DOI:
10.1039/D4RA08362H
(Review Article)
RSC Adv., 2025,
15, 3646-3663
Quinoline analogs: multifaceted heterocyclic compounds with varied synthetic strategies and potent antitubercular properties
Received
26th November 2024
, Accepted 20th January 2025
First published on 5th February 2025
Abstract
Tuberculosis cases have continuously increased by 64% over the last nine years, from 2014 to 2023. Approximately 33% of the global population is affected by TB. It is a bacterial disease, and Mycobacterium tuberculosis is the most common bacteria that affects the lungs of human beings during the infection. Other hazardous bacterial species causing tuberculosis are M. pinnipedii, M. canettii, M. caprae, M. bovis, M. africanum, and M. microti. TB symptoms in TB-infected patients include fever, chest pain, weight loss, and fatigue. Depending on the stage of infection, the treatment for TB can take approximately six months to two years. Quinoline comprises a pyridine ring fused with a benzene ring, and both these rings share two adjacent carbon atoms and can take part in electrophilic substitution reactions. Quinoline-based heterocyclic compounds are attracting substantial interest owing to their vital role as a class of synthetic and natural molecules. Quinoline and its derivatives display various biological activities, including anti-TB, anticonvulsant, antibiotic, antifungal, antimalarial, antipsychotic, antihypertensive, antileishmanial, antioxidant, tyrosine kinase inhibitory, anti-inflammatory, anticancer, anti-asthmatic, cardiotonic, anthelmintic, antiprotozoal, anti-HIV, and anti-Alzheimer effects. Some fused analogs of quinoline, such as graveolinine, ciprofloxacin, kokusaginine, bedaquiline, levofloxacin, moxifloxacin, and mefloquine, are commercially available as antitubercular drugs. There are various methods available to synthesize quinoline-containing antitubercular drugs. In this review paper, we present three types of synthetic methods in which substituted quinolines, substituted anilines, and miscellaneous starting materials are used and outline MIC values for all the synthesized compounds to signify their anti-TB activity.
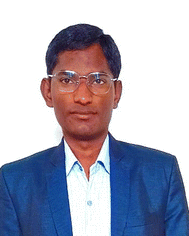 Rajendra Prasad Pakhariya | Mr Rajendra Prasad Pakhariya received his BSc (2018) and MSc (2020) degrees from Raj Rishi Bhartrihari Matsya University, Alwar, Rajasthan. Presently, he is Junior Research Fellow (CSIR UGC-NET) at the Department of Chemistry, Mohanlal Sukhadia University, under the supervision of Dr Gangotri Pemawat. His research is focused on synthetic organic chemistry, heterocyclic synthesis, green chemistry, and medicinal chemistry. |
 Ayushi Bhatnagar | Ms Ayushi Bhatnagar received her BSc (2013) and MSc (2018) degrees from the University College of Science, Mohanlal Sukhadia University, Udaipur, Rajasthan. Presently, she is pursuing a PhD under the supervision of Dr Gangotri Pemawat at the Department of Chemistry, Mohanlal Sukhadia University, Udaipur. She has published 10 articles in various national and international journals. Her research is focused on synthetic organic chemistry, heterocyclic synthesis, green chemistry, and medicinal chemistry. |
 Gangotri Pemawat | Dr Gangotri Pemawat is presently working as Assistant Professor at the Department of Chemistry, University College of Science, Mohanlal Sukhadia University (MLSU), Udaipur, Rajasthan. She completed her MSc in Organic Chemistry in 2007 from MLSU. She was awarded a Junior Research Fellowship (JRF) under CSIR and qualified for the GATE exam. She received her PhD in 2011 from MLSU and has published 17 research articles in various national and international journals. Additionally, she has contributed 10 book chapters in edited books. Her research interests lie in organic chemistry, heterocyclic chemistry, medicinal chemistry, and synthetic chemistry. She has handled two research projects sanctioned by the UGC and RUSA. She has more than thirteen years of teaching experience. |
1 Introduction
Tuberculosis (TB) is a disease involving lung infection1 and is one of the top ten causes of death globally.2 It can particularly arise in HIV patients, in whom the pathogenic alliance between HIV and TB can have severe consequences.3 Mycobacterium tuberculosis (MTB) is the main cause of TB, while some other hazardous species of bacteria that can also cause TB are M. caprae, M. pinnipedii, M. bovis, M. africanum, M. microti, and M. canetti.4 In 2023, India recorded a total of 2.31 lakh deaths due to TB. The country saw 2.43 million TB cases reported in 2022, while 2.23 million TB patients were documented by the end of November 2023. Over the past nine years, from 2014 to 2023, TB cases have steadily increased by 64% worldwide, with the maximum number of cases reported in China, Russia, and India.5 Around one-third of the global population is estimated to be infected with TB.6 The WHO is dedicated to eradicating TB worldwide and has included this aim as part of the SDGs, with a target to reduce TB mortality by 90% and its incidence by 80% by 2035.7
The TB infection process begins when an infected individual expels MTB-laden aerosols into the air, which are then inhaled by a new host, leading to bacterial deposition in their lungs. Upon entry, the innate immune system responds by recruiting macrophages to engulf the bacteria. The bacteria then multiply within the macrophages, prompting an inflammatory response that attracts immune cells, such as lymphocytes and neutrophils, to form an early-stage granuloma.8 As the granuloma matures, immune cells such as T cells, natural killer cells, dendritic cells, and fibroblasts contribute to its structure, surrounding the bacteria and containing the infection in a latent state, termed the “solid granuloma”. In some cases, when the immune system is weakened due to factors like HIV, malnutrition, or chronic stress, the granuloma breaks down, releasing bacteria into the surrounding tissues.9 This disintegration of the granuloma leads to caseous necrosis, resulting in the formation of a “caseous granuloma”, characterized by a cheese-like center. If left unchecked, the breakdown continues, causing further bacterial spread and symptomatic TB. Here, the immune system plays a balancing role; whereby a strong immune response keeps the bacteria contained within the granuloma, whereas a compromised immune system allows the granuloma to rupture, leading to active disease and dissemination of the bacteria throughout the body (Fig. 1).10
 |
| Fig. 1 Diagrammatic representation of the mechanism of tubercular activity. | |
Quinolines are bicyclic N-containing heterocyclic naturally occurring organic compounds, which serve as important segments of both synthetic and pharmacologically active compounds.11 Quinoline's ability to form salts with acids makes it a weak tertiary base, displaying electrophilic substitution reactions similar to pyridine and benzene.12 The logical design of small compounds that can target crucial MTB enzymes and pathways has attracted much attention due to the urgent need for new therapeutic medicines. Because of their wide range of biological actions and proven effectiveness in blocking important MTB targets, quinoline derivatives have become one of the most promising classes of such chemicals.13 These include enzymes that are essential for cell wall production, fatty acid metabolism, and DNA replication, such as decaprenylphosphoryl-beta-D-ribose 2′-epimerase (DprE1), enoyl-acyl carrier protein reductase (InhA), and DNA gyrase. By directly targeting the MTB ATP synthase, such as diarylquinoline, bedaquiline has demonstrated extraordinary efficacy and has been approved for treating multi-drug-resistant (MDR) TB. Molecular docking, structure-based drug design, and computer methods like QSAR modeling have all sped up the creation of new quinoline derivatives with enhanced pharmacokinetic, selectivity, and effectiveness profiles. Utilizing these developments, it is considered that quinoline-based drug discovery has enormous potential to provide safer and more efficient treatment choices while also addressing the problems caused by drug-resistant TB.14 An effective foundation for comprehending and maximizing the antitubercular potential of quinoline derivatives can be provided by the utilization of computational approaches. Through these, researchers can precisely understand the binding mechanisms of such drugs by modeling their interactions with important MTB targets, including DprE1, InhA, and DNA gyrase. Meanwhile, molecular dynamics simulations can allow evaluating the stability of ligand–protein complexes over time, guaranteeing their applicability in physiological settings, while molecular docking can assist in identifying crucial binding residues and quantifying interaction energies.15 Furthermore, in silico toxicity and ADMET predictions can expedite lead selection by guaranteeing that the drugs have advantageous pharmacokinetic characteristics. In addition to confirming the biological activity of quinoline derivatives, these computational approaches can also speed up the search for new candidates that are more effective against strains of MTB that are resistant to drugs.16 Several important proteins linked to TB have also been found through computational research, and these could be research targets for future medication development. Such enzymes include DNA gyrase, QcrB T313I, InhA, DprE1, the mycobacterial membrane protein large 3 (MmpL3), and the gyrase enzyme. Numerous quinoline derivatives have been demonstrated to bind to these proteins with differing affinities, offering important information about their possible medical uses.16 These interactions demonstrate the vital role that quinoline derivatives can play in developing TB treatment plans, especially when it comes to combating MDR bacteria.
