DOI:
10.1039/D4SC07817A
(Edge Article)
Chem. Sci., 2025,
16, 709-720
Regio- and diastereoselective synthesis of cyclobutylated phenothiazines via [2 + 2] photocycloaddition: demonstrating wavelength-gated cycloreversion inside live cells†
Received
18th November 2024
, Accepted 4th December 2024
First published on 13th December 2024
Abstract
Herein, we unveiled a regio- and diastereoselective synthesis of cyclobutylated phenothiazines, a unique class of structural congeners of phenothiazines via visible-light-irradiated intermolecular [2 + 2]-cycloaddition reaction, from readily available naphthoquinones, 2-aminothiophenols, and styrenes, either in a two-step or three-component coupling process. By varying substitutions in all three coupling partners, a library of cyclobutylated phenothiazines, including late-stage derivatization with five commercial drugs, has been realized with up to 97% isolated yield. In contrast to the reported pathways, the developed [2 + 2]-photocycloaddition seems to proceed via a ‘photoinduced-electron-transfer’ (PET) mechanism, which is well corroborated with the experimental observations, Rehm–Weller equation, and computation studies. Delightfully, a wavelength-gated reversibility of the [2 + 2]-photocycloaddition reaction has been accomplished on the synthesized cyclobutylated phenothiazines. By monitoring the rate of the cycloreversion reactions for different derivatives, a structure–activity relationship has also been achieved. Interestingly, this phenomenon was further replicated inside living cells, which leads to turn-on emission and is applied for photoresponsive cell imaging. This marks the first report of a light-triggered [2 + 2]-cycloreversion phenomenon occurring inside a live cell, leading to cell imaging. Moreover, the synthesized drug derivatives were utilized for synchronous cell imaging as well as drug delivery through the developed [2 + 2]-photocycloreversion process, which demonstrated the potential applicability of this class of molecules.
Introduction
Facile synthesis of strained carbocyclic motifs, especially cyclobutane scaffolds, has garnered substantial interest in the past few decades for their use as valuable intermediate and molecular building blocks for synthesizing various natural products and pharmaceuticals.1 Cyclobutanes, being the second most strained monocyclic alkanes in terms of total strain and strain per carbon atom,2 can undergo facile ring expansion or fragmentation, producing the desired or active molecular structure. On the other hand, phenothiazines are very well known to be a valuable core in several commercially available drugs, e.g., chlorpromazine, fluphenazine, methylene blue, etc. (Fig. 1a).3 Since the pioneering discovery of phenothiazine in 1883 by Bernthsen, numerous derivatives of it have been synthesized to date and widely acknowledged for their remarkable bioactivity as well as extensive applications.4 Phenothiazine drugs not only have revolutionized the realm of psychiatric medicines5 but also exhibit significant contributions as antihistamines and antiemetics.6 Due to this, the WHO has enlisted three phenothiazine drugs as essential medicines.7
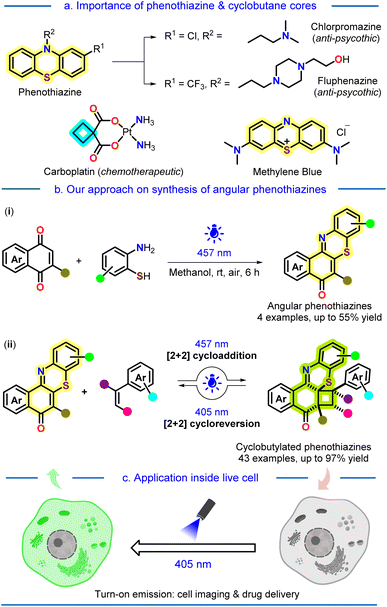 |
| Fig. 1 (a) Importance of phenothiazine and cyclobutane cores; (b) our approach for synthesizing cyclobutylated phenothiazines via the light-irradiated pathway; (c) wavelength-gated [2 + 2] cycloreversion of the synthesized cyclobutylated phenothiazines inside live cells, utilized for cell imaging and drug delivery. | |
In recent years, photocatalysis has increasingly become a valuable and potent technique for constructing diverse molecular assemblies that were previously challenging using conventional methods.8 The greener, environmentally benign, and milder approach of this method facilitates the rapid construction of intricate molecular frameworks with reduced side products, thereby enhancing atom and step economy. In this context, various research groups across the world are utilizing photo-irradiated strategies for carbon–carbon,9 and carbon–heteroatom10 bond formation to access valuable molecules. Recently, the trend for monitoring a light-induced chemical reaction at the cellular level has gained significant attention as this provides an appropriate insight into drug delivery and the mode of action of molecules for biological applications.11 In this context, the cellular localization of a light-mediated chemical phenomenon is an attractive approach to manipulate and study subcellular events with high spatiotemporal resolution.
Our group is actively engaged in the development of organochalcogen (chalcogens = S, Se, and Te) chemistry, mainly accessing newer methodologies, preparing novel molecular architectures, and exploring their catalytic applications.12
In the last few years, we also have embarked on the light-driven construction of biologically relevant value-added molecules,13 where very recently C–H-acylation, -amination, and -alkylation of naphthoquinones have been achieved by utilizing alcohols,13c amines,13d and even alkanes.13e
In this regard, we hypothesized whether we could make a unique conjuncture between the two structurally valuable cores: sulfur-heterocycle phenothiazine and cyclobutane, via a mild and sustainable light-driven protocol. The aim behind this hypothesis was to utilize this unique conjuncture for further cellular applications, as our laboratory is focused on synthesizing various small molecule fluorophores and utilizing them as markers for the bio-imaging of live cells.14 Although phenothiazines are widely explored, their structural congener angular phenothiazines are underexplored despite their reported bioactivity.15
To our delight, at first, we successfully synthesized the angular phenothiazine 1 utilizing a visible-light-irradiated method by oxidative dehydrogenative coupling of C–H and S–H bonds of 1,4-quinones and aminothiophenols using aerial oxygen as an oxidant (Fig. 1b, eqn (i)). Finally, the synthesized angular phenothiazines 1, upon light irradiated [2 + 2]-cycloaddition with styrenes, provided the desired cyclobutylated phenothiazines 2 (Fig. 1b, eqn (ii)) with excellent regio- & diastereoselectivity. A ‘photoinduced electron transfer’ (PET) mechanism has been proposed for the cycloaddition reaction, which is well corroborated with experimental observations as well as Density Functional Theory (DFT) studies. Later, we established a wavelength-gated reversibility in the synthesized cyclobutylated phenothiazines based on the subsequent photophysical studies and HPLC analysis. Further, this wavelength-gated photocycloreversion phenomenon was utilized for turn-on emission-mediated photoresponsive cell imaging and drug delivery for the first time (Fig. 1c).
Results and discussion
Initially, we sought to synthesize the angular phenothiazine 1a through a conventional heating method using 1,4-naphthoquinone and 2-aminothiophenol via dehydrogenative oxidative coupling. We conducted the reactions using oxidants, namely, Cu(OAc)2 and atmospheric oxygen, in the presence of solvents like DMSO and DMF. However, the desired transformation could not be achieved even by using conventional coupling between 2-bromo-1,4-naphthoquinone and 2-aminothiophenol. Next, by recognizing the exceptional reactivity of quinones under light-irradiated conditions,13c–e,16 we sought to utilize the same in our quest to realize the angular phenothiazine 1a.
Gratifyingly, under blue-light-irradiation (λmax = 457 nm), the reaction of 1,4-naphthoquinone and 2-aminothiophenol yielded the angular phenothiazine 1a in 40% yield in acetone under the aerial atmosphere.
Better yields (51 and 55%) of 1a were realized in acetonitrile and methanol, keeping the light source unaltered (Scheme 1a, eqn (i)). 2-Amino-4-(trifluoromethyl)benzenethiol was also reacted smoothly with 1,4-naphthoquinone to enable trifluoromethyl-substituted angular phenothiazine 1ac (see ESI, Page S6†). The coupling of 1,4-anthraquinone with 2-aminothiophenol occurred sluggishly to afford the respective angular phenothiazine 1ad and was used further without any characterization (see ESI, Page S7†). The α,β-unsaturated double bond was tri-substituted in both 1ac and 1ad. To achieve a tetra-substituted derivative, 2-methylnaphthalene-1,4-dione was coupled with 2-aminobenzenethiol to afford 1ae (see ESI, Page S7†).
