DOI:
10.1039/D0GC03510F
(Paper)
Green Chem., 2021,
23, 592-596
Highly efficient synthesis of alkylidene cyclic carbonates from low concentration CO2 using hydroxyl and azolate dual functionalized ionic liquids†
Received
17th October 2020
, Accepted 8th December 2020
First published on 9th December 2020
Abstract
A highly efficient catalytic system was developed for the reaction between CO2 and propargylic alcohols for alkylidene cyclic carbonates. Ionic liquids (ILs) with different anions and cations were designed as cocatalysts, in order to find out the effect of the cation and the anion on this reaction. The results indicated that the effect of the cation was significant, especially the hydroxyl group on the cation played an important role due to the presence of a hydrogen bond. It was also found that the basicity of the anion was important for its catalytic activity, where the anion with moderate basicity gave the best activity. Moreover, this hydroxyl and azolate dual functionalized catalytic system showed excellent reusability and generality. It is worth mentioning that at a low concentration of CO2, this dual functionalized catalytic system showed excellent catalytic activity even in a gram-scale reaction, indicating its potential in carbon capture and utilization processes.
Introduction
CO2 capture and utilization (CCU) has evoked great interest in the past few decades because CO2 can be regarded as an abundant, cheap, and environmentally friendly C1 synthon, which is widely used for the synthesis of many fine chemicals.1–6 Unfortunately, because of its inherent thermodynamic stability and kinetic inertness, the CCU processes usually require high energy input (including high temperature, high pressure) and the addition of metal catalysts or excessive strong base.7,8 In addition, compared with other CO2 sources, fixation of CO2 from flue gas, one of the major CO2 sources, is still a major challenge due to its low CO2 concentration (∼10 vol%).9–11 Thus, effective catalysts are still urgently desired for CO2 upgradation at low concentration.
Ionic liquids (IL) are promising and eco-friendly candidates as CO2 absorbents, because of their unique properties, such as high thermal stability, negligible vapor pressure, and tunable structures. By finely tuning IL structures and properties, significant achievements could be made in designing ILs with impressive CO2 capacity.12–25 At the same time, thanks to their tunable structures and superior CO2 absorption ability, ILs can be used to realize CCU processes even under mild conditions.26–33 There have been many systematical reports focusing on the influence of anions in ILs, where the anion plays key roles in CCU processes as an absorbent and catalyst.34–38 However, few studies could be realized on the important role of the cation in CCU processes such as the synthesis of alkylidene cyclic carbonates.
Recently, carbonates have drawn much attention because of their potential bioactivities and widespread applications in Li-ion batteries and the pharmaceutical industry.39,40 Based on the principles of green and sustainable chemistry, the carboxylative cyclization of propargylic alcohols and CO2 is a promising route to obtain α-alkylidene cyclic carbonates.41,42 Till now, although numerous heterogeneous catalysts have been reported for this reaction,43–57 most of them suffered from harsh reaction conditions including high CO2 pressure, excessive catalyst loading and poor reusability of catalysts, which can be ascribed to the poor activity or low stability of catalysts. How to realise these processes efficiently at a low concentration of CO2 (such as 10%) is still a puzzle for researchers.
Herein, we developed a highly efficient catalytic system used for the reaction between CO2 and propargylic alcohols. ILs with different anions and cations were used as cocatalysts (Fig. 1), in order to find out the effect of ILs on this reaction. The results indicated that both the cation and anion played a key role. Therefore, a dual functionalized IL with hydroxyl and azolate groups [Ch][Triz] was designed, which exhibited excellent activity and good reversibility in this reaction. It is worth mentioning that under 10% CO2, the catalytic system (AgNO3/[Ch][Tri]) showed excellent catalytic activity even in a gram-scale reaction.
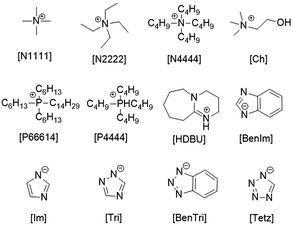 |
| Fig. 1 Structures of the cation and anion of the IL used in this work. | |
Experimental
Materials and methods
All chemicals used in this work were commercial available and used without further purification unless otherwise stated. 3-Methyl-1-pentyn-3-ol, 2-phenyl-3-butyn-2-ol and 1-ethynyl-cyclopentanol were purchased from J&K Scientific Ltd. 2-Methyl-3-butyn-2-ol was purchased from Aladdin Ind. Co., Ltd. 1H-Tetrazole (Tetz), 1H-benzotriazole (Bentri), 1,2,4-triazole (Triz), benzimidazole (BenIm), tetrabutylammonium hydroxide ([N4444][OH]), imidazole (Im), tetraethylammonium hydroxide ([N2222][OH]), tetramethylammonium hydroxide ([N1111][OH]), choline (Ch) and 1-ethynyl-1-cyclohexanol were purchased from Energy-Chemistry Co., Ltd. CO2 (99.99%), CO2 (10%) and N2 (99.99%) were purchased from Hangzhou Jingong Special Gas Co., Ltd. 1H NMR and 13C NMR spectra were recorded on a Bruker spectrometer (400 MHz) in DMSO-d6, which was obtained from Adamas-beta, with DMSO as the standard.
The synthesis of functionalized ILs
These functionalized ILs were easily prepared by the neutralization of superbases or the solution of [N1111]OH, [N2222]OH, [N4444]OH, [P4444]OH or choline with azole compound. In a typical example, the reaction mixture was stirred at 50 °C for 6 h and then the vacuum-rotary evaporation procedure was applied to remove most of the water. After that, ILs were dried under vacuum at 80 °C for more than 24 hours to reduce the possible traces of water. Before use, the water content of ILs was determined by Karl Fisher titration and found to be less than 0.1 wt%.
General procedure for the synthesis of alkylidene carbonates
As a typical example, the procedure using 2-methyl-3-butyn-2-ol (1a) as a typical substrate is described. AgNO3 (0.066 g, 0.4 mmol), [Ch][Triz] (0.138 g, 0.8 mmol), and 1a (0.336 g, 8 mmol) were added into a Schlenk flask, connected with a CO2 balloon filled with CO2 (99.99%). Then the reaction mixture was stirred at 30 °C for 24 h. The conversion and yield of product 1b were quantitatively analyzed by gas chromatography (GC) with biphenyl as the internal standard. To investigate the reusability of the catalyst system, the reaction mixture was extracted with n-hexane (4 × 15 mL). The upper layer was collected and the solvent was removed to obtain the product, and the lower layer, composed of AgNO3 and [Ch][Triz], was dried in a vacuum at 60 °C for the next cycle.
Results and discussion
At first, various catalysts were applied to the reaction; the results are listed in Table S1.† The effect of different metal catalysts on this reaction was obvious, where AgNO3 and AgOAc catalysts displayed better catalytic reactivity. Considering the basicity of AgOAc that may affect the catalytic reactivity of ILs, we chose AgNO3 for further research.
The effect of the cation on catalytic activity was investigated, which can be seen in Table 1. At first, the reaction could not take place with no IL as the cocatalyst (entry 1). When a protic IL ([DBU][Tri]) was used as the cocatalyst, the reaction did not occur. It was clear that the type of IL made a big difference. Then, a series of aprotic ILs with different kinds of cations were designed and prepared for the reaction. It was reported that the chain length of the cation affected its catalyst activity significantly. When aprotic ILs, [N1111][Tri], [N2222][Tri] and[N4444][Tri], were used as the cocatalyst, yields of 81%, 69% and 67% were obtained (Table 1, entries 3–5), respectively. In other words, the cation with a shorter chain length gave a higher yield of 2a in this reaction. We could come to a similar conclusion by comparing entry 5 and entry 6. Besides, [N4444][Tri] and [P4444][Tri] resulted in comparable yields of 67% and 64% (Table 1, entries 4 and 5), which indicated that the impact of the central atom of the cation was weak.
