DOI:
10.1039/D4PM00133H
(Review Article)
RSC Pharm., 2025,
2, 19-43
Navigating rheumatoid arthritis: insights into ligand-anchored nanoparticle strategies for anti-inflammatory therapy and relief
Received
2nd May 2024
, Accepted 28th October 2024
First published on 8th November 2024
Abstract
Rheumatoid arthritis (RA) is a chronic autoimmune inflammatory disease that primarily affects the synovial joints, causing substantial physical impairment, socioeconomic challenges and, in severe cases, death. Symptoms often appear between the ages of 35 and 60, with varying severity caused by periods of remission and exacerbation. In addition, children under the age of 16 might develop juvenile rheumatoid arthritis (JRA). According to a 2021 CDC poll, the World Health Organization estimates that 14 million people worldwide suffer with RA, with 0.92% of India's adult population afflicted. Non-steroidal anti-inflammatory drugs (NSAIDs), synthetic disease-modifying anti-rheumatic drugs (sDMARDs), and biological DMARDs are among the current therapeutic interventions. However, these therapies frequently exhibit limitations such as systemic side effects, short biological half-lives, erratic absorption, and frequent dosing regimens. Recent advances in ligand-based nanotechnology have introduced ligands such as folic acid and sialic acid that improve the targeted delivery when conjugated with nanoparticles. This approach has demonstrated efficacy in improving therapeutic outcomes while alleviating the side effects associated with conventional drug delivery systems. This review highlights the key molecular targets in RA, including T cells, B cells, and TNF-α, while exploring novel ligand-based active targeting strategies as innovative therapeutic avenues. Furthermore, it gives in-depth insights into critical molecular targets and their corresponding ligands, emphasizing the rising importance of ligand-based nanotechnology in the development of targeted drug therapy for autoimmune illnesses such as RA. The findings show the potential for these technologies to revolutionize RA therapy by improving medication specificity and reducing side effects via precise novel targeting mechanisms.
1. Introduction
Rheumatoid arthritis (RA) is a systemic chronic autoimmune disease that affects women more than men, because women have more reactive immunity than men and it appears that hormones influence the risk of possible flare-ups. It is also most common in the elderly.1 The prevalence of RA in India varies from 0.28 to 0.7 percent. RA is a chronic and disabling condition that affects around 2% of individuals in North America. Work disability is common early in the disease's course, with 20%–35% of people discontinuing working within 2–3 years. Employers must cover direct healthcare expenditures, such as medicine costs, job loss, productivity loss, and adaption costs, in order to keep employees with RA in the workforce.2 Intangible expenses such as quality of life (QOL) and early death bring the overall RA cost to $39.2 billion per year. A model created by Kobelt et al. discovered that overall expenses were highly connected with health assessment questionnaire responses, with the exception of expenditure associated with short- to medium-term work absences. In Sweden, indirect expenses were significantly greater as fewer patients remained in employment. On similar lines, Matzel et al. discovered that the economic costs associated with RA far outweigh those associated with osteoarthritis, hypertension, and combined osteoarthritis and hypertension. Comorbidities demonstrated a substantial relationship with illness expenses, and overall healthcare expenditure in patients with RA and concomitant depression and/or cardiovascular disease was considerably greater than in those with RA only. Multiple studies have suggested the relationship between lower socioeconomic status and worse disease outcomes.3 While drug costs constitute a major part of the direct costs associated with RA, studies also show that biological drugs lead to patients retaining employment, improving work ability and reducing indirect costs.4 Genetic and environmental factors, geographical location, and socioeconomic status of patients play a vital role in this disparity in prevalence.5 In addition to environmental