B, 2017, 95, 184106 CrossRef.
- U. Yorulmaz, A. Ozden, N. Perkgoz, F. Ay and C. Sevik, Nanotechnology, 2016, 27, 335702 CrossRef PubMed.
- O. Mashtalir, M. Lukatskaya, A. Kolesnikov, E. Raymundo-Piñero, M. Naguib, M. Barsoumand and Y. Gogotsi, Nanoscale, 2016, 8, 9128–9133 RSC.
- B. Anasori, M. R. Lukatskaya and Y. Gogotsi, Nat. Rev. Mater., 2017, 2, 1–17 Search PubMed.
- V. Borysiuk, V. Mochalin and Y. Gogotsi, Nanotechnology, 2015, 26, 265705 CrossRef PubMed.
- V. Borysiuk and V. Mochalin, MRS Commun., 2019, 9, 1–6 CrossRef.
- C. Zhang and V. Nicolosi, Energy Storage Mater., 2018, 16, 102–125 CrossRef.
- A. Ivanovskii and A. Enyashin, Russian Chem. Rev., 2013, 82, 735 CrossRef.
- K. Hantanasirisakul and Y. Gogotsi, Adv. Mater., 2018, 30, 1804779 CrossRef PubMed.
- H. Kim, Z. Wang and H. Alshareef, Nano Energy, 2019, 60, 179–197 CrossRef CAS.
- L. Dong, H. Kumar, B. Anasori, Y. Gogotsi and V. Shenoy, J. Phys. Chem. Lett., 2016, 8, 422–428 CrossRef.
- B. Anasori, Y. Xie, M. Beidaghi, J. Lu, B. Hosler, L. Hultman, P. Kent, Y. Gogotsi and M. Barsoum, ACS Nano, 2015, 9, 9507–9516 CrossRef CAS PubMed.
- B. Anasori, C. Shi, E. J. Moon, Y. Xie, C. Voigt, P. Kent, S. May, S. Billinge, M. Barsoum and Y. Gogotsi, Nanoscale Horiz., 2016, 1, 227–234 RSC.
- W. Sun, Y. Xie and P. Kent, Nanoscale, 2018, 10, 11962–11968 RSC.
- Q. Tao, M. Dahlqvist, J. Lu, S. Kota, R. Meshkian, J. Halim, J. Palisaitis, L. Hultman, M. Barsoum, P. Persson and J. Rosén, Nat. Commun., 2017, 8, 14949 CrossRef PubMed.
- M. Dahlqvist, A. Petruhins, J. Lu, L. Hultman and J. Rosen, ACS Nano, 2018, 12, 7761–7770 CrossRef CAS.
- J. Zhu, A. Chroneos, J. Eppinger and U. Schwingenschlögl, Appl. Mater. Today, 2016, 5, 19–24 CrossRef.
- A. Bandyopadhyay, D. Ghosh and S. Pati, Phys. Chem. Chem. Phys., 2018, 20, 4012–4019 RSC.
-
K. Chaudhuri, Z. Wang, M. Alhabeb, K. Maleski, Y. Gogotsi, V. Shalaev and A. Boltasseva, Optical properties of MXenes, 2019, pp. 327–346.
- J. Halim, I. Persson, E. Moon, P. Kühne, V. Darakchieva, P. Persson, P. Eklund, J. Rosén and M. Barsoum, J. Phys.: Condens. Matter, 2019, 31, 165301 CrossRef CAS PubMed.
- X. Jiang, S. Liu, W. Liang, C. S. Luo, Z. He, Y. Ge, H. Wang, R. Cao, F. Zhang, Q. Wen, J. Li, Q. Bao and D. Fan, Laser Photonics Rev., 2018, 12, 1700229 CrossRef.
- X. Jiang, A. Kuklin, A. Baev, Y. Ge, H. Agren and P. Prasad, Phys. Rep., 2020, 1–58 CrossRef.
- O. Akhavan and E. Ghaderi, ACS Nano, 2010, 4, 5731–5736 CrossRef CAS PubMed.
- S. Chernousova and M. Epple, Angew. Chem., Int. Ed., 2012, 52, 1636–1653 CrossRef.
- J. Lemire, J. Harrison and R. Turner, Nat. Views, Microbiology, 2013, 11, 371–384 CrossRef CAS PubMed.
- Y. Li, W. Zhang, J. Niu and Y. Chen, ACS Nano, 2012, 6, 5164–5173 CrossRef CAS PubMed.
- K. Rasool, M. IHelal, A. Ali, C. Ren, Y. Gogotsi and K. Mahmoud, ACS Nano, 2016, 10, 3674–3684 CrossRef CAS PubMed.