Quinoline derivatives exhibit several biological activities and uses, including anti-bacterial,17 histamine H3 receptor antagonist,18,19 antibiotic,20 anti-inflammatory,21,22 antihypertensive,21 antileishmanial,23 anti-oxidant,24 tyrosine kinase inhibitors,25 antimalarial,26 anticancer,27 anti-HIV,28 anti-asthmatic,29 cardiotonic,30 anticonvulsant,31 anthelmintic,32 antifungal,33 antiprotozoals,34 anti-alzheimer,35 tyro kinase PDGF-RTK-inhibitor, agents for the treatment of lupus, antipsychotics,36 antiglaucoma, and local anesthetic.12 The structures of some important biologically active quinoline-containing drugs are presented in Fig. 2. TB is a highly challenging task in the medicinal field for human beings. Presently, various anti-TB new medicines are required that are more cost-effective, less toxic, affordable, simpler, and more potent than current anti-TB drugs, which are typically costly, toxic, and complex. Anti-TB drugs are generally classified into two categories: first-line drugs (i.e. rifampicin, pyrazinamide, and isoniazid) and second-line anti-TB drugs (i.e. gatifloxacin, levofloxacin, moxifloxacin, ciprofloxacin, ofloxacin, and trovafloxacin).37 Quinoline-based anti-TB drugs have both nonpolar and polar properties, which allow them to permeate bacterial cells.2 Bedaquiline is a vital anti-TB drug based on diarylquinoline. It is typically used in combination with rifampicin, pyrazinamide, and isoniazid as a highly effective therapy for the treatment of MDR-TB.7 It was first prepared by Janssen in 2005 (ref. 38) and later permitted by the US FDA and WHO in 2012. Some other important marketed anti-TB drugs bearing quinoline nucleus are gatifloxacin,39 ciprofloxacin,40 moxifloxacin,39,41 sparfloxacin, ofloxacin, enrofloxacin, levofloxacin,37 kokusaginine,42 lomefloxacin,43 mefloquine, and norfloxacin44 (Fig. 3).
 |
| Fig. 2 Biologically active agents bearing a quinoline nucleus. | |
 |
| Fig. 3 Quinoline-containing marketed anti-TB drugs. | |
The use of minimum inhibitory concentration (MIC) values has significantly enhanced the understanding of how to develop quinoline derivatives for more effective TB treatment. Modifications to the structure, such as the incorporation of alkene linkers and specific substituents, have proven crucial to enhancing the efficacy of synthesized compounds against TB. Research has indicated that the strategic placement of functional groups, like –F, –CF3,45 and azomethine (–C
N–)3 linkages, at a particular position on the quinoline nucleus can lead to varied inhibitor effects on MMTB. Furthermore, investigations into various quinoline hybrids, including quinoline–triazole,45 quinoline–tetrazole,3 oxazoloquinoline,45 quinoline–pyrazole,46 and trifluoromethyl-fused quinoline combinations, have yielded promising results.45 Quinoline hydrazones as well as fluorine-substituted hydrazones have been reported to have anti-TB activity with high potency compared to rifampicin and isoniazid.5 The pharmacological effectiveness and safety of quinoline derivatives in the treatment of TB are largely determined by their metabolic metabolism. The liver's cytochrome P450 enzymes are the main catalysts for the several biotransformation processes that these substances go through. Typical metabolic processes that increase their solubility and ease of excretion include hydroxylation, N-oxidation, and conjugation reactions, including glucuronidation and sulfation.47 The bioactivity of quinoline scaffolds is frequently influenced by the site-specific metabolism of their functional groups; metabolites may show more potency or less toxicity than the parent molecule. The addition of fluorine atoms or sterically hindered groups are examples of structural changes that can increase metabolic stability, delay fast degradation, and increase the drug's half-life.48 It was reported that expanding the ring size and incorporating a dimethylamine group within the quinoline ring could maintain strong potency. However, the introduction of highly polar substituents, such as N-methyl piperazine, and secondary amines, resulted in a significant reduction in anti-TB activity.49 Furthermore, the ADMET properties of quinoline derivatives can be studied in vitro and computationally, which offers important insights into their metabolic fate and aids in optimizing their pharmacokinetic profiles. Researchers can increase the therapeutic potential of these derivatives while reducing side effects by comprehending and modifying their metabolic activity.13 Quinoline Schiff base-derived metal complexes find diverse applications, including as an anti-TB antioxidant, catalyst for olefin polymerization, corrosion inhibitor in acidic conditions, anticancer and antimicrobial applications, and as a dye in solar cells. Lugosi et al. reported on BCG, which was derived from a virulent strain of M. Bovis and utilized as a vaccine for totally drug-resistant TB and MTB.3
2 Synthesis of quinoline-based anti-TB drugs
There are several classical named reactions for synthesizing quinoline derivatives, often using simple arylamines as starting materials. Here are few notable methods that involve arylamines: Reihm synthesis, Gould–Jacob reaction, Skraup synthesis, combes quinoline synthesis, Conrad–Limpach synthesis, and Doebner synthesis. In this review paper, we have classified the synthesis of quinoline derivatives into three categories based on their starting materials.