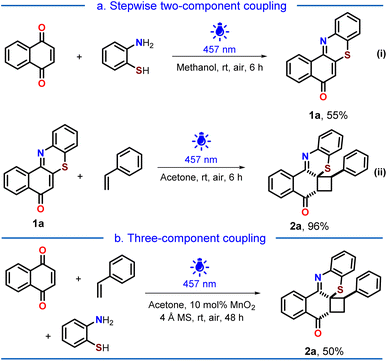 |
| Scheme 1 Synthesis of the cyclobutylated phenothiazine 2a: (a) stepwise two-component coupling (eqn (i) and (ii)); (b) three-component coupling. | |
The intermolecular [2 + 2] photocycloaddition between 1a and styrene yielded the desired cyclobutylated phenothiazine 2a in 96% isolated yield (Scheme 1a, eqn (ii)). Observing similar light-irradiated conditions for both steps, we were curious whether the cyclobutylated phenothiazine 2a could be achieved via a three-component coupling. Realizing that an oxidative dehydrogenative coupling was occurring between 1,4-naphthoquinone and 2-aminothiophenol in the first step, we perceived that some reactive oxygen species might be generated in the reaction mixture as side products, which might impede the second step. Therefore, to facilitate the second step, we planned to quench the in situ generated species by introducing a catalytic amount of MnO2 along with molecular sieves. Pleasingly, the desired cycloadduct 2a was obtained in 50% yield from a three-component coupling process (Scheme 1b).
The regiochemistry and spatial arrangement of 2a were assigned by its single crystal XRD analysis (Fig. 2), which revealed that a strong intramolecular π–π stacking interaction (3.765 Å) is present between the phenyl rings of styrene and benzothiazine. This intramolecular π–π stacking seems to be responsible for the excellent regio- and diastereoselectivity of the cycloadducts.
 |
| Fig. 2 Single crystal X-ray structure of cyclobutylated phenothiazine 2a (CCDC no. 2332714). | |
Substrate scopes
Next, we proceeded to the substrate scope variations (Scheme 2). To our delight, o-, m- and p-halogenated, alkylated, alkoxylated, arylated, and disubstituted styrenes underwent the [2 + 2]-photocycloaddition reaction very smoothly to afford the corresponding cyclobutylated phenothiazines 2a–2v with excellent yields (82–97%). The 97% isolated yield of the cyclobutylated phenothiazine 2a on gram-scale synthesis demonstrated the practical applicability of the developed protocol. The α- and trans-β-methylated styrenes provided moderate yields of 2w and 2x, probably due to increased steric crowding around the reactive double bond (54% and 58%). A 30% yield of the desired cycloadduct 2y was obtained with trans-stilbene, while cis-stilbene led to only a trace amount of product 2z. The electron-deficient 2- and 4-vinyl pyridines also exhibited only traces of their respective products 2aa and 2ab. Noteworthily, styrenes with electron-withdrawing substituents were unreactive toward the photocycloaddition reaction. Next, we varied the angular phenothiazine counterpart by taking trifluoromethyl-substituted angular phenothiazine 1ac and 1,4-anthraquinone coupled angular phenothiazine 1ad (Scheme 2b and c). Both of them reacted smoothly with styrene to afford the corresponding cyclobutylated phenothiazines 2ac and 2ad in 90% and 69% yields, respectively.
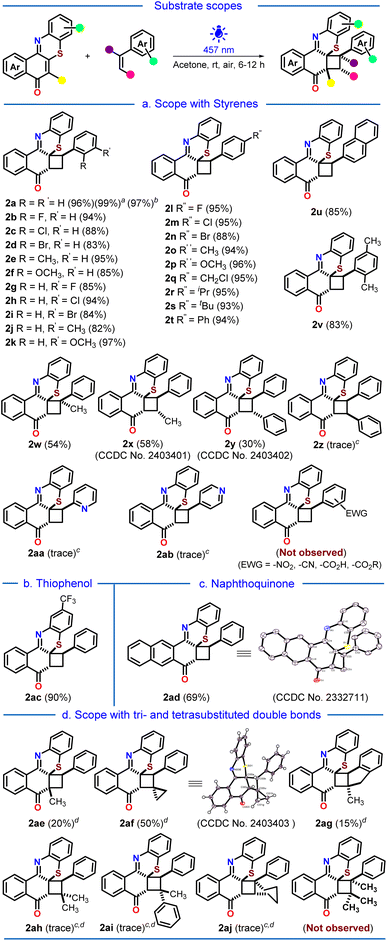 |
| Scheme 2 Substrate scopes for the cycloaddition reaction [angular phenothiazine (1.0 equiv., 200 mg), styrene (3 equiv.), acetone (3 mL), 6 h]. aNMR yield, bgram scale synthesis [1a (1.0 g, 3.8 mmol), 2a (1.3 mL, 11.4 mmol), acetone (10 mL), 6 h], ctraces of the product were characterized by mass spectrometry, dreaction time 12 h. | |
Thereafter, we proceed to investigate the scope of the developed [2 + 2] photocycloaddition reaction with tri- and tetrasubstituted double bond-containing precursors (Scheme 2d). When the tetrasubstituted phenothiazine 1ae was reacted with styrene, it produced the corresponding cycloadduct 2ae only in low yield (20%), presumably due to increased steric hindrance around the double bonds. We then explored the tri- and tetrasubstituted double bond containing styrenes in the photocycloaddition reaction. (Cyclopropylidenemethyl)benzene and 2-methyl-1H-indene provided the corresponding cycloadducts 2af and 2ag with moderate (50%) and low (15%) yields, respectively. However, trisubstituted styrenes with increased steric crowding around the double bond provided only traces of the corresponding products 2ah, 2ai, and 2aj. The tetrasubstituted styrenes did not participate in the cycloaddition reaction at all. Alleviation of ring strain in the cyclopropyl ring upon [2 + 2] photocycloaddition in the case of 2af might facilitate the reaction to provide comparatively better yield (50% vs. 20%-trace).
Moving forward, we proceed with the post-synthetic modification of our synthesized cyclobutylated phenothiazine 2a (Scheme 3a). Upon reduction with NaBH4, 2a gets reduced to phenothiazin-8-ol 2ak, which upon oxidation with m-CPBA results in the sulfoxide 2al with good yields. Lastly, we pondered upon the possibility of combining the cyclobutylated phenothiazine 2a with commercially available drug molecules (Scheme 3b). By following the reported procedures,17 4-chloromethyl styrene was coupled with five commercial drugs, namely, ibuprofen, flurbiprofen, gemfibrozil, clofibric acid, and fenbufen, to afford the corresponding drug-coupled styrenes.
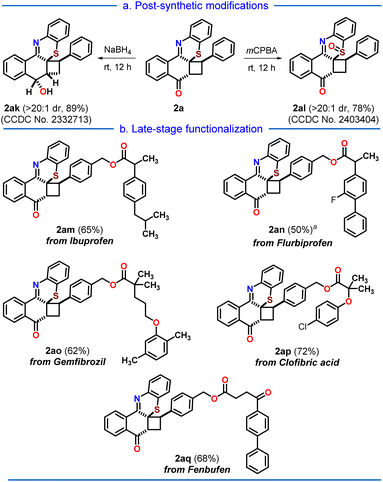 |
| Scheme 3 (a) Post-synthetic modifications, and (b) late-stage drug derivatization of the cycloadduct 2a; aNa2EosinY (10 mol%) was added. | |
To our delight, these drug-coupled styrenes reacted smoothly with the synthesized angular phenothiazine 1a under the developed [2 + 2] photocycloaddition reaction conditions, providing the late-stage functionalization of cyclobutylated phenothiazine (2am–2aq) in moderate to good (50–72%) yields.
Control experiments for mechanistic understanding
After synthesizing a library of cyclobutylated phenothiazines, we turned our focus on gaining insights into the mechanism of the reaction by performing some control experiments (Scheme 4). To understand whether the reaction is proceeding via a concerted pathway or via a stepwise mechanism, we have performed radical trapping/quenching experiments. In the presence of the radical trapper TEMPO, not only was the yield of the desired product decreased, but three TEMPO adducts were also realized through the mass spectrometry analysis (Scheme 4a). Also, radical scavenger TBHP led to a decrement in the yield to 19% of the product (Scheme 4b). These experiments indicated that our photocycloaddition is proceeding via a stepwise pathway involving radical intermediates. Next, we performed the photocycloaddition in the presence of triplet state quenchers 9,10-phenanthrenequinone and ferrocene having triplet energies >60 kcal mol−1 (styrene has a triplet energy of ∼60 kcal mol−1), which lowered the yield of the cyclobutylated phenothiazine to 22% and 15%, respectively, indicated that the reaction might proceeds via a triplet excited state (Scheme 4c).
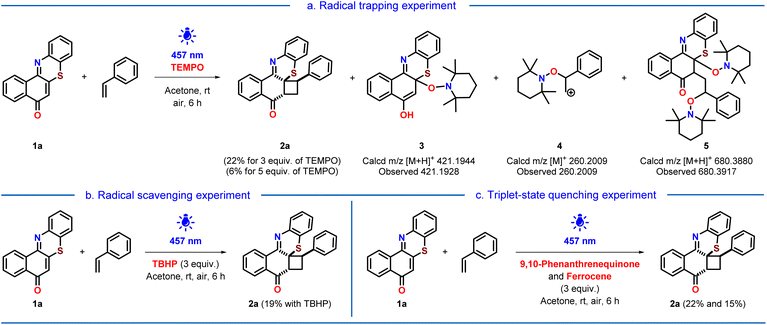 |
| Scheme 4 Control experiments in the presence of (a) TEMPO, (b) TBHP, and (c) 9, 10-phenanthrenequinone and ferrocene. | |
PET process verified using the Rehm–Weller equation
For the Rehm–Weller equation, the excited state redox potential of 1a and ground state oxidation potential of styrene were obtained from cyclic voltammetry, absorption, and emission studies (see ESI, Pages S38 and S39†). The more positive, excited-state redox potential
*Red1/2 value of angular phenothiazine 1a (+1.765 V) in comparison to the one-electron oxidation potential value of styrene [EOx1/2(D) = +1.1 V] strongly suggested that angular phenothiazine 1a, in its excited state, is capable of oxidizing the styrene. Further, the corresponding negative ΔGET value (−64.16 kJ mol−1) strongly suggested the feasibility of a photoinduced electron transfer (PET) process.
DFT computation
To gain further insight into the plausible mechanism of the [2 + 2] photocycloaddition, we have studied the molecular orbitals of excited state phenothiazine I (see ESI, Fig. S12 and Page S51†) and styrene (see ESI, Fig. S11 and Page S51†). The calculated energy difference between the SOMO of excited state phenothiazine I and the HOMO of styrene is +6.11 kcal mol−1. This indicated a feasible condition for electron transfer from styrene to excited state phenothiazine I under the blue-LED irradiation condition.
Photophysical investigations
Next, we turned our attention toward the photophysical investigations of synthesized molecules (see ESI, Fig. S5 and Page S31†). The synthesized angular phenothiazine 1a exhibited distinct absorption at 470, 364, and 310 nm. However, the cyclobutylated phenothiazine 2a provides a featureless broad absorption spectrum ranging from almost 322 nm to 455 nm with an absorbance maximum at 348 nm. Compared to the initial precursor of phenothiazines, namely, 1,4-naphthoquinone (λmax = 330 nm), the absorption maxima of both the angular phenothiazine 1a and cyclobutylated phenothiazine 2a were red-shifted; where, in the case of 1a, the red-shift is more prominent. Moreover, the angular phenothiazine 1a shows significant emissive properties in comparison to 1,4-naphthoquinone and cyclobutylated phenothiazine 2a.
Kinetic experiment of the [2 + 2] photocycloaddition reaction
To understand the kinetics of the photocycloaddition reaction, the blue light irradiated (λmax = 457 nm) reaction mixture of angular phenothiazine 1a and styrene was studied by UV-visible spectroscopy at different time intervals (Fig. 3). The absorption spectrum of the reaction mixture suggests that the angular phenothiazine 1a and styrene precursors were nearly consumed after 360 minutes, as the maximum absorption at 470 nm corresponding to precursor 1a gets saturated (Fig. 3a and b). This indicated that the reaction was completed within 360 minutes. A similar trend was also observed in emission spectra (see ESI, Fig. S8 and Page S35†).
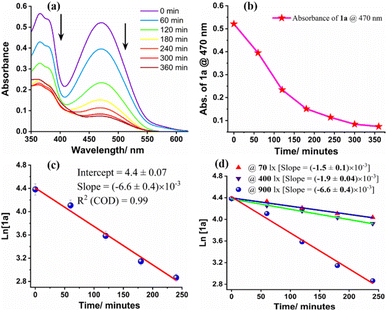 |
| Fig. 3 Kinetic monitoring of the [2 + 2] cycloaddition reaction between angular phenothiazine 1a and styrene: (a) time-dependent absorption spectra of the [2 + 2] photocycloaddition at 900 lx; (b) change in the absorbance at 470 nm of angular phenothiazine 1a with respect to time; (c) plot of Ln[1a] with respect to time for determining the rate of reaction at 900 lx; (d) rate of the [2 + 2] cycloaddition reaction at different incident light intensities (lx). These studies were performed at 80 µM concentration in acetonitrile. | |
Next, the rate of the [2 + 2] cycloaddition reaction was monitored by the UV-visible absorption, which indicated that the rate is linearly dependent on the concentration of angular phenothiazine 1a and styrene. It follows first-order kinetics with respect to both the reactants individually with a rate constant of (6.6 ± 0.4) × 10−3 min−1 (Fig. 3c). Further, the reaction rate was calculated from the respective rate constants for the initial 240 minutes of the reaction using the first-order rate law (see ESI, Pages S32 and S33†). The reaction rate for [2 + 2] cycloaddition was observed as 0.26 µM min−1 for the initial 240 minutes of the reaction.
Further, we have also investigated the dependency of the photocycloaddition reaction rate on the incident light intensity. For that, we conducted the reaction between angular phenothiazine 1a and styrene under optimized reaction conditions, at variable light intensities. The reactions were carried out up to saturation and the rate of each reaction was monitored up to 240 minutes (Fig. 3d).
At the optimal light intensity of 900 lx, the reaction rate was at its maximum (0.26 µM min−1), with a rate constant of (6.6 ± 0.4) × 10−3 min−1 (see ESI, Page S33 and S34†). As the incident photon concentration decreased, the reaction rate also gradually declined. At 400 lx, the rate was 0.12 µM min−1 with a rate constant of (1.9 ± 0.04) × 10−3 min−1, and at 70 lx, the rate was 0.097 µM min−1 with a rate constant of (1.5 ± 0.1) × 10−3 min−1. These results are suggestive of the dependency of rate law on the intensity of incident light.
Proposed mechanism
Based on the above experimental observations and DFT computation (see ESI, Pages S40–S60†), we proposed a PET mechanism for the cycloaddition reaction (Scheme 5). Upon light irradiation, phenothiazine 1a gets excited to produce the excited state phenothiazine I. The high oxidizing nature of the excited state phenothiazine I could facilitate the single electron transfer from styrene to the oxidizing excited state phenothiazine I to afford the radical–ion pair II. The electron transfer from styrene to the excited state phenothiazine moiety seems to be endergonic by 6.48 kcal mol−1 (0.28 eV). This is reasonably a feasible condition for photo-induced electron transfer. In the close proximity of the radical–ion pair II, the π–π stacking plays its role and helps in the regioselective formation of a C–C bond generating the 1,4-diradical III. This step is exergonic by −14.30 kcal mol−1 which indicates the feasibility of this path. Finally, the prompt radical recombination in III resulted in the desired cycloadduct 2a with excellent regio and diastereoselectivity.
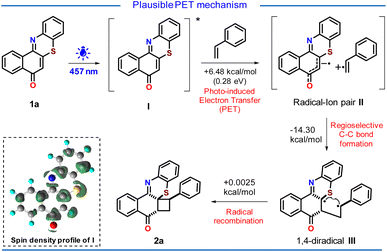 |
| Scheme 5 Plausible reaction mechanism of the [2 + 2] photocycloaddition. The calculated relative Gibb's free energy of the reaction (ΔG° in kcal mol−1) was obtained at a DFT-B3LYP/6-311+G(d,p)/CPCM(acetone) level of theory. | |
It is worth mentioning that the lower yield of the product in nonpolar solvents (see ESI, Table 1,† entry 4), traces of product formation with electron-deficient styrenes (2aa & 2ab), and the nonreactivity of styrenes with electron-withdrawing substituents support this PET process.
From both the SOMOs of radical ion pair II, it can be seen that one of the electrons is lying on the phenothiazine backbone and the other electron is lying on the styrene backbone (Fig. 4). Also from the electron density plot, we have observed that most of the electron density is lying over the phenothiazine moiety (see ESI, Fig. S13 and Page S52†).
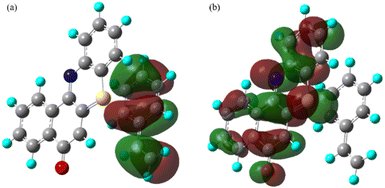 |
| Fig. 4 (a) SOMO and (b) SOMO+1, pictures of radical–ion pair II. | |
Here, it is worth noticing that the proposed PET process is in contrast to the literature precedences, where the [2 + 2] photocycloaddition between an enone and olefin is documented to proceed via an ‘energy transfer’ (EnT) process between an excited triplet-state enone and a ground-state olefin.18
Wavelength-gated [2 + 2] photocycloreversion of cyclobutylated phenothiazines
During these experiments, a color change was observed in cyclobutylated phenothiazine 2a upon exposure to light. Before exposure to any light irradiation, the colorless solution of 2a provides only a featureless broad absorption spectrum spanning from 322 nm to 455 nm (Fig. 5A, and ESI Fig. S5 and Page S31†). However, upon being subjected to an external laser source of 405 nm, the absorbance value of 2a gradually increases beyond 455 nm and reveals well-defined vibronic features (λmax = 470 nm). After 5 minutes of irradiation, the spectra became saturated, and the final spectrum perfectly mirrored the absorption spectrum of angular phenothiazine 1a. This reshaping of absorption spectra indicated that upon light exposure, the cyclobutylated phenothiazine 2a might be converting back to the angular phenothiazine 1avia the [2 + 2] photocycloreversion process. Also, irradiation on different concentrations of cyclobutylated phenothiazine 2a resulted in the complete conversion into angular phenothiazine 1a, which indicated that the [2 + 2] photocycloreversion phenomenon is concentration-independent (Fig. S26 and Page S69, ESI†).
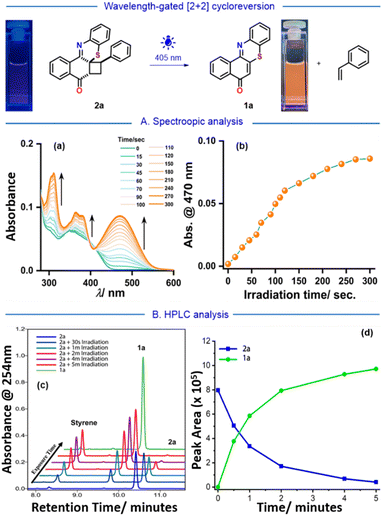 |
| Fig. 5 Wavelength-gated [2 + 2] cycloreversion of cyclobutylated phenothiazine 2a (10 µM in acetonitrile) upon irradiation with a 405 nm external laser source. (A) Spectroscopic analysis: (a) time-dependent UV-vis absorption spectra of 2a; and (b) corresponding OD values at various time intervals. (B) HPLC analysis: (c) time-dependent HPLC analysis; and (d) concentration of 2a and 1a in the reaction mixture at various time intervals. | |
To further authenticate this phenomenon, a high-performance liquid chromatography (HPLC) experiment was conducted at various time intervals (Fig. 5B). Initially, a solution of cyclobutylated phenothiazine 2a was examined to establish the peak retention time prior to any light exposure, revealing an isolated peak at 10.4 min. Exposure to the external laser source (405 nm) gave rise to two new peaks that precisely aligned with angular phenothiazine 1a (9.8 min) and styrene (8.2 min). After 5 minutes of light exposure, the intensified peaks of 1a and styrene, whereas the reduced peak of 2a nearly to the baseline, indicated the spectrum saturation (see ESI, Fig. S21, Pages S60 and S61†). Eventually, this HPLC study authenticated that the [2 + 2] photocycloreversion of the synthesized cyclobutylated phenothiazine 2a precisely produced back its precursors, namely, angular phenothiazine 1a and styrene, without formation of any side product.
Cell imaging and drug delivery by the developed wavelength-gated [2 + 2] photocycloreversion
Having these experimental results, we were intrigued by the prospect of replicating the developed light-triggered [2 + 2] cycloreversion phenomenon within live cells, aiming for cell imaging. To ascertain the feasibility of this process within biological systems, a similar light-triggered [2 + 2] cycloreversion reaction was performed in PBS (Fig. S27 and Page S69, ESI†).
To explore cell viability, we examined the cytotoxicity of both the synthesized angular phenothiazine 1a and cyclobutylated phenothiazine 2a probes using the MTT assay in live HeLa cells (Fig. 6). The results showed that more than 80% of the cells remained viable at 5 µM concentration of both probes, affirming their suitability for cell imaging. The green fluorescence emitting from all over the cells upon treatment with angular phenothiazine 1a indicated a good distribution of the probe throughout the cytoplasm. In contrast, the cyclobutylated phenothiazine 2a probe did not exhibit any significant fluorescence in the green channel under normal conditions.
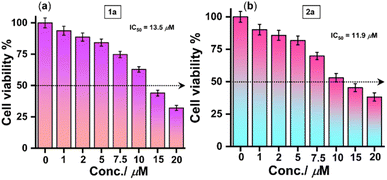 |
| Fig. 6 Cell viability assay in live HeLa cells for (a) angular phenothiazine 1a and (b) cyclobutylated phenothiazine 2a probes, respectively. | |
Next, to conduct the [2 + 2] cycloreversion experiment, HeLa cells were pre-treated with cyclobutylated phenothiazine 2a for 15 minutes, and subsequent images were acquired. As expected, the FITC channel does not show any fluorescence despite healthy cells being visualized from the DIC images (Fig. 7a). Surprisingly when cells in the same field of view (FOV) were irradiated for 30 seconds with an external laser source (405 nm), an intense green fluorescence was observed from all over the cells (Fig. 7b). The intensity became more prominent after 1 minute of irradiation (Fig. 7c). The merged images displayed how the cells glowed up after light irradiation. Therefore, considering all the experimental outcomes, we postulated that within the cellular environment as well, upon light-irradiation, the non-fluorescent cyclobutylated phenothiazine 2a undergoes a [2 + 2] cycloreversion to generate fluorescent angular phenothiazine 1a.
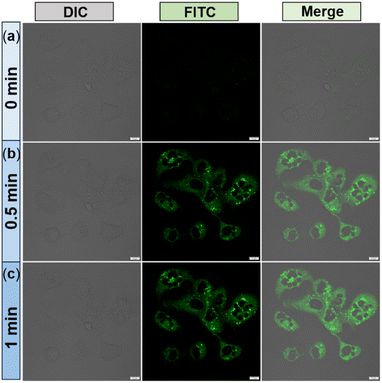 |
| Fig. 7 Confocal laser scanning microscopy (CLSM) images of HeLa cells after treatment with 5 µM of cyclobutylated probe 2a upon irradiation without (upper panel: (a)) and with (middle and lower panel: (b) and (c)) light. Scale bar = 10 µm. | |
It is worth mentioning that, to the best of our knowledge, this presents the first report of a light-triggered [2 + 2] photocycloreversion reaction occurring inside a cell, resulting in a remarkable turn-on emission that leads to photoresponsive live cell imaging.
Further, the internalization pathway of the angular phenothiazine 1a was investigated in order to understand how this probe stains the cytoplasm. As depicted in Fig. S29 (Page S70, ESI),† when HeLa cells were incubated at a low temperature, the fluorescence intensity coming from the cytoplasm was significantly reduced. This result suggests that the angular phenothiazine 1a probe might be penetrating the cells through an energy-dependent pathway.
To gain deeper insight into a particular endocytosis pathway involved in the internalization of 1a, several cellular uptake assays were carried out in the presence of various inhibitors such as chloroquine (CQ), chlorpromazine (CPZ), methyl-β-cyclodextrin (MβCD), amiloride hydrochloride (AMR) and cytochalasin-D (CyD). According to Fig. S29 and S30 (Pages S71, ESI),† there is no substantial alteration in the fluorescence intensity when cells were treated with CQ, CPZ, MβCD, AMR, and CyD compared to the control group. This suggested that the endocytosis pathway of the angular phenothiazine 1a is independent of autophagic flux, clathrin, and lipid raft, as well as micropinocytosis and phagocytosis through actin polymerization. The corresponding fluorescence intensities were plotted to better represent the effect of cellular internalization at a low temperature and in the presence of different inhibitors (Fig. S30, ESI†). It is evident from Fig. S30† that cellular uptake is majorly affected when the cells were kept at 4 °C for incubation.
All these results implied that cellular uptake of the angular phenothiazine 1a occurs through an energy-dependent pathway. Encouraged by these results, we next proceeded to investigate the structure–activity relationship of the synthesized cyclobutylated phenothiazines in terms of the rates of their cycloreversion reactions. Consequently, we have performed the [2 + 2] photocycloreversion reaction of the synthesized cycloadducts 2a–2af using the external laser source of 405 nm and monitored the corresponding time-dependent UV-visible absorption data (for a detailed discussion see ESI, Page S62–S66†). When ortho-, meta- and para-substituted cyclobutylated phenothiazines were compared, a general trend was observed that the ortho-substituted ones 2b, 2d, 2e, and 2f were exhibiting the highest rates while the meta-substituted cycloadducts 2l, 2n, 2o, and 2p were the slowest. Para-substituted cycloadducts 2g, 2i, 2j, and 2k showed rates in between ortho- and meta-ones (see ESI, Page S63†). Further, when the rates of the photocycloreversion of all the synthesized cycloadducts were compared, it was found that among all the cyclobutylated phenothiazines, the ortho-methyl substituted cyclobutylated phenothiazine 2e exhibited the highest rate (0.049 µM s−1), whereas the trans-stilbene derived cycloadduct 2y provided the slowest rate (0.016 µM s−1). As can be seen from Fig. 8, the rate of cycloreversion of 2e is three times higher in comparison to 2y. Subsequently, we proceeded to explore the structure–activity relationship within live HeLa cells and observed that in the case of 2e, no signal was detected from the cells prior to light irradiation (Fig. 9a). However, after 1 minute of irradiation, a significant enhancement in signal intensity was observed throughout the cells, which was visible in the FITC channel (Fig. 9b). On the other hand, when the cells were treated with 2y and subjected to photo-irradiation, no distinguishable signal was observed in the FITC channel after 1 minute of irradiation (Fig. 10a).
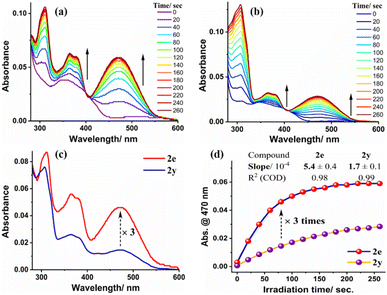 |
| Fig. 8 (a) Time-dependent UV-vis absorption spectra of 2e for photocycloreversion; (b) time-dependent UV-vis absorption spectra of 2y for photocycloreversion; (c) comparison of the absorption spectra of 2e and 2y after 80 seconds of photoirradiation: 2e exhibited three times higher absorbance than 2y; (d) comparison of the rates of [2 + 2] photocycloreversion of 2e and 2y after 80 seconds of photoirradiation: rate of 2e was three times higher than that of 2y. These studies were performed at 5 µM concentration in acetonitrile. | |
 |
| Fig. 9 Confocal laser scanning microscopy (CLSM) images of HeLa cells after treatment with 5 µM of probe 2e upon without irradiation (upper panel (a)) and with irradiation (lower panel (b)). | |
 |
| Fig. 10 Confocal laser scanning microscopy (CLSM) images of HeLa cells after treatment with 5 µM of probe 2y upon 1 min irradiation (upper panel (a)) and 5 min irradiation (lower panel (b)). | |
Even after 5 minutes of irradiation, only a very weak emission signal was detected in the FITC channel (Fig. 10b), suggesting that the stilbene-derived 2y undergoes cycloreversion at a slower rate than ortho-methylated 2e and it takes a longer time for cell imaging. Therefore, the cellular experimental observations were well corroborated with the corresponding spectroscopic evidence, demonstrating the structure–activity relationship.
Encouraged by this, we were curious to explore whether both cell imaging as well as drug delivery could be achieved, by replicating the photocycloreversion phenomenon with drug-coupled cyclobutylated phenothiazines (2am–2aq). Ibuprofen-coupled cyclobutylated phenothiazine 2am was taken as the representative probe, and its UV-visible absorption spectra revealed that upon light irradiation, it undergoes similar [2 + 2] cycloreversion (see ESI, Fig. S31 and Page S71†). Gratifyingly, within the HeLa cell as well, the probe was enabled for light-triggered cell imaging (see ESI, Fig. S32 and Page S72†), indicating the release of the ibuprofen moiety inside the cell. The further impact of the released drug is currently under investigation.
Conclusions
In summary, a mild and sustainable visible light irradiated protocol has been introduced to develop a new series of distinctly regio- and diastereoselective cyclobutylated phenothiazines from readily available naphthoquinones, 2-aminothiophenols, and styrenes either via a two-step or a one-pot-three-component coupling pathway. The developed photocycloaddition between the synthesized angular phenothiazines and styrenes seems to proceed via a PET mechanism, based on the substrate scope observations, experimental findings, and computational studies. A wavelength-gated [2 + 2] photocycloreversion was realized and elucidated by spectroscopic and HPLC analyses. We could successfully replicate this photocycloreversion phenomenon inside live cells, which leads to turn-on emission and is utilized for photoresponsive live cell imaging. Utilizing the synthesized drug-coupled cyclobutylated phenothiazines, we have achieved photoresponsive synchronous cell imaging and drug delivery, which could be further extended to theranostic types of applications. Moreover, by monitoring the photocycloreversion rates of various derivatives, a structure–activity relationship was established and further explored within live HeLa cells. To the best of our knowledge, this presents the first instance of a [2 + 2] photocycloreversion reaction occurring inside a cell, resulting in photoresponsive live cell imaging and drug delivery. Therefore, the presented work not only demonstrates its potential for exploring further theranostic types of applications but also opens up new avenues for developing innovative bio-probes.
Data availability
Detailed synthetic procedures, reaction condition optimization, data characterization, controlled experiments, X-ray diffraction structural analyses, and geometries and energies of theoretically investigated structures have been provided in the ESI.†
Author contributions
SK and SS designed the project and wrote the manuscript. All the compounds have been synthesized and characterized by SS and SM. Kinetic studies, mechanistic investigations, photophysical studies, structure–activity relationship and related optical spectroscopy experiments were done by SS. RKJ carried out DFT computation. Cells and related optical spectroscopy experiments have been designed by ALK and BC and performed by BC. The cell biology and related spectroscopy part were written by BC and ALK.
Conflicts of interest
The authors declare no conflicts of interest.
Acknowledgements
SK acknowledges DST-SERB (CRG/2023/002473), New Delhi, and IISER Bhopal for financial support. SS acknowledges DST-INSPIRE (IF170996) for the fellowship. BC and RKJ acknowledge IISER Bhopal for the fellowship. Dr Debasish Manna (IISER Bhopal) and Mr Pritam Kumar Pain (IISER Bhopal) are sincerely acknowledged for the HPLC experiment. Dr Sachin Dev Verma (IISER Bhopal) is sincerely acknowledged for his guidance in the light intensity-dependent kinetics study. Dr Saravanan Raju is sincerely acknowledged for his help with single-crystal analysis. Miss Nisha is acknowledged for helping in synthesizing a few compounds. Mr Svastik Jaiswal is acknowledged for the CV experiments. ALK thanks IISERB for the funding and infrastructural support. We also acknowledge the confocal facility at IISER Bhopal.
Notes and references
-
(a) J. D. Winkler, C. M. Bowen and F. Liotta, Chem. Rev., 1995, 95, 2003–2020 Search PubMed;
(b) E. Lee-Ruff and G. Mladenova, Chem. Rev., 2003, 103, 1449–1483 CrossRef CAS PubMed;
(c) J. C. Namyslo and D. E. Kaufmann, Chem. Rev., 2003, 103, 1485–1537 CrossRef CAS;
(d) P. Raster, S. Weiss, G. Hilt and B. König, Synthesis, 2011, 6, 905–908 Search PubMed;
(e) Y. Xu, M. L. Conner and M. K. Brown, Angew. Chem., Int. Ed., 2015, 54, 11918–11928 CrossRef CAS;
(f) S. K. Pagire, A. Hossain, L. Traub, S. Kerres and O. Reiser, Chem. Commun., 2017, 53, 12072–12075 RSC.
-
(a) N. L. Allinger, M. T. Tribble, M. A. Miller and D. H. Wertz, J. Am. Chem. Soc., 1971, 93, 1637–1648 CrossRef CAS;
(b) A. de Meijere, Angew Chem. Int. Ed. Engl., 1979, 18, 809–826 CrossRef;
(c) Cyclobutane-Physical Properties and Theoretical Studies: K. B. Wiberg in The Chemistry of Cyclobutanes, Part 1, ed. Z. Rappoport and J. F. Liebman, Wiley, Chichester, 2005, pp. 1–15 Search PubMed;
(d)
K. B. Wiberg, Z. Rappoport and J. F. Liebman, in The Chemistry of Cyclobutanes, John Wiley and Sons Inc, 2005 Search PubMed.
-
Phenothiazines, DrugBank Online: https://go.drugbank.com/categories/DBCAT000801.
-
(a) A. Bernthsen, Ber. Dtsch. Chem. Ges., 1883, 16, 2896–2904 CrossRef;
(b) K. Pluta, B. M. Młodawska and M. Jeleń, Eur. J. Med. Chem., 2011, 46, 3179–3189 CrossRef CAS PubMed ; In the treatment of prion diseases:;
(c) C. Korth, B. C. H. May, F. E. Cohen and S. B. Prusiner, Proc. Natl. Acad. Sci. U. S. A., 2001, 98, 9836 CrossRef CAS PubMed ; As antimalarial:;
(d) M. Kalkanidis, N. Klonis, L. Tilley and L. W. Deady, Biochem. Pharmacol., 2002, 63, 833 CrossRef CAS PubMed ; As antitubercular:;
(e) A. J. Warman, T. S. Rito, N. E. Fisher, D. M. Moss, N. G. Berry, P. M. O'Neill, S. A. Ward and G. A. Biagini, J. Antimicrob. Chemother., 2013, 68, 869 CrossRef CAS PubMed;
(f) D. Addla, A. Jallapally, D. Gurram, P. Yogeeswari, D. Sriram and S. Kantevari, Bioorg. Med. Chem. Lett., 2014, 24, 233 CrossRef CAS ; As cancer cell inhibitors:;
(g) X.-J. Dai, L.-J. Zhao, L.-H. Yang, T. Guo, L.-P. Xue, H.-M. Ren, Z.-L. Yin, X.-P. Xiong, Y. Zhou, S.-K. Ji, H.-M. Liu, H. -M. Liu, Y. Liu and Y.-C. Zheng, J. Med. Chem., 2023, 66, 3896–3916 CrossRef CAS ; As organic sensitizer:;
(h) H. Tian, X. Yang, R. Chen, Y. Pan, L. Li, A. Hagfeldt and L. Sun, Chem. Commun., 2007, 3741–3743 RSC;
(i) C.-J. Yang, Y. J. Chang, M. Watanabe, Y.-S. Hon and T. J. Chow, J. Mater. Chem., 2012, 22, 4040–4049 RSC ; Antioxidants in lubricants and fuels in petroleum industries:;
(j) C. M. Rarner, H. Murphy and N. L. Smith, Ind. Eng. Chem., 1950, 42, 2479 CrossRef ; As sensitizers for dye-sensitized solar cells:;
(k) A. F. Buene, E. E. Ose, A. G. Zakariassen, A. Hagfeldt and B. H. Hoff, J. Mater. Chem. A, 2019, 7, 7581–7590 RSC ; Miscellaneous:;
(l) R. Y. Lai, X. Kong, S. A. Jenekhe and A. J. Bard, J. Am. Chem. Soc., 2003, 125, 12631–12639 CrossRef CAS;
(m) D. Sun, S. V. Rosokha and J. Kochi, J. Am. Chem. Soc., 2004, 126, 1388–1401 CrossRef CAS PubMed;
(n) E. A. Weiss, M. J. Tauber, R. F. Kelley, M. J. Ahrens, M. A. Ratner and M. R. Wasielewski, J. Am. Chem. Soc., 2005, 127, 11842–11850 CrossRef CAS;
(o) H. W. Rhee, S. J. Choi, S. H. Yoo, Y. O. Jang, H. H. Park, R. M. Pinto, J. C. Cameselle, F. J. Sandoval, S. Roje, K. Han, D. S. Chung, J. Suh and J. I. Hong, J. Am. Chem. Soc., 2009, 131, 10107–10112 CrossRef CAS PubMed.
-
(a)
Phenothiazine antipsychotics, https://www.drugs.com/drug-class/phenothiazine-antipsychotics.html;;
(b)
How do phenothiazine antipsychotics work?, https://www.rxlist.com/antipsychotics_phenothiazine/drug-class.htm:.
-
(a)
Phenothiazine antiemetics, https://www.drugs.com/drug-class/phenothiazine-antiemetics.html;;
(b)
Phenergan, https://www.drugs.com/phenergan.html#side-effects.
-
WHO Model List of Essential Medicines – 23rd list, 2023.
-
(a) M. D. Kärkäs, ACS Catal., 2017, 7, 4999–5022 CrossRef;
(b) H. Yi, G. Zhang, H. Wang, Z. Huang, J. Wang, A. K. Singh and A. Lei, Chem. Rev., 2017, 117, 9016–9085 CrossRef CAS PubMed;
(c) Y. Sakakibara, E. Ito, T. Kawakami, S. Yamada, K. Murakami and K. Itami, Chem. Lett., 2017, 46, 1014–1016 CrossRef CAS;
(d) C. Song, X. Dong, Z. Wang, K. Liu, C.-W. Chiang and A. Lei, Angew. Chem., Int. Ed., 2019, 58, 12206–12210 CrossRef CAS;
(e) A. M. Nair, S. Kumar and C. M. R. Volla, Adv. Synth. Catal., 2019, 361, 4983–4988 CrossRef CAS;
(f) J. Kim, D. Kim and S. Chang, J. Am. Chem. Soc., 2020, 142, 19052–19057 CrossRef CAS PubMed;
(g) S. Wang, H. Wang and B. König, J. Am. Chem. Soc., 2021, 143, 15530–15537 CrossRef CAS PubMed;
(h) B. Maeda, G. Mori, Y. Sakakibara, A. Yagi, K. Murakami and K. Itami, Asian J. Org. Chem., 2021, 10, 1428–1431 CrossRef CAS;
(i) B. Maeda, Y. Sakakibara, K. Murakami and K. Itami, Org. Lett., 2021, 23, 5113–5117 CrossRef CAS PubMed;
(j) M. J. Genzink, J. B. Kidd, W. B. Swords and T. P. Yoon, Chem. Rev., 2022, 122, 1654–1716 CrossRef CAS PubMed;
(k) Y.-M. Tian, H. Wang, Ritu and B. König, Chem. Sci., 2022, 13, 241–246 RSC;
(l) Z. Yang, D. Yang, J. Zhang, C. Tan, J. Li, S. Wang, H. Zhang, Z. Huang and A. Lei, J. Am. Chem. Soc., 2022, 144, 13895–13902 CrossRef CAS PubMed;
(m) R. Rahaman, A. M. Nair and C. M. R. Volla, Chem.–Eur. J., 2022, 28, e202201290 CrossRef CAS PubMed;
(n) W. Liu, Y. Ke, C. Liu and W. Kong, Chem. Commun., 2022, 58, 11937–11940 RSC;
(o) S. K. Jana, M. Maiti, P. Dey and B. Maji, Org. Lett., 2022, 24, 1298–1302 CrossRef CAS PubMed;
(p) A. Ghosh, P. Pyne, S. Ghosh, D. Ghosh, S. Majumder and A. Hajra, Green Chem., 2022, 24, 3056–3080 RSC;
(q) P. Bellotti, H.-M. Huang, T. Faber and F. Glorius, Chem. Rev., 2023, 123, 4237–4352 CrossRef CAS;
(r) R. Kleinmans, S. Dutta, K. Ozols, H. Shao, F. Schäfer, R. E. Thielemann, H. T. Chan, C. G. Daniliuc, K. N. Houk and F. Glorius, J. Am. Chem. Soc., 2023, 145, 12324–12332 CrossRef CAS;
(s) P. Bellotti and F. Glorius, J. Am. Chem. Soc., 2023, 145, 20716–20732 CrossRef CAS;
(t) I. Halder, A. M. Nair and C. M. R. Volla, Chem. Commun., 2023, 59, 5862–5865 RSC;
(u) S. Gupta, A. Kundu, S. Ghosh, A. Chakraborty and A. Hajra, Green Chem., 2023, 25, 8459–8493 RSC;
(v) S. Ghosh, P. Pyne, A. Ghosh, S. Choudhury and A. Hajra, Org. Biomol. Chem., 2023, 21, 1591–1628 RSC;
(w) N. S. Shlapakov, A. D. Kobelev, J. V. Burykina, A. Y. Kostyukovich, B. König and V. P. Ananikov, Angew. Chem., Int. Ed., 2024, 63, e202314208 CrossRef CAS PubMed.
-
(a) W. Xie, S.-W. Park, H. Jung, D. Kim, M.-H. Baik and S. Chang, J. Am. Chem. Soc., 2018, 140, 9659–9668 CrossRef CAS PubMed;
(b) L. Niu, J. Liu, X.-A. Liang, S. Wang and A. Lei, Nat. Commun., 2019, 10, 467 CrossRef;
(c) P. Rai, K. Maji and B. Maji, Org. Lett., 2019, 21, 3755–3759 CrossRef CAS PubMed;
(d) S. Xu, H. Chen, Z. Zhou and W. Kong, Angew. Chem., Int. Ed., 2021, 60, 7405–7411 CrossRef CAS;
(e) Z. Guan, X. Zhong, Y. Ye, X. Li, H. Cong, H. Yi, H. Zhang, Z. Huang and A. Lei, Chem. Sci., 2022, 13, 6316–6321 RSC;
(f) W. Liu, C. Liu, M. Wang and W. Kong, ACS Catal., 2022, 12, 10207–10221 CrossRef CAS;
(g) K. Maji, P. R. Thorve, P. Rai and B. Maji, Chem. Commun., 2022, 58, 9516–9519 RSC;
(h) P. Rai, K. Maji, S. K. Jana and B. Maji, Chem. Sci., 2022, 13, 12503–12510 RSC;
(i) S. Laru, S. Bhattacharjee and A. Hajra, Chem. Commun., 2022, 58, 13604 RSC;
(j) S. Xu, Y. Ping, W. Li, H. Guo, Y. Su, Z. Li, M. Wang and W. Kong, J. Am. Chem. Soc., 2023, 145, 5231–5241 CrossRef CAS.
-
(a) S. Mukherjee, B. Maji, A. Tlahuext -Aca and F. Glorius, J. Am. Chem. Soc., 2016, 138, 16200–16203 CrossRef CAS;
(b) L. Niu, H. Yi, S. Wang, T. Liu, J. Liu and A. Lei, Nat. Commun., 2017, 8, 14226 CrossRef;
(c) E. Ito, T. Fukushima, T. Kawakami, K. Murakami and K. Itami, Chem, 2017, 2, 383–392 CrossRef CAS;
(d) I. Ghosh, J. Khamrai, A. Savateev, N. Shlapakov, M. Antonietti and B. König, Science, 2019, 365, 360–366 CrossRef CAS;
(e) W. Lee, H. J. Jeon, H. Jung, D. Kim, S. Seo and S. Chang, Chem, 2021, 7, 495–508 CrossRef CAS;
(f) S. Neogi, A. K. Ghosh, S. Mandal, D. Ghosh, S. Ghosh and A. Hajra, Org. Lett., 2021, 23, 6510–6514 CrossRef CAS PubMed;
(g)
Y. Sakakibara, K. Itami and K. Murakami, ChemRxiv, 2022, preprint, DOI:10.26434/chemrxiv-2022-drf9p;
(h) Z. Zhou, J. Kweon, H. Jung, D. Kim, S. Seo and S. Chang, J. Am. Chem. Soc., 2022, 144, 9161–9171 CrossRef CAS PubMed;
(i) W. Lee, D. Kim, S. Seo and S. Chang, Angew. Chem., Int. Ed., 2022, 61, e202202971 CrossRef CAS;
(j) A. Jati, K. Dey, M. Nurhuda, M. A. Addicoat, R. Banerjee and B. Maji, J. Am. Chem. Soc., 2022, 144, 7822–7833 CrossRef CAS PubMed;
(k) G. Tan, F. Paulus, A. Petti, M.-A. Wiethoff, A. Lauer, C. Daniliuc and F. Glorius, Chem. Sci., 2023, 14, 2447–2454 RSC;
(l) H. Wang, H. Shao, A. Das, S. Dutta, H. T. Chan, C. Daniliuc, K. N. Houk and F. Glorius, Science, 2023, 381, 75–81 CrossRef CAS PubMed;
(m) H. Wang, Z. Liu, A. Das, P. Bellotti, S. Megow, F. Temps, X. Qi and F. Glorius, Nat. Synth., 2023, 2, 1116–1126 CrossRef CAS;
(n) I. Ghosh, N. Shlapakov, T. A. Karl, J. Düker, M. Nikitin, J. V. Burykina, V. P. Ananikov and B. König, Nature, 2023, 619, 87–93 CAS;
(o) J. A. C. Delgado, Y.-M. Tian, M. Marcon, B. König and M. W. Paixão, J. Am. Chem. Soc., 2023, 145, 26452–26462 CrossRef CAS PubMed;
(p) S. Khan, A. M. Nair and C. M. R. Volla, Org. Chem. Front., 2023, 10, 157–162 RSC;
(q) A. Jati, S. Dam, S. Kumar, K. Kumar and B. Maji, Chem. Sci., 2023, 14, 8624–8634 RSC;
(r) A. K. Ghosh, S. Neogi, P. Ghosh and A. Hajra, Adv. Synth. Catal., 2023, 365, 2271–2278 CrossRef;
(s) H. Keum, H. Ryoo, D. Kim and S. Chang, J. Am. Chem. Soc., 2024, 146, 1001–1008 CrossRef CAS.
-
(a) D. H. M. Dam, J. H. Lee, P. N. Sisco, D. T. Co, M. Zhang, M. R. Wasielewski and T. W. Odom, ACS Nano, 2012, 6, 3318–3326 CrossRef CAS PubMed;
(b) I. Roy, S. Bobbala, J. Zhou, M. T. Nguyen, S. K. M. Nalluri, Y. Wu, D. P. Ferris, E. A. Scott, M. R. Wasielewski and J. F. Stoddart, J. Am. Chem. Soc., 2018, 140, 7206–7212 CrossRef CAS PubMed;
(c) I. Nakase, M. Miyai, K. Noguchi, M. Tamura, Y. Yamamoto, Y. Nishimura, M. Omura, K. Hayashi, S. Futaki, S. Tokonami and T. Iida, Nano Lett., 2022, 22, 9805–9814 CrossRef CAS.
-
(a) M. Batabyal, A. Upadhyay, R. Kadu, N. C. Birudukota, D. Chopra and S. Kumar, Inorg. Chem., 2022, 23, 8729–8745 CrossRef;
(b) S. Jain, S. S. Satpute, R. K. Jha, M. S. Patel and S. Kumar, Chem.–Eur. J., 2024, 30, e202303089 CrossRef CAS;
(c) M. Batabyal, S. Jaiswal, R. K. Jha and S. Kumar, J. Am. Chem. Soc., 2024, 146(1), 57–61 CrossRef CAS.
-
(a) S. Jana, A. Verma, R. Kadu and S. Kumar, Chem. Sci., 2017, 8, 6633–6644 RSC;
(b) V. Rathore and S. Kumar, Green Chem., 2019, 21, 2670–2676 RSC;
(c) R. K. Jha, A. Upadhyay, S. J. Kanika, K. A. Neena and S. Kumar, Org. Lett., 2022, 24, 7605–7610 CrossRef CAS;
(d) R. K. Jha, M. Batabyal and S. Kumar, J. Org. Chem., 2023, 88, 7401–7424 CrossRef CAS PubMed;
(e) R. K. Jha, K. Rohilla, S. Jain, D. Parganiha and S. Kumar, Chem.–Eur. J., 2024, 30, e202303537 CrossRef PubMed.
-
(a) S. Biswas, T. Dutta, D. Chopra, A. Silswal, R. Bhowal and A. L. Koner, Chem. Sci., 2021, 12, 9630–9644 RSC;
(b) T. Dutta, S. Das, I. Gupta and A. L. Koner, Chem. Sci., 2022, 13, 12987–12995 RSC;
(c) B. Chakraborty, S. Biswas, A. Pramanik and A. L. Koner, ACS Appl. Mater. Interfaces, 2022, 14, 55957–55970 CrossRef CAS PubMed;
(d) N. Dubey, S. Dhiman and A. L. Koner, ACS Appl. Nano Mater., 2023, 6, 4078–4096 CrossRef CAS.
- Reports on the synthesis of angular phenothiazines:
(a) C. O. Okafor, Tetrahedron, 1986, 42, 2771–2775 CrossRef CAS;
(b) W. B. Kang, S. Nańya, E. Maekawa and Y. Ueno, J. Heterocycl. Chem., 1988, 25, 113–117 CrossRef CAS;
(c) E. L. Ayuk, A. N. Njokunwogbu, S. U. Ilo, G. A. Engwa, U. C. Okoro and T. O. Oni, Am. J. Org. Chem., 2015, 5, 78–81 CAS;
(d) I. A. Ikenna, O. U. Chris and J. A. David, Chem. Mat. Res., 2015, 7, 144–146 Search PubMed;
(e) B. E. Ezema, C. G. Ezema, J. I. Ayogu, D. I. Ugwu and E. A. Onoabedje, Orient. J. Chem., 2015, 31, 379–385 CrossRef;
(f) E. A. Onoabedje, B. E. Ezema, S. A. Egu, F. U. Eze, C. U. Ibeji, F. N. Ibeanu and U. S. Oruma, Asian J. Chem., 2017, 29, 1–3 CrossRef CAS;
(g) Q. Zhang and G. Zhang, ChemistrySelect, 2021, 6, 4312–4318 CrossRef CAS ; Reports on cellular applications using angular phenothiazines:;
(h) C. O. Okafor, Dyes Pigm., 1986, 7, 249–287 CrossRef CAS;
(i) E. M. Odin, P. K. Onoja and J. F. Saleh, Int. J. Phys. Sci., 2013, 8, 1374–1381 CAS;
(j) E. U. Godwin-nwakwasi, U. C. Okoro, A. O. Ijeomah, I. Agbo and M. A. Ezeokonkwo, Asian J. Chem., 2017, 29, 742–748 CrossRef CAS;
(k) E. M. Odin, S. A. Egu and K. J. Okere, Pharm. Chem. J., 2020, 7, 43–53 CAS.
-
(a) J. M. Bruce and P. Knowles, J. Chem. Soc. C, 1966, 1627–1634 RSC;
(b) J. M. Bruce, D. Creed and J. N. Ellis, J. Chem. Soc. C, 1967, 1486–1490 RSC;
(c) J. M. Bruce and K. Dawes, J. Chem. Soc. C, 1970, 645–648 RSC;
(d) J. M. Bruce and D. Creed, J. Chem. Soc. C, 1970, 649–653 RSC;
(e) J. M. Bruce, D. Creed and K. Dawes, J. Chem. Soc. C, 1971, 3749–3756 RSC;
(f) J. M. Bruce and A.-u.-h. Chaudhry, J. Chem. Soc., Perkin Trans. 1, 1972, 372–379 RSC;
(g) J. M. Bruce and A.-u.-h. Chaudhry, J. Chem. Soc., Perkin Trans. 1, 1974, 295–297 RSC;
(h) F. Müh, C. Glöckner, J. Hellmich and A. Zouni, Biochim. Biophys. Acta, 2012, 1817, 44–65 CrossRef PubMed.
-
(a) For ibuprofen: H. Miao, M. Guan, T. Xiong, G. Zhang and Q. Zhang, Angew. Chem., Int. Ed., 2023, 62, e2022139 Search PubMed;
(b) For flurbiprofen, gemfibrozil, clofibric acid, and fenbufen: A. Granados, R. K. Dhungana, M. Sharique, J. Majhi and G. A. Molander, Org. Lett., 2022, 24, 4750–4755 CrossRef CAS PubMed.
-
(a) E. J. Corey, J. D. Bass, R. LeMahieu and R. B. Mitra, J. Am. Chem. Soc., 1964, 86, 5570–5583 CrossRef CAS;
(b)
Handbook of Synthetic Photochemistry, ed. A. Albini and M. Fagnoni, VCH, Weinheim, 2009 Search PubMed;
(c) S. Poplata, A. Tröster, Y.-Q. Zou and T. Bach, Chem. Rev., 2016, 116, 9748–9815 CrossRef CAS PubMed;
(d) H. Yu, S. Dong, Q. Yao, L. Chen, D. Zhang, X. Liu and X. Feng, Chem.–Eur. J., 2018, 24, 19361–19367 CrossRef CAS;
(e) T. Lei, C. Zhou, X.-Z. Wei, B. Yang, B. Chen, C.-H. Tung and L.-Z. Wu, Chem.–Eur. J., 2019, 25, 879–884 CrossRef CAS PubMed;
(f) D. Sarkar, N. Bera and S. Ghosh, Eur. J. Org Chem., 2020, 1310–1326 CrossRef CAS;
(g) D. K. Kölmel, A. S. Ratnayake, M. E. Flanagan, M. H. Tsai, C. Duan and C. Song, Org. Lett., 2020, 22, 2908–2913 CrossRef;
(h) F. M. Hörmann, C. Kerzig, T. S. Chung, A. Bauer, O. S. Wenger and T. Bach, Angew. Chem., Int. Ed., 2020, 59, 9659–9668 CrossRef PubMed;
(i) T. Rigotti, R. M. Ballesté and J. Alemán, ACS Catal., 2020, 10, 5335–5346 CrossRef CAS.
Footnotes |
† Electronic supplementary information (ESI) available: Synthetic procedures, spectroscopic data, theoretical analysis and crystallographic information. CCDC 2332714 (2a), 2332712 (2o), 2403401 (2x), 2403402 (2y), 2332711 (2ad), 2403403 (2af), 2332713 (2ak), and 2403404 (2al). For ESI and crystallographic data in CIF or other electronic format see DOI: https://doi.org/10.1039/d4sc07817a |
‡ Both contributed equally. |
|
This journal is © The Royal Society of Chemistry 2025 |
Click here to see how this site uses Cookies. View our privacy policy here.