- K. Rasool, K. Mahmoud, D. Johnson, M. IHelal, G. Berdiyorov and Y. Gogotsi, Sci. Rep., 2017, 7, 1598 CrossRef.
- A. Shamsabadi, G. H. Sharifian, B. Anasori and M. Soroush, ACS Sustainable Chem. Eng., 2018, 6, 16586–16596 CrossRef.
- G. Lim, C. Soon, M. Morsin, M. K. Ahmad, N. Nayan and K. Tee, Ceram. Int., 2020, 46, 20306–20312 CrossRef CAS.
- S. Mahmoudi, E. Mancini, L. Xu, A. Moore, F. Jahanbani, K. Hebestreit, R. Srinivasan, X. Li, K. Devarajan, L. Prélot, C. E. Ang, Y. Shibuya, B. Benayoun, A. Chang, M. Wernig, J. Wysocka, M. Longaker, M. Snyder and A. Brunet, Nature, 2019, 574, 553–558 CrossRef CAS.
- R. Tetley, M. Staddon, S. Banerjee and Y. Mao, Tissue Fluid. Promotes Epithelial Wound Heal., 2018, 1195–1203 Search PubMed.
- X. Zhao, A. Vashisth, J. Blivin, Z. Tan, D. Holta, V. Kotasthane, S. Shah, T. Habib, S. Liu, J. Lutkenhaus, M. Radovic and M. Green, Adv. Mater. Interfaces, 2020, 7, 2000845 CrossRef CAS.
- R. Hou, L. Wu, J. Wang, Z. Yang, Q. Tu and N. Huang, Biomolecules, 2019, 9, 69 CrossRef CAS PubMed.
- X. Wang, J. Jin, R. Hou, M. Zhou, X. Mou, K. Xu, Y. Zhu, Z. Shen and X. Zhang, ACS Appl. BioMater., 2019, 735–746 CAS.
- J. Ouyang, X. Ji, X. Zhang, C. Feng, Z. Tang, N. Kong, A. Xie, Æ. Wang, X. Sui, L. Deng, Y. Liu, J. Kim, Y. Cao and W. Tao, Proc. Natl. Acad. Sci. U. S. A., 2020, 117, 28667–28677 CrossRef CAS PubMed.
- T. Cui, J. Yu, Q. Li, C.-F. Wang, S. Chen, W. Li and G. Wang, Adv. Mater., 2020, 32, 2000982 CrossRef CAS PubMed.
- W. Srifa, N. Kosaric, A. Amorin, O. Jadi, Y. Park, S. Mantri, J. Camarena, G. Gurtner and M. Porteus, Nat. Commun., 2020, 11, 1–4 CrossRef.
- Z.-K. Li, Y. Wei, X. Gao, L. Ding, Z. Lu, J. Deng, X. Yang, J. Caro and H. Wang, Angew. Chem., Int. Ed., 2020, 59, 9838–9843 CrossRef.
- D. Xu, Z. Li, L. Li and J. Wang, Adv. Funct. Mater., 2020, 30, 2000712 CrossRef CAS.
- J. Lao, S. Wu, J. Gao, A. Dong, G. Li and J. Luo, Nano Energy, 2020, 70, 104481 CrossRef CAS.
- Q. Gao and H. Zhang, Nanoscale, 2020, 12, 5995–6001 RSC.
- S. Pan, J. Yin, L. Yu, C. Zhang, Y. Zhu, Y. Gao and Y. Chen, Adv. Sci., 2020, 7, 1901511 CrossRef CAS.
- X. Lin, Z. Li, J. Qiu, Q. Wang, J. Wang and T. Chen, Biomater. Sci., 2021, 9, 5437–5471 RSC.
- D. Gao, X. Guo, X. Zhang, S. Chen, Y. Wang, T. Chen, G. Huang, Y. Gao, Z. Tian and Z. Yang, Mater. Today Bio, 2020, 5, 100035 CrossRef CAS PubMed.
- S. Pan, J. Yin, L. Yu, C. Zhang, Y. Zhu, Y. Gao and Y. Chen, Adv. Sci., 2020, 2, 1901511 CrossRef PubMed.
- H. Liao, X. L. Guo, P. B. Wan and G. H. Yu, Adv. Funct. Mater., 2019, 29, 19040507 Search PubMed.
- A. Nikpasand and M. R. Parvizi, Bull. Emerg. Trauma, 2019, 4, 366 CrossRef PubMed.
- A. M. Jastrzębska, A. Szuplewska, T. Wojciechowski, M. Chudy, W. Ziemkowska, L. Chlubny, A. Rozmysłowska and A. Olszyna, J. Hazardous Mater., 2017, 339, 1–8 CrossRef PubMed.