2.1. From substituted quinolines
Leveraging the inherent reactivity of quinoline derivatives to create novel compounds, this method often involves strategic modifications to the existing quinoline framework, allowing the introduction of various functional groups that can enhance biological activity. Through techniques such as cyclization, oxidation, or rearrangement, substituted quinolines can be transformed into more complex structures with diverse pharmacological properties. This synthetic strategy not only facilitates the exploration of structure–activity relationships but also contributes to an expansion of the range of novel therapeutic agents available, particularly for use in the fight against diseases like TB. The flexibility in substituent placement offers chemists the opportunity to tailor quinoline derivatives for specific applications, thus enlarging their utility in medicinal chemistry. Alegaon's team reported obtaining the target semicarbazones 4a–b in good yields from the reaction of 4,7-dichloroquinoline 1a with 4-amino acetophenone 2 in dry methanol to form the corresponding amino derivative, which was then treated with various aromatic aldehydes 3a–b in the presence of NaOH to get the relative chalcones through the Claisen–Schmidt mechanism. Compounds 4a–b were prepared by the reaction of these chalcones and semicarbazide hydrochloride in glacial acetic acid. Likewise, another semicarbazone 7 was synthesized by the reaction of 1a and 3-amino acetophenone 5 to produce the relative amino derivative. This derivative reacted with 4-methyl benzaldehyde 6 in the presence of NaOH to obtain the corresponding chalcone, which was treated with semicarbazide hydrochloride to produce compound 7 (Fig. 4). Excellent activity against MTB was exhibited by 4a, 4b, and 7 with MIC values of 1.56, 1.56, and 6.25 μM, respectively50 (Table 1). Similarly, Alcaraz and colleagues prepared Schiff bases of the 7-chloroquinoline-isoniazid hybrids 10a–b and 11a–d from 4-azido-7-chloroquinoline 1b and the N-(2-azidoalkyl)-7-chloroquinolin-4-amines 1c–d, respectively. Compounds 1b and 1c–d were treated with alkynes 8a–b in the presence of CuSO4·5H2O and sodium ascorbate, at room temperature for 3 h to produce the relative triazolyl quinolines, which were treated with isoniazid 9 in a microwave synthesizer at 110 °C in the presence of ethanol through a condensation reaction to form the corresponding compounds 10a–b and 11a–d. Additionally, the target compound 13 was prepared by substituted piperazin-1-yl-quinoline 1e, which was reacted with 2-azidobenzaldehyde 12 according to the above-mentioned process to produce the relative triazolyl quinoline, which was then treated with 9 in the microwave synthesizer in ethanol at 110 °C to form the desired compound 13 (Fig. 4). These compounds 10a–b, 11a–d, and 13 all showed excellent activity against MTB, with MIC values in the range of 0.25–0.50 μg mL−1 (ref. 51) (Table 1). In another investigation, Borsoi and teammates prepared 2,4,6-trisubstituted quinolines 15 and 16 with good yields by the nucleophilic substitution reaction of 6-methoxy-2-methylquinolin-4-ol 1f with 3,4-dichloro benzyl bromide 14 and 2-bromo-1-arylethanone, respectively, in K2CO3 as a base in DMF solvent at 25 °C (Fig. 5). These compounds were most potent against MTB, with MIC values of 0.3 and 1.3 μM (Table 1). The excellent activity of compound 15 was due to the existence of chloro groups at the 3 and 4 positions.52
 |
| Fig. 4 Synthesis of anti-TB quinoline analogs from substituted quinolines. | |
Table 1 MIC values and yields of quinoline derivatives for anti-TB activity
S. No. |
Compound |
MIC value |
Yield (%) |
S. No. |
Compound |
MIC value |
Yield (%) |
1 |
4a |
1.56 μM |
56 |
28 |
38a |
16 μg mL−1 |
33.3 |
2 |
4b |
1.56 μM |
51 |
29 |
38b |
16 μg mL−1 |
50 |
3 |
7 |
6.25 μM |
55 |
30 |
41a |
31.5 μM |
85 |
4 |
10a |
0.25 μg mL−1 |
74 |
31 |
41b |
34.8 μM |
88 |
5 |
10b |
0.5 μg mL−1 |
74 |
32 |
43a |
12.5 μg mL−1 |
93 |
6 |
11a |
0.5 μg mL−1 |
72 |
33 |
43b |
12.5 μg mL−1 |
80 |
7 |
11b |
0.5 μg mL−1 |
75 |
34 |
47a |
4 μg mL−1 |
54 |
8 |
11c |
0.5 μg mL−1 |
73 |
35 |
47b |
4 μg mL−1 |
56 |
9 |
11d |
0.25 μg mL−1 |
71 |
36 |
47c |
4 μg mL−1 |
51.4 |
10 |
13 |
0.25 μg mL−1 |
76 |
37 |
49 |
4.7 μg mL−1 |
90.14 |
11 |
15 |
0.3 μM |
63 |
38 |
53a |
1.6 μg mL−1 |
67 |
12 |
16 |
1.3 μM |
38 |
39 |
53b |
1.6 μg mL−1 |
70 |
13 |
18 |
0.07 μM |
69.8 |
40 |
53c |
1.6 μg mL−1 |
63 |
14 |
19 |
1.1 μM |
68 |
41 |
53d |
1.6 μg mL−1 |
61 |
15 |
21 |
0.90 μM |
84.81 |
42 |
53e |
1.6 μg mL−1 |
64 |
16 |
23 |
6.25 μg mL−1 |
84 |
43 |
53f |
1.6 μg mL−1 |
71 |
17 |
25 |
18.27 μM |
85 |
44 |
56a |
62.5 μg mL−1 |
78 |
18 |
27 |
15 μM |
91 |
45 |
56b |
62.5 μg mL−1 |
70 |
19 |
29 |
0.26 μg mL−1 |
84 |
46 |
56c |
25 μg mL−1 |
78 |
20 |
32 |
9.97 μM |
25 |
47 |
56d |
62.5 μg mL−1 |
75 |
21 |
35 |
0.24 μg mL−1 |
84 |
48 |
56e |
12.5 μg mL−1 |
48 |
22 |
37a |
3.12 μg mL−1 |
89 |
49 |
56f |
50 μg mL−1 |
80 |
23 |
37b |
1.60 μg mL−1 |
90 |
50 |
56g |
62.5 μg mL−1 |
78 |
24 |
37c |
1.60 μg mL−1 |
88 |
51 |
59a |
14.4 μg mL−1 |
68 |
25 |
37d |
3.12 μg mL−1 |
78 |
52 |
59b |
9.2 μg mL−1 |
65 |
26 |
37e |
1.60 μg mL−1 |
90 |
53 |
63 |
3.1 μg mL−1 |
83 |
27 |
37f |
1.60 μg mL−1 |
90 |
|
|
|
|
 |
| Fig. 5 Synthesis of anti-TB quinoline hybrids from substituted quinolines. | |
In another research effort, Gnanavelu et al. synthesized quinoline-piperazine hybrids of sulfonamides 18 and 19 in excellent amounts by the reaction of 4,6-dimethoxy- and 6-fluoro-4-methoxy-2-(piperazin-1-ylmethyl)quinolines 1g–h as a TFA salt with 2-fluorobenzenesulfonyl chloride 17 in DCM solvent, at 0 °C, in the presence of Hünig's base (Fig. 5). These compounds presented good activity against MTB, with MIC values of 0.07 and 1.1 μM, respectively53 (Table 1).
Likewise, Moodley and associates prepared 21 as a main product with a good yield from 4,7-dichloroquinoline 1a, which reacted with propane-1,2-diamine by a nucleophilic substitution reaction to form N1-(7-chloroquinolin-4-yl)propane-1,2-diamine, which was then treated with 20 in dry ACN and set to reflux at 85 °C for 24 h to obtain compound 21 (Fig. 5). This showed good activity against MTB, with an MIC value 0.90 μM.6 Kanchrana's group developed the targeted compound 23 in an 84% yield by the reaction of (Z)-2-chloro-N-hydroxyquinoline-3-carbimidoyl chloride 1i and 22 in the presence of Et3N base through ultrasonication in DCM solvent at room temperature for 15 min, while in MeOH solvent, when using the conventional method at room temperature for 12 h without any base, they found only a trace amount of the targeted compound 23 (Fig. 5). This showed significant resistance activity against MTB, with an MIC value of 6.25 μg mL−154 (Table 1).
2.2. From substituted anilines
The synthesis of quinoline from substituted aniline represents a valuable strategy in organic chemistry, facilitating the production of these important heterocyclic compounds. This method typically involves the cyclization of substituted anilines, utilizing various reagents and catalysts to promote the formation of the quinoline ring. By altering the substituents on the aniline, chemists can fine-tune the properties of the resulting quinoline derivatives, thereby enhancing their biological activity and pharmacological potential. Different synthetic routes, such as the Skraup and Friedländer methods, allow for the introduction of diverse functional groups, contributing to the versatility of quinoline compounds. This approach not only streamlines the synthesis process but also allows expanding the repertoire of quinoline derivatives available for therapeutic applications, particularly in treating TB and other diseases. In a similar vein, Zaheer et al. prepared the novel compounds N′-((2-chloro-6-methoxyquinolin-3-yl)methyl)isonicotinohydrazide 25 and N-((2-chloro-6-methoxyquinoilin-3-yl)methyl)-3-fluoro-4-morpholinoaniline 27 by 4-methoxy acetanilide 24a, which was reacted with POCl3/DMF at 80 °C to give 6-methoxy 2-chloroquinoline-3-carbaldehyde through the Vilsmeier–Haack reaction, which was then treated with 9 and 3-fluoro-4-morpholinoaniline 26 to form the corresponding Schiff bases. These Schiff bases were reduced by NaBH4 in methanol solvent to produce the corresponding compounds 25 and 27, respectively (Fig. 6). These compounds exhibited exceptional activity against MTB, with MIC values of 15.00 and 18.27 μM, respectively55 (Table 1). Similarly, Baliram's team synthesized Schiff base 29 by 4-methylaniline 24b, which reacted with acetic anhydride to form N-(p-tolyl)acetamide, which was then treated with POCl3/DMF and water in acetic acid to obtain 2-hydroxy-6-methylquinoline-3-carbaldehyde in two steps, which was then combined with 4-methylbenzenesulfonohydrazide 28 and acetic acid in ethanol at 70 °C to synthesize the desired compound 29 (Fig. 6). This showed good activity with an MIC value of 0.26 μg mL−1 against MTB3 (Table 1). By comparison, Alsayed and colleagues synthesized arylamide N-((3s,5s,7s)-adamantan-1-yl)-5-bromo-4-hydroxyquinoline-2-carboxamide 32 from 3-bromo aniline 24c, which was treated with dimethyl acetylenedicarboxylate 30 to prepare the substituted 1,4-dihydroquinoline-2-carboxylate through an intramolecular Friedel–Crafts reaction. This was then hydrolyzed by 10% basic NaOH to form the corresponding carboxylic acid, which reacted with (3s,5s,7s)-adamantan-1-amine 31 in the presence of DIPEA as a base, and HOBt hydrate and EDC-HCl as coupling agents to produce compound 32 (Fig. 6). This exhibited excellent activity against MTB with an MIC value of 9.97 μM56 (Table 1). The novel product (Z)-N′-(1-benzyl-2-oxoindolin-3-ylidene)-7-chloro-4-hydroxyquinoline-3-carbohydrazide 35 was synthesized by Abdelrahman et al. through 3-chloro aniline 24d, which was treated with diethyl 2-(ethoxymethylene)malonate 33 and phenyl ether under reflux conditions in two steps to give ethyl 7-chloro-4-hydroxyquinoline-3-carboxylate, which was reacted with hydrazine and 1-benzylindoline-2,3-dione 34 to produce compound 35 in two steps (Fig. 6). This compound showed excellent activity with an MIC value of 0.24 μm mL−1 against MTB57 (Table 1).
 |
| Fig. 6 Synthesis of anti-TB quinoline derivatives from substituted anilines. | |
Quinolinyl-1,2,3-triazoly-1,2,4-triazol-3(4H)-ones 37a–f were prepared by Nesaragi and associates in a very short time with good yields through 2/3/4-substituted N-phenylacetamides 24e–h, which were reacted with POCl3 and DMF under reflux conditions to produce 2-chloro-6/7/8-substituted quinoline-3-carbaldehydes through a Vilsmeier–Haack reaction, which were then treated with NaBH4, PBr3, and NaN3 in three steps to form 6/7/8-substituted 3-(azidomethyl)-2-bromoquinolines, which were reacted with acetylenic dipolarophiles 36a–b to prepare compounds 37a–f, respectively, through a cycloaddition reaction in the presence of CuSO4 and sodium ascorbate under microwave conditions at 100 °C (Fig. 6). These showed an anti-TB activity with MIC values ranging from 1.60–3.25 μg mL−1 (ref. 58) (Table 1). Likewise, Quimque's team developed quinoline derivatives 38a–b from 4-butylaniline 24i and 4-isopropylaniline 24j, respectively. These were then reacted with hydroxylamine hydrochloride and chloral hydrate in aqueous Na2SO4 to produce the corresponding 2-(hydroxyamino)-N-phenylacetamide intermediates, which reacted with H2SO4 to form 5-substituted isatins, respectively. These diones were treated with substituted ketones in the presence of 33% KOH in ethanol solvent at 120 °C under microwave conditions for 90 min to obtain compounds 38a–b (Fig. 7). These showed highly effective resistance against MTB with MIC values of more than 16 μg mL−1 (Table 1). Their activity varied due to the effect of the aryl group at the C-2 position and the expansion of the alkyl chain at the C-6 position.59 In a similar fashion, Ramprasad et al. developed a syntheticsic protocol for obtaining 6-bromo-3-((4-(3-fluorophenyl)-1H-1,2,3-triazol-1-yl)(phenyl)methyl)-2-methoxyquinoline 41a and 6-bromo-2-methoxy-3-((4-octyl-1H-1,2,3-triazol-1-yl)(phenyl)methyl)quinoline 41b using 4-bromo aniline 24k, which was reacted with 3-phenylpropanoyl chloride 39, POCl3/DMF, NaOMe/MeOH, respectively, in three steps to form 3-benzyl-6-bromo-2-methoxyquinoline. This was then treated with N-bromosuccinamide and NaN3/DMF in two steps to prepare 3-(azido(phenyl)methyl)-6-bromo-2-methoxyquinoline, which was reacted with 1-ethynyl-3-fluorobenzene 40a and dec-1-yne 40b in the presence of CuI and ACN at 80 °C for 3 h to obtain 41a–b, respectively, through a click reaction (Fig. 7). These showed excellent resistance against the growth of M. bovis with MIC values of 31.5 and 34.8 μM, respectively60 (Table 1). Sahana and researchers synthesized 2,4,5-trisubstituted imidazole-quinolines 43a–b from N-phenylacetamide 24l with POCl3/DMF under reflux conditions to form 2-chloroquinoline-3-carbaldehyde, which was refluxed with 70% acetic acid for 4 h to give 2-hydroxyquinoline-3-carbaldehyde, which was further reacted with 42a–b in the presence of NH4OAc and acetic acid under reflux conditions to synthesize compounds 43a–b (Fig. 7). These demonstrated good resistance activity against MTB with MIC value of 12.5 μg mL−1 (Table 1). Due to the presence of halogen substituents on the aryl ring, the activity was increased.2
 |
| Fig. 7 Synthesis of anti-TB quinoline derivatives from substituted anilines. | |
Quinoline derivatives with a hydrazone moiety 47a–c were prepared with excellent yields by Shruthi et al. from 3-chloro aniline 24m. Specifically, this was treated with diethyl ethoxymethylenemalonate 44 and then heated at 240 °C in DOWTHERM medium in two steps to produce 7-chloroquinolin-4-ol, which then reacted with POCl3 and piperazine in two steps to prepare 7-chloro-4-(piperazin-1-yl)quinoline, which was coupled with 4-chloromethyl-benzaldehyde 45 to obtain the substituted benzaldehyde intermediate. This intermediate combined with hydrazine hydrochloride 46 in ethanol to give the targeted compounds 47a–c (Fig. 7). These showed moderate inhibitory activity against MTB with MIC values of 4 μg mL−1. Due to the occupancy of the chloro group at the 7-position of the quinoline nucleus and hydrazones with 4-tolyl-4-flouro- and 4-isopropyl-phenyl groups, these showed elevated activity.5 Murnane and teammates synthesized the quinoline hybrid 49 by the reaction of 4-methoxyaniline 24n with ethyl acetoacetate to form 6-methoxy-2-methylquinolin-4-ol, which was then treated with 2-(bromomethyl)quinoline 48 in K2CO3 and anhydrous DMF at RT to obtain compound 49 (Fig. 7). This exhibited high resistance against QcrB T313I with MIC value of 4.7 μm mL−1.61
2.3. From miscellaneous starting materials
These methods typically involve non-traditional reactants, thereby expanding the scope of possible structural modifications. The flexibility in choosing the starting materials enables the introduction of unique functional groups into the quinoline ring, enhancing properties like anti-TB activity. Some examples include cyclization reactions, multi-component reactions, and the use of heterocyclic intermediates. This approach not only broadens the range of synthesized quinolines but also contributes to the discovery of novel therapeutic agents targeting TB and other diseases. For instance, Babu et al. prepared 53a–f from the reaction of 50 and 51 in the presence of DMF under reflux conditions to form 5,5-dimethyl-3-((3-methyl-1H-pyrazol-5-yl)amino)cyclohex-2-en-1-one, which was reacted with substituted aromatic aldehydes 52a–f in DMF under reflux conditions to prepare the corresponding intermediates. These intermediates were then treated with furoyl chloride to synthesize compounds 53a–f under reflux conditions for 5–6 h (Fig. 8). All six compounds 53a–f showed MIC values of 1.6 μg mL−1 (Table 1) and had exceptional resistance against MTB. An excellent anti-TB activity was also reported due to the presence of the heteroaryl group pyrazole linked to the quinoline ring.46 In the same way, Kadam and colleagues synthesized 56a–g from 54 and bromine in acetic acid to obtain 8-benzyloxy-5-(2-bromo-acetyl)-1H-quinoline-2-one, which was treated with various aromatic thioamides 55a–g under reflux conditions in ethanol reaction medium to give the final compounds 56a–g (Fig. 8). These displayed potential activity against MTB with MIC values of 62.5, 62.5, 25, 62.5, 12.5, 50, and 62.5 μg mL−1 respectively62 (Table 1). Shinde and colleagues designed 59a–b from the 5-substituted isatins 57a–b and 58 to get the corresponding 6-substituted quinoline-2,4-dicarboxylic acids through a Pfitzinger reaction, which were then selectively decarboxylated in nitrobenzene at 210 °C to give the 6-substituted-4-quinoline carboxylic acids and then coupled with N,O-dimethylhydroxyamine hydrochloride, in the presence of the coupling agent EDC, and HCl and DMAP as a base to synthesize the corresponding carboxamides at 0 °C. These carboxamides reacted with CH3MgBr and the epoxidizing agent S(CH3)3I in two steps to obtain the relative 6-substituted-4-(2-methyloxiran-2-yl)quinolines, which were finally combined with alcohols to synthesize compounds 59a–b (Fig. 8). These displayed good activity against MTB with MIC values of 14.4 and 9.2 μg mL−1, respectively63 (Table 1). Similarly, Rani et al. prepared 63 in a good yield from 60, which was reacted with 61 under microwave heating at 150 °C to obtain N-(5-fluoro-1,3-dioxoisoindolin-2-yl)isonicotinamide. This was then treated with 7-chloro-4-(piperazin-1-yl)quinoline 62 under microwave heating at 180 °C for 15 min to synthesize compound 63 (Fig. 8). This compound displayed potential activity against MTB with an MIC50 value of 3.1 μg mL−164 (Table 1).
 |
| Fig. 8 Synthesis of anti-TB quinolines from miscellaneous reactants. | |
3 Study compendium
Quinoline and its derivatives exhibit a broad spectrum of pharmacological activities. The consideration of MIC values has significantly helped advance our understanding of how to develop more effective quinoline-based drugs for combating TB. Structural modifications, such as incorporating specific substituents, as well as adding alkene, tetrazole, hydrazone, and pyrazole groups to the quinoline ring, have been shown to enhance anti-TB activity. In this review, we compiled 53 commonly synthesized quinoline-containing anti-TB agents. Of these, 17 compounds were synthesized via substituted quinolines, 20 compounds were derived from substituted anilines, and the remaining 16 compounds were formed using miscellaneous reactant molecules, all with good yields (Fig. 9).
 |
| Fig. 9 Quinoline analogues as anti-TB agents. | |
The synthesized compounds exhibited strong efficacy against MTB, with varying MIC values. In summary, reviews of this nature are crucial in the current landscape, as there is a crucial requirement to develop anti-TB drugs that are less toxic, more cost-effective, simpler to produce, and highly potent. This is especially true as the current tuberculosis treatments are typically costly, have high toxicity, and are complex, driving the search for molecules that can interact with novel targets or support multi-target combination therapies. As the number of newly tested compounds against mycobacteria continues to rise, there is significant potential to develop innovative drugs aimed at fully eradicating TB. We also highlighted the latest advancements in the synthesis of quinoline compounds. Several traditional methods have been refined and improved over the years, while new synthetic techniques have been developed. There is a pressing need for new anti-TB drug regimens that can reduce treatment duration and effectively combat the rising threat of drug-resistant and MDR-TB strains.
One of the main variables affecting quinoline derivatives' MIC values against MTB is their substitution patterns. By increasing the electron density on the quinoline ring, electron-donating groups, like –OH or –NH2, can improve the binding affinity with bacterial enzymes and thereby reduce the MIC.65 By lowering the electron density, electron-withdrawing groups, such as –NO2 or –CN, on the other hand, impair the compound's interaction with the target and raise the MIC values. Heterocyclic or aryl substitutions can decrease the MIC by increasing the affinity for particular MTB targets, such as enzymes involved in cell wall formation.66 Additionally, by increasing the stability and bioavailability, these changes may maximize effectiveness. Bulkier groups, on the other hand, may make it more difficult to bind or penetrate cell walls, which would raise the MIC values. Thus, improving quinoline compounds for the successful treatment of drug-resistant TB requires strategic replacements of the groups.67 The use of MIC values has significantly helped the understanding of how to make quinoline derivatives for more effective TB treatment. The MIC values for all the quinoline derivatives mentioned in this paper are summarized in the table given below.
4 Future challenges
There are several current and likely upcoming obstacles to the development and commercialization of target-based quinoline core designs for the treatment of MDR MTB. The development of drug resistance as a result of mutations in important therapeutic targets is one significant barrier and would make it challenging for quinoline derivatives to remain effective.8 Furthermore, it is still very difficult to optimize these drugs' pharmacokinetics for increased bioavailability, tissue penetration, and low toxicity. Targeting certain MTB processes, including resistance-related enzymes or efflux pumps, necessitates a thorough comprehension of the bacterium's adaptive mechanisms. The development process is further complicated by the requirement for quinoline derivatives to work in synergistic combinations with other anti-TB medicines. Another layer of difficulty is the possibility of cytotoxicity and off-target effects brought on by the highly reactive nature of quinoline derivatives, particularly when heterocyclic groups are added. Overcoming the adaptation mechanisms of resistant MTB strains, the development of creative design approaches, and thorough pharmacological profiling will be necessary to meet these obstacles.68
5 Conclusion
Quinoline and its derivatives have attracted significant attention for their potential in anti-TB drug development. The structural versatility of the molecule allows for modifications that can enhance its antibacterial efficacy, particularly against MTB, the causative agent of TB. These compounds have demonstrated promising activity by targeting various bacterial enzymes and processes, including DNA gyrase, cell wall synthesis, and energy metabolism. Additionally, quinoline derivatives have shown the ability to act synergistically with existing TB treatments, which is a potential avenue for improving therapeutic outcomes. The ability to design derivatives with improved pharmacokinetic properties would further enhance their therapeutic potential. The pharmacokinetic properties of quinoline derivatives, including ADME, are crucial in determining their efficacy and toxicity. Factors like lipophilicity, molecular size, and stability influence bioavailability and tissue penetration, while metabolism via cytochrome P450 enzymes can affect their half-life and active forms. Quinoline derivatives with favorable pharmacokinetics often exhibit lower IC50 values, indicating their higher potency against MTB. Various enhancements have been investigated and reported, such as an aryl core, triazole ring, and heterocyclic analogs like nicotinic acid hydrazide, that can improve their anti-TB activity. However, challenges remain, including the excessive accumulation of reactive nitrogen species, which can cause cytotoxicity, damaging essential macromolecules, and affecting efficacy. Other challenges remain in overcoming resistance mechanisms, optimizing selectivity for the TB pathogen, and ensuring low toxicity profiles to minimize adverse effects. Continued research and development in this area could lead to novel quinoline-based therapies that could address the urgent necessity for new and effective anti-TB drugs, especially in the face of rising drug resistance and the limited efficacy of current treatments against MDR and widely drug-resistant TB strains. The initial transformation of quinoline by D. indolicum proceeded via hydroxylation at the 2-position. This mechanism has been reported several times under various redox conditions; however, other degradation pathways have also been identified for the metabolism of quinoline under sulfate-reducing conditions. In earlier studies of the anaerobic degradation of quinoline, the successive transformation of 2-OH-quinoline was only described under methanogenic conditions, leading to the formation of methylated and hydroxylated intermediates. Another new pathway leading to hydroxylated and hydrogenated intermediates was thus observed.
Abbreviations
TB | Tuberculosis |
HIV | Human immunodeficiency virus |
MTB | Mycobacterium tuberculosis |
DprE1 | Decaprenylphosphoryl-β-D-ribose 2′-epimerase |
InhA | Enoyl-acyl carrier protein reductase |
MmpL3 | Mycobacterial membrane protein large 3 |
ATP | Adenosine triphosphate |
QSAR | Quantitative structure–activity relationships |
ADMET | Absorption, distribution, metabolism, excretion, and toxicity |
QcrB T313I | A subunit of cytochrome bcc, working as a component of the electron-transport chain of MTB |
MDR | Multidrug resistance |
WHO | World Health Organization |
US-FDA | US Food and Drug Administration |
BCG | Bacillus Calmette-Guerin |
M. Bovis | Mycobacterium bovis |
HOBt | Hydroxybenzotriazole hydrate |
DIPEA | Diisopropylethylamine |
EDC-HCl | 1-Ethyl-3-(3-dimethylaminopropyl)carbodiimide hydrochloride |
DMHA-HCl | Dimethylhydroxyamine hydrochloride |
DMAP | Dimethyl amino pyridine |
MIC | Minimum inhibitory concentration |
SDGs | Sustainable development goals |
PDGF | Platelet-derived growth factor |
RTK | Receptor tyrosine kinase |
DMF | Dimethylformamide |
TFA | Trifluoroacetic acid |
DCM | Dichloromethane |
TFA | Trifluoroacetic acid |
DNA | Deoxyribonucleic acid |
RT | Room temperature |
Data availability
No primary results, software, or code has been included and no new data were generated or analyzed as part of this review.
Conflicts of interest
There are no conflicts to declare.
Acknowledgements
The authors are thankful to the University College of Science, MLSU, Udaipur, Rajasthan, India for providing essential research and library facilities and also grateful to the University Grant Commission (UGC), New Delhi, India for financial support.
References
- M. C. Mandewale, B. R. Thorat and R. S. Yamgar, Synthesis and anti-mycobacterium study of some fluorine containing Schiff bases of quinoline and their metal complexes, Pharma Chem., 2015, 7(5), 207–215 CAS.
- S. Sahana, G. Vijayakumar, R. Sivakumar, D. Sriram and D. Saiprasad, Synthesis, docking study and in-vitro evaluation of anti-tuberculosis activity of tri substituted imidazoles containing Quinoline Moiety, J. Korean Chem. Soc., 2022, 66(3), 194–201 CAS.
- T. V. Baliram, K. P. Sanjay and M. P. Arvind, Synthesis, spectral characterization and antitubercular study of novel quinoline schiff base and its metal complexes, Anal. Chem. Lett., 2021, 11(4), 523–538 CrossRef.
- A. Zumla, P. Nahid and S. T. Cole, Advances in the development of new tuberculosis drugs and treatment regimens, Nat. Rev. Drug Discovery, 2013, 12(5), 388–404 CrossRef CAS PubMed.
- S. TG, S. Subramanian and S. Eswaran, Design, synthesis and study of antibacterial and antitubercular activity of quinoline hydrazone hybrids, Heterocycl. Commun., 2020, 26(1), 137–147 CrossRef.
- R. Moodley, C. Mashaba, G. H. Rakodi, N. B. Ncube, M. V. Maphoru, M. O. Balogun, A. Jordan, D. F. Warner, R. Khan and M. Tukulula, New quinoline–urea–benzothiazole hybrids as promising antitubercular agents: Synthesis, in vitro antitubercular activity, cytotoxicity studies, and in silico ADME profiling, Pharmaceuticals, 2022, 15(5), 576 CrossRef CAS PubMed.
- S. Kumar, N. Kaushik, J. K. Sahu and S. Jatav, Quinoline Analogues and Nanocarrier Systems: A Dual Approach to Anti-Tubercular Therapy, Eur. J. Med. Chem. Rep., 2024, 100212 CAS.
- A. N. S. da Gama and M. N. Soeiro, Quinoline-based compounds as key candidates to tackle drug discovery programs of microbicidal agents, Curr. Pharm. Des., 2021, 27(15), 1757–1762 CrossRef PubMed.
- N. Tuyiringire, D. Tusubira, J.-P. Munyampundu, C. U. Tolo, C. M. Muvunyi and P. E. Ogwang, Application of metabolomics to drug discovery and understanding the mechanisms of action of medicinal plants with anti-tuberculosis activity, Clin. Transl. Med., 2018, 7, 1–12 CrossRef.
- S. S. Alsayed and H. Gunosewoyo, Tuberculosis: pathogenesis, current treatment regimens and new drug targets, Int. J. Mol. Sci., 2023, 24(6), 5202 CrossRef CAS PubMed.
- A. Weyesa and E. Mulugeta, Recent advances in the synthesis of biologically and pharmaceutically active quinoline and its analogues: a review, RSC Adv., 2020, 10(35), 20784–20793 RSC.
- R. Kaur and K. Kumar, Synthetic and medicinal perspective of quinolines as antiviral agents, Eur. J. Med. Chem., 2021, 215, 113220 CrossRef CAS PubMed.
- P. S. Fasinu, B. L. Tekwani, B. Avula, N. D. Chaurasiya, N. D. Nanayakkara, Y.-H. Wang, I. A. Khan and L. A. Walker, Pathway-specific inhibition of primaquine metabolism by chloroquine/quinine, Malar. J., 2016, 15, 1–12 CrossRef PubMed.
- S. Nandi, S. Ahmed and A. Saxena, Combinatorial design and virtual screening of potent anti-tubercular fluoroquinolone and isothiazoloquinolone compounds utilizing QSAR and pharmacophore modelling, SAR QSAR Environ. Res., 2018, 29(2), 151–170 CrossRef CAS.
- S. E. Adeniji and O. B. Adalumo, Computational modeling and ligand-based design of some novel hypothetical compound as prominent inhibitors against Mycobacterium tuberculosis, Futur. J. Pharm. Sci., 2020, 6, 1–11 CrossRef.
- A. Martorana, G. La Monica and A. Lauria, Quinoline-based molecules targeting c-Met, EGF, and VEGF receptors and the proteins involved in related carcinogenic pathways, Molecules, 2020, 25(18), 4279 CrossRef CAS PubMed.
- S. Eswaran, A. V. Adhikari, I. H. Chowdhury, N. K. Pal and K. Thomas, New quinoline derivatives: Synthesis and investigation of antibacterial and antituberculosis properties, Eur. J. Med. Chem., 2010, 45(8), 3374–3383 CrossRef CAS PubMed.
- P. A. Procopiou, R. A. Ancliff, P. M. Gore, A. P. Hancock, S. T. Hodgson, D. S. Holmes, S. P. Keeling, B. E. Looker, N. A. Parr and J. E. Rowedder, The discovery of quinoline based single-ligand human H1 and H3 receptor antagonists, Bioorg. Med. Chem. Lett., 2016, 26(24), 5855–5859 CrossRef CAS PubMed.
- R. J. Altenbach, H. Liu, P. N. Banfor, K. E. Browman, G. B. Fox, R. M. Fryer, V. A. Komater, K. M. Krueger, K. Marsh and T. R. Miller, Synthesis, potency, and in vivo profiles of quinoline containing histamine H3 receptor inverse agonists, J. Med. Chem., 2007, 50(22), 5439–5448 CrossRef CAS PubMed.
- T. Troïa, J. Siad, C. Di Giorgio and J. M. Brunel, Design and synthesis of new polyamine quinoline antibiotic enhancers to fight resistant gram-negative P. aeruginosa bacteria, Eur. J. Med. Chem. Rep., 2022, 5, 100054 Search PubMed.
- H. Kumar, V. Devaraji, R. Joshi, M. Jadhao, P. Ahirkar, R. Prasath, P. Bhavana and S. K. Ghosh, Antihypertensive activity of a quinoline appended chalcone derivative and its site specific binding interaction with a relevant target carrier protein, RSC Adv., 2015, 5(80), 65496–65513 RSC.
- I. Chaaban, O. H. Rizk, T. M. Ibrahim, S. S. Henen, E.-S. M. El-Khawass, A. E. Bayad, I. M. El-Ashmawy and H. A. Nematalla, Synthesis, anti-inflammatory screening, molecular docking, and COX-1, 2/-5-LOX inhibition profile of some novel quinoline derivatives, Bioorg. Chem., 2018, 78, 220–235 CrossRef CAS PubMed.
- A. Upadhyay, P. Kushwaha, S. Gupta, R. P. Dodda, K. Ramalingam, R. Kant, N. Goyal and K. V. Sashidhara, Synthesis and evaluation of novel triazolyl quinoline derivatives as potential antileishmanial agents, Eur. J. Med. Chem., 2018, 154, 172–181 CrossRef CAS PubMed.
- E. A. Wilhelm, A. T. Ferreira, M. P. Pinz, A. S. D. Reis, A. G. Vogt, A. L. Stein, G. Zeni and C. Luchese, Antioxidant effect of quinoline derivatives containing or not selenium: Relationship with antinociceptive action quinolines are antioxidant and antinociceptive, An. Acad. Bras. Cienc., 2017, 89(1 suppl), 457–467 CrossRef CAS PubMed.
- K. Hengphasatporn, T. Aiebchun, P. Mahalapbutr, A. Auepattanapong, O. Khaikate, K. Choowongkomon, C. Kuhakarn, J. Meesin, Y. Shigeta and T. Rungrotmongkol, Sulfonylated Indeno [1, 2-c] quinoline Derivatives as Potent EGFR Tyrosine Kinase Inhibitors, ACS Omega, 2023, 8(22), 19645–19655 CrossRef CAS PubMed.
- B. Sureshkumar, Y. S. Mary, C. Y. Panicker, S. Suma, S. Armaković, S. J. Armaković, C. Van Alsenoy and B. Narayana, Quinoline derivatives as possible lead compounds for anti-malarial drugs: Spectroscopic, DFT and MD study, Arabian J. Chem., 2020, 13(1), 632–648 CrossRef CAS.
- S. Ökten, A. Aydın, Ü. M. Koçyiğit, O. Çakmak, S. Erkan, C. A. Andac, P. Taslimi and İ. Gülçin, Quinoline-based promising anticancer and antibacterial agents, and some metabolic enzyme inhibitors, Arch. Pharm., 2020, 353(9), 2000086 CrossRef PubMed.
- A. M. Jassem, A. M. Dhumad, F. A. Almashal and J. M. Alshawi, Microwave-assisted synthesis, molecular docking and anti-HIV activities of some drug-like quinolone derivatives, Med. Chem. Res., 2020, 29, 1067–1076 CrossRef CAS.
- A. R. Chabukswar, B. S. Kuchekar, S. C. Jagdale, P. D. Lokhande, V. V. Chabukswar, S. U. Shisodia, R. H. Mahabal, A. M. Londhe and N. S. Ojha, Synthesis and evaluation of analgesic, anti-asthmatic activity of (E)-1-(8-hydroxyquinolin-7-yl)-3-phenylprop-2-en-1 ones, Arabian J. Chem., 2016, 9(5), 704–712 CrossRef CAS.
- A. Saral, R. Shahidha, M. Thirunavukkarasu and S. Muthu, Molecular structure, spectral, computational, IEFPCM investigation, and topological study on the biologically potent; cardiotonic drug 2-chloroquinolin-3-amine with structural optimization, Chem. Phys. Impact, 2023, 6, 100193 CrossRef.
- S.-B. Wang, X.-Q. Deng, Y. Zheng, H.-J. Zhang and Z.-S. Quan, Synthesis and anticonvulsant activity evaluation of 8-alkoxy-5-(4 H-1, 2, 4-triazol-4-yl) quinoline derivatives, Arch. Pharmacal Res., 2013, 36, 32–40 CrossRef CAS PubMed.
- K. R. Devi, D. Ashok, K. Patnaik, R. Bathula, S. S. Rani and V. Bhakshi, Synthesis of Quinoline Derivatives by Microwave Irradiation Method and Evaluation for Their Anti-helminthic Activity, IOSR J. Pharm. Biol. Sci., 2019, 14, 16–22 Search PubMed.
- V. Antoci, L. Oniciuc, D. Amariucai-Mantu, C. Moldoveanu, V. Mangalagiu, A. M. Amarandei, C. N. Lungu, S. Dunca, I. I. Mangalagiu and G. Zbancioc, Benzoquinoline derivatives: A straightforward and efficient route to antibacterial and antifungal agents, Pharmaceuticals, 2021, 14(4), 335 CrossRef CAS PubMed.
- J. Ramírez-Prada, S. M. Robledo, I. D. Vélez, M. del Pilar Crespo, J. Quiroga, R. Abonia, A. Montoya, L. Svetaz, S. Zacchino and B. Insuasty, Synthesis of novel quinoline–based 4, 5–dihydro–1H–pyrazoles as potential anticancer, antifungal, antibacterial and antiprotozoal agents, Eur. J. Med. Chem., 2017, 131, 237–254 CrossRef PubMed.
- H. Chen, J. Mi, S. Li, Z. Liu, J. Yang, R. Chen, Y. Wang, Y. Ban, Y. Zhou and W. Dong, Design, synthesis and evaluation of quinoline-O-carbamate derivatives as multifunctional agents for the treatment of Alzheimer's disease, J. Enzyme Inhib. Med. Chem., 2023, 38(1), 2169682 CrossRef PubMed.
- R. A. Mekheimer, M. A. Al-Sheikh, H. Y. Medrasi and K. U. Sadek, Advancements in the synthesis of fused tetracyclic quinoline derivatives, RSC Adv., 2020, 10(34), 19867–19935 RSC.
- M. Asif, Study of clinically used and recently developed antimycobacterial agents, Orient. Pharm. Exp. Med., 2012, 12(1), 15–34 CrossRef.
- J. M. Robey, S. Maity, S. L. Aleshire, A. Ghosh, A. K. Yadaw, S. Roy, S. J. Mear, T. F. Jamison, G. Sirasani and C. H. Senanayake, Application of Chiral Transfer Reagents to Improve Stereoselectivity and Yields in the Synthesis of the Antituberculosis Drug Bedaquiline, Org. Process Res. Dev., 2023, 27(11), 2146–2159 CrossRef CAS PubMed.
- Q. Ruan, Q. Liu, F. Sun, L. Shao, J. Jin, S. Yu, J. Ai, B. Zhang and W. Zhang, Moxifloxacin and gatifloxacin for initial therapy of tuberculosis: a meta-analysis of randomized clinical trials, Emerging Microbes Infect., 2016, 5(1), 1–8 CrossRef PubMed.
- P. Cilliers, R. Seldon, F. J. Smit, J. Aucamp, A. Jordaan, D. F. Warner and D. D. N'Da, Design, synthesis, and antimycobacterial activity of novel ciprofloxacin derivatives, Chem. Biol. Drug Des., 2019, 94(2), 1518–1536 CrossRef CAS PubMed.
- F. Gao, Z. Chen, L. Ma, Y. Fan, L. Chen and G. Lu, Synthesis and biological evaluation of moxifloxacin-acetyl-1, 2, 3-1H-triazole-methylene-isatin hybrids as potential anti-tubercular agents against both drug-susceptible and drug-resistant Mycobacterium tuberculosis strains, Eur. J. Med. Chem., 2019, 180, 648–655 CrossRef CAS PubMed.
- A. P. Macabeo and A. Aguinaldo, Chemical and phytomedicinal investigations in Lunasia amara, Pharmacogn. Rev., 2008, 2(4), 317 Search PubMed.
- J. Lowther and A. Bryskier, Fluoroquinolones and tuberculosis, Expert Opin. Invest. Drugs, 2002, 11(2), 233–258 CrossRef PubMed.
- M. Lagdhir, C. Pandya, A. Pandya, R. H. Vekariya and D. P. Rajani, Indian J. Chem., 2021, 60, 986–998 Search PubMed.
- M. Owais, A. Kumar, S. M. Hasan, K. Singh, I. Azad, A. Hussain, Suvaiv and M. Akil, Quinoline Derivatives as Promising Scaffolds for Antitubercular Activity: A Comprehensive Review, Mini-Rev. Med. Chem., 2024, 24(13), 1238–1251 CrossRef CAS PubMed.