factors, lifestyle and epigenetic factors also contribute to the pathogenesis of RA. Although the exact pathogenesis of RA is uncertain, numerous pathways and key inflammatory mediators that contribute to the development of the disease have been established. The synovial membrane of RA patients shows the aggravated accumulation of antigen-presenting cells such as macrophages, dendritic cells, and activated CD4+ T cells which secrete inflammatory markers interleukin-2 (IL-2) and interferon-α (IFN-α).6,7 T cells secrete interleukin-17 (IL-17), which in turn stimulates macrophage and fibroblast-like synoviocyte activity, causing inflammation. T cells contribute to the receptor activator for the nuclear factor κ B ligand (RANKL) pathway, which leads to activation of osteoclasts and results in bone destruction.8 By producing cytokines, antibodies, and auto-antibodies, B cells further aid in the pathogenesis and progression of RA. There are various cellular pathways such as the RANKL/RANK pathway, light chain enhancer nuclear factor kappa of activated B cells (NF-KB) signaling pathway, and mitogen-activated protein kinase (MAPK) pathway that cause the progression of RA.9 Due to these cellular changes at an early stage of the disease, the distal interphalangeal and metacarpophalangeal joints of the wrist and hands, and tiny joints of the foot, particularly the metatarsophalangeal joints, are affected. As the disease progresses, other features that are observed are “swan neck” (distal interphalangeal joint flex and the proximal interphalangeal joint is hyperextended), “boutonniere deformity” (the condition is opposite to the swan neck condition), “deformities of the thumb” and “ulnar deviation” (hands deviated medially).10 Furthermore, the disease also affects the other body organs like the lungs, heart, and brain by increasing the chances of heart disease and stroke in RA patients.11 Currently, there are several therapies available to treat the disease progression and mitigate the pain associated with it, categorized as first-line, second-line and third-line therapy. The first-line therapy includes NSAIDs, often prescribed to reduce pain and inflammation. However, these drugs are prone to side effects such as gastric irritation and allergic reactions, and their long-term use can lead to liver and kidney damage.12 The second line of treatment includes DMARDs, which are disease-modifying antirheumatic drugs. DMARDs like leflunomide, methotrexate, hydroxychloroquine, and sulphasalazine slow down the progression of RA by suppressing the immune systems via different molecular targets. Suppression of the immune response due to extensive use of DMARDs increases the risk of mycobacterial infections such as tuberculosis and histoplasmosis, and other opportunistic pathogenic infections, such as aspergillosis, listeriosis, and nocardiosis. The third-line treatment includes bDMARDs. The FDA has approved several bDMARDs for the treatment of RA in the past 20 years as shown in Table 1. These drugs have a transient biological half-life and therefore their frequent dosage often leads to lower patient compliance.13 Moreover, these drugs cause compromised immunity, which can lead to other serious infections like tuberculosis. It is therefore necessary to develop a medication that specifically targets and inhibits the mediator responsible for inflammation. In order to address some of the drawbacks and combat the side effects of conventional therapy, as discussed in the following sections researchers are envisaging the potential of nanotechnology-based strategies. Nanocarriers increase bioavailability at the site of inflammation, thus reducing the required dose and associated side effects. Additionally, nanocarriers enable sustained release of the active moiety.14+0
Table 1 bDMARDS in management of Rheumatoid Arthritis
S. no. |
Drug and brand name |
Name of company |
Year of approval |
Mechanism of action |
Formulation available |
Side effects |
Ref. |
1 |
Upadacitinib (Rinvoq) |
AbbVie Inc. |
2019 |
It inhibits Janus kinase (JAK) |
Extended-release tablet |
Risk of TB infections, extra-pulmonary disease, pulmonary embolism |
15 and 16 |
2 |
Baricitinib (Olumiant) |
Eli Lilly and Company |
2018 |
It inhibits Janus kinase (JAK) |
Film-coated release tablet, Immediate release tablet |
Risk of heart attacks, strokes, and blood clots increases |
16 and 17 |
3 |
Sarilumab (Kevzara) |
Sanofi and Regeneron |
2017 |
Antagonist of IL-6 receptor |
Subcutaneous injection |
Weight loss, severe belly pain, chest pain, and shortness of breath |
16 and 18 |
4 |
Tofacitinib (Xeljanz XR) |
Pfizer Inc. |
2016 |
It inhibits Janus kinase (JAK) |
Extended-release tablet, Immediate release tablet |
Increased risk of blood clots, heart attacks, and strokes |
16 and 19 |
5 |
Golimumab (Simponi Aria) |
Janssen Biotech, Inc. |
2013 |
TNF blocker |
IV infusion |
Susceptibility to bacterial (especially TB), fungal and viral infection |
16 and 20 |
6 |
Iguratimod |
Hetero Healthcare |
2011 |
Inhibits nuclear factor-kappa B (NF-κB) activation |
Tablet |
Nausea, vomiting, headache, stomach pain |
16 and 21 |
7 |
Tocilizumab (Actemra) |
Genentech, Inc. |
2010 |
Antagonist of IL-6 receptor |
IV infusion, Subcutaneous injection |
The risk of other opportunistic infections increases when patient is on long-term therapy |
16 and 22 |
8 |
Certolizumabpegol (Cimzia) |
UCB, Inc. |
2009 |
TNF blocker |
Injection |
Compromised immunity and increased risk of infections |
16 and 23 |
9 |
Rituximab (Rituxan) |
Genentech, Inc. |
2006 |
Cytolytic antibody (CD20-directed) |
IV infusion |
Progressive multifocal leuko-encephalopathy |
16 and 24 |
10 |
Abatacept (Orencia) |
Bristol-Myers Squibb Company |
2005 |
Selective T cell co-stimulation modulator |
IV infusion, Subcutaneous injection |
Risk of cancer increases. Difficulty in breathing and swallowing |
16 and 25 |
11 |
Adalimumab (Humira) |
AbbVieInc. |
2002 |
TNF-α blocker |
Injection |
Risk of upper respiratory tract infection (URTI) |
16 and 26 |
12 |
Anakinra (Kineret) |
SwedishOrphan BiovitrumAB |
2001 |
IL-1 antagonist |
Subcutaneous injection |
Decreases WBC count hence immunity is compromised and patient prone to other infections |
16 and 27 |
2. Immunopathogenesis and rheumatoid arthritis risk factors
In rheumatoid arthritis, immune cells migrate to the inflammatory site and interact with local synoviocytes. This contact produces cytokines, which stimulate immunological and mesenchymal cells and enhance their release. Monocytes release IL-6 and IL-1β, promoting Th17 differentiation. Th17 cells secrete IL-17, which leads to chronic inflammation by stimulating synoviocytes to create IL-6 and monocytes to produce IL-1 and TNF. IL-1 and TNF stimulate synoviocytes to create IL-6 and GM-CSF, which synergize with IL-17 and boost IL-6 production.28 Synoviocytes release IL-6, which promotes hyperplasia. IL-6 stimulates Th17 cells, which generate IL-17. In addition, IL-6 acts on osteoblasts, causing bone degradation. Cytokines stimulate synoviocytes, causing them to produce chemokines that recruit leukocytes and increase the inflammatory response. Matrix metalloproteinase (MMP) production also promotes cartilage degradation. Cell contacts and cytokines control cytokine production and maintain a pro-inflammatory state.29 Environmental triggers for RA include smoking, airborne agents, and infections, as well as host-related factors such as inheritance, autoimmune conditions, hormonal influences, and lifestyle factors, all of which contribute to chronic immune activation. These elements work together to drive the molecular targets leading to rheumatoid arthritis, as given in Table 2 and Fig. 1.
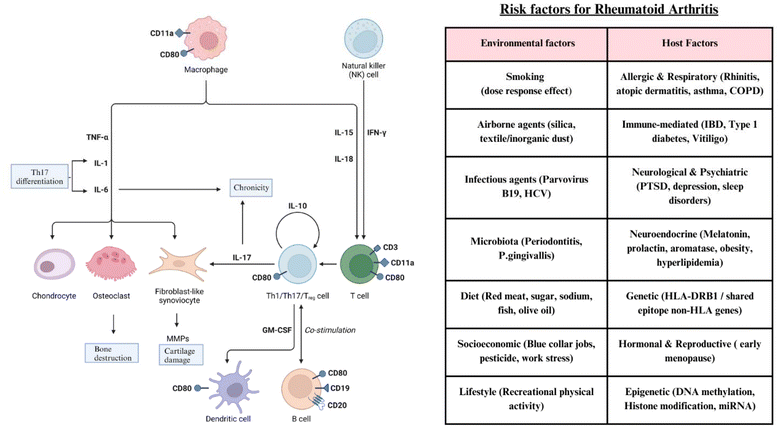 |
| Fig. 1 During RA, immune cells move to the inflammatory site and interact with local synoviocytes. This contact produces cytokines, which stimulate immunological and mesenchymal cells and enhance their release. Monocytes release IL-6 and IL-1β, promoting Th17 differentiation. Th17 cells secrete IL-17, which leads to chronic inflammation by stimulating synoviocytes to create IL-6 and monocytes to produce IL-1 and TNF. IL-1 and TNF stimulate synoviocytes to create IL-6 and GM-CSF, which synergise with IL-17 and boost IL-6 production. Synoviocytes release IL-6, which promotes hyperplasia. IL-6 stimulates Th17 cells, which generate IL-17. In addition, IL-6 acts on osteoblasts, causing bone degradation.28 Cytokines stimulate synoviocytes, causing them to produce chemokines that recruit leukocytes and increase the inflammatory response. MMP production also promotes cartilage degradation. Cell contacts and cytokines control cytokine production and maintain a pro-inflammatory state. | |
Table 2 The various molecular targets and their roles in RA
Molecular targets |
Role |
Ref. |
TnF-α |
TNF-α is a key cytokine involved in the pathology of synovial inflammation. RA is primarily caused by immune regulation, triggering an autoimmune response, primarily triggered by TNF-α. This response stimulates dendritic cells, T cells, and B-cells and leads to bone damage |
30 and 31 |
Interleukin-6 (IL-9) |
Interleukin 9 (IL-9) inhibits the generation of immunosuppressive cytokines by regulatory T cells. Blocking IL-9 may restore immunosuppressive activity of synovial regulatory T cells while inhibiting inflammatory T cells. Blocking IL-9 may be a unique therapeutic method for reducing synovial inflammation in RA |
32
|
Interleukin-7 (IL-7) |
The IL-7R mediates IL-7's effects, regulating CD4-T cell homeostasis. Elevated IL-7 levels in RA patients’ joints are associated with higher disease activity. IL2 family cytokines help mature T-lymphocytes proliferate and differentiate |
33
|
Janus kinases (JAKs) |
JAKs regulate the immune system and play important roles in hematopoiesis and neural development. Targeting JAK3 can reduce side effects and improve tolerability issues |
34–36
|
T-cell |
T cells, which are central to immune response, play important roles in RA by contributing to inflammation, joint injury, adaptive immunological response, and increasing inflammation and autoimmunity through cytokine production. T cells enter the synovial membrane and activate macrophages and synovial fibroblasts, converting them into tissue-destructive effector cells in rheumatoid arthritis |
37 and 38 |
B-cells |
B cells, the only cell type capable of producing antibodies, can be selectively depleted to improve various immune-mediated inflammatory diseases like RA, including those not primarily driven by autoantibodies |
39 and 40 |
Matrix metalloproteinases (MMPs) |
Chondrocytes and synovial fibroblasts produce matrix metalloproteinases (MMPs), which are thought to be the primary enzymes responsible for this breakdown. Several investigations have found that MMP expression is higher in cartilage and synovial tissues in OA and RA |
41–43
|
CTLA-4 (cytotoxic T-lymphocyte antigen 4) |
CD80 or CD86 binds to CD28 on the surface of CD4+ T cells. This activates T cells, causing them to proliferate and produce cytokines. When T cells are stimulated, they express cytotoxic t-lymphocyte antigen 4 (CTLA-4). This results in the inhibition of activated T cells and decreased cytokine production |
44 and 45 |
CD305 |
CD305, an anti-inflammatory molecule, is down-regulated on memory CD4+ T cells, negatively affecting RA disease activity. This leads to abnormal activation of T cells, causing inflammation |
46 and 47 |
CD305 regulates T-cell activation and inflammatory responses, promoting immune homeostasis and potentially influencing the pathogenesis of RA by influencing T-cell function and promoting an anti-inflammatory environment |
CD28 |
Immunophenotypes of CD8+ T cells in early and advanced RA, including homing and regulation. PD-1 is increased during inflammation and chronic T-cell activation, although upregulation of CD8+ CD28− T cells in the inflammatory synovium may be associated with RA development and clinical relapse. It is most likely unrelated to IFN-γ levels |
48
|
NF-kB (nuclear factor kappa-light-chain enhancer of activated B cells) |
NF-κB regulates the expression of cytokines IL-1β and TNFα, which are key mediators of inflammation in RA. TNFα and IL-1 trigger NF-κB activation, indicating a link between NF-κB activity and IL-1 and TNFα levels over time |
49 and 50 |
RANKL (receptor Activator of nuclear factor kB ligand) |
Promotes osteoclast development and activation, resulting in bone resorption and joint damage in RA |
Vascular endothelial growth factor (VEGF) |
In RA, angiogenesis is induced by a variety of proangiogenic agents, including VEGF. VEGF is overexpressed in RA patients, and its circulating levels are substantially associated with their disease activity |
51
|
METTL3 |
METTL3 is significantly increased in RA patients’ peripheral blood mononuclear cells, with positive correlations with RA activity markers. In vivo experiments show METTL3 up-regulation in synovial tissue and FLSs, suppressing proliferation, migration, invasion, and IL-6 expression |
52–54
|
METTL3 is up-regulated in RA patients’ synovial tissue and FLSs, and silencing it suppresses FLS proliferation, migration, invasion, and IL-6 expression, possibly by inhibiting the NF-κB pathway |
PKM2 |
PKM2 plays a crucial role in RA by optimizing energy supply and substrate supply for synoviocytes and immune cells. It interacts with transcription factors like STAT3, Bcl-2, HIF-1, and Erk1/2, regulating cell proliferation, apoptosis, and immune activation |
55–57
|
N6-methyladenosine (m6A) |
m6A levels are decreased in RA patients’ peripheral blood mononuclear cells (PBMCs), knockdown of METTL14 downregulates m6A, promoting inflammatory cytokines and joint inflammation. M6A affected TNFAIP3 expression through messenger RNA stability and translocation |
58–60
|
RA-related immune cells interact with cytokines secreted because of microvascular system damage and fibroblast-like synoviocyte proliferation. M6A methylation modification regulates immune cells, and abnormal genes may be biomarkers or targets for treatment of RA for targeted drug therapy |
3. Potential side effects of conventional drugs
Rheumatoid arthritis is a chronic autoimmune illness characterized by inflammatory synovium that damages joints and has systemic effects. Treatment seeks to alleviate symptoms, minimise inflammation, and avoid joint injury. Current therapies for RA, such as nonsteroidal anti-inflammatory drugs (NSAIDs), traditional synthetic disease-modifying anti-rheumatic drugs (sDMARDs), and biological DMARDs, have proved successful in managing disease progression. These conventional agents work well to control inflammatory arthritis, but some patients don't respond well to them or face serious side effects.61 Corticosteroids are divided into glucocorticoids and mineralocorticoids, each with unique properties. Glucocorticoids are anti-inflammatory, immunosuppressive, and vasoconstrictive, while mineralocorticoids regulate water balance and electrolytes. They are commonly prescribed for patients with RA due to their strong anti-inflammatory and immunosuppressive activities. The anti-inflammatory effect is due to their attachment to glucocorticoid receptors, forming a receptor–glucocorticoid complex that alters gene transcription. However, glucocorticoids can also present adverse effects, such as diabetes, osteoporosis, metabolic syndrome, cataracts, and peptic ulcers, especially in chronic or high-dose treatment.62 On top of that, these treatments might even speed up joint damage. Even using corticosteroids for a short time can lead to osteoporosis, weak bones and permanently reduced calcium bioavailability in the body.63
Also, anti-inflammatory drugs, except for low-dose prednisone, can lead to heart failure issues by raising blood pressure and keeping sodium in the body. Prednisone, which removes both sodium (Na+) and potassium (K+), can also cause risks like aplastic anemia, making it easier to get infections such as those from enteropathic E. coli.64 It is also indicated that there is an increased risk of mortality with prednisone.65 Non-steroidal anti-inflammatory drugs (NSAIDs) are a primary management strategy for managing pain and stiffness in RA patients. These drugs are known for their anti-inflammatory, analgesic, and antipyretic activities, mediated by inhibiting inflammatory mediators in the cyclooxygenase (COX) pathway. However, most NSAIDs are nonselective inhibitors, causing adverse effects like blood pressure changes, acute kidney ischemia, increased bleeding, serious upper gastrointestinal complications, and increased cardiovascular risks.66,67
Synthetic DMARDs like MTX reduce inflammation by binding to IL-1 receptor antagonists and regulating cytokine cascades. In RA therapy, MTX decreases inflammatory cytokines such as IL-1, IL-6, and TNF-α while increasing anti-inflammatory cytokines like IL-4 and IL-10. However, because of MTX's antifolate properties, long-term use can result in side effects such as vomiting, nausea, diarrhea, myelosuppression, liver dysfunction, renal failure, pancytopenia, pulmonary symptoms, mucositis, stomatitis, gastrointestinal ulcers, and cutaneous ulcerations, which can lead to MTX toxicity.68,69 Hydroxychloroquine suppresses antigen-presenting macrophage–T helper cell contact, lowering inflammation, yet it may induce gastrointestinal, dermatological, and retinal issues.70 Sulfasalazine can cause kidney damage, leukopenia, granulocytosis, central nervous system toxicity, and infertility by increasing inflammation-related adenosine production, inhibiting TNF-α expression, and suppressing B-cell activity.71 Targeting the molecular targets, primarily the T cells, B cells and TNF-APHA, has advanced the rheumatoid arthritis treatment significantly, but not without the possibility of potential side effects and limitations. Biologically disease-modifying Antirheumatic drugs (bDMARDs) are medications that target particular molecular pathways implicated in inflammatory disorders, such as RA. The FDA licensed the first bDMARD, TNF-α inhibitor (TNF-i), which has subsequently been developed to suppress pro-inflammatory cytokines and target cell-surface antigens. TNF-α is generated by different cell types and modulates the inflammatory response in RA via TNF receptors 1 and 2. It is implicated in the upregulation of RANKL, which promotes osteoclast development and bone resorption. High TNF-α levels in plasma can stimulate B cells, resulting in increased TNF-α production and aberrant B-cell activity. 30–50% of treated patients with TNF-i experienced primary and secondary failures, particularly those with long-standing illnesses.72 The TNF-α inhibitors, for instance, are very effective but can also increase the risk of infections, hepatotoxicity, malignity, demyelinating diseases and congestive heart failure.73 B-cell-targeting molecules like Rituximab may lead to hypogammaglobulinemia.74 T-cell-targeting therapies can also cause immunosupression, which raises the risk of infections and malignancies. The newer targets, IL-17 and IL-6 inhibitors, are also susceptible to causing infections.75,76 Tocilizumab, the first humanized IL-6 receptor antagonist, has sparked interest in treating patients with moderate to severe RA due to its safety profile and quick and long-lasting benefits. However, long-term usage has been linked to side effects such as headaches, high blood pressure and upper respiratory tract infections, emphasizing the need for further IL-6 prevention methods.77,78 In one study, NRF2's role in RA was highlighted in NRF2-knockout mice with antibody-induced arthritis, causing severe joint damage, synovial hyperplasia, pannus formation, cartilage erosion, and increased bone fractures. NRF2 activation may be a promising alternative to conventional antioxidant supplements, but it may not be effective in chronic diseases and may have long-term negative effects.79,80 Newer targets, like JAK inhibitors, provide oral administration and precise inflammatory pathway targeting but raise the risks of venous thromboembolism and malignancies.81 In humans, efalizumab, a monoclonal antibody against the αL subunit, was pulled from the market due to substantial adverse effects caused by its immunosuppressive impact.82 The suppression of ICAM-1, a critical cytokine, has been demonstrated to reduce lymphocyte activation in RA. Studies in mice and people have demonstrated that blocking ICAM-1 with an anti-ICAM-1 monoclonal antibody has favorable benefits, although this antibody is immunogenic and can induce fever and leukopenia.83
4. Current research on nanoparticulate system for treatment of rheumatoid arthritis
Nanoparticles have been thoroughly studied recently for a wide range of therapeutic applications. Liposomes, polymeric nanoparticles, dendrimers, niosomes, and solid lipid nanoparticles (SLNs) are examples of nanoparticulate carriers whose primary purpose is to minimize the numerous shortcomings of conventional systems. Nanoparticulate carriers provide enhanced cellular uptake, decreased toxicity, targeted and localized drug delivery, controlled release kinetics, and increased drug solubility. Lipid nanoparticles, polymeric micelles, and polymeric nanoparticles are among the several nanocarrier systems that have undergone substantial research for RA treatment, and are listed in Table 3.
Table 3 Current research on nanoparticulate system for treatment of RA
Types of nano-formulation |
Nano-carrier system |
Characteristics of nanoparticles |
API |
Result |
Ref. |
Nