DOI:
10.1039/D1SC04846E
(Edge Article)
Chem. Sci., 2021,
12, 14618-14623
When SF5 outplays CF3: effects of pentafluorosulfanyl decorated scorpionates on copper†
Received
1st September 2021
, Accepted 15th October 2021
First published on 15th October 2021
Abstract
Polyfluorinated, electron-withdrawing, and sterically demanding supporting ligands are of significant value in chemistry. Here we report the assembly and use of a bis(pyrazolyl)borate, [Ph2B(3-(SF5)Pz)2]− that combines all such features, and involves underutilized pentafluorosulfanyl substituents. The ethylene and carbonyl chemistry of copper(I) supported by [Ph2B(3-(SF5)Pz)2]−, a comparison to the trifluoromethylated counterparts involving [Ph2B(3-(CF3)Pz)2]−, as well as copper catalyzed cyclopropanation of styrene with ethyl diazoacetate and CF3CHN2 are presented. The results from cyclopropanation show that SF5 groups dramatically improved the yields and stereoselectivity compared to the CF3.
Introduction
Substituents are the key to modulating the chemical and physical properties of molecules, including those of metal complexes and catalysts. The number of electron-withdrawing substituents that can be utilized for this purpose that are also relatively inert and practical, however, are quite limited. Fluorinated substituents such as the trifluoromethyl (CF3) group are especially useful in this regard as they often drastically alter the properties of a molecule compared to their hydrocarbon counterparts.1 The pentafluorosulfanyl (SF5) is a noticeably underutilized fluorinated substituent compared to the CF3 group in chemistry.2 It is, however, gaining increasing attention due to its unique and attractive properties including large size (marginally smaller than a tert-butyl group), strong electron-withdrawing capabilities, high lipophilicity and excellent chemical and thermal stability, and showing great promise in agrochemical, medicinal and materials chemistry applications.2,3 Furthermore, molecules with pentafluorosulfanyl groups are also becoming more accessible via effective and convenient routes.4 A number of derivatization reactions of SF5-group containing molecules are also known.3a,3b,4e,5
The metal complexes featuring SF5 groups are quite limited,3a,6 although it was a substituent first introduced in 1960.7 Promising outcomes noted in recent reports suggest that pentafluorosulfanyl moiety merits more closer scrutiny and wider utility. For example, recent work by Mecking and co-workers illustrated the benefits of SF5 over CF3 groups on Ni(II) salicylaldiminato complexes in ethylene polymerization catalysis (to get more linear and higher molecular weight polymers),6a as well as on tetraphenylborate ions in Ni(II) mediated butadiene polymerizations.8 In addition, SF5 group has been utilized in luminescent transition metal complexes to minimize the aggregation in the solid-state, improve the solubility, and alter the emission features such as blue shifting of the phosphorescent emissions more significantly relatively to CF3 bearing analogs.2b,3a,9
Poly(pyrazolyl)borates, commonly referred to as scorpionates,10 are very valuable class of ligands in coordination chemistry and catalysis, and form complexes with most metals of the periodic table. Here we report the first metal scorpionates decorated with pentafluorosulfanyl groups. In particular, we describe the synthesis of [Ph2B(3-(SF5)Pz)2]− and the effects of this ligand support on copper(I) as reflected in the structures and bonding of ethylene and CO complexes (which represent two classes of organometallic complexes with significant fundamental and practical significance),11 and catalytic alkene cyclopropanation, as well as a direct comparison to the related trifluoromethylated analogs (Fig. 1). It is also notable that there is only an isolated example of a copper complex involving a 4-SF5C6H4-substituted ligand to our knowledge,12 whereas CF3-bearing ligands with copper are more common and valued in many applications.11,13
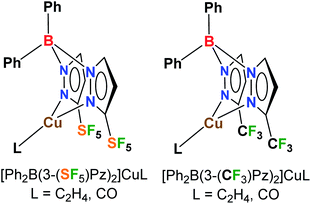 |
| Fig. 1 Bis(pyrazolyl)boratocopper(I) complexes decorated with pentafluorosulfanyl (SF5) and trifluoromethyl (CF3) groups. | |
Results and discussion
Synthesis of the SF5-pyrazole
In 1964, Hoover and Coffman reported that a reaction of alkyne 2 with diazomethane in diethyl ether at 0 °C led to the formation of a mixture of isomeric products 1 and 3 (3
:
2) (Scheme 1).14 The authors also mentioned that: “these pyrazoles were not separated.” Therefore, we needed to develop a robust practical protocol for the SF5-prazole 1. After some optimization, we found that the reaction of alkene 4 with diazomethane at −10 °C gave pyrazoline 5 in 85% yield. Oxidation of the latter with MnO2 followed by crystallization of the resulting material from hexane gave the needed compound SF5-pyrazole (1) in 38% yield. This product was obtained in 11 g scale in one run (Scheme 1).
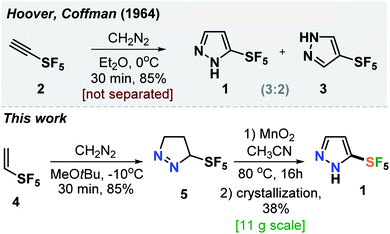 |
| Scheme 1 Synthesis of SF5-pyrazole 1. | |
Synthesis of fluorinated scorpionate ligands and copper–ethylene complexes
The fluorinated bis(pyrazolyl)borate [Ph2B(3-(SF5)Pz)2]− ligand possessing SF5 groups at the pyrazolyl ring 3-positions was prepared by a reaction of SF5-pyrazole (1) with NaBPh4via a benzene elimination pathway (Scheme 2, see ESI† section). This resulting sodium salt was converted to [Ph2B(3-(SF5)Pz)2]Tl (6) through metathesis using TlOAc, and utilized in the synthesis of [Ph2B(3-(SF5)Pz)2]Cu(C2H4) (7) by treating with CuOTf and ethylene (Scheme 2). The related copper–ethylene, complex [Ph2B(3-(CF3)Pz)2]Cu(C2H4) was also synthesized for a comparison. They are colorless crystalline solids, and stable to loss of ethylene in a nitrogen atmosphere at room temperature. The 19F NMR spectra of the two adducts are very different due to the unique square pyramidal arrangement of fluorine atoms in SF5 moieties vs. trigonal pyramidal array in CF3 groups, leading to a doublet and a pentet in the former and a singlet in the latter.
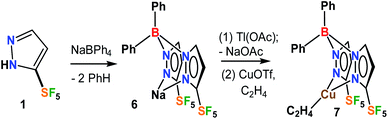 |
| Scheme 2 Synthesis of [Ph2B(3-(SF5)Pz)2]Cu(C2H4). | |
The ethylene 13C NMR signal in [Ph2B(3-(SF5)Pz)2]Cu(C2H4) was observed at δ = 86.4 ppm. This resonance in [Ph2B(3-(CF3)Pz)2]Cu(C2H4) was observed at δ 82.7 ppm, which is an even larger upfield shift from the free C2H4 (δ 123.1 ppm) signal. Larger upfield shift of the metal bound ethylene 13C resonance from the free C2H4 (δ 123.1 ppm) signal has been attributed to the increased shielding resulting from metal-to-ethylene π-back-donation.15 Thus [Ph2B(3-(SF5)Pz)2]Cu(C2H4), with a smaller upfield shift points to relatively lower Cu → ethylene π-backbonding. This is reasonable considering the presence of more electron withdrawing SF5 groups (with an estimated electronegativity of 3.65 vs. 3.36, and Hammett substituent constant σm of 0.61 vs. 0.43 for SF5vs. CF3)2,16 on the scorpionate ligand backbone of this copper complex. For comparison, three coordinate [t-Bu2P(NSiMe3)2]Cu(C2H4) with a more strongly backbonding copper site displays its ethylene carbon shift at δ 73.0 ppm.17 The 13C NMR data are particularly useful for such bonding analysis since they are less affected by the ring current effects.
The ethylene protons of [Ph2B(3-(R)Pz)2]Cu(C2H4) (R = –SF5, –CF3) in the 1H NMR spectrum appear at δ 3.72 and 3.69 ppm, respectively. These protons are most likely affected by the ring currents of flanking phenyl groups sitting over ethylene moieties (see molecular structures below). The presence of additional ethylene in CDCl3 solutions at room temperature leads to separate broad signals of free and coordinated ethylene in [Ph2B(3-(CF3)Pz)2]Cu(C2H4) whereas these signals remain sharp for the –SF5 analog 7, suggesting a quite rapid olefin exchange only in the former at room temperature on the NMR time scale.
X-ray crystal structures of Cu–ethylene complexes
Molecular structure of [Ph2B(3-(R)Pz)2]Cu(C2H4) (R = SF5, CF3) were unambiguously established by single-crystal X-ray diffraction (Fig. 2). Compound [Ph2B(3-(SF5)Pz)2]Cu(C2H4) crystallizes with two chemical identical but crystallographically distinct molecules in the asymmetric unit. Selected bond distance and angles are given in Table S3 (ESI†). They are three-coordinate, trigonal planar copper complexes with an η2-bound C2H4 moieties. The bis(pyrazolyl)borate ligands coordinate to copper in κ2 fashion via nitrogen atoms of two pyrazolyl arms and adopt a boat configuration. One of the phenyl groups on boron sits above the ethylene group. Most of the key features are similar between the two adducts, although the [Ph2B(3-(SF5)Pz)2]Cu(C2H4) has slightly longer Cu–C and Cu–N distances compared to those of the CF3 analog. This could be a result of either greater steric demand or more weakly donating nature of scorpionate in [Ph2B(3-(SF5)Pz)2]Cu(C2H4).
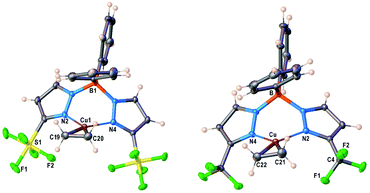 |
| Fig. 2 Molecular structures of [Ph2B(3-(SF5)Pz)2]Cu(C2H4) (7) and [Ph2B(3-(CF3)Pz)2]Cu(C2H4), from left to right. | |
Analysis of the topographic steric maps of the two metal complexes using SambVca18 and the X-ray crystallographic data indicate percent buried volumes of 69.9% and 64.0% for [Ph2B(3-(SF5)Pz)2]Cu(C2H4) and [Ph2B(3-(CF3)Pz)2]Cu(C2H4), respectively (Fig. 3), clearly indicating more protected copper sites in the former as a result of having sterically more demanding SF5 groups at the periphery of the coordination pocket. Sluggish ethylene exchange in [Ph2B(3-(SF5)Pz)2]Cu(C2H4) noted above is probably a result of having greater steric protection at the copper site of this –SF5 bearing molecule.
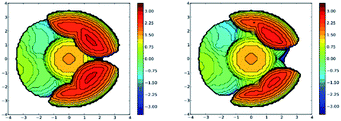 |
| Fig. 3 Steric maps of [Ph2B(3-(SF5)Pz)2]Cu (left) and [Ph2B(3-(CF3)Pz)2]Cu (right) moieties based on the calculations using SambVca tool and X-ray data from the ethylene complexes [Ph2B(3-(SF5)Pz)2]Cu(C2H4) and [Ph2B(3-(CF3)Pz)2]Cu(C2H4). The resulting % buried volume values are 69.9% (average for the two molecules in the asymmetric unit) and 64.0%, respectively. | |
Computational analysis of copper–ethylene complexes
We have also investigated alkene–copper(I) bonding of [Ph2B(3-(SF5)Pz)}2]Cu(C2H4), [Ph2B(3-(CF3)Pz)2]Cu(C2H4), and the hypothetical [Ph2B(3-(CH3)Pz)2]Cu(C2H4) via density functional calculations. The calculated interaction energy (ΔEint) between the ethylene and Cu(I) center remains similar, ranging from −44.9, −45.9, to −45.2 kcal mol−1 (Table 1), respectively, which is further dissected in different contributions within the Ziegler–Rauk energy decomposition analysis (EDA).19 It shows that these interactions are primarily electrostatic in nature for all three [Ph2B(3-(R)Pz)2]Cu(C2H4) complexes as evident from ΔEelstat of about ∼60%, with the remainder consists of ∼36% orbital contributions (ΔEorb) and ∼4% dispersion-type interactions (ΔEdisp). The ΔEorb of [Ph2B(3-(SF5)Pz)}2]Cu(C2H4) composed of σ-donation and π-backdonation ascribed to the π1-C2H4 → Cu and
(Fig. S43, ESI†) in a 29.9% and 54.9% contribution, respectively, which is similar in trend but shows a gradual decrease and an increase in the two components going from –SF5 to the –CF3 and –CH3 analogs, culminating in 24.5% and 63.7%, σ/π-contributions in the most electron rich scorpionate ligand analog [Ph2B(3-(CH3)Pz)2]Cu(C2H4). These interactions cause a more red-shifted
(C
C) as evident from the computed values of 1516.3, 1513.5 and 1509.3 cm−1, for [Ph2B(3-(R)Pz)2]Cu(C2H4) (R = –SF5, –CF3, –CH3, respectively). These numbers follow the order of Hammett substituent constant σm/σp (0.61/0.68, 0.43/0.54, and −0.07/−0.17 for –SF5, –CF3, –CH3, respectively),2,16 and are inversely related to the
backbonding contribution (Table 1). This trend is also consistent with computed proton affinities of the [Ph2B(3-(R)Pz)2]− ligands (and therefore, the donor features of the scorpionate nitrogen sites; see ESI Table S17†), and indicate that [Ph2B(3-(SF5)Pz)2]Cu(C2H4) features the most weakly donating scorpionate and least backbonding copper site in this series.
Table 1 Energy decomposition analyses for the C2H4–Cu interaction for different [Ph2B(3-(R)Pz)2]Cu(C2H4) complexes, with R = –SF5, –CF3, and –CH3. Values in kcal mol−1. In addition, π-backbonding and σ-donation components are given as
and π1-C2H4 → Cu, respectively. Calculated
(C
C) (in cm−1) values are also given
Parameter |
[Ph2B(3-(SF5)Pz)2]Cu(C2H4) |
[Ph2B(3-(CF3)Pz)2]Cu(C2H4) |
[Ph2B(3-(CH3)Pz)2]Cu(C2H4) |
Percentage contribution to the total attractive interactions ΔEelstat + ΔEorb + ΔEdisp.
Percentage contribution to the total orbital interactions ΔEorb.
|
ΔEint |
−44.9 |
|
−45.9 |
|
−45.2 |
|
ΔEPauli |
114.0 |
|
112.5 |
|
125.4 |
|
ΔEdisp |
−6.5 |
4.1%a |
−5.6 |
3.5%a |
−5.3 |
3.1%a |
ΔEelstat |
−95.2 |
59.9%a |
−95.0 |
59.9%a |
−102.1 |
59.9%a |
ΔEorb |
−57.2 |
36.0%a |
−57.9 |
36.6%a |
−63.2 |
37.1%a |
π1-C2H4 → Cu |
−17.1 |
29.9%b |
−16.5 |
28.5%b |
−15.5 |
24.5%b |
|
−31.4 |
54.9%b |
−33.5 |
57.9%b |
−40.2 |
63.7%b |
|
−8.7 |
|
−7.8 |
|
−7.5 |
|
(C C) calc. |
1516.3 |
|
1513.5 |
|
1509.3 |
|
Olefin displacement leading to Cu-carbonyl complexes
Some reactivities and catalytic features of [Ph2B(3-(SF5)Pz)2]Cu(C2H4), [Ph2B(3-(CF3)Pz)2]Cu(C2H4) have also been investigated. Upon treatment with CO in CH2Cl2, both adducts afford the corresponding copper carbonyl complexes. They do not lose CO under reduced pressure. The CO stretching frequencies of [Ph2B(3-(SF5)Pz)2]Cu(CO) and [Ph2B(3-(CF3)Pz)2]Cu(CO) were observed at 2121 and 2117 cm−1, respectively. For comparison, the
(CO) for the highly fluorinated [H2B(3,5-(CF3)2Pz)2]Cu(CO)20 and relatively electron rich [(Ph3B)CH(3,5-(CH3)2Pz)2]Cu(CO)21 appear at 2127 and 2092 cm−1, respectively. These data indicate that [Ph2B(3-(SF5)Pz)2]Cu(CO) has a notably Lewis acidic copper site, and a relatively weakly donating supporting scorpionate, consistent with the observed carbon chemical shifts and DFT analysis of the corresponding ethylene complex. DFT calculations show that the Cu–CO interaction (ESI†) is slightly less favorable than Cu–C2H4 (Table 1) in the corresponding [Ph2B(3-(R)Pz)2]Cu(CO) (ΔEint = −39.9 (R = SF5), −39.8 (R = CF3), and −40.9 kcal mol−1 (for hypothetical R = CH3)). The thermochemical parameters for the observed C2H4 → CO replacement in 7 and its –CF3 counterpart were also estimated computationally, which show that the free-energy change at room temperature (ΔG298 K) for these reactions are very small at +1.30 and +0.59 kcal mol−1, respectively. They are essentially thermo-neutral processes. Indeed, it is possible to treat CHCl3 solutions of [Ph2B(3-(SF5)Pz)2]Cu(CO) and [Ph2B(3-(CF3)Pz)2]Cu(CO) with ethylene at room temperature to re-generate the corresponding ethylene complexes. The Cu–CO bonding features of [Ph2B(3-(R)Pz)2]Cu(CO) were also investigated using DFT and found to vary systematically along the R = –SF5, –CF3, and –CH3 series, with the lowest 2π* ← Cu backbonding observed for [Ph2B(3-(SF5)Pz)2]Cu(CO), leading to the calculated
(CO) of 2110, 2099, and 2080 cm−1, respectively (ESI†).
Molecular structures of [Ph2B(3-(SF5)Pz)2]Cu(CO), [Ph2B(3-(CF3)Pz)2]Cu(CO) are illustrated in Fig. 4. There are two chemically identical molecules of [Ph2B(3-(SF5)Pz)2]Cu(CO) in its asymmetric unit. Selected bond distances and angles are presented in Table S3 (ESI†). The Cu–CO moieties are essentially linear. The scorpionate coordinates to the metal ion in κ2 fashion and adopts a boat configuration. One of the phenyl groups on boron sits above the copper center. The metal to ipso-carbon distances are 2.58 and 2.78 Å in [Ph2B(3-(R)Pz)2]Cu(C2H4) (R = –SF5, –CF3), respectively. These separations are within the sum of van der Waals radii of Cu and C (3.10 Å). However, these contacts do not appear to be significant enough to distort the coordination geometry at the metal center because these molecules feature trigonal planar metal sites as evident from the sum of angles at the metal center (∼360°). Furthermore, the
(CO) values suggest that the copper sites remain quite Lewis acidic despite the close approach of the phenyl groups. Note that three-coordinate, trigonal planar copper carbonyls are very limited.22
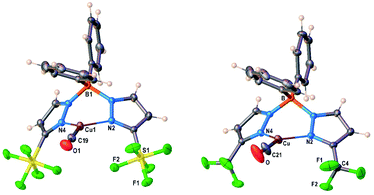 |
| Fig. 4 Molecular structures of [Ph2B(3-(SF5)Pz)2]Cu(CO) and [Ph2B(3-(CF3)Pz)2]Cu(CO), from left to right. | |
Analysis of the topographic steric maps of the two metal complexes using SambVca18 and the X-ray crystallographic data indicate percent buried volumes (%Vbur) of 72.8% and 66.3% for [Ph2B(3-(SF5)Pz)2]Cu(CO) and [Ph2B(3-(CF3)Pz)2]Cu(CO), respectively clearly indicting more protected copper sites in the former as a result of having sterically more demanding SF5 groups at the periphery of the coordination pocket (Fig. S41, ESI†). These percent buried volume values are larger than those observed for the related ethylene analogs (described above), indicating the adaptability of the scorpionate to accommodate organometallic fragments of different sizes.
Catalytic activity of copper-complexes
Finally, we have also investigated the catalytic potential of [Ph2B(3-(R)Pz)2]Cu(C2H4) (R = –SF5, –CF3) in cyclopropanation via a carbene transfer process. It was found that on reaction of styrene with ethyl diazoacetate (EDA), both copper(I) complexes serve as carbene transfer agents providing the expected cyclopropane as a diastereomeric mixture (Scheme 2). However, [Ph2B(3-(SF5)Pz)2]Cu(C2H4) gave dramatically higher cyclopropane product yields (99% vs. 62%) and greater cis-selectivity (3
:
2 vs. 1
:
1) compared to the –CF3 substituted analog (Scheme 2).
These results are consistent with the previous reports by Perez and co-workers involving tris(pyrazolyl)boratocopper complexes and EDA, which indicate that the higher cis-selectivities are associated with bulkier supporting ligands.23 Interestingly, when CF3CHN2 was used as the carbene source,24 [Ph2B(3-(SF5)Pz)2]Cu(C2H4) again gave notably higher product yields than the [Ph2B(3-(CF3)Pz)2]Cu(C2H4) catalyzed process, but this time, the trans-isomer was the major product. It is also known that the cis-isomer is the kinetic product while the trans-isomer is the thermodynamically favored product.23 Therefore, it is possible that the greater steric bulk of the diazo reagent CF3CHN2 (compared to EDA) favors the latter, causing this interesting reversal in diastereoselectivity. Indeed, Doyle et al. has observed high trans-selective cyclopropanations in rhodium chemistry with bulky diazo reagents (Scheme 3).25
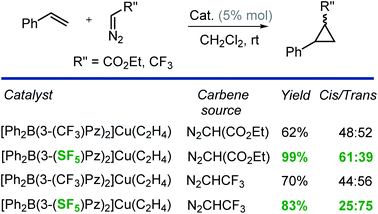 |
| Scheme 3 Cyclopropanation of styrene with N2CHCO2Et (EDA) and CF3CHN2. | |
Conclusions
Overall, we have described the preparation and characterization of the first pentafluorosulfanyl decorated scorpionate [Ph2B(3-(SF5)Pz)2]− and some of its copper chemistry, as well as a new, regioselective route to SF5-pyrazole. The [Ph2B(3-(SF5)Pz)2]− is a more sterically demanding and weakly donating ligand compared to the [Ph2B(3-(CF3)Pz)2]−, as evident from the copper ethylene and carbonyl chemistry and computational analysis. Moreover, the [Ph2B(3-(SF5)Pz)2]Cu(C2H4) (7) complex displays significantly better efficacy in cyclopropanation of styrene with EDA and CF3CHN2 compared to that of [Ph2B(3-(SF5)Pz)2]Cu(C2H4). Fluorinated ligands are important as they often provide metal complexes with certain beneficial features relative to the non-fluorinated, hydrocarbon group bearing ligands. Given the common appearance of CF3-ligands in various areas of chemistry,1f we believe that with this work, the SF5-analogues will also become popular. Further studies on metal complexes supported by SF5 containing ligands and practical approaches to other SF5-heterocycles are currently underway.