- N. Babu, R. Raju, R. Alavala, N. Malothu and Y. Padmavathi, Design, synthesis, anti-tubercular evaluation and teratogenicity studies of furanyl Pyrazolo [3, 4-b] quinoline-5-ones, Russ. J. Bioorg. Chem., 2023, 49(1), 127–138 CrossRef CAS PubMed.
- V. Sahasrabudhe, T. Zhu, A. Vaz and S. Tse, Drug metabolism and drug interactions: potential application to antituberculosis drugs, J. Infect. Dis., 2015, 211(suppl_3), S107–S114 CrossRef PubMed.
- Q. Sun, Y. Bai, C. Zhao, Y. Xiao, D. Wen and X. Tang, Aerobic biodegradation characteristics and metabolic products of quinoline by a Pseudomonas strain, Bioresour. Technol., 2009, 100(21), 5030–5036 CrossRef CAS PubMed.
- W. D. Hong, P. D. Gibbons, S. C. Leung, R. Amewu, P. A. Stocks, A. Stachulski, P. Horta, M. L. Cristiano, A. E. Shone and D. Moss, Rational design, synthesis, and biological evaluation of heterocyclic quinolones targeting the respiratory chain of Mycobacterium tuberculosis, J. Med. Chem., 2017, 60(9), 3703–3726 CrossRef CAS PubMed.
- S. Alegaon, K. Kashniyal, S. Kuncolienkar, R. Kavalapure, P. Salve, M. Palled, S. Suryawanshi and S. Jalalpure, Synthesis and biological evaluation of some 4-aminoquinoline derivatives as potential antitubercular agents, Futur. J. Pharm. Sci., 2020, 6, 1–12 CrossRef.
- M. Alcaraz, B. Sharma, F. Roquet-Baneres, C. Conde, T. Cochard, F. Biet, V. Kumar and L. Kremer, Designing quinoline-isoniazid hybrids as potent anti-tubercular agents inhibiting mycolic acid biosynthesis, Eur. J. Med. Chem., 2022, 239, 114531 CrossRef CAS PubMed.
- A. F. Borsoi, J. D. Paz, B. L. Abbadi, F. S. Macchi, N. Sperotto, K. Pissinate, R. S. Rambo, A. S. Ramos, D. Machado and M. Viveiros, Design, synthesis, and evaluation of new 2-(quinoline-4-yloxy) acetamide-based antituberculosis agents, Eur. J. Med. Chem., 2020, 192, 112179 CrossRef CAS PubMed.
- K. Gnanavelu, V. K. KS, S. Eswaran and K. Sivashanmugam, Novel quinoline-piperazine hybrids: the design, synthesis and evaluation of antibacterial and antituberculosis properties, RSC Med. Chem., 2023, 14(1), 183–189 RSC.
- M. Kanchrana, R. K. Gamidi, J. Kumari, D. Sriram and S. Basavoju, Design, synthesis, anti-mycobacterial activity, molecular docking and ADME analysis of spiroquinoxaline-1, 2, 4-oxadiazoles via [3+ 2] cycloaddition reaction under ultrasound irradiation, Mol. Diversity, 2024, 1–13 Search PubMed.
- Z. Zaheer, S. I. Shaikh, S. N. Mokale and D. K. Lokwani, Synthesis, biological evaluation and computational study of new quinoline hybrids as antitubercular agent, Lett. Drug Des. Discovery, 2018, 15(9), 914–922 CrossRef CAS.
- S. S. Alsayed, S. Lun, G. Luna, C. C. Beh, A. D. Payne, N. Foster, W. R. Bishai and H. Gunosewoyo, Design, synthesis, and biological evaluation of novel arylcarboxamide derivatives as anti-tubercular agents, RSC Adv., 2020, 10(13), 7523–7540 RSC.
- M. A. Abdelrahman, H. Almahli, T. Al-Warhi, T. A. Majrashi, M. M. Abdel-Aziz, W. M. Eldehna and M. A. Said, Development of novel isatin-tethered quinolines as anti-tubercular agents against multi and extensively drug-resistant mycobacterium tuberculosis, Molecules, 2022, 27(24), 8807 CrossRef CAS PubMed.
- A. R. Nesaragi, R. R. Kamble, P. K. Bayannavar, S. K. J. Shaikh, S. R. Hoolageri, B. Kodasi, S. D. Joshi and V. M. Kumbar, Microwave assisted regioselective synthesis of quinoline appended triazoles as potent anti-tubercular and antifungal agents via copper (I) catalyzed cycloaddition, Bioorg. Med. Chem. Lett., 2021, 41, 127984 CrossRef CAS PubMed.
- M. T. J. Quimque, A. D. Go, J. A. K. Lim, W. S. Vidar and A. P. G. Macabeo, Mycobacterium tuberculosis inhibitors based on arylated quinoline carboxylic acid backbones with anti-mtb gyrase activity, Int. J. Mol. Sci., 2023, 24(14), 11632 CrossRef CAS PubMed.
- J. Ramprasad, V. K. Sthalam, R. L. M. Thampunuri, S. Bhukya, R. Ummanni, S. Balasubramanian and S. Pabbaraja, Synthesis and evaluation of a novel quinoline-triazole analogs for antitubercular properties via molecular hybridization approach, Bioorg. Med. Chem. Lett., 2019, 29(20), 126671 CrossRef CAS PubMed.
- R. Murnane, M. Zloh, S. Tanna, R. Allen, F. Santana-Gomez, T. Parish and F. Brucoli, Synthesis and antitubercular activity of novel 4-arylalkyl substituted thio-, oxy-and sulfoxy-quinoline analogues targeting the cytochrome bc1 complex, Bioorg. Chem., 2023, 138, 106659 CrossRef CAS PubMed.
- V. Kadam, T. S. Choudhare, D. S. Wagare, A. Chatterjee and P. D. Netankar, Synthesis and Anti-Tubercular Screening of 8-Benzyloxy-5-[2-(Substituted-Phenyl)-Thiazol-4-yl]-1 H-Quinolin-2-One Derivatives, Polycyclic Aromat. Compd., 2024, 44(3), 1937–1946 CrossRef CAS.
- A. Shinde, P. P. Thakare, Y. Nandurkar, A. Chavan, A. L. N. Shaikh and P. C. Mhaske, Synthesis of 2-(6-substituted quinolin-4-yl)-1-alkoxypropan-2-ol as potential antimycobacterial agents, Chem. Pap., 2023, 77(7), 3791–3802 CrossRef CAS PubMed.
- A. Rani, M. D. Johansen, F. Roquet-Banères, L. Kremer, P. Awolade, O. Ebenezer, P. Singh and V. Kumar, Design and synthesis of 4-Aminoquinoline-isoindoline-dione-isoniazid triads as potential anti-mycobacterials, Bioorg. Med. Chem. Lett., 2020, 30(22), 127576 CrossRef CAS PubMed.
- M. Charton, Electrical effects of ortho-substituents in pyridines and quinolines, J. Am. Chem. Soc., 1964, 86(10), 2033–2037 CrossRef CAS.
- T.-a. Kato, K.-i. Saeki, Y. Kawazoe and A. Hakura, Effects of oligofluorine substitution on the mutagenicity of quinoline: a study with twelve fluoroquinoline derivatives, Mutat. Res., Genet. Toxicol. Environ. Mutagen., 1999, 439(2), 149–157 CrossRef CAS PubMed.
- C. O'Donnell, G. Knesel, T. Spencer and F. Stermitz, Substitution effects on the emissive properties of N-heteroaromatics. I. Substituted quinolines, J. Phys. Chem., 1970, 74(20), 3555–3559 CrossRef.
- R. S. Keri and S. A. Patil, Quinoline: a promising antitubercular target, Biomed. Pharmacother., 2014, 68(8), 1161–1175 CrossRef CAS PubMed.
|
This journal is © The Royal Society of Chemistry 2025 |
Click here to see how this site uses Cookies. View our privacy policy here.