DOI:
10.1039/D2MA00444E
(Review Article)
Mater. Adv., 2022,
3, 7425-7444
Therapeutic applications of magnetic nanoparticles: recent advances
Received
21st April 2022
, Accepted 5th August 2022
First published on 18th August 2022
Abstract
Magnetic nanoparticles (MNPs) show tremendous possibilities in the field of biomedicine, especially as therapeutic agents for use over a prolonged duration. Most notably, magnetic nanoparticles are widely used in magnetic hyperthermia, targeted drug delivery, photothermal therapy, photodynamic therapy and magnetofection for the treatment of cancer. The background of these applications relies mainly on interaction of the MNPs with an applied magnetic field, as well as light. The characteristics of the particles, such as size, crystal structure, shape, optical absorption, surface chemistry, magnetization and toxicity, have become important for building nanoparticles intended for various therapeutic applications. In this review advances in therapeutic MNPs for the field of biomedicine are highlighted, with a primary focus on synthetic methods, magnetic properties, therapeutic applications, challenges and future outlook.
1. Introduction
Magnetic nanoparticles (MNPs) are nanomaterials that consist of magnetic elements such as iron, cobalt, nickel and their alloys (Fig. 1), which show properties such as ferromagnetism and superparamagnetism.1 The use of magnetic nanoparticles in therapeutic applications has been recorded since ancient times. Hippocrates, the father of medicine, used styptic iron oxide to stop bleeding and to control haemorrhages.2 Currently, cellular labelling/repair, drug delivery, magnetic resonance imaging (MRI), tissue repair, magnetic hyperthermia therapy (MHT), photodynamic therapy (PDT), photothermal therapy (PTT) and magnetofection for gene delivery are some of the prominent examples of biomedical applications shown by MNPs.3–12 Such a variety of biomedical applications demands a narrow size distribution, a high surface-to-volume ratio, high biocompatibility, low toxicity, a high magnetic moment and the high magnetization of nanoparticles.13,14 Among the different MNPs, iron oxide nanoparticles (IONPs) have been the most widely studied nanomaterials for decades since they are safe, biocompatible, and have significant clinical utility. Different ways can be applied to initiate their therapeutic activity.15,16 Cancer hyperthermia therapy and MRI contrast enhancement are applications that have already been shown by US Food and Drug Administration (FDA)-approved IONPs.16,17 In addition, superparamagnetic iron oxide nanoparticles (SPIONs) are used as MRI contrast agents as they are non-toxic and have the ability to exhibit no residual magnetic force after the removal of the applied magnetic field.3
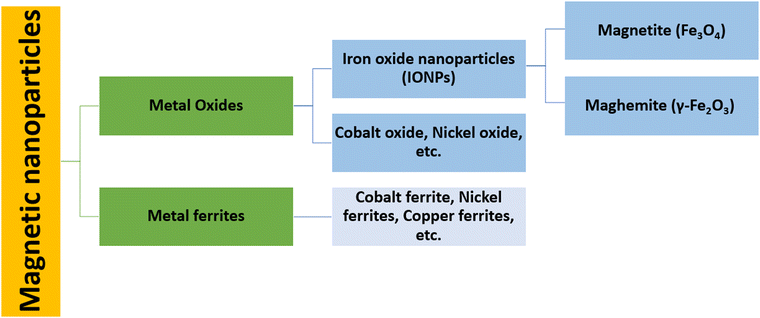 |
| Fig. 1 Classification of biomedically useful magnetic nanoparticles. | |
For more effective therapeutic treatments, transition metals (Fe, Co, Ni) or metal oxides (Fe3O4, γ-Fe2O3) are usually considered because of their high magnetic saturation.18,19 Although pure metals possess a high magnetic saturation, their high toxicity and extreme sensitivity to oxidation make them largely inappropriate for therapeutic applications.20 However, a stable magnetic response is given by iron oxide as it is less sensitive to oxidation. Nanoparticles of magnetite (Fe3O4) and maghemite (γ-Fe2O3) show a wide range of biomedical applications, such as drug delivery, cancer treatment via MHT, contrast enhancement in MRI, immunoassays, etc., as they are non-toxic, biocompatible, and relatively easy to functionalize with polymers such as polyethylene glycol (PEG), polyvinyl pyrrolidine (PVP), or functional groups such as thiols, carboxyls and amines.21–28 For practical purposes, the nanoparticle (NP) surface must be functionalized to reduce agglomeration, improve biocompatibility, prevent protein absorption, reduce toxicity, and extend the NP time in blood circulation.4 Without any surface modification, these MNPs that have a hydrophobic surface and a high surface-to-volume ratio agglomerate to form large clusters17
This review highlights the advances in MNPs for various therapeutic applications. Initially, a short description of the various synthetic procedures for MNPs is provided. Next, information about recent advances in the use of MNPs for various therapeutic applications, including MHT, targeted drug delivery, PTT, PDT and magnetofection for gene delivery, is discussed in detail. Finally, the challenges, future outlook and conclusions regarding MNPs in therapeutic applications are described.
2. Synthesis of magnetic nanoparticles
The synthesis of MNPs deserves critical attention because of their colloidal nature and the multistep procedure required. Key demands in the synthesis of NPs include the experimental conditions, which define the size, shape and surface properties that can be reproduced without any complex purification method (such as magnetic filtration and size-exclusion chromatography).
Several chemical methods for MNP synthesis have been established, in which the most commonly used methods are co-precipitation, thermal decomposition and microemulsion-templated synthesis (Fig. 2). These methods lead to facile control over the size, shape and composition of MNPs, and are also effective and economical. On the other hand, some physical methods that lead to uncontrollable particle size include gas-phase decomposition and electron beam lithography. Whichever synthetic scheme is used, ideally, it should produce the highly crystalline nanoparticles with uniform dimensions and a high saturation magnetization. A summary of the various synthesis methods of MNP synthesis is also given in Table 1.
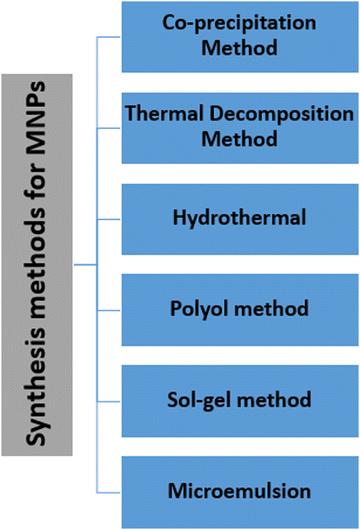 |
| Fig. 2 Various synthetic methods for nanoparticle synthesis. | |
Table 1 Summary of various synthesis methods for magnetic nanoparticles
Method |
Conditions |
Reaction temperature (°C) |
Solvent |
Advantages |
Disadvantages |
Ref. |
Co-precipitation |
Very simple, ambient condition |
25–85 |
Water |
Safe, cost-effective, requires low synthesis temperature. |
Surface oxidation, poor size distribution |
29–31
|
Thermal decomposition |
Inert atmosphere |
100–320 |
Organic compounds |
High-quality monodisperse particles, small size. |
Complicated, time-consuming, low production rate, demands protracted purification. |
33–41
|
Hydrothermal |
High pressure |
220 |
Water–ethanol |
Simple, eco-friendly, cost-effective, versatile. |
High pressure and temperature, longer synthesis time. |
42–45
|
Polyol |
Simple |
25 up to boiling point |
Ethylene, PEG |
Precise control over the size and shape, cost-effective, low-cost industrial method. |
Difficult to synthesise small particles. |
41,47,48
|
Sol–gel |
Ambient conditions |
10–30 |
Water |
High purity, homogeneity |
Impurities hard to remove. |
49,50
|
Microemulsion |
Ambient conditions |
20–50 |
Organic |
High magnetization value. |
Complicated, slow reaction kinetics. |
51–53
|
2.1 Co-precipitation method
In biomedical applications, co-precipitation is the most commonly used method because the particles produced are comparatively less harmful and easy to prepare.29 In addition, this method is safe, cost-effective and requires a low synthesis temperature compared with other conventional methods.30,31 As an example, a one-step co-precipitation reaction of ferrous (Fe2+) and ferric (Fe3+) salts in an alkaline solution is shown in Fig. 3.
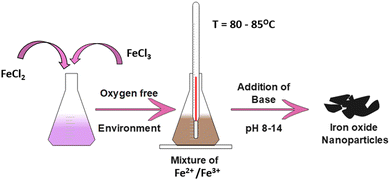 |
| Fig. 3 Steps to synthesize IONPs through the co-precipitation method. Fe2+ and Fe3+ are added in a 2 : 1 molar ratio in an oxygen-free environment and heated to 80–85 °C followed by the addition of base. The precipitated particles are then centrifuged or magnetically separated. | |
The general composition used for the method is a 2
:
1 ratio of Fe2+ and Fe3+ salts at either room temperature or an elevated temperature (80–85 °C). Upon completion of the reaction, a precipitate of Fe3O4 is formed at the bottom of the reactor, which can be recovered through centrifugation or magnetic isolation. The expected pH of the precipitate formed should be between 7.5 and 14. The general reaction can be given as:
Fe2+ + 2Fe3+ + 8OH− → Fe3O4 + 4H2O. |
If the molar ratio and pH of the precipitate are maintained properly then it may be oxidized to Fe(OH)3, which will drastically affect the physical and chemical properties of the NPs. The process can be described with the following reaction:
4Fe3O4 + O2 + 18H2O → 12Fe(OH)3. |
To prevent the imminent oxidation, inert or alkaline conditions are required during the synthesis of Fe3O4 nanoparticles. Moreover, during the precipitation process, the NPs are usually coated with organic or inorganic molecules such as detergents, proteins, starch, polyelectrolytes, etc. Other reaction parameters, such as the dropping speed of the basic solution and the stirring rate, also affect the quality of the nanoparticles. Despite the nanoparticles being produced having a polydisperse nature, the pH value of the reaction mixture needs to be regulated during both the synthesis and purification steps. In addition, nanoparticles have a high surface-to-volume ratio, and they tend to form aggregates in solution to reduce their surface energy.32 Thus, other methods have been developed with better surface characteristics and more uniform dimensions.
2.2 Thermal decomposition method
The thermal decomposition method, also known as pyrolysis, has been used to synthesise highly crystalline and monodisperse MNPs.33 It is carried out at high temperatures, commonly using organometallic precursors.1,34,35 To overcome the limitations of the co-precipitation method, this method was developed using non-aqueous solvents with a high boiling point, as depicted in Fig. 4.36 NPs produced using this method are reproducible, monodisperse and monocrystalline and generally of better quality.1 Any residual surfactants present may affect the efficiency of surface modification. Oleic acid, hexadecylamine and fatty acids are the most frequently used surfactants in this method. To attain a high degree of uniformity and a size of 4–30 nm, the optimum temperature should be within 100–350 °C.37,38
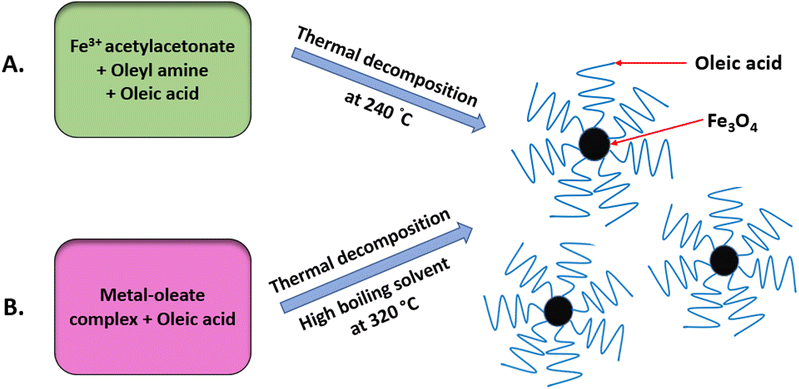 |
| Fig. 4 Widely used methods for the synthesis of monodisperse IONPs via thermal decomposition: (A) using an iron precursor in surfactants oleyl amine and oleic acid; and (B) using a metal oleate complex in oleic acid. | |
Thermal decomposition is advantageous for the MRI process since the nanoparticle size is a critical parameter and the size can easily be controlled using this method.39 Other advantages provided by this method include high crystallinity, dispersibility, uniformity and a fine particle size distribution.40 Despite having tremendous advantages, this method demands protracted purification steps before it can be used for biomedical applications.41 Furthermore, this method is time-consuming, has a low production rate, and requires costly and hazardous organic solvents.33
2.3 Hydrothermal method
The hydrothermal method is a commonly used method for preparing NPs in autoclaves or reactors over a wide range of temperatures and pressures, resulting in the fast nucleation and growth of newly synthesized MNPs.42 The particles produced by this method are pure with a controlled morphology.41 This method is considered to be eco-friendly, cost-effective and versatile as it does not use any organic solvent, thus eliminating the need for further treatment after synthesis.43,44 The main drawbacks of this method are that it fails to synthesize particles smaller than 10 nm, and at high temperatures it shows slow reaction kinetics. There are, however, some noteworthy advantages of the hydrothermal method over others, for example, it offers magnetic controllability, and a greater control over the size, shape and dispersion.45
2.4 Polyol method
The polyol method is used for synthesizing inorganic compounds from NPs to alloys, sulphides, oxides, fluorides, etc.46 In this method, the polyols act as a polar organic solvent for metal precursors, as a reducing agent, and in some cases as a complexation agent for metal cations. For the synthesis of metal and alloy nanoparticles, this method is versatile for controlling the size and shape of the particles. In addition, by varying the precursor concentration, the shape and size can be controlled, which can be a useful tool for biomedical applications.47 The particle size can be increased by increasing the reaction temperature.39 Moreover, particles of different morphologies, such as spheres, flowers, nanorods, etc., can be obtained using the polyol method.44 As this method does not require separate calcination and uses only polyol as the solvent, it is one of the easiest methods for synthesizing nanoparticles. Furthermore, polyols are considered to be green solvents and are cost-effective, and consequently are used extensively in industry.48
2.5 Sol–gel method
The sol–gel method uses condensation and hydrolysis reactions of metal alkoxides or their precursors to produce NPs. To secure NPs of high crystallinity, the intermediates need to be treated further.49 In this method, to prepare the sol the precursors are dissolved in water, followed by a series of stirring and heating steps; and for preparing the gel the MNPs are dried. The solvent is removed to achieve the desired MNP. Although the MNPs produced via the sol–gel method are of high purity and homogeneity, this method leads to the formation of impurities that are hard to remove. Like other methods, it provides a high level of control over the particle size and composition.50
2.6 Microemulsion method
Microemulsions are thermodynamically stable isotropic liquid mixtures of oil, water and surfactant.51 MNPs are produced through a series of emulsion steps and give high magnetization values. The MNPs synthesized via this method are larger in size. Moreover, the reaction kinetics are slow, even though the method requires a high temperature for synthesis.45,52 By controlling the water-to-surfactant ratio, concentration of the reactants, and surfactant film flexibility, one can control the size of the MNP microdroplets. The advantage of using the microemulsion method is that one can achieve precise control over the size, shape, and composition of the nanoparticles.53
2.7 Other methods
For synthesizing MNPs, other methods such as sonolysis, flow-injection techniques, electrochemical, aerosol/vapour methods, etc., have also been used.54 In sonolysis, by decomposing organometallic precursors, SPIONs can be produced at very high temperatures using ultrasound. Structural hosts, polymers, and organic capping agents are used to control the particle growth. In addition, these ultrasonic effects cause cavitation in the solution, which can thereby be used to modulate the nucleation, growth and formation of the MNPs.55 Sonolysis is a simple, green process that offers a narrow size distribution and diverse applications in biomedicine.56 Flow-injection techniques are carried out under a laminar flow system in a capillary reactor, which involves continuous or segmented mixing of the reagents. High reproducibility due to the plug-flow operation, high mixing homogeneity, accurate external control over the process and laminar conditions are a few benefits of this technique. The electrochemical method is an eco-friendly ‘green’ method that provides high selectivity at low cost, and includes diverse applications in biomedical and electronic fields.55,56 Under oxidizing conditions, aqueous solutions of dimethylformamide and cationic surfactants are used to synthesize <8 nm IONPs using iron electrodes by simply altering the current or applied potential. The disadvantages of this method include poor reproducibility and a high number of confounding factors. By contrast, the aerosol method gives a high-quality output, high purity and a comparatively straightforward process, although large aggregates are formed in this process, reducing the quality and leading to difficulty in scaling up.55,57
3. The basic magnetic properties of MNPs
Ferromagnetism and superparamagnetism are the two main types of magnetic behaviour displayed by MNPs, and they are governed primarily by the MNP size. Typically, bulk magnetic materials are ferromagnetic, where the magnetic moments remain aligned even after the withdrawal of the external magnetic field. Therefore, these ‘permanent magnets’ show high remanent magnetisation (i.e., net magnetisation at zero external magnetic fields) and coercivity (i.e., require a high magnetic field strength in the reverse direction to bring the net magnetisation to zero). Large, multidomain MNPs also display ferromagnetism; however, when their size is reduced further (approach single-domain dimensions), their coercivity increases, reaches a maximum, and then falls sharply to zero. At this stage, the magnetic behaviour converts to superparamagnetism, where the magnetic moments revert to the non-aligned (random) state from the aligned state once the external field is withdrawn. These ‘temporary magnets’, therefore, have negligible remanent magnetisation and coercivity. This size-dependent magnetic behaviour is depicted in Fig. 5.58
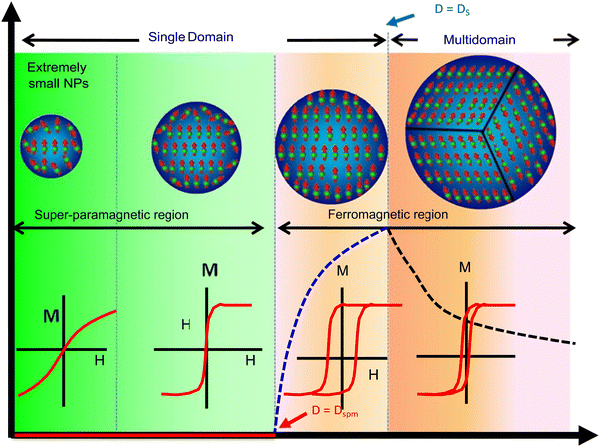 |
| Fig. 5 Size-dependent variation of magnetism in MNPs.58 Copyright 2018, Elsevier. | |
A Weiss domain or magnetic domain, which describes the volume of magnetic material where the magnetization is in a uniform direction, is used to differentiate between ferromagnetism and paramagnetism. The size-dependent variation can be determined by the domain structure of ferromagnetic material (also shown in Fig. 5). Frenkel and Dorfman first predicted that when the particle size is lower than a critical value (<15 nm), it becomes a single domain.59 In accordance with magnetic domain theory, the magnetic saturation, exchange forces, surface energy and the shape of particles are several factors that affect the critical size of a single domain.
While ferromagnetic nanoparticles are advantageous in certain applications, such as in magnetic data storage, superparamagnetic nanoparticles are highly preferred for biomedical applications due to their negligible remanent magnetisation, which enables exquisite control of their magnetic behaviour using an external magnetic field. For example, superparamagnetic iron oxide nanoparticles (SPIONs) have been used widely in all classes of biological applications.60 In general, MNPs with a size range of around 10–20 nm are usually preferred in most medical applications, not only because of their superparamagnetism but also due to their low toxicity and agglomeration, high circulation time, better pharmacokinetics/pharmacodynamics, ability to diffuse across tissues and biological barriers, and feasibility of targeting tumours via the enhanced permeability and retentivity (EPR) effect, among other factors.
4. Therapeutic applications of magnetic nanoparticles
The various types of therapeutic applications of magnetic nanoparticles are depicted in Fig. 6. We discuss these applications with an emphasis on recent advances.
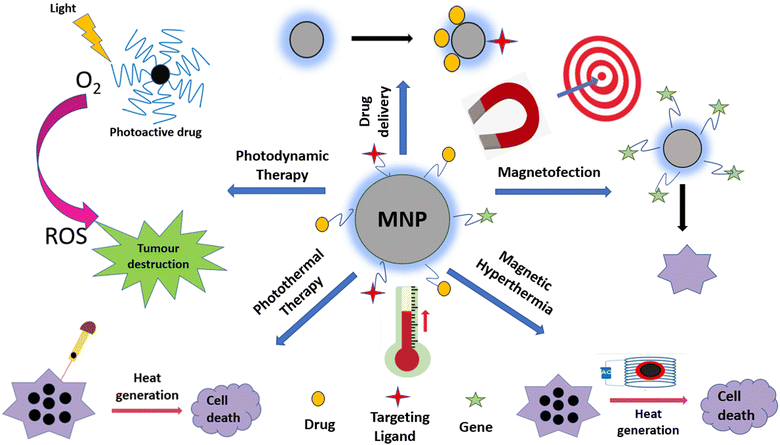 |
| Fig. 6 Various therapeutic applications of magnetic nanoparticles. | |
4.1 Magnetic hyperthermia therapy (MHT)
The use of MHT has been identified since ancient times for the treatment of malignant tumours. The father of medicine Hippocrates proposed that hot iron can be used to treat surface tumours. Later, unconventional methods like hot water baths, magnetic hyperthermia and high-frequency radiation were used for the thermal ablation of tumours.61 MHT uses MNPs to treat cancer through heat generation under the influence of an applied alternating (AC) magnetic field. It is a non-invasive method. The magnetic field can penetrate deep inside the body as it is not absorbed by living tissues. The working principle relies on increasing the temperature of the tumour to 41–47 °C to kill cancer cells, via either apoptosis or necrosis, as shown in Fig. 7.62,63
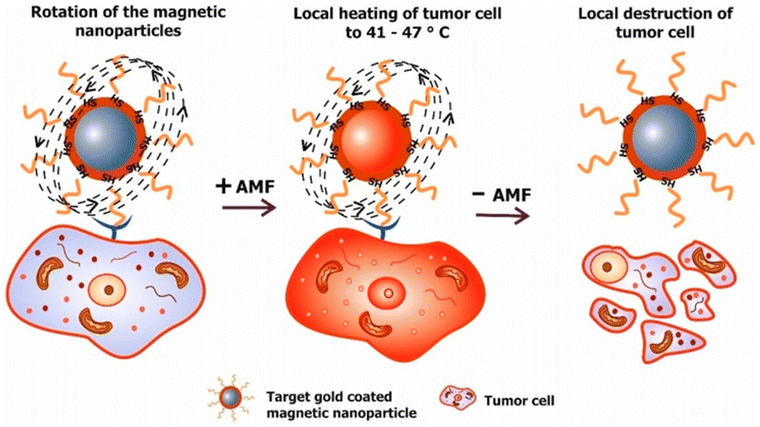 |
| Fig. 7 Working principle of magnetic hyperthermia. Targeted MNPs delivered to a tumour site are exposed to an external magnetic field. Afterward, the increase of the tumour temperature to 41–47 °C is responsible for cell death.63 Reprinted with permission under Creative Commons Attribution (CC BY). Copyright 2018, Multidisciplinary Digital Publishing Institute. | |
MNPs are first introduced inside the body by injecting a calculated amount of MNP solution, whereby they accumulate at the tumour site through passive or active targeting. MNPs are then subjected to an external magnetic field to produce heat that raises the temperature of cancerous cells more than that in non-cancerous cells.64 The benefit of MHT over chemotherapy is that it specifically targets the tumour and does not damage the surrounding healthy tissues, thereby having an increased efficiency over chemotherapy. In addition, cancerous cells have a higher sensitivity to temperature than healthy cells, which is referred to as ‘thermal ablation’. An increase in temperature during magnetic hyperthermia can be explained via Brownian relaxation (in which the entire NP domain rotates to reverse the direction of the magnetic moment), or Néel relaxation (which is the re-orientation of the MNP domain within the particle), during exposure to an alternating magnetic field (AMF).65 The AMF can penetrate deep inside the tissues, which enables the treatment of tumours at different positions within patients.
The transfer of magnetic energy to thermal energy can be measured as the specific absorption rate (SAR), which is given in watts g−1 by the following equation:66
where
C is the specific heat capacity of the solvent, d
T/d
t is the initial slope of the time-dependent heating curve,
ms is the mass of the solvent, and
mm is the mass of magnetic nanoparticles.
SAR is a crucial factor for the clinical application of MNPs and must be exploited because a higher SAR value requires only a small quantity of MNPs that must be injected inside the body of the patient, as well as a lower AMF exposure time.67 The size, shape and magnetic properties greatly influence the hyperthermia properties of MNPs.68 Normally, SPIONs are used for hyperthermia studies as they have a high magnetic saturation.69
For improving the SAR, significant research has been done for synthesising nanomaterials with a specific shape, size and surface modification. For effective treatment in a physiological environment, the MNPs must be stable at neutral pH (pH = 7). The stability of the MNPs is dictated by their size and surface charge density.70 The size-dependent properties of MNPs are responsible for the localised heating of cells.71 MNPs with a size larger than 200 nm are easily absorbed by the spleen and liver, whereas smaller MNPs with a size of around 10 nm are rapidly eliminated by renal clearance. This shows that the size of the MNPs plays an important role in their uptake by target cells and their removal from the body.72
The shape is another important parameter that influences the heating performance of MNPs. The cubic shape is considered the best for magnetic hyperthermia applications.73 The SAR values of iron oxide nanoparticles of different shapes follow the order SARnanocubes > SARnanoflowers > SARnano-octahedra > SARtruncated MNPs > SARnanorods.74
The surface coating can also affect the heating efficacy of MNPs. Due to the direct connectivity between the surface coating and a biological system, it is assumed that the surface-functionalisation properties will be more important than the core properties of MNPs. Without any surface modification, MNPs with a hydrophobic surface and a high surface-to-volume ratio agglomerate to form large clusters, resulting in a larger particle size.75 Therefore, it is important to coat MNPs with biocompatible materials. In addition, the thickness of the coating material can vary the heating rate.76
Manohar et al. prepared Ca-doped zinc ferrite NPs that can be used for the magnetic-hyperthermia treatment of cancer. Moreover, as the concentration of the dopant was increased, the SAR value decreased.77 Gawali et al. showed that biocompatible BSA-conjugated IONPs have a higher heating efficacy than glutaric acid-coated MNPs and show considerable uptake in cancer cells, which makes them efficient for magnetic hyperthermia.78 Using a modelling approach, Ali et al. showed that clustering can significantly decrease the thermal effects.55
Microwave-assisted nanocomposites of cubic SPION coated with PEG demonstrated heating efficiency for magnetic hyperthermia with a SAR value of 58.33 W g−1 in acidic solution. A toxicity assay revealed that after 48 hours the cell survival was 70%, which showed that the synthesized nanocomposite is a promising candidate for magnetic hyperthermia.79 Nanoplates of copper ferrite fabricated with aromatic polyamide chains showed a maximum SAR at 1 mg mL−1 at different magnetic field frequencies for a time interval of 5–20 min.80 Xing et al. reported that nanoparticles of iron carbide (Fe5C2) were used for multimodal hyperthermia treatment. Briefly, they showed via a comparative study of Fe5C2 nanoparticles with IONPs that Fe5C2 NPs have higher magnetization and SAR values than Fe3O4 nanoparticles at room temperature.81
Manganese-doped iron oxide showed promising results in both the MHT and photothermal therapy (PTT) of glioblastoma. A high SAR value of ∼600 W g−1 achieved effective hyperthermia therapy along with photothermal therapy, which demonstrated their efficacy in glioma cell death.82 Yang et al. synthesized Fe3O4 nanorods of different sizes (460, 350, and 250 nm) to study their effectiveness in magnetic hyperthermia using a mouse model. Among these three sizes, the 350 nm nanorods showed the highest SAR value of 1045 W g−1 at an Fe concentration of 0.2 mg mL−1, which demonstrated a satisfactory reduction in tumour volume with the mouse model, along with decent biocompatibility via MTT assay.83 Niclosamide is an FDA-approved drug that is used as an anticancer agent, which inhibits intercellular pathways when combined with hyperthermia to provide therapy against colorectal cancer cells; it can also be used to treat other cancer cells.84
Kaushik et al. reported a biosynthetic mechanism to produce MNPs that induce hyperthermia inside tumour cells. In short, they treated cancer cells with FeCl2 and zinc gluconate, which thereby increased the Fe and Zn content inside the cells, leading to the in situ formation of the MNPs with the help of intracellular reactive oxygen species (ROS). As cancer cells have a higher ROS level than non-cancerous cells, the synthesis of the particles did not occur in healthy cells due to the lower ROS levels. Their exposure to AMF for 30 min resulted in a temperature increase of 4–5 °C within the cellular environment. Therefore, this mechanism targets only cancerous cells, and once the biosynthesis of the particles is complete, the particles can be used to induce cell death using magnetic hyperthermia.85
After injection, the rapid clearance of MNPs by the liver, bone marrow, spleen and kidneys must be avoided. The size, shape and surface charge greatly influence their mode of clearance. MNPs greater than 100 nm are excreted very quickly from the body; therefore, smaller particles are preferred as they favour protein adhesion due to their high surface-to-volume ratio. Macrophages easily recognize these protein adhesions, however, and MNPs are cleared through the spleen and liver. The circulation rate, retention time and heating performance of MNPs are also affected by their surface charge: a high surface charge usually leads to the adsorption of plasma proteins, with subsequent sequestration by macrophages, thus reducing their circulation time in the blood.86
Combination therapies have gained much interest from researchers as they offer a broader spectrum of clinical applications. Curcio et al. prepared iron oxide nanoflowers with a copper sulfide shell for tri-modal cancer therapy (Fig. 8).
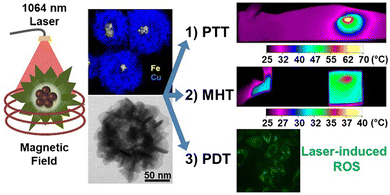 |
| Fig. 8 Nanoflowers of iron oxide with a copper sulfide shell that show MHT, PDT and PTT suitability.87 Reprinted with permission under Creative Commons Attribution (CC BY-NC). Copyright 2022, Ivyspring International Publisher. | |
Combining MHT, PDT and PTT indicates the use of NPs that show a response to light as well as to a magnetic field. Multi-therapeutic nanoparticles enable a lower dosage to be used for greater effectiveness, reduced exposure to laser power, and lower side effects, in contrast to conventional chemo- or radio-therapies used to treat cancer.87
4.2 Drug delivery
Another important therapeutic application of MNPs is as a carrier tool for targeted drug delivery. MNPs, using an external magnetic field, can be guided deep inside the tissue, which increases the bioavailability of the drug, and magnetic field control enables the precise targeting of the drug (Fig. 9). The main target of magnetically guided drug delivery is to deliver the drug directly to the diseased tissue, without having an impact on the rest of the body.14 A pharmaceutical drug coated on a biocompatible MNP carrier that is delivered under an applied magnetic field to the targeted organ is also known as a nanoparticulate drug-delivery system (nano-DDS). Such a nano-DDS not only enhances delivery and controls release of the drug at pathogenic sites but also conquers the multidrug resistance (MDR) effect of cancer cells.88,89 Targeted drug delivery overcomes the disadvantages of conventional therapies like chemotherapy and radiotherapy by providing precise targeting and enabling a reduced dosage, which thereby reduces the toxic side effects associated with it.
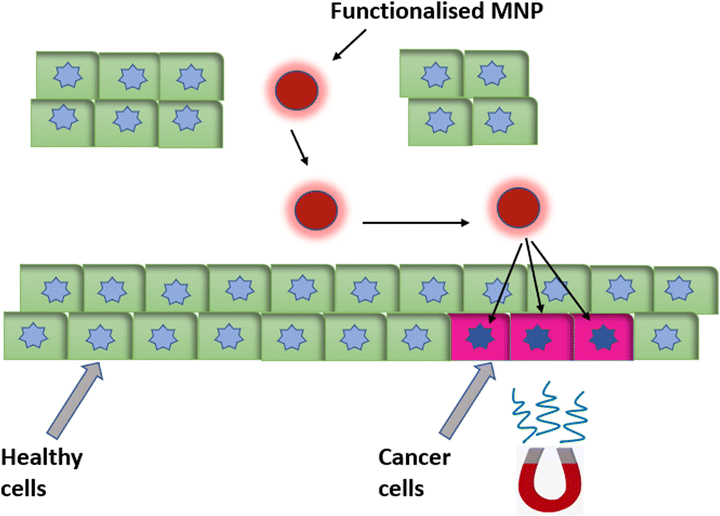 |
| Fig. 9 Magnetic nanoparticles functionalized with a targeting fluorophore, therapeutic drugs or targeting ligands, etc., for magnetically guided targeted drug delivery. | |
The rate of drug delivery at the site can be controlled via the intensity of the magnetic field. Numerous other factors, such as size, shape, and surface coating of the biocompatible nanoparticles, contribute to the effective use of MNPs for drug-delivery applications. The size of MNPs must be optimal so that they can easily enter into the bloodstream and escape from capture and elimination by the reticuloendothelial system (RES).90
The challenges associated with using MNPs as a drug carrier include drug-agglomeration, fast clearance by the RES, a low drug-carrying capacity, etc. However, these can be addressed by coating the MNPs with organic or inorganic multi-functional entities such as proteins, dendrimers, silica, etc.
Chitosan (CS)-coated IONPs conjugated with the anticancer drug telmisartan (TEL) were prepared for controlled and targeted drug delivery. Cytotoxicity studies were carried out on PC-3 human prostate cancer cells, which demonstrated a dose-dependent reduction of cell viability. Thus, in this study it was established that the MNP–CS–TEL nano-formulation showed potential as a nanocarrier for increasing the delivery of a poorly soluble drug (TEL) to cancer cells.91
4.3 Photothermal therapy (PTT)
PTT is another minimally invasive, localized therapy based on thermal energy, and has attracted the attention of researchers as an emerging tool for local cancer treatment. In PTT, malignant tissues loaded with nanoparticles are irradiated using a near-infrared (NIR) laser, leading to the generation of heat for the destruction of malignant tissues, as depicted in Fig. 10. This technique can be carried out using longer-wavelength light (700–1200 nm), which emits less energy and, hence, does not harm the surrounding non-malignant tissues.92,93
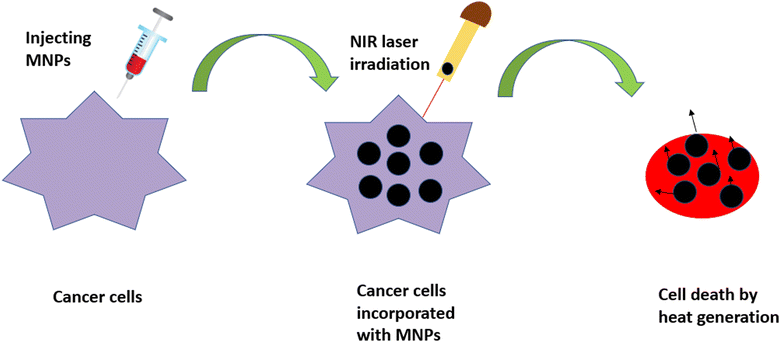 |
| Fig. 10 Schematic representation of cell death via PTT for the treatment of cancer. MNPs injected into the cancerous cells followed by NIR laser irradiation result in the generation of heat, which is responsible for the death of the cancer cells. | |
We can define a good photothermal agent as the one that has the ability to absorb NIR radiation, has high biocompatibility, low toxicity and a maximum light-to-heat conversion rate, i.e., a high absorption cross-section.94 Nowadays, gold-based compounds are the most commonly used photothermal agents, but their undefined toxicity is still the main cause of concern in the field of medicine. MNPs have become a viable alternative as photothermal probes for researchers because of their ability to combine with other photothermal agents, thus increasing the capacity of the therapeutic technique. Their downside of having a low molar absorption coefficient in the NIR region, however, can be resolved through controlled clustering.95
Several MNPs are also known to show optical absorption in the visible and NIR regions. It has been observed that when these MNPs are excited using visible or NIR light, localized heating (hyperthermia) is generated, which facilitates their use as agents for PTT. IONPs have higher biocompatibility, biodegradability and are relatively easy to functionalize, making them a promising candidate for PTT and other biomedical applications.96,97 Bu et al. demonstrated that IONPs coated on platelet-cancer stem cells can enhance the PTT of head and neck squamous cell carcinoma.98
There are various types of photothermal agents that comprise inorganic and organic materials, as shown in Fig. 11. Organic compounds, mainly NIR dyes with good photophysical properties and large-scale chemical production compared with inorganic materials, have become attractive candidates for PTT.99
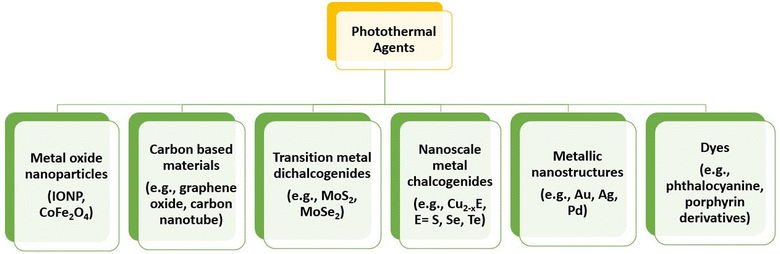 |
| Fig. 11 Various photothermal agents for photothermal therapy. | |
IONPs of various shapes (spherical, hexagonal and wire-like) irradiated using NIR laser light of wavelength 808 nm showed a strong photothermal effect. Using a human esophageal cancer model, Cu et al. demonstrated that spherical IONPs showed NIR-induced hyperthermia, which effectively inhibited the tumour growth compared with other IONP shapes. Thus, the photothermal effect of IONPs can be utilized in clinical cancer therapy applications.100
Recently, Deng et al. showed multifunctional magnetic nanoprobes for image-guided PTT. They demonstrated a seed-mediated growth method for the preparation of superparamagnetic Mn@Co MNPs, which were further functionalised with indocyanine green (photosensitiser) for MRI/NIR imaging and PTT. Using 808 nm laser irradiation in an MGC-803 tumour-bearing mouse model, these biocompatible nanoprobes exhibit good on-point targeting capability and photothermal therapeutic efficiency.101 Iron-based nanoparticles also show magnetothermal as well as photothermal effects when irradiated at 808 nm. Iron carbide (Fe5C2) nanoparticles were prepared using sol–gel and thermal decomposition methods. The carbon coating on Fe5C2 enhanced its optical absorption, which upon laser irradiation showed a rapid photothermal effect.81
Indocyanine green-loaded IONPs showed a synergistic effect in photothermal therapy and immunotherapy for ovarian cancer.102 Similar work was shown by Zhang et al., where indocyanine green (ICG)-loaded IONPs coated with polyphenols (MIRDs) were used for controlled cancer treatment via PTT/immunotherapy. After intravenous injection, the MIRDs showed a long blood-circulation time, magnetic targeting and acted as MRI guides. Using NIR irradiation, these MIRDs demonstrated potent tumour ablation, and further antigens associated with the tumour induced an immunological response to the body. As a result, the synergism of PTT with immunotherapy caused tumour ablation, suppressed metastasis and prevented tumour recurrence, with fewer side effects (Fig. 12).103
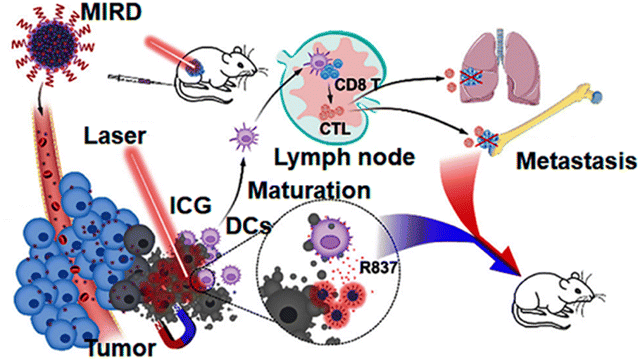 |
| Fig. 12 Schematic representation of MIRDs for cancer treatment using combined PTT/immunotherapy.103 Copyright 2020, Elsevier. | |
In 2020, a comparative study on iron oxide (magnetite and maghemite) nanospheres and nanoflowers for PTT and MHT was demonstrated by Cabana et al. in the second optical biological window (NIR-II). In comparison with nanospheres, nanoflowers are known to be better nano-heaters and are largely used in biomedical applications. For PTT, the heating measurements were recorded at 0.3 and 1 W cm−2, irradiated using a 1064 nm laser and MHT at 18 mT and a 470 kHz frequency (Fig. 13). For both therapies, magnetite nanoparticles were more effective, irrespective of their shape. Moreover, in cells, at a lower dosage of nanoparticles, PTT is much more effective than MHT. In addition, the cellular uptake shown by the magnetite nanoflowers was higher than that of nanospheres. During antitumour therapy, complete cell death was observed at a power density of 0.3 W cm−2.104
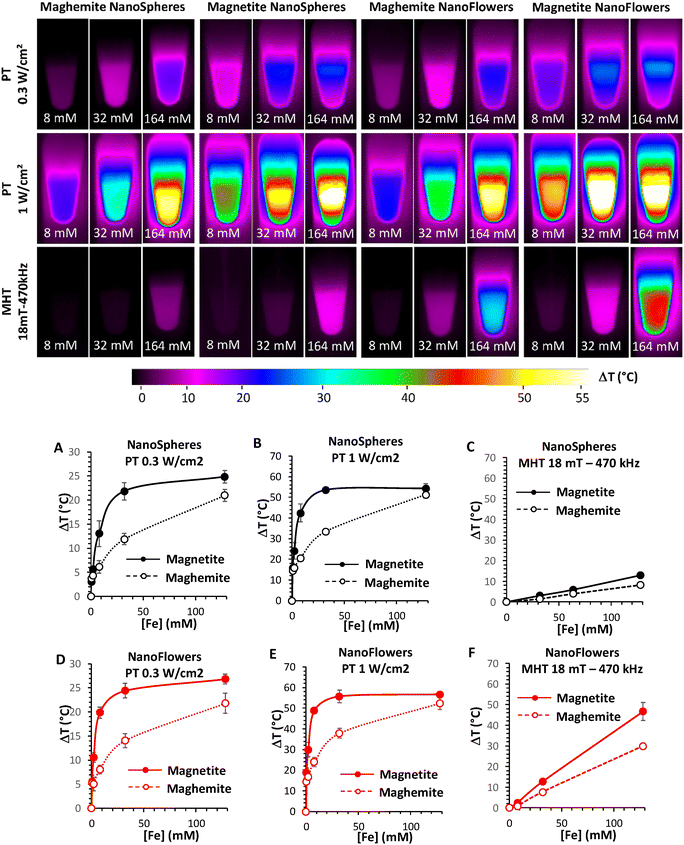 |
| Fig. 13 (Upper images) Infrared images of 50 μL of magnetite and maghemite nanospheres and nanoflowers at different concentrations (8, 32 and 164 mM) for 10 min of exposure to different stimuli (Photothermal, or PT, at laser powers of 0.3 and 1 W cm−2; and magnetothermal, or MHT, at magnetic fields of 18 mT-470 kHz). (Lower images) Elevated-temperature profiles of magnetite and maghemite nanospheres (Panels A, B and C) and nanoflowers (Panels D, E and F) for PTT (Panels A, B, D and E) and MHT (Panels C and F).104 Reprinted with permission under Creative Common Attribution 4.0 International License. Copyright 2020, Multidisciplinary Digital Publishing Institute. | |
4.4 Photodynamic therapy (PDT)
PDT is a form of drug delivery that has gained much interest over the past few years as a new therapeutic tool for the treatment of cancer.105 It is a light-activated therapy in which the drug/photosensitizer (PS) is targeted to the tumour. The photosensitizer is then irradiated with a specific wavelength at which the PS absorbs, resulting in the formation of cytotoxic reactive oxygen species that in turn are responsible for the death of cancer cells.
Due to its minimally invasive nature, low side effects, and high cure rates, it has become an alternative to conventional cancer treatments like chemotherapy and radiotherapy.106,107 Warrier et al. also stated that these properties of PDT can also be used to control microbes in biofilms that do not respond to conventional antibiotic therapies as well as for treating infections caused by bacteria. Photosensitizer molecules used can be naturally occurring compounds to increase the efficiency of PDT.108
The mechanism of PDT can be explained through a Jablonski diagram, which gives the electronic states and their transitions as the basic requirement of light-activated therapeutic reagents.109 When a PS is irradiated with light, it gets excited from the ground singlet state (So) to an excited singlet state (Sn). It may undergo internal conversion and relax to a lower energy level of (S1). The PS can gain energy in two different ways: [1] the molecule in the S1 state releases a photon of a higher wavelength than So; this process is called fluorescence; and [2] the molecule in the S1 state undergoes intersystem crossing, leading to the transition from S1 to the excited triplet state (T1).110,111 By releasing a photon, the molecule can relax from T1 to the So state. This process is known as phosphorescence. Moreover, the excited triplet state can form radicals for two different types of PDT.112,113
In Type I reactions, the PS reacts directly to endogenous substrates that have an electron/hydrogen atom which subsequently produces superoxide or reactive oxygen species that destroy the cancer cells via oxidation.114 A Type II process can also occur in which the T1 state directly transfers its energy to molecular oxygen (3O2) to generate highly cytotoxic singlet oxygen (1O2).115
In 2019, Amirshaghaghi et al. developed nanoclusters of chlorine e6 (Ce6)-coated SPIONs for dual-mode imaging and PDT. Briefly, the second-generation photosensitizer Ce6-coated SPIONs were synthesized via an oil-in-water emulsion system. The Ce6-SPOINs show high biocompatibility, solubility and significantly slowed down tumour growth in the murine tumour model due to the generation of ROS, making it a theranostic agent for clinical translation.116
The synergism of MHT with PDT demonstrated by Wang et al. showed an antitumor immune response when bullet-like organosilica-modified MNPs loaded with the photosensitizer Ce6 were used to treat breast cancer cells. Notably, these particles exhibited a high blood circulation time with homogenous targeting. The synthetic procedure with PDT and MHT application for an enhanced synergistic antitumor efficiency against tumour metastasis is shown in Fig. 14.117 In addition, Ce6 and folic acid (FA)-formulated IONPs can be used as a therapeutic tool in photodynamic therapy (PDT).118
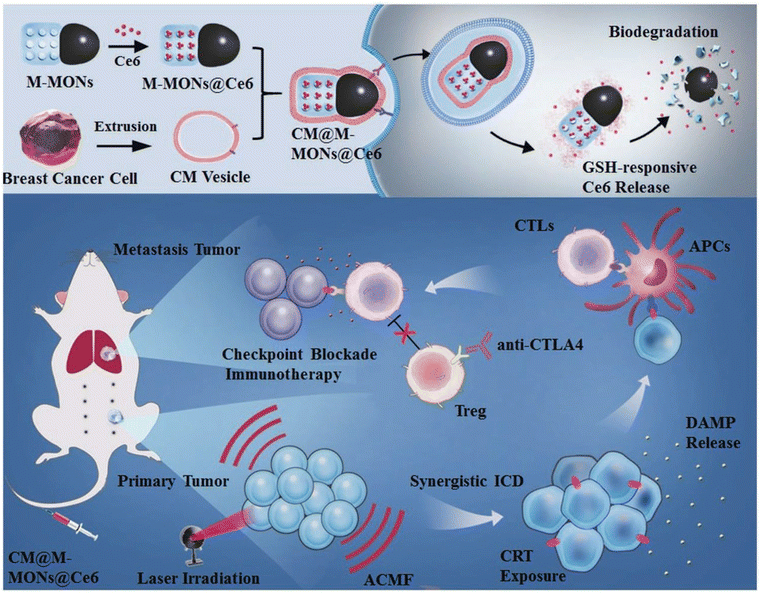 |
| Fig. 14 Schematic representation of the synthetic procedure of Ce6-loaded Janus magnetic mesoporous nanoparticles (CM@M-MON@Ce6) for cancer cell membrane targeting. Combined PDT and MHT are shown to enhance the synergistic antitumour efficiency in combating cancer treatment.117 Reprinted with permission under Creative Common Attribution 4.0 International License. Copyright 2019, Wiley and Co. | |
Recently, Yu and co-workers developed Ce6 loaded with PG functionalized IONPs linked with glucose, which can give both targeted photodynamic therapy and boosted immunogenicity of lung carcinoma.119 Combining two therapies in one nanoparticle will increase its efficacy and decrease the side effects associated with it. This synergistic effect has been shown by many researchers. Ngen et al. combined PDT with magnetic hyperthermia for the treatment of prostate cancer.120 Ashkbar et al. demonstrated an encouraging alternative to chemotherapy using PDT and PTT for the treatment of breast cancer. Briefly, iron oxide nanoparticles coated with silica and further mobilized with curcumin showed a 27% decrease in tumour volume when treated using PDT and PTT together.121 In another study, SPOIN loaded with the photosensitizer curcumin were used for PDT that was free of any organic reagents.122 Mn- and Cr-based photosensitizers were used for PDT and delivery of the drug curcumin, where it was found that the Mn-based nanocomposite loaded with curcumin was more suitable than the Cr-based nanocomposite nanocarrier as a drug-delivery system, as seen via its ability to reduce the cell viability to 4.6%.123
4.5 Magnetofection
Nanoparticle-mediated magnetofection is a transfection method used for delivering nucleic acid under the influence of an external magnetic field.124 The particles containing vectors are attached to MNPs via non-covalent bonds. Magnetofection is a novel and powerful technique, which increase the efficiency of delivering genetic material into cells.125–128 Using this approach, DNA/RNA/plasmids can be transfected to the targeted cells.129,130
In the cell culture medium, the external magnetic field moves nucleic acid-bound MNPs from the medium to the cell surface. Rapid sedimentation to the targeted area results in lowering the time and dosage of the vector with low cell cytotoxicity, thereby increasing the efficiency of transfection.131
Genes bound to MNPs are injected intravenously, and guided to the targeted cells/tissues using high-gradient magnets. At the target site, the functional genes are freed via enzymatic cleavage or polymer degradation, as shown in Fig. 15.132,133 Chemical reagents like peptides, polymers and liposomes have a low transfection efficacy and are non-toxic, whereas the in vitro and in vivo transfection of nucleic acids, such as plasmids, siRNA, shRNA and antisense oligonucleotides, has a high efficiency of transfection.130
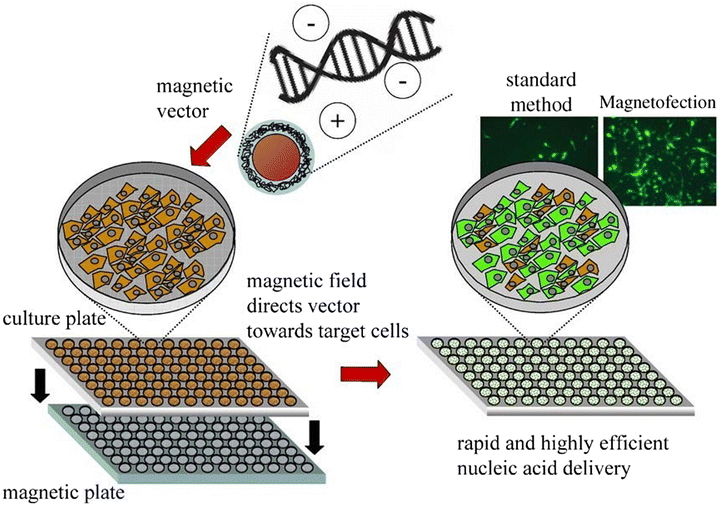 |
| Fig. 15 Various steps of magnetofection in cell culture.133 Copyright 2005, Elsevier. | |
Though appropriate surface modification, MNPs can act as efficient transfection agents. In addition, the drug/gene can be magnetically guided to the targeted site. In cancer gene therapy, MNPs@siRNA (silencing RNA) in a tumour-bearing mouse model does not show any adverse side-effects when delivered magnetically.134 In addition, this approach helped to cross the blood–brain barrier in brain gene delivery while other methods show inflammatory reactions with a low transfection rate.130 However, a key disadvantage associated with magnetofection is the agglomeration of MNPs, which can result following the removal of the external magnetic field.135
5. Challenges and future outlook
MNPs show significant potential in various therapeutic applications, particularly in magnetic field hyperthermia and drug targeting. Research in the biomedical field has improved the design and safety of MNPs. However, there are still some challenges, like agglomeration and low magnetization, that need to be addressed by researchers. For in vivo clinical applications, the biocompatibility and safety of nanoparticles is the most important aspect. Toxicity caused by MNPs depends on various factors such as the dosage, size, biodegradability, solubility, etc. Thus, the toxicity of MNPs needs accurate assessment.
Controlling the chemical composition, size, and shape of MNPs, which strongly affects their properties, is a big challenge for biomedical applications. The nature of the components that respond to a magnetic field, such as iron, cobalt, nickel, etc., and the size of NPs with their core and coating are responsible for the safety and biocompatibility of MNPs. Owing to their excellent biocompatibility, both magnetite (Fe3O4) and maghemite (γ-Fe2O3) show a wide range of biomedical applications, such as drug delivery, cancer treatment through magnetic hyperthermia, contrast enhancement in MRI, immunoassay, etc. By contrast, magnetic nanomaterials made up of cobalt, nickel, etc., despite being highly magnetic, have a poor safety profile and are highly susceptible to oxidation; hence, they have limited potential in biomedical applications.
The choice of synthesis protocol and particle functionalization is also important as it strongly influences the particle characteristics. Another important challenge associated with MNPs in therapy applications is site-specific delivery. Researchers used a permanent magnet to control the direction of the drug, which was not ideal as it has a very low tissue penetration. Initially, a small magnetic carrier was used to attract the MNPs. MHT is a promising application but cannot be used in the early stages of cancer as the tumour needs to be localized. In addition, MHT does not allow deep tissue penetration as the magnetic gradient is inversely proportional to the distance. Thus, MHT needs further advances.
The biggest reward of magnetic nanoparticles lies in combined therapy and diagnostics applications using a single nano-formulation. The see–treat–see action in the near future will be appealing as it will enable the real-time monitoring of theranostic agents that will be beneficial for the patient. The synthesis of multi-functional nanoparticles will be of great interest, as it will enable multi-modal therapy with lower or minimal side effects. In addition, using low-toxicity elements for the development of new-generation nanocarriers that have unique imaging and therapy applications will be attractive for researchers in the future. We can say that MNPs are likely to be the future in cancer theranostics, i.e., diagnosis with precise drug delivery. Moreover, the issues of toxicity will be resolved in the future to increase their efficacy. Therefore, more research is needed in this field to fully exploit the unique properties of MNPs in biomedical applications.
6. Conclusion
This Review aims to present a wide view of recent advances in the use of magnetic nanoparticles for therapy applications. We have provided accounts of various synthesis approaches to prepare magnetic nanoparticles. Advancements in the synthesis and formulation of monodisperse nanoparticles with controlled size, shape and magnetic properties help scientists to address and overcome difficulties associated with the use of magnetic nanoparticles for clinical applications. Magnetic hyperthermia gives the medical community an incredible tool to fight diseases such as cancer via apoptosis and necrosis. The properties of MNPs, like the high surface-to-volume ratio and magnetic saturation, make them an excellent candidate for targeted drug delivery. The challenges of low blood-circulation times, low targeting efficacies and premature drug release can be overcome using magnetic nanocomposites (magnetic hydrogels, mesoporous silica nanoparticles, magnetic liposomes, etc.), thus making them excellent choices for drug delivery. Light-activated photothermal and photodynamic therapies have low side effects and high cure rates, and thus they serve as an alternative to conventional cancer treatments like chemotherapy and radiotherapy. The synergistic effect of either magnetic hyperthermia with drug delivery or magnetic hyperthermia with photodynamic therapy has presented promising results in treating cancer. Providing two therapies in one carrier will significantly reduce the dosage and toxicity of NPs. Indeed, nanomaterials for biomedical research encompases a broad and comprehensive interdisciplinary research field that offers numerous opportunities to treat disease in a timely, effective, and risk-free manner.
Abbreviations
MNPs | Magnetic nanoparticles |
MRI | Magnetic resonance imaging |
PTT | Photothermal therapy |
PDT | Photodynamic therapy |
IONPs | Iron oxide nanoparticles |
FDA | US Food and drug administration |
SPIONs | Superparamagnetic iron oxide nanoparticles |
PEG | Polyethylene glycol |
PVP | Polyvinyl pyrrolidine |
SAR | Specific absorption rate |
ROS | Reactive oxygen species |
PS | Photosensitizer |
FA | Folic acid |
RES | Reticuloendothelial system |
Author contributions
Both authors contributed to the conceptualization, writing, and editing of the manuscript.
Conflicts of interest
There are no conflicts to declare.
Acknowledgements
The authors acknowledge funding support from the BRICS STI Framework Programme (Sanction order DST/IMRCD/BRICS/PilotCall2/TherNC/2018 (G)).
References
- S. Liu, B. Yu, S. Wang, Y. Shen and H. Cong, Preparation, surface functionalization and application of Fe3O4 magnetic nanoparticles, Adv. Colloid Interface Sci., 2020, 281, 102165 CrossRef CAS PubMed.
-
U. Häfeli, Magnetism in Medicine: A Handbook, WILEY-VCH, 2nd edn, 2007 Search PubMed.
- C. Shasha and K. M. Krishnan, Nonequilibrium Dynamics of Magnetic Nanoparticles with Applications in Biomedicine, Adv. Mater., 2021, 33, 1904131 CrossRef CAS PubMed.
- M. I. Anik, M. K. Hossain, I. Hossain, A. M. U. B. Mahfuz, M. T. Rahman and I. Ahmed, Recent progress of magnetic nanoparticles in biomedical applications: A review, Nano Select, 2021, 2, 1146 CrossRef CAS.
- A. Avasthi, C. Caro, E. P. Torres, M. P. Leal and M. L. A. Martin, Magnetic Nanoparticles as MRI Contrast Agents, Top. Curr. Chem., 2020, 378, 40 CrossRef CAS PubMed.
- C. Wilhelm and F. Gazeau, Universal cell labelling with anionic magnetic nanoparticles, Biomaterials, 2008, 29, 3161–3174 CrossRef CAS PubMed.
- C. Corot, P. Robert, J.-M. Idée and M. Port, Recent advances in iron oxide nanocrystal technology for medical imaging, Adv. Drug Delivery Rev., 2006, 58, 1471–1504 CrossRef CAS PubMed.
- F. Yang, Y. Li, Z. Chen, Y. Zhang, J. Wu and N. Gu, Superparamagnetic iron oxide nanoparticle-embedded encapsulated microbubbles as dual contrast agents of magnetic resonance and ultrasound imaging, Biomaterials, 2009, 30, 3882–3890 CrossRef CAS PubMed.
- N. Tran and T. J. Webster, Nanotechnology for bone materials, Wiley Interdiscip. Rev.: Nanomed. Nanobiotechnol., 2009, 1, 336–351 CAS.
- F. Daoyang, W. Qi, Z. Tengjiao, W. Hufei, L. Bingchuan, W. Yifan, L. Zhongjun, L. Xunyong, F. Dongwei and W. Xing, Recent Advances of Magnetic Nanomaterials in Bone Tissue Repair, Front. Chem., 2020, 8, 745 CrossRef PubMed.
- C. A. M. Iglesias, J. C. R. Araújo, J. de Xavier, R. L. Anders, J. M. de Araújo, R. B. da Silva, J. M. Soares, E. L. Brito, L. Streck, J. L. C. Fonseca, C. C. Plá Cid, M. Gamino, E. F. Silva, C. Chesman, M. A. Correa, S. N. de Medeiros and F. Bohn, Magnetic nanoparticles hyperthermia in a non-adiabatic and radiating process, Sci. Rep., 2021, 11, 11867 CrossRef CAS PubMed.
- T. Nann, Nanoparticles in Photodynamic Therapy, Nano Biomed. Eng., 2011, 3, 137–143 CAS.
- A. Farzin, S. A. Etesami, J. Quint, A. Memic and A. Tamayol, Magnetic Nanoparticles in Cancer Therapy and Diagnosis, Adv. Healthcare Mater., 2020, 9, 1901058 CrossRef CAS PubMed.
- K. Wu, D. Su, J. Liu, R. Saha and J. P. Wang, High-moment magnetic nanoparticles, Nanotechnology, 2020, 22, 66 Search PubMed.
- E. Alphandéry, Iron oxide nanoparticles for therapeutic applications, Drug Discovery Today, 2020, 25, 141 CrossRef PubMed.
- F. Soetaert, P. Korangath, D. Serantes, S. Fiering and R. Ivkov, Cancer therapy with iron oxide nanoparticles: Agents of thermal and immune therapies, Adv. Drug Delivery Rev., 2020, 163-164, 65–83 CrossRef CAS PubMed.
- D. D. Stueber, J. Villanova, I. Aponte, Z. Xiao and V. L. Colvin, Magnetic Nanoparticles in Biology and Medicine: Past, Present, and Future Trends, Pharmaceutics, 2021, 13, 943 CrossRef CAS PubMed.
- N. Tran and T. J. Webster, Magnetic nanoparticles: biomedical applications and challenges, J. Mater. Chem., 2010, 20, 8760–8767 RSC.
- V. Salgueirino-Maceira, M. A. Correa-Duarte, M. Farle, M. A. Lopez-Quintela, K. Sieradzki and R. Diaz, Synthesis and characterization of large colloidal cobalt particles, Langmuir, 2006, 22, 1455–1458 CrossRef CAS PubMed.
- L. S. Ganapathe, M. A. Mohamed, R. Y. Mohamad and D. D. Berhanuddin, Magnetite (Fe3O4) Nanoparticles in Biomedical Application: From Synthesis to Surface Functionalisation, Magnetochemistry, 2020, 6, 68 CrossRef CAS.
- M. Arruebo, R. Pacheco, M. R. Ibarra and J. Santamaría, Magnetic nanoparticles for drug delivery, Nano Today, 2007, 2, 22–32 CrossRef.
- C. Y. Haw, F. Mohamed, C. H. Chia, S. Radiman, S. Zakaria, N. M. Huang and H. N. Lim, Hydrothermal synthesis of magnetite nanoparticles as MRI contrast agents, Ceram. Interfaces, 2010, 36, 1417–1422 CrossRef CAS.
- L. Xiao, J. Li, D. F. Brougham, E. K. Fox, N. Feliu, A. Bushmelev, A. Schmidt, N. Mertens, F. Kiessling, M. Valldor, B. Fadeel and S. Mathur, Water-Soluble superparamagnetic magnetite nanoparticles with biocompatible coating for enhanced Magnetic Resonance Imaging, ACS Nano, 2011, 5, 6315–6324 CrossRef CAS PubMed.
- Y. V. Kolen’ko, M. B. López, C. R. Abreu, E. C. Argibay, A. Sailsman, Y. P. Redondo, M. F. Cerqueira, D. Y. Petrovykh, K. Kovnir, O. I. Lebedev and J. Rivas, Large-scale synthesis of colloidal Fe3O4 nanoparticles exhibiting high heating efficiency in Magnetic Hyperthermia, J. Phys. Chem. C, 2014, 118, 8691–8701 CrossRef.
- D. K. Chatterjee, P. Diagaradjane and S. Krishnan, Nanoparticle-mediated hyperthermia in cancer therapy, Ther. Delivery, 2011, 2, 1001–1014 CrossRef CAS.
- K. Sjaastad, M. W. Radomski, Y. Volkov, A. O. L. Gobbo and P. Mello, Magnetic nanoparticles in Cancer Theranostics, Theranostics, 2015, 5, 1249–1263 CrossRef PubMed.
- G. Zhang, Y. Liu, C. Zhang, W. Hu, W. Xu, Z. Li, S. Liang, J. Cao and Y. Wang, Aqueous immune magnetite nanoparticles for immunoassay, J. Nanopart. Res., 2009, 11, 441–448 CrossRef CAS.
- H. Shokrollahi, A review of the magnetic properties, synthesis methods and applications of maghemite, J. Magn. Magn. Mater., 2017, 426, 74–81 CrossRef CAS.
- H. Mohammadi, E. Nekobahr, J. Akhtari, M. Saeedi, J. Akbari and F. Fathi, Synthesis and characterization of magnetite nanoparticles by co-precipitation method coated with biocompatible compounds and evaluation of in-vitro cytotoxicity, Toxicol. Rep., 2021, 8, 331 CrossRef CAS.
- T. Ajeesha, A. Ashwini, M. George, A. Manikandan, J. A. Mary, Y. Slimani, M. A. Almessiere and A. Baykal, Nickel substituted MgFe2O4 nanoparticles via co-precipitation method for photocatalytic applications, Phys. B, 2021, 606, 412660 CrossRef CAS.
- S. Slimani, C. Meneghini, M. Abdolrahimi, A. Talone, J. P. M. Murillo, G. Barucca, N. Yaacoub, P. Imperatori, E. Illés, M. Smari, E. Dhahri and D. Peddis, Spinel Iron Oxide by the Co-Precipitation Method: Effect of the Reaction Atmosphere, Appl. Sci., 2021, 11, 5433 CrossRef CAS.
- N. D. Kandpal, N. Sah, R. Loshali, R. Joshi and J. Prasad, Co-precipitation method of synthesis and characterization of iron oxide nanoparticles, J. Sci. Ind. Res., 2014, 73, 87 CAS.
- A. G. Niculescu, C. Chircov and A. M. Grumezescu, Magnetite nanoparticles: Synthesis methods – A comparative review, Methods, 2021, 1046 Search PubMed.
- Q. Dong, Z. Meng, C. L. Ho, H. Guo, W. Yang, I. Manners, L. Xu and W. Y. Wong, A molecular approach to magnetic metallic nanostructures from metallopolymer precursors, Chem. Soc. Rev., 2018, 47, 4934–4953 RSC.
- Z. Wei, D. Wang, Y. Liu, X. Guo, Y. Zhu, Z. Meng, Z. Q. Yu and W. Y. Wong, Ferrocene-based hyperbranched polymers: a synthetic strategy for shape control and applications as electroactive materials and precursor-derived magnetic ceramics, J. Mater. Chem. C, 2020, 8, 10774–10780 RSC.
-
S. Rahim, F. J. Iftikhar and M. I. Malik, Biomedical applications of magnetic nanoparticles, Metal Nanoparticles for Drug Delivery and Diagnostic Applications, Elsevier, 2020, 301–328 Search PubMed.
- S. Belaïd, D. Stanicki, L. Vander Elst, R. N. Muller and S. Laurent, Fractal surface maghemite nanoparticles prepared by co-precipitation: the influence of Iron concentration and base nature, Nanotechnology, 2018, 29, 165603 CrossRef.
- N. Jović Orsini, B. Babić-Stojić, V. Spasojević, M. P. Calatayud, N. Cvjetićanin and G. F. Goya, Magnetic and power absorption measurements on iron oxide nanoparticles synthesized by thermal decomposition of Fe(acac)3, J. Magn. Magn. Mater., 2018, 449, 286 CrossRef.
- P. Tartaj, T. G. Carreño and C. J. Serna, Magnetic Behavior of γ-Fe2O3 Nanocrystals Dispersed in Colloidal Silica Particles, The, J. Phys. Chem. B, 2003, 107, 20 CrossRef CAS.
- P. Y. R. Rodríguez, D. A. C. Hernández, C. A. A. Orta, J. Sánchez, M. A. Guel, A. H. Guerrero, C. C. Alvarado and V. H. R. Martínez, Synthesis of Pluronic F127-coated magnesium/calcium (Mg1-xCaxFe2O4) magnetic nanoparticles for biomedical applications, J. Magn. Magn. Mater., 2021, 521, 167518 CrossRef.
- P. Cheah, J. Qu, Y. Li, D. Cao, X. Zhu and Y. Zhao, The key role of reaction temperature on a polyol synthesis of water-dispersible iron oxide nanoparticles, J. Magn. Magn. Mater., 2021, 540, 168481 CrossRef CAS.
- Y. X. Gan, A. H. Jayatissa, Z. Yu, X. Chen and M. Li, Hydrothermal Synthesis of Nanomaterials, J. Nanomater., 2020, 2020, 3 Search PubMed.
- A. Abbasi, H. Khojasteh, A. H. Keihan, K. Adib, A. S. Nasab and M. R. Nasrabadi, Co-precipitation synthesis of Ag-doped NiCr2O4 nanoparticles: investigation of structural, optical, magnetic, and photocatalytic properties, J. Mater. Sci.: Mater. Electron., 2021, 32, 1413 CrossRef CAS.
- M. Adibi, S. M. Mirkazemi and S. Alamolhoda, The influence of citric acid on the microstructure and magnetic properties of cobalt ferrite nanoparticles synthesized by hydrothermal method, Appl. Phys. A: Mater. Sci. Process., 2021, 127, 497 CrossRef CAS.
- S. Ansari, E. Ficiarà, F. Ruffinatti, I. Stura, M. Argenziano, O. Abollino, R. Cavalli, C. Guiot and F. D’Agata, Characterization and Functionalization for Biomedical Applications in the Central Nervous System, Materials, 2019, 12, 465 CrossRef CAS PubMed.
-
P. Ruz and V. Sudarsan, Polyol Method for Synthesis of Nanomaterials, Handbook on Synthesis Strategies for Advanced Materials, Indian Institute of Metals Series, Springer; Singapore, 2021, 293 Search PubMed.
- S. Ammar and F. Fiévet, Polyol Synthesis: A Versatile Wet-Chemistry Route for the Design and Production of Functional Inorganic Nanoparticles, Nanomaterials, 2020, 10, 1217 CrossRef CAS.
- A. H. Oh, H. Y. Park, Y. G. Jung, S. C. Choi and G. S. An, Synthesis of Fe3O4 nanoparticles of various size via the polyol method, Ceram. Int., 2020, 46, 10723 CrossRef CAS.
- P. Y. R. Rodríguez, C. A. A. Orta, M. A. Guel, D. A. C. Hernández, A. H. Guerrero, C. C. Alvarado, J. S. Fuentes, V. H. R. Martínez, J. A. V. Garza and G. F. H. López, Synthesis and characterization of magnetic nanoparticles Zn1-xMgxFe2O4 with partial substitution of Mg2+ (x = 0.0, 0.25, 0.5, 0.75 and 1.0) for adsorption of uremic toxins, Ceram. Int., 2020, 46, 27913 CrossRef.
- J. C. R. Araújo, S. A. Barbosa, A. L. R. Souza, C. A. M. Iglesias, J. Xavier, P. B. Souza, C. C. P. Cid, S. Azevedo, R. B. D. Silva, M. A. Correa, S. N. D. Medeiros, E. F. Silva and F. Bohn, Tuning structural, magnetic, electrical, and dielectric properties of MgFe2O4 synthesized by sol-gel followed by heat treatment, J. Phys. Chem. Solids, 2021, 154, 110051 CrossRef.
- A. A. Adewunmi, M. S. Kamal and T. I. Solling, Application of magnetic nanoparticles in demulsification: A review on synthesis, performance, recyclability, and challenges, J. Pet. Sci. Eng., 2021, 196, 107680 CrossRef CAS.
- R. López, M. Pineda, G. Hurtado, R. León, S. Fernández, H. Saade and D. Bueno, Chitosan-Coated Magnetic Nanoparticles Prepared in One Step by Reverse Microemulsion Precipitation, Int. J. Mol. Sci., 2013, 14, 19636 CrossRef PubMed.
- M. Salvador, G. Gutiérrez, S. Noriega, A. Moyano, M. C. B. López and M. Matos, Microemulsion Synthesis of Superparamagnetic Nanoparticles for Bio-applications, Int. J. Mol. Sci., 2021, 22, 427 CrossRef CAS.
- S. Majidi, F. Z. Sehrig, S. M. Farkhani, M. S. Goloujeh and A. Akbarzadeh, Current methods for synthesis of magnetic nanoparticles, Artif. Cells, Nanomed., Biotechnol., 2016, 44, 722 CrossRef CAS PubMed.
- A. Ali, H. Zafar, M. Zia, I. U. Haq, A. R. Phull, J. S. Ali and A. Hussain, Synthesis, characterization, applications, and challenges of iron oxide nanoparticles, Nanotechnol., Sci. Appl., 2016, 9, 49 CrossRef CAS PubMed.
-
A. B. Patil and B. M. Bhanage, in Handbook of Nanoparticles, Cham; Springer International Publishing, 2016, 143–166 Search PubMed.
- B. Buesser and S. E. Pratsinis, Design of Nanomaterial Synthesis by Aerosol Processes, Annu. Rev. Chem. Biomol. Eng., 2012, 3, 103 CrossRef CAS.
-
J. Mohapatra and J. P. Liu, Rare-Earth-Free Permanent Magnets: The Past and Future, Handbook of Magnetic Materials, Elsevier, 2018, 27, 1–57 Search PubMed.
- S. G. Grancharov, H. Zeng, S. H. Sun, S. X. Wang, S. O’Brien, C. B. Murray, J. R. Kirtley and G. A. Held, Biofunctionalization of monodisperse magnetic nanoparticles and their use as biomolecular labels in a magnetic tunnel junction based sensor, J. Phys. Chem. B, 2005, 109, 13030–13035 CrossRef CAS PubMed.
- A. Akbarzadeh, M. Samiei and S. Davaran, Magnetic nanoparticles: preparation, physical properties, and applications in biomedicine, Nanoscale Res. Lett., 2012, 7, 144 CrossRef PubMed.
- O. S. Nielsen, M. Horsman and J. Overgaard, A future for hyperthermia in cancer treatment, Eur. J. Cancer, 2001, 37, 1587–1589 CrossRef CAS PubMed.
- L. P. Mona, P. Lijo, S. P. Songca and A. P. Ajibade, Synthesis and encapsulation of iron oxide nanorods for application in magnetic hyperthermia and photothermal therapy, Nanotechnol. Rev., 2022, 11, 176–190 CrossRef CAS.
- I. Belyanina, O. Kolovskaya, S. Zamay, A. Gargaun, T. Zamay and A. Kichkailo, Targeted Magnetic Nanotheranostics of Cancer, Molecules, 2017, 22, 975 CrossRef PubMed.
- M. Peiravi, H. Eslami, M. Ansari and H. Z. Zardini, Magnetic hyperthermia: Potentials and limitations, J. Indian Chem. Soc., 2022, 99, 100269 CrossRef CAS.
- A. F. Abu-Bakr and A. Y. Zubarev, Effect of ring-shaped clusters on magnetic hyperthermia: modelling approach, Philos. Trans. R. Soc., A, 2021, 379, 2205 CrossRef.
- M. I. Anik, M. K. Hossain, I. Hossain, A. M. U. B. Mahfuz, M. T. Rahman and I. Ahmed, Recent progress of magnetic nanoparticles in biomedical applications: A review, Nano Select, 2021, 2, 1146 CrossRef CAS.
- X. Zuo, H. Ding, J. Zhang, T. Fang and D. Zhang, Carbothermal treated iron oxide nanoparticles with improving magnetic heating efficiency for hyperthermia, Results Phys., 2022, 32, 105095 CrossRef.
- S. L. Gawali, S. B. Shelar, J. Gupta, K. C. Barick and P. A. Hassan, Immobilization of protein on Fe3O4 nanoparticles for magnetic hyperthermia application, Int. J. Biol. Macromol., 2021, 166, 851 CrossRef CAS PubMed.
- A. Alkhayal, A. Fathima, A. H. Alhasan and E. H. Alsharaeh, PEG Coated Fe3O4/RGO Nano-Cube-Like Structures for Cancer Therapy via Magnetic Hyperthermia, Nanomaterials, 2021, 11, 2398 CrossRef CAS PubMed.
- C. Kittel, Theory of the structure of ferromagnetic domains in films and small particles, Phys. Rev., 1946, 70, 965 CrossRef CAS.
- A. H. Lu, E. E. L. Salabas and F. Schüth, Magnetic nanoparticles: Synthesis, protection, functionalization, and application, Angew. Chem., Int. Ed., 2007, 46, 1222 CrossRef CAS PubMed.
- P. Pradhan, J. Giri, G. Samanta, H. D. Sarma, K. P. Mishra, J. Bellare, R. Banerjee and D. Bahadur, Comparative evaluation of heating ability and biocompatibility of different ferrite-based magnetic fluids for hyperthermia application, J. Biomed. Mater. Res., Part B, 2007, 81, 12 CrossRef PubMed.
- O. S. Sánchez, T. Castelo-Grande, P. A. Augusto, J. M. Compaña and D. Barbosa, Cubic Nanoparticles for Magnetic Hyperthermia: Process Optimization and Potential Industrial Implementation, Nanomaterials, 2021, 11, 1652 CrossRef.
- H. Gavilán, S. K. Avugadda, T. Fernández-Cabada, N. Soni, M. Cassani, B. T. Mai, R. Chantrell and T. Pellegrino, Magnetic nanoparticles and clusters for magnetic hyperthermia: optimizing their heat performance and developing combinatorial therapies to tackle cancer, Chem. Soc. Rev., 2021, 50, 11614 RSC.
- A. Rezanezhad, A. Hajalilou, F. Eslami, E. Parvini, E. Abouzari-Lotf and B. Aslibeiki, Superparamagnetic magnetite nanoparticles for cancer cells treatment via magnetic hyperthermia: effect of natural capping agent, particle size and concentration, J. Mater. Sci.: Mater. Electron., 2021, 32, 24026 CrossRef CAS.
- X. L. Liu, H. M. Fan, J. B. Yi, Y. Yang, E. S. G. Choo, J. M. Xue, D. D. Fan and J. Ding, Optimization of surface coating on Fe3O4 nanoparticles for high performance magnetic hyperthermia agents, J. Mater. Chem., 2012, 22, 8235 RSC.
- A. Manohar, V. Vijayakanth and K. H. Kim, Influence of Ca doping on ZnFe2O4 nanoparticles magnetic hyperthermia and cytotoxicity study, J. Alloys Compd., 2021, 886, 161276 CrossRef CAS.
- S. L. Gawali, S. B. Shelar, J. Gupta, K. C. Barick and P. A. Hassan, Immobilization of protein on Fe3O4 nanoparticles for magnetic hyperthermia application, Int. J. Biol. Macromol., 2021, 166, 851 CrossRef CAS PubMed.
- A. Alkhayal, A. Fathima, A. H. Alhasan and E. H. Alsharaeh, PEG Coated Fe3O4/RGO Nano-Cube-Like Structures for Cancer Therapy via Magnetic Hyperthermia, Nanomaterials, 2021, 11, 2398 CrossRef CAS PubMed.
- R. Eivazzadeh-Keihan, S. Asgharnasl, M. S. Bani, F. Radinekiyan, A. Maleki, M. Mahdavi, P. Babaniamansour, H. Bahreinizad, A. E. Shalan and S. Lanceros-Méndez, Magnetic Copper Ferrite Nanoparticles Functionalized by Aromatic Polyamide Chains for Hyperthermia Applications, Langmuir, 2021, 37, 8847 CrossRef CAS.
- M. Xing, J. Mohapatra, J. Beatty, J. Elkins, N. K. Pandey, A. Chalise, W. Chen, M. Jin and J. Ping Liu, Iron-based magnetic nanoparticles for multimodal hyperthermia heating, J. Alloys Compd., 2021, 871, 159475 CrossRef CAS.
- R. Gupta and D. Sharma, Manganese-Doped Magnetic Nanoclusters for Hyperthermia and Photothermal Glioblastoma Therapy, ACS Appl. Nano Mater., 2020, 3, 2026 CrossRef CAS.
- Y. Yang, M. Huang, J. Qian, D. Gao and X. Liang, Tunable Fe3O4 Nanorods for Enhanced Magnetic Hyperthermia Performance, Sci. Rep., 2020, 10, 8331 CrossRef CAS PubMed.
- A. Ahmad, A. Gupta, M. M. Ansari, A. Vyawahare, G. Jayamurugan and R. Khan, Hyperbranched Polymer-Functionalized Magnetic Nanoparticle-Mediated Hyperthermia and Niclosamide Bimodal Therapy of Colorectal Cancer Cells, ACS Biomater. Sci. Eng., 2020, 6, 1102–1111 CrossRef CAS PubMed.
- S. Kaushik, J. Thomas, V. Panwar, H. Ali, V. Chopra, A. Sharma, R. Tomar and D. Ghosh, In Situ Biosynthesized Superparamagnetic Iron Oxide Nanoparticles (SPIONS) Induce Efficient Hyperthermia in Cancer Cells, ACS Appl. Bio Mater., 2020, 3, 779 CrossRef CAS PubMed.
- A. Lesniak, A. Salvati, M. J. Santos-Martinez, M. W. Radomski, K. A. Dawson and C. Åberg, Nanoparticle adhesion to the cell membrane and its effect on nanoparticle uptake efficiency, J. Am. Chem. Soc., 2013, 135, 1438–1444 CrossRef CAS PubMed.
- A. Curcio, A. K. A. Silva, S. Cabana, A. Espinosa, B. Baptiste, N. Menguy, C. Wilhelm and A. Abou-Hassan, Iron Oxide Nanoflowers @ CuS Hybrids for Cancer Tri-Therapy: Interplay of Photothermal Therapy, Magnetic Hyperthermia and Photodynamic Therapy, Theranostics, 2019, 9, 1288–1302 CrossRef CAS.
- G. Chen, I. Roy, C. Yang and P. N. Prasad, Nanochemistry and Nanomedicine for Nanoparticle-based Diagnostics and Therapy, Chem. Rev., 2016, 116, 2826–2885 CrossRef CAS PubMed.
- M. Ansari, A. Bigham, S. A. Hassanzadeh-Tabrizi and H. A. Ahangar, Synthesis and characterization of Cu0.3Zn0.5Mg0.2Fe2O4 nanoparticles as a magnetic drug delivery system, J. Magn. Magn. Mater., 2017, 439, 67–75 CrossRef CAS.
- R. N. Li, X. H. Da, X. Li, Y. S. Lu, F. F. Gu and Y. Liu, Functionalized magnetic nanoparticles for drug delivery in tumor therapy, Chin. Phys. B, 2021, 30, 017502 CrossRef CAS.
- R. P. Dhavale, R. P. Dhavale, S. C. Sahoo, P. Kollu, S. U. Jadhav, P. S. Patil, T. D. Dongale, A. D. Chougale and P. B. Patil, Chitosan coated magnetic nanoparticles as carriers of anticancer drug Telmisartan: pH-responsive controlled drug release and cytotoxicity studies, J. Phys. Chem. Solids, 2021, 148, 109749 CrossRef CAS.
- A. Doughty, A. R. Hoover, E. Layton, C. K. Murray, E. W. Howard and W. R. Chen, Nanomaterial applications in photothermal therapy for cancer, Materials, 2019, 12, 779 CrossRef CAS PubMed.
- H. Maeda, J. Wu, T. Sawa, Y. Matsumura and K. Hori, Tumor vascular permeability and the EPR effect in macromolecular therapeutics: a review, J. Controlled Release, 2000, 65, 271 CrossRef CAS.
- M. Vert, Y. Doi, K. H. Hellwich, M. Hess, P. Hodge, P. Kubisa, M. Rinaudo and F. Schue, Terminology for biorelated polymers and applications (IUPAC recommendations 2012), Pure Appl. Chem., 2012, 84, 377 CrossRef CAS.
- J. R. Melamed, R. S. Edelstein and E. S. Day, Elucidating the fundamental mechanisms of cell death triggered by photothermal therapy, ACS Nano, 2015, 9, 6–11 CrossRef CAS PubMed.
- J. Estelrich and M. A. Busquets, Iron Oxide Nanoparticles in Photothermal Therapy, Molecules, 2018, 23, 1567 CrossRef PubMed.
- R. A. Revia and M. Zhang, Magnetite nanoparticles for cancer diagnosis, treatment, and treatment monitoring: recent advances, Mater. Today, 2016, 19, 157–168 CrossRef CAS PubMed.
- L. L. Bu, L. Rao, G. T. Yu, L. Chen, W. W. Deng, J. F. Liu, H. Wu, Q. F. Meng, S. S. Guo, X. Z. Zhao, W. F. Zhang, G. J. Chen, Z. Gu, W. Liu and Z. J. Sun, Cancer Stem Cell-Platelets Hybrid Membrane Coated Magnetic Nanoparticles for enhanced Photothermal therapy of head and neck squamous cell carcinoma, Adv. Funct. Mater., 2019, 29, 1807733 CrossRef.
- S. Luo, E. Zhang, Y. Su, T. Cheng and C. Shi, A review of NIR dyes in cancer targeting and imaging, Biomaterials, 2011, 32, 7127–7138 CrossRef CAS.
- M. Chu, Y. Shao, J. Peng, X. Dai, H. Li, Q. Wu and D. Shi, Near-infrared laser light mediated cancer therapy by photothermal effect of Fe3O4 magnetic nanoparticles, Biomaterials, 2013, 34, 4078–4088 CrossRef CAS.
- Z. Deng, G. Qiao, L. Ma, Q. Zhang, P. Zhang and D. Cui, Photosensitizer-Functionalized Mn@Co Magnetic Nanoparticles for MRI/NIR-Mediated Photothermal Therapy of Gastric Cancer, ACS Appl. Nano Mater., 2021, 4, 13523–13533 CrossRef CAS.
- J. Xiong, M. Wu, J. Chen, Y. Liu, Y. Chen, G. Fan, Y. Liu, J. Cheng, Z. Wang, S. Wang, Y. Liu and W. Zhang, Cancer-Erythrocyte Hybrid Membrane-Camouflaged Magnetic Nanoparticles with Enhanced Photothermal-Immunotherapy for Ovarian Cancer, ACS Nano, 2021, 15, 19756–19770 CrossRef CAS PubMed.
- F. Zhang, G. Lu, X. Wen, F. Li, X. Ji, Q. Li, M. Wu, Q. Cheng, Y. Yu, J. Tang and L. Mei, Magnetic nanoparticles coated with polyphenols for spatio-temporally controlled cancer photothermal/immunotherapy, J. Controlled Release, 2020, 326, 131–139 CrossRef CAS PubMed.
- S. Cabana, A. Curcio, A. Michel, C. Wilhelm and A. Abou-Hassan, Iron Oxide Mediated Photothermal Therapy in the Second Biological Window: A Comparative Study between Magnetite/Maghemite Nanospheres and Nanoflowers, Nanomaterials, 2020, 10, 1548 CrossRef CAS PubMed.
- X. Wang, D. Luo and J. P. Basilion, Photodynamic Therapy: Targeting Cancer Biomarkers for the Treatment of Cancers, Cancers, 2021, 13, 2992 CrossRef CAS.
- H. Montaseri, C. A. Kruger and H. Abrahamse, Inorganic Nanoparticles Applied for Active Targeted Photodynamic Therapy of Breast Cancer, Pharmaceutics, 2021, 13, 296 CrossRef CAS PubMed.
- T. C. Pham, V. N. Nguyen, Y. Choi, S. Lee and J. Yoon, Recent Strategies to Develop Innovative Photosensitizers for Enhanced Photodynamic Therapy, Chem. Rev., 2021, 121, 13454–13619 CrossRef CAS PubMed.
-
A. Warrier, N. Mazumder, S. Prabhu, K. Satyamoorthy and T. S. Murali, Photodynamic therapy to control microbial biofilms, Photodiagnosis and Photodynamic Therapy, Elsevier, 2021, 33, 102090 Search PubMed.
- B. S. Dash, S. Das and J. P. Chen, Photosensitizer-Functionalized Nanocomposites for Light-Activated Cancer Theranostics, Int. J. Mol. Sci., 2021, 22, 6658 CrossRef CAS PubMed.
- J. Jun, D. Chenoweth and E. Petersson, Rational Design of Small Molecule Fluorescent Probes for Biological Applications, Org. Biomol. Chem., 2020, 18, 5747–5763 RSC.
- G. Feng, G. Q. Zhang and D. Ding, Design of Superior Phototheranostic Agents Guided by Jablonski Diagrams, Chem. Soc. Rev., 2020, 49, 8179–8234 RSC.
- B. Zhao and Y. He, Recent Advances in the Prevention and Treatment of Skin Cancer Using Photodynamic Therapy, Expert Rev. Anticancer Ther., 2010, 10, 1797–1809 CrossRef CAS PubMed.
- T. D. Martins, E. Lima, R. E. Boto, D. Ferreira, J. R. Fernandes, P. Almeida, L. F. E. Ferreira, A. M. Silva and L. V. Reis, Red and Near-Infrared Absorbing Dicyanomethylene Squaraine Cyanine Dyes: Photophysicochemical Properties and Anti-Tumor Photosensitizing Effects, Materials, 2020, 13, 2083 CrossRef CAS.
- J. Zhang, C. Jiang, J. P. F. Longo, R. B. Azevedo, H. Zhang and L. A. Muehlmann, An Updated Overview on the Development of New Photosensitizers for Anticancer Photodynamic Therapy, Acta Pharm. Sin. B, 2018, 8, 137–146 CrossRef PubMed.
- A. Castano, T. Demidova and M. Hamblin, Mechanisms in Photodynamic Therapy: Part Two-Cellular Signaling, Cell Metabolism and Modes of Cell Death, Photodiagn. Photodyn. Ther., 2005, 2, 1–23 CrossRef CAS PubMed.
- A. Amirshaghaghi, L. Yan, J. Miller, Y. Daniel, J. M. Stein, T. M. Busch, Z. Cheng and A. Tsourkas, Chlorin e6-Coated Superparamagnetic Iron Oxide Nanoparticle (SPION) Nanoclusters as a Theranostic Agent for Dual- Mode Imaging and Photodynamic Therapy, Sci. Rep., 2019, 9, 2613 CrossRef PubMed.
- Z. Wang, F. Zhang, D. Shao, Z. Chang, L. Wang, H. Hu, X. Zheng, X. Li, F. Chen, Z. Tu, M. Li, W. Sun, L. Chen and W. F. Dong, Janus Nanobullets Combine Photodynamic Therapy and Magnetic Hyperthermia to Potentiate Synergetic Anti-Metastatic Immunotherapy, Adv. Sci., 2019, 6, 1901690 CrossRef CAS PubMed.
- K. C. Nam, Y. S. Han, J. M. Lee, S. C. Kim, G. Cho and B. J. Park, Photo-Functionalized Magnetic Nanoparticles as a Nanocarrier of Photodynamic Anticancer Agent for Biomedical Theragnostics, Cancers, 2020, 12, 571 CrossRef CAS.
- T. T. Yu, X. C. Peng, M. F. Wang, N. Hang, H. Z. Xu, Q. R. Li, X. Xu, Q. L. Ma, B. Liu, J. Wang, L. Zhao, X. Chen and T. F. Li, Harnessing chlorin e6 loaded by functionalized iron oxide nanoparticles linked with glucose for target photodynamic therapy and improving of the immunogenicity of lung cancer, J. Cancer Res. Clin. Oncol., 2022, 148, 867 CrossRef CAS PubMed.
- E. J. Ngen, Y. Chen, B. B. Azad, S. Boinapally, D. Jacob, A. Lisok, C. Shen, M. S. Hossain, J. Jin, Z. M. Bhujwalla, M. G. Pomper and S. R. Banerjee, Prostate-specific membrane antigen (PSMA)-targeted photodynamic therapy enhances the delivery of PSMA-targeted magnetic nanoparticles to PSMA-expressing prostate tumors, Nanotheranostics, 2021, 5, 182–196 CrossRef PubMed.
- A. Ashkbar, F. Rezaei, F. Attari and S. Ashkevarian, Treatment of breast cancer in vivo by dual photodynamic and photothermal approaches with the aid of curcumin photosensitizer and magnetic nanoparticles, Sci. Rep., 2020, 10, 21206 CrossRef CAS PubMed.
- W. M. O. S. de Santana, B. L. Caetano, S. R. de Annunzio, S. H. Pulcinelli, C. Ménager, C. R. Fontana and C. V. Santilli, Conjugation of superparamagnetic iron oxide nanoparticles and curcumin photosensitizer to assist in photodynamic therapy, Colloids Surf., B, 2020, 196, 111297 CrossRef CAS PubMed.
- M. Aghajanzadeh, E. Naderi, M. Zamani, A. Sharafi, M. Naseri and H. Danafar, In vivo and in vitro biocompatibility study of MnFe2O4 and Cr2Fe6O12 as photosensitizer for photodynamic therapy and drug delivery of anti-cancer drugs, Drug Dev. Ind. Pharm., 2020, 46, 846–851 CrossRef CAS PubMed.
-
L. Prosen, S. Prijic, B. Music, J. Lavrencak, M. Cemazar and G. Sersa, Magnetofection: A Reproducible Method for Gene Delivery to Melanoma Cells, BioMed Research International, Hindawi Publishing Corporation, 2013, 2013, 11 Search PubMed.
- J. Pardo, Y. E. Sosa, P. Reggiani, M. L. Arciniegas, F. H. Sanchez and R. G. Goya, Magnetic fieldassisted gene transfer: Studies in glial cells, Acta Bioquim. Clin. Latinoam., 2013, 47, 399–406 CAS.
- S. Govindarajan, K. Kitaura, M. Takafuji, H. Ihara, K. S. Varadarajan, A. B. Patel and V. Gopal, Gene delivery into human cancer cells by cationic lipid-mediated magnetofection, Int. J. Pharm., 2013, 446, 87–99 CrossRef CAS PubMed.
- J. Yu, D. Y. Huang, M. Z. Yousaf, Y. L. Hou and S. Gao, Magnetic nanoparticle-based cancer therapy, Chin. Phys. B, 2013, 22, 027506 CrossRef.
- N. Laurent, C. Sapet, L. L. Gourrierec, E. Bertosio and O. Zelphati, Nucleic acid delivery using magnetic nanoparticles: the Magnetofection technology, Ther. Delivery, 2011, 2, 471–482 CrossRef CAS PubMed.
- M. Rostami, A. S. Nasab, M. F. Ramandi, A. Badiei, M. R. Ganjali, M. R. Nasrabadi and F. Ahmadi, Cur-loaded magnetic ZnFe2O4@mZnO-Ox-p-g-C3N4 composites as dual pH and ultrasound responsive nano-carriers for controlled and targeted cancer chemotherapy, Mater. Chem. Phys., 2021, 271, 124863 CrossRef CAS.
- Y. S. Borghei, S. Hosseinkhani and M. R. Ganjali, Engineering in modern medicine using ‘magnetic nanoparticles’ in understanding physicochemical interactions at the nano–bio interfaces, Mater. Today Chem., 2022, 23, 100733 CrossRef CAS.
-
S. Uthaman, M. Muthiah, I. K. Park and C. S. Cho, 9 – Fabrication and development of magnetic particles for gene therapy, Polymers and Nanomaterials for Gene Therapy, Woodhead Publishing, 2016, 215–230 Search PubMed.
- J. Dobson, Gene therapy progress and prospects: magnetic nanoparticle-based gene delivery, Gene Ther., 2006, 13, 283–287 CrossRef CAS PubMed.
- U. Schillinger, T. Brill, C. Rudolph, S. Huth, S. Gersting, F. Krötz, J. Hirschberger, C. Bergemann and C. Plank, Advances in magnetofection—magnetically guided nucleic acid delivery, J. Magn. Magn. Mater., 2005, 293, 501–508 CrossRef CAS.
- Y. Namiki, T. Namiki, H. Yoshida, Y. Ishii, A. Tsubota, S. Koido, K. Nariai, M. Mitsunaga, S. Yanagisawa, H. Kashiwagi, Y. Mabashi, Y. Yumoto, S. Hoshina, K. Fujise and N. Tada, A novel magnetic crystal–lipid nanostructure for magnetically guided in vivo gene delivery, Nat. Nanotechnol., 2009, 4, 598–606 CrossRef CAS PubMed.
- R. Hadianamrei and X. Zhao, Current state of the art in peptide-based gene delivery, J. Controlled Release, 2022, 343, 600–619 CrossRef CAS PubMed.
|
This journal is © The Royal Society of Chemistry 2022 |