Data availability
All data associated with this article can be found in the ESI.†
Author contributions
Conceptualization: HVRD, PM; investigation: AN-P, AM-C, AB; writing and validation: AN-P, AM-C, AB, PM, HVRD; project administration: HVRD.
Conflicts of interest
There are no conflicts to declare.
Acknowledgements
This work was supported by the Robert A. Welch Foundation (Grant Y-1289) and Ministry of Education and Science of Ukraine for perspective development of a scientific direction “Mathematical sciences and natural sciences” at Taras Shevchenko National University of Kyiv. AB and PKM are grateful to Oleksandr I. Khyzhan (Enamine) for setting up a reaction of alkene 4 with CH2N2.
Notes and references
-
(a) O. A. Tomashenko and V. V. Grushin, Chem. Rev., 2011, 111, 4475–4521 CrossRef CAS PubMed
;
(b) T. Furuya, A. S. Kamlet and T. Ritter, Nature, 2011, 473, 470–477 CrossRef CAS PubMed
;
(c) C. Alonso, E. Martinez de Marigorta, G. Rubiales and F. Palacios, Chem. Rev., 2015, 115, 1847–1935 CrossRef CAS PubMed
;
(d) X. Liu, C. Xu, M. Wang and Q. Liu, Chem. Rev., 2015, 115, 683–730 CrossRef CAS PubMed
;
(e) J. Wang, M. Sanchez-Rosello, J. L. Acena, C. del Pozo, A. E. Sorochinsky, S. Fustero, V. A. Soloshonok and H. Liu, Chem. Rev., 2014, 114, 2432–2506 CrossRef CAS PubMed
;
(f) P. K. Mykhailiuk, Chem. Rev., 2021, 121, 1670–1715 CrossRef CAS PubMed
.
-
(a) P. R. Savoie and J. T. Welch, Chem. Rev., 2015, 115, 1130–1190 CrossRef CAS PubMed
;
(b) J. M. W. Chan, J. Mater. Chem. C, 2019, 7, 12822–12834 RSC
.
-
(a) N. M. Shavaleev, G. Xie, S. Varghese, D. B. Cordes, A. M. Z. Slawin, C. Momblona, E. Ortí, H. J. Bolink, I. D. W. Samuel and E. Zysman-Colman, Inorg. Chem., 2015, 54, 5907–5914 CrossRef CAS PubMed
;
(b) S. Garg and J. n. M. Shreeve, J. Mater. Chem., 2011, 21, 4787–4795 RSC
;
(c) S. Altomonte and M. Zanda, J. Fluorine Chem., 2012, 143, 57–93 CrossRef CAS
;
(d) M. Herder, B. M. Schmidt, L. Grubert, M. Paetzel, J. Schwarz and S. Hecht, J. Am. Chem. Soc., 2015, 137, 2738–2747 CrossRef CAS PubMed
;
(e) M. F. Sowaileh, R. A. Hazlitt and D. A. Colby, ChemMedChem, 2017, 12, 1481–1490 CrossRef CAS PubMed
;
(f) H. Qianzhu, A. P. Welegedara, H. Williamson, A. E. McGrath, M. C. Mahawaththa, N. E. Dixon, G. Otting and T. Huber, J. Am. Chem. Soc., 2020, 142, 17277–17281 CrossRef CAS PubMed
;
(g) P. Liebing, C. R. Pitts, M. Reimann, N. Trapp, D. Rombach, D. Bornemann, M. Kaupp and A. Togni, Chem.–Eur. J., 2021, 27, 6086–6093 CrossRef CAS PubMed
.
-
(a) T. Umemoto, L. M. Garrick and N. Saito, Beilstein J. Org. Chem., 2012, 8, 461–471 CrossRef CAS PubMed
;
(b) B. Cui, M. Kosobokov, K. Matsuzaki, E. Tokunaga and N. Shibata, Chem. Commun., 2017, 53, 5997–6000 RSC
;
(c) O. S. Kanishchev and W. R. Dolbier Jr, Angew. Chem., Int. Ed., 2015, 54, 280–284 CrossRef CAS PubMed
;
(d) C. R. Pitts, D. Bornemann, P. Liebing, N. Santschi and A. Togni, Angew. Chem., Int. Ed., 2019, 58, 1950–1954 CrossRef CAS PubMed
;
(e) J.-Y. Shou, X.-H. Xu and F.-L. Qing, Angew. Chem., Int. Ed., 2021, 60, 15271–15275 CrossRef CAS PubMed
.
-
(a) A. Gilbert, X. Bertrand and J.-F. Paquin, Org. Lett., 2018, 20, 7257–7260 CrossRef CAS PubMed
;
(b) M. Cloutier, M. Roudias and J.-F. Paquin, Org. Lett., 2019, 21, 3866–3870 CrossRef CAS PubMed
;
(c) W. R. Dolbier, S. Aït-Mohand, T. D. Schertz, T. A. Sergeeva, J. A. Cradlebaugh, A. Mitani, G. L. Gard, R. W. Winter and J. S. Thrasher, J. Fluorine Chem., 2006, 127, 1302–1310 CrossRef CAS
;
(d) A. Penger, C. N. von Hahmann, A. S. Filatov and J. T. Welch, Beilstein J. Org. Chem., 2013, 9, 2675–2680 CrossRef PubMed
.
-
(a) P. Kenyon and S. Mecking, J. Am. Chem. Soc., 2017, 139, 13786–13790 CrossRef CAS PubMed
;
(b) M. Talavera, S. Hinze, T. Braun, R. Laubenstein and R. Herrmann, Molecules, 2020, 25, 3977 CrossRef CAS PubMed
;
(c) R. D. W. Kemmitt, R. D. Peacock and J. Stocks, J. Chem. Soc. D, 1969, 554 RSC
.
- W. A. Sheppard, J. Am. Chem. Soc., 1960, 82, 4751–4752 CrossRef CAS
.
- D. Langford, I. Goettker-Schnetmann, F. P. Wimmer, L. A. Casper, P. Kenyon, R. F. Winter and S. Mecking, Organometallics, 2019, 38, 2710–2713 CrossRef CAS
.
-
(a) X.-F. Ma, X.-F. Luo, Z.-P. Yan, Z.-G. Wu, Y. Zhao, Y.-X. Zheng and J.-L. Zuo, Organometallics, 2019, 38, 3553–3559 CrossRef CAS
;
(b) P. Gautam, Y. Wang, G. Zhang, H. Sun and J. M. W. Chan, Chem. Mater., 2018, 30, 7055–7066 CrossRef CAS
;
(c) H. R. A. Golf, H.-U. Reissig and A. Wiehe, J. Org. Chem., 2015, 80, 5133–5143 CrossRef CAS PubMed
;
(d) L. M. Groves, C. Schotten, J. Beames, J. A. Platts, S. J. Coles, P. N. Horton, D. L. Browne and S. J. A. Pope, Chem.–Eur. J., 2017, 23, 9407–9418 CrossRef CAS PubMed
;
(e) A. K. Pal, A. F. Henwood, D. B. Cordes, A. M. Z. Slawin, I. D. W. Samuel and E. Zysman-Colman, Inorg. Chem., 2017, 56, 7533–7544 CrossRef CAS PubMed
.
-
(a) S. Trofimenko, Chem. Rev., 1993, 93, 943–980 CrossRef CAS
;
(b) C. Pettinari and C. Santini, Compr. Coord. Chem. II, 2004, 1, 159–210 CAS
.
- H. V. R. Dias and C. J. Lovely, Chem. Rev., 2008, 108, 3223–3238 CrossRef CAS PubMed
.
- K.-C. Chan, S.-C. Cheng, L. T.-L. Lo, S.-M. Yiu and C.-C. Ko, Eur. J. Inorg. Chem., 2018, 2018, 897–903 CrossRef CAS
.
-
(a) N. B. Jayaratna, M. G. Cowan, D. Parasar, H. H. Funke, J. Reibenspies, P. K. Mykhailiuk, O. Artamonov, R. D. Noble and H. V. R. Dias, Angew. Chem., Int. Ed., 2018, 57, 16442–16446 CrossRef CAS PubMed
;
(b) D. Parasar, A. H. Elashkar, A. A. Yakovenko, N. B. Jayaratna, B. L. Edwards, S. G. Telfer, H. V. R. Dias and M. G. Cowan, Angew. Chem., Int. Ed., 2020, 59, 20713 CrossRef CAS
;
(c) H. V. R. Dias, H. V. K. Diyabalanage, M. G. Eldabaja, O. Elbjeirami, M. A. Rawashdeh-Omary and M. A. Omary, J. Am. Chem. Soc., 2005, 127, 7489–7501 CrossRef CAS PubMed
;
(d) A. Noonikara-Poyil, S. G. Ridlen and H. V. R. Dias, Inorg. Chem., 2020, 59, 17860–17865 CrossRef CAS PubMed
;
(e) B. Esser, J. M. Schnorr and T. M. Swager, Angew. Chem., Int. Ed., 2012, 51, 5752–5756 CrossRef CAS PubMed
.
- F. W. Hoover and D. D. Coffman, J. Org. Chem., 1964, 29, 3567–3570 CrossRef CAS
.
-
(a) H. V. R. Dias and J. Wu, Eur. J. Inorg. Chem., 2008, 509–522 CrossRef CAS
;
(b) T. F. van Dijkman, M. A. Siegler and E. Bouwman, Dalton Trans., 2015, 44, 21109–21123 RSC
;
(c) P. O. Oguadinma and F. Schaper, Organometallics, 2009, 28, 6721–6731 CrossRef CAS
.
- C. Hansch, A. Leo and R. W. Taft, Chem. Rev., 1991, 91, 165–195 CrossRef CAS
.
- B. F. Straub, F. Eisentrager and P. Hofmann, Chem. Commun., 1999, 2507–2508 RSC
.
- L. Falivene, Z. Cao, A. Petta, L. Serra, A. Poater, R. Oliva, V. Scarano and L. Cavallo, Nat. Chem., 2019, 11, 872–879 CrossRef CAS PubMed
.
-
(a) T. Ziegler and A. Rauk, Inorg. Chem., 1979, 18, 1558–1565 CrossRef CAS
;
(b) M. P. Mitoraj, A. Michalak and T. Ziegler, J. Chem. Theory Comput., 2009, 5, 962–975 CrossRef CAS PubMed
.
- G. Pampaloni, R. Peloso, D. Belletti, C. Graiff and A. Tiripicchio, Organometallics, 2007, 26, 4278–4286 CrossRef CAS
.
- S. G. Ridlen, N. V. Kulkarni and H. V. R. Dias, Inorg. Chem., 2017, 56, 7237–7246 CrossRef CAS PubMed
.
-
(a) H. V. R. Dias and S. Singh, Inorg. Chem., 2004, 43, 5786–5788 CrossRef CAS PubMed
;
(b) D. Parasar, N. B. Jayaratna, A. Muñoz-Castro, A. E. Conway, P. K. Mykhailiuk and H. V. R. Dias, Dalton Trans., 2019, 48, 6358–6371 RSC
;
(c) K. Huse, H. Weinert, C. Wölper and S. Schulz, Dalton Trans., 2020, 49, 9773–9780 RSC
;
(d) R. A. Peralta, M. T. Huxley, J. Albalad, C. J. Sumby and C. J. Doonan, Inorg. Chem., 2021, 60, 11775–11783 CrossRef CAS PubMed
.
- M. M. Diaz-Requejo, A. Caballero, T. R. Belderrain, M. C. Nicasio, S. Trofimenko and P. J. Perez, J. Am. Chem. Soc., 2002, 124, 978–983 CrossRef CAS PubMed
.
-
(a) P. K. Mykhailiuk, S. Afonin, A. S. Ulrich and I. V. Komarov, Synthesis, 2008, 2008, 1757–1760 CrossRef
;
(b) P. K. Mykhailiuk, Chem. Rev., 2020, 120, 12718–12755 CrossRef CAS PubMed
.
-
(a) H. Lebel, J.-F. Marcoux, C. Molinaro and A. B. Charette, Chem. Rev., 2003, 103, 977–1050 CrossRef CAS PubMed
;
(b) M. P. Doyle, V. Bagheri, T. J. Wandless, N. K. Harn, D. A. Brinker, C. T. Eagle and K. L. Loh, J. Am. Chem. Soc., 1990, 112, 1906–1912 CrossRef CAS
.
Footnote |
† Electronic supplementary information (ESI) available: Experimental details, synthesis, catalysis, spectroscopic and structural data, steric maps, computational data, additional figures and tables. The CCDC 2104704–2104707 files contain the supplementary crystallographic data. For ESI and crystallographic data in CIF or other electronic format see DOI: 10.1039/d1sc04846e |
|
This journal is © The Royal Society of Chemistry 2021 |
Click here to see how this site uses Cookies. View our privacy policy here.