Table 1 The effect of the cation on catalytic activitya
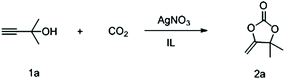
|
Entry |
Ionic liquid |
Conversionb [%] |
Yieldb [%] |
Reaction conditions: 1a (4 mmol), AgNO3 (0.4 mmol), base (0.8 mmol) and CO2 (1 bar) for 24 h at 30 °C.
Determined by GC using the internal standard method.
Without AgNO3.
|
1 |
— |
— |
<1 |
2 |
[DBU][Tri] |
4 |
<1 |
3 |
[N1111][Tri] |
90 |
81 |
4 |
[N2222][Tri] |
82 |
69 |
5 |
[N4444][Tri] |
77 |
67 |
6 |
[P4444] [Tri] |
75 |
64 |
7 |
[P66614][Tri] |
70 |
59 |
8 |
[P66614][Tri] |
70 |
59 |
9c |
[Ch][Tri] |
<1 |
<1 |
Considering that the hydrogen bond played an important role in some CCU processes, a hydroxyl functionalized aprotic IL [Ch][Tri] was designed, prepared, and used in this reaction. The conversion and yield could be improved to 99% and 93% (Table 1, entry 8), respectively. These results indicated that the hydroxyl group of the cation could promote this reaction well. In a control experiment, no reaction proceeded without AgNO3 (Table 1, entry 9). Clearly, both AgNO3 and base (also called a co-catalyst) were indispensable for this reaction.
To investigate the influence of the basicity of the anion, the reaction was carried out using ILs with various basic anions, and the results are listed in Table 2. When [Ch][Cl] was used, only 4% yield of the product was obtained, which implied that the basicity was also significant for this reaction. By tuning the basicity of the anion, it was obvious that this reaction was affected by the strength of the base, where higher conversion was achieved when a stronger basic IL was used. As for the anion with weak basicity, like [Tetz] and [BenTri], the reaction was not complete within 24 hours, implying poor catalytic activity (entries 2 and 3). To our delight, [Tri], whose pKa was 18.6, could improve the conversion and yield to 99% and 93% (entry 4), respectively. However, strongly basic ILs, [Ch][BenIm] and [Ch][Im], resulted in much lower yields of the product than [Ch][Tri], due to the decreased selectivity (entries 5 and 6). Therefore, a dual functionalized IL with a hydroxyl group and moderate basicity [Ch][Tri] exhibited the best catalytic activity. Besides, compared with other AgNO3-based systems, AgNO3/[Ch][Tri] exhibited excellent performance for CO2 utilization (Table S4†).
Table 2 The effect of different basic anions on catalytic activitya
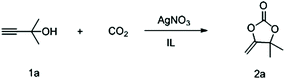
|
Entry |
Ionic liquid |
pKa of anionsb |
Conversionc [%] |
Yieldc [%] |
Reaction conditions: 1a (4 mmol), AgNO3 (0.4 mmol), base (0.8 mmol) and CO2 (1 bar) for 24 h at 30 °C.
Values in DMSO.
Determined by GC using the internal standard method.
|
1 |
[Ch][Cl] |
1.8 |
15 |
4 |
2 |
[Ch][Tetz] |
8.2 |
23 |
17 |
3 |
[Ch][BenTri] |
11.9 |
67 |
64 |
4 |
[Ch][Tri] |
13.9 |
99 |
93 |
5 |
[Ch][BenIm] |
18.2 |
97 |
67 |
6 |
[Ch][Im] |
18.9 |
99 |
64 |
Compared with other CO2 sources, capturing CO2 from flue gas is a major challenge due to its low concentration (∼10 vol%). Therefore, to further evaluate its practicability, the reaction was also carried out at a low concentration CO2 of 10%. To our delight, after extending the reaction time to 48 hours, this catalytic system composed of AgNO3 and [Ch][Tri] gave 2a in a yield of 90% after bubbling CO2 at 0.1 bar under 30 °C, even at a gram-scale (Fig. 2).
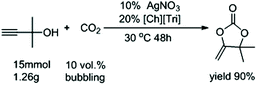 |
| Fig. 2 A gram-scale reaction under low concentration of CO2 using AgNO3 as the catalyst and [Ch][Triz] as the cocatalyst. | |
The reusability of the catalytic system is a critical property for CO2 utilization, which has a direct impact on the cost because it determined the frequency of the replacement of the catalyst. Therefore, we investigated the recycling performance of the AgNO3 and [Ch][Tri] catalytic system under the optimized reaction conditions. The results for 8 cycles are shown in Fig. 3. It was found that good catalytic activity was well-maintained during the 8 cycles.
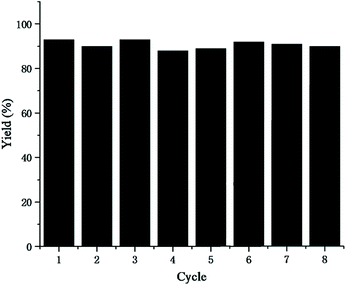 |
| Fig. 3 Recycling experiments using AgNO3 as the catalyst and [Ch][Triz] as the cocatalyst. | |
The substrate scope and generality of this reaction were investigated by using this dual functionalized catalytic system. As illustrated in Table 3, all of these substrates could react with CO2 under mild conditions, and good to excellent yields were obtained. With an ethyl group, 2b was given in a yield of 92% (entry 4). By extending the reaction time, a substrate with a large substituent group could also convert totally. The reactivity of phenyl substituted propargylic alcohol was investigated, and a 95% yield of 2c was obtained (entry 5). In addition, the reaction of 1d and 1f was found to occur smoothly with yields of 92% and 89%, respectively, which means that this dual functionalized IL also has a good catalytic activity on propargylic alcohol with a cycloalkane group.
Table 3 The synthesis of alkylidene carbonates from CO2 using [Ch][Tri]a
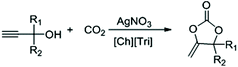
|
Entry |
Substrate |
R1 |
R2 |
T [h] |
Yieldb [%] |
Reaction conditions: Substrate (4 mmol), AgNO3 (0.4 mmol), [Ch][Tri] (0.8 mmol) and CO2 (1 bar) at 30 °C.
Determined by NMR using the internal standard method.
10 vol% of CO2.
15 vol% of CO2.
Isolated yield.
|
1 |
1a
|
Me |
Me |
24 |
93 |
2 |
1a
|
Me |
Me |
48 |
85 |
3 |
1a
|
Me |
Me |
48 |
87 |
4 |
1b
|
Me |
Et |
24 |
92 |
5 |
1c
|
Me |
Ph |
36 |
95 (87)e |
6 |
1d
|
–(CH2)5– |
36 |
92 |
7 |
1f
|
–(CH2)4– |
30 |
89 |
On the basis of the experimental results and previous literature reports, we proposed a plausible mechanism for this reaction (Fig. 4). Firstly, the carbon–carbon triple bond on the substrate 1a could be activated by silver ions (AgNO3). Meanwhile, [Tri] had a sufficient basicity to extract the proton from the hydroxyl group on 1a to form intermediate I, and the hydroxyl group on [Ch] could stabilize the intermediate I through intermolecular hydrogen bonding. Subsequently, intermediate II was obtained by intermediate I nucleophilic attacked CO2, which was captured by [Tri]. Finally, 2a was generated through an intramolecular ring-closing reaction in II, and silver ions and [Ch][Tri] were regenerated. With this proposed mechanism, ILs acted as a CO2 absorbent and a base at the same time. As a CO2 absorbent, it can be seen that ILs with higher basicity could capture more CO2, while the strong interaction between ILs and CO2 would impede the reaction of CO2 and propargylic alcohols. On the other hand, as a base, ILs with weak basicity were hard to initiate the reaction by abstracting hydrogen from propargylic alcohols.
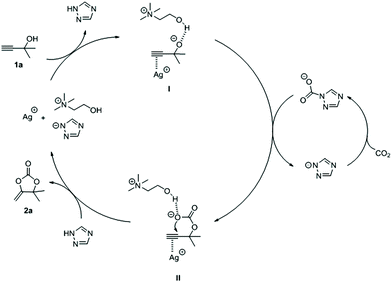 |
| Fig. 4 Possible mechanisms for this reaction. | |
Conclusions
In summary, an efficient catalytic system was developed for the reaction between CO2 and propargylic alcohols under mild conditions. We found that the effect of the cation on the reaction was significant, where the hydroxyl group on the cation could promote this reaction well. It was also found that the basicity of the anion was important to its activity, where the anion with moderate basicity gave the best activity. Therefore, the catalytic system comprising the dual functionalized IL [Ch][Tri] and AgNO3 exhibited excellent reusability and generality. To be specific, this catalytic system showed excellent performance in a gram-scale reaction even under low concentration of CO2. We believe that this method developed by us through tuning both the cation and the anion is not only important for CCU processes, but also for gas separation.
Conflicts of interest
There are no conflicts to declare.
Acknowledgements
We acknowledge the support of the National Natural Science Foundation of China (21776239), the Zhejiang Provincial Natural Science Foundation of China (LZ17B060001), and the State Key Laboratory of Chemical Engineering (No. SKL-ChE-20T05).
Notes and references
- J. Y. Hu, J. Ma, Q. G. Zhu, Z. F. Zhang, C. Y. Wu and B. X. Han, Angew. Chem., Int. Ed., 2015, 54, 5399–5403 CrossRef CAS.
- M. D. Porosoff, B. H. Yan and J. G. G. Chen, Energy Environ. Sci., 2016, 9, 62–73 RSC.
- T. Sakakura, J. C. Choi and H. Yasuda, Chem. Rev., 2007, 107, 2365–2387 CrossRef CAS.
- Z. Z. Yang, Y. N. Zhao and L. N. He, RSC Adv., 2011, 1, 545–567 RSC.
- Y. F. Zhao, Z. Z. Yang, B. Yu, H. Y. Zhang, H. J. Xu, L. D. Hao, B. X. Han and Z. M. Liu, Chem. Sci., 2015, 6, 2297–2301 RSC.
- Y. F. Zhao, B. Yu, Z. Z. Yang, H. Y. Zhang, L. D. Hao, X. Gao and Z. M. Liu, Angew. Chem., Int. Ed., 2014, 53, 5922–5925 CrossRef CAS.
- J. P. Hallett and T. Welton, Chem. Rev., 2011, 111, 3508–3576 CrossRef CAS.
- F. Maier, Angew. Chem., Int. Ed., 2011, 50, 10133–10134 CrossRef CAS.
- W. Clegg, R. W. Harrington, M. North and R. Pasquale, Chem. – Eur. J., 2010, 16, 6828–6843 CrossRef CAS.
- K. Motokura, S. Itagaki, Y. Iwasawa, A. Miyaji and T. Baba, Green Chem., 2009, 11, 1876–1880 RSC.
- Y. Yang, Y. Hayashi, Y. Fujii, T. Nagano, Y. Kita, T. Ohshima, J. Okuda and K. Mashima, Catal. Sci. Technol., 2012, 2, 509–513 RSC.
- E. D. Bates, R. D. Mayton, I. Ntai and J. H. Davis, J. Am. Chem. Soc., 2002, 124, 926–927 CrossRef CAS.
- F. F. Chen, K. Huang, Y. Zhou, Z. Q. Tian, X. Zhu, D. J. Tao, D. E. Jiang and S. Dai, Angew. Chem., Int. Ed., 2016, 55, 7166–7170 CrossRef CAS.
- G. K. Cui, J. J. Wang and S. J. Zhang, Chem. Soc. Rev., 2016, 45, 4307–4339 RSC.
- B. E. Gurkan, J. C. de la Fuente, E. M. Mindrup, L. E. Ficke, B. F. Goodrich, E. A. Price, W. F. Schneider and J. F. Brennecke, J. Am. Chem. Soc., 2010, 132, 2116–2117 CrossRef CAS.
- Y. J. Huang, G. K. Cui, Y. L. Zhao, H. Y. Wang, Z. Y. Li, S. Dai and J. J. Wang, Angew. Chem., Int. Ed., 2017, 56, 13293–13297 CrossRef CAS.
- M. D. Soutullo, C. I. Odom, B. F. Wicker, C. N. Henderson, A. C. Stenson and J. H. Davis, Chem. Mater., 2007, 19, 3581–3583 CrossRef CAS.
- C. M. Wang, X. Y. Luo, H. M. Luo, D. E. Jiang, H. R. Li and S. Dai, Angew. Chem., Int. Ed., 2011, 50, 4918–4922 CrossRef CAS.
- W. Z. Wu, B. X. Han, H. X. Gao, Z. M. Liu, T. Jiang and J. Huang, Angew. Chem., Int. Ed., 2004, 43, 2415–2417 CrossRef CAS.
- Y. S. Zhao, R. Gani, R. M. Afzal, X. P. Zhang and S. J. Zhang, AIChE J., 2017, 63, 1353–1367 CrossRef CAS.
- K. H. Chen, W. J. Lin, X. N. Yu, X. Y. Luo, F. Ding, X. He, H. R. Li and C. M. Wang, AIChE J., 2015, 61, 2028–2034 CrossRef CAS.
- K. H. Chen, G. L. Shi, X. Y. Zhou, H. R. Li and C. M. Wang, Angew. Chem., Int. Ed., 2016, 55, 14362–14366 Search PubMed.
- K. Huang, Y. L. Chen, X. M. Zhang, S. Xia, Y. T. Wu and X. B. Hu, Chem. Eng. J., 2014, 237, 478–486 CrossRef CAS.
- C. M. Wang, G. K. Cui, X. Y. Luo, Y. J. Xu, H. R. Li and S. Dai, J. Am. Chem. Soc., 2011, 133, 11916–11919 CrossRef CAS.
- K. Zhang, S. H. Ren, L. Y. Meng, Y. C. Hou, W. Z. Wu and Y. Y. Bao, Energy Fuels, 2017, 31, 1786–1792 CrossRef CAS.
- Z. Z. Yang, L. N. He, J. Gao, A. H. Liu and B. Yu, Energy Environ. Sci., 2012, 5, 6602–6639 RSC.
- P. G. Jessop, S. M. Mercer and D. J. Heldebrant, Energy Environ. Sci., 2012, 5, 7240–7253 RSC.
- X. B. Lu, L. Shi, Y. M. Wang, R. Zhang, Y. J. Zhang, X. J. Peng, Z. C. Zhang and B. Li, J. Am. Chem. Soc., 2006, 128, 1664–1674 CrossRef CAS.
- J. Liu, W. M. Ren, Y. Liu and X. B. Lu, Macromolecules, 2013, 46, 1343–1349 CrossRef CAS.
- W. M. Ren, G. P. Wu, F. Lin, J. Y. Jiang, C. Liu, Y. Luo and X. B. Lu, Chem. Sci., 2012, 3, 2094–2102 RSC.
- D. Z. Xiong, G. K. Cui, J. J. Wang, H. Y. Wang, Z. Y. Li, K. S. Yao and S. J. Zhang, Angew. Chem., Int. Ed., 2015, 54, 7265–7269 CrossRef CAS.
- W. H. Yao, H. Y. Wang, G. K. Cui, Z. Y. Li, A. L. Zhu, S. J. Zhang and J. J. Wang, Angew. Chem., Int. Ed., 2016, 55, 7934–7938 CrossRef CAS.
- Z. J. Guo, Q. W. Jiang, Y. M. Shi, J. Li, X. N. Yang, W. Hou, Y. Zhou and J. Wang, ACS Catal., 2017, 7, 6770–6780 CrossRef CAS.
- X. F. Liu, X. Y. Li, C. Qiao, H. C. Fu and L. N. He, Angew. Chem., Int. Ed., 2017, 56, 7425–7429 CrossRef CAS.
- Y. F. Zhao, B. Yu, Z. Z. Yang, H. Y. Zhang, L. D. Hao, X. Gao and Z. M. Liu, Angew. Chem., Int. Ed., 2014, 53, 5922–5925 CrossRef CAS.