- A. J. Alanis, Arch. Med. Res., 2005, 36, 697–705 CrossRef.
- L. Mao, S. Hu, Y. Gao, L. Wang, W. Zhao, L. Fu, H. Cheng, L. Xia, S. Xie, W. Ye, Z. Shi and G. Yang, Adv. Healthcare Mater., 2020, 2000872 CrossRef CAS PubMed.
- L. Jin, X. Guo, D. Gao, C. Wu, B. Hu, G. Tan, N. Du, X. Cai and Z. Yang, NPG Asia Mater., 2021, 13, 1–9 CrossRef.
- E. Mayerberger, R. Street, R. McDaniel, M. Barsoum and C. Schauer, RSC Adv., 2018, 8, 35386–35394 RSC.
- L. Zhou, H. Zheng, Z. Liu, S. Wang, C. K. Liu, F. Chen, H. Zhang, J. Kong, F. Zhou and Q. Zhang, ACS Nano, 2021, 15, 2468–2480 CrossRef CAS.
- L. Sun, L. Fan, F. Bian, G. Chen, Y. Wang and Y. Zhao, Research, 2021, 1–9 Search PubMed.
- R. Yu, H. Zhang and B. Guo, Nano-Micro Lett., 2022, 1, 1–46 Search PubMed.
- Y. Niu, J. Li, J. Gao, X. Ouyang, L. Cai and Q. Xu, Nano Res., 2021, 11, 3820–3839 CrossRef.
- K. Khan, A. K. Tareen, M. Aslam, R. U. Sagar, B. Zhang, W. Huang, A. Mahmood, N. Mahmood, K. Khan, H. Zhang and Z. Guo, Nano-Micro Lett., 2020, 1, 1–77 Search PubMed.
- S. Song, X. Jiang, H. Shen, W. Wu, Q. Shi, M. Wan, J. Zhang, H. Mo and J. Shen, ACS Appl. BioMater., 2021, 4, 6912–6923 CrossRef CAS.
- L. Zong, H. Wu, H. Lin and Y. Chen, Nano Res., 2018, 8, 4149–4168 CrossRef.
- X. Zhang, L. Cheng, Y. Lu, J. Tang, Q. Lv, X. Chen, Y. Chen and J. Liu, Nano-Micro Lett., 2022, 1, 1–21 Search PubMed.
- O. Akhavan, E. Ghaderi and A. Akhavan, Biomaterials, 2012, 33, 8017–8025 CrossRef CAS PubMed.
- J. Yin, S. Pan, X. Guo, Y. Gao, D. Zhu, Q. Yang, J. Gao, C. Zhang and Y. Chen, Nano-Micro Lett., 2021, 1, 1–8 Search PubMed.
- O. Akhavan, Carbon, 2015, 81, 158–166 CrossRef CAS.
- A. Rozmysłowska-Wojciechowska, J. Mitrzak, A. Szuplewska, M. Chudy, J. Woźniak, M. Petrus, T. Wojciechowski, A. Vasilchenko and A. Jastrzębska, Materials, 2020, 13, 17 Search PubMed.
- M. Xin, J. Li, Z. Ma, L. Pan and Y. Shi, Front. Chem., 2020, 8, 297 CrossRef CAS PubMed.
- G. JamalipourSoufi and S. Iravani, Green Chem., 2020, 22, 2662–2687 RSC.
- S. Iravani and R. Varma, Environ. Chem. Lett., 2020, 18, 703–727 CrossRef CAS PubMed.
- S. Iravani and R. Varma, Mater. Adv., 2021, 2906–2917 RSC.
- H. Lin, Y. Hen and J. Shi, Adv. Sci., 2018, 5, 1800518 CrossRef PubMed.
-
G. JamalipourSoufi, P. Iravani, A. Hekmatnia, E. Mostafavi, M. Khatami and S. Iravani, Comments on Inorganic Chemistry, 2021, pp. 1–34 Search PubMed.
- L. Wang, P. Hu, Z. Liu and X. He, J. Mater. Chem. A, 2017, 5, 22855–22876 RSC.
- A. Zavabeti, A. Jannat, L. Zhong, A. Haidry, Z. Yao and J. Ou, Nano-Micro Lett., 2020, 12, 1–34 CrossRef PubMed.
- B. Lu, Z. Zhu, B. Ma, W. Wang, R. Zhu and J. Zhang, Small, 2021, 2100946 CrossRef CAS PubMed.
Footnote |
† Academy of Council Scientific and Industrial Research-Advanced Materials and Processes Research Institute (AMPRI), Hoshangabad Road, Bhopal (M.P), 462026, India. |
|
This journal is © The Royal Society of Chemistry 2022 |
Click here to see how this site uses Cookies. View our privacy policy here.