- M. Hulla, S. Chamam, M. A. G. Laurenczy, S. Das and P. J. Dyson, Angew. Chem., Int. Ed., 2017, 56, 10559–10563 CrossRef CAS.
- K. H. Chen, G. L. Shi, W. D. Zhang, H. R. Li and C. M. Wang, J. Am. Chem. Soc., 2016, 138, 14198–14201 CrossRef CAS.
- K. H. Chen, G. L. Shi, R. N. Dao, K. Mei, X. Y. Zhou, H. R. Li and C. M. Wang, Chem. Commun., 2016, 52, 7830–7833 RSC.
- A. A. Shaikh and S. Sivaram, Chem. Rev., 1996, 96, 951–976 CrossRef CAS.
- T. Sakakura and K. Kohno, Chem. Commun., 2009, 1312–1330 RSC.
- X. B. Lu, R. He and C. X. Bai, J. Mol. Catal. A: Chem., 2002, 186, 1–11 CrossRef CAS.
- Y. Du, F. Cai, D. L. Kong and L. N. He, Green Chem., 2005, 7, 518–523 RSC.
- Y. Sugawara, W. Yamada, S. Yoshida, T. Ikeno and T. Yamada, J. Am. Chem. Soc., 2007, 129, 12902–12903 CrossRef CAS.
- W. Yamada, Y. Sugawara, H. M. Cheng, T. Ikeno and T. Yamada, Eur. J. Org. Chem., 2007, 2604–2607 CrossRef CAS.
- Z. Z. Yang, Y. F. Zhao, H. Y. Zhang, B. Yu, Z. S. Ma, G. P. Ji and Z. M. Liu, Chem. Commun., 2014, 50, 13910–13913 RSC.
- Y. L. Gu, F. Shi and Y. Q. Deng, J. Org. Chem., 2004, 69, 391–394 CrossRef CAS.
- Y. Kayaki, M. Yamamoto and T. Ikariya, Angew. Chem., Int. Ed., 2009, 48, 4194–4197 CrossRef CAS.
- Y. B. Wang, Y. M. Wang, W. Z. Zhang and X. B. Lu, J. Am. Chem. Soc., 2013, 135, 11996–12003 CrossRef CAS.
- Y. B. Wang, D. S. Sun, H. Zhou, W. Z. Zhang and X. B. Lu, Green Chem., 2014, 16, 2266–2272 RSC.
- Y. Kayaki, M. Yamamoto and T. Ikariya, J. Org. Chem., 2007, 72, 647–649 CrossRef CAS.
- T. Kimura, K. Kamata and N. Mizuno, Angew. Chem., Int. Ed., 2012, 51, 6700–6703 CrossRef CAS.
- Q. W. Song, W. Q. Chen, R. Ma, A. Yu, Q. Y. Li, Y. Chang and L. N. He, ChemSusChem, 2015, 8, 821–827 CrossRef CAS.
- Q. W. Song, B. Yu, X. D. Li, R. Ma, Z. F. Diao, R. G. Li, W. Li and L. N. He, Green Chem., 2014, 16, 1633–1638 RSC.
- Q. W. Song and L. N. He, Adv. Synth. Catal., 2016, 358, 1251–1258 CrossRef CAS.
- Y. Yuan, Y. Xie, C. Zeng, D. D. Song, S. Chaemchuen, C. Chen and F. Verpoort, Green Chem., 2017, 19, 2936–2940 RSC.
- Y. Yuan, Y. Xie, C. Zeng, D. D. Song, S. Chaemchuen, C. Chen and F. Verpoort, Appl. Organomet. Chem., 2017, 31, e3867 CrossRef.
- Y. Yuan, Y. Xie, C. Zeng, D. D. Song, S. Chaemchuen, C. Chen and F. Verpoort, Catal. Sci. Technol., 2017, 7, 2935–2939 RSC.
Footnote |
† Electronic supplementary information (ESI) available: NMR, and Tables S1 and S2. See DOI: 10.1039/d0gc03510f |
|
This journal is © The Royal Society of Chemistry 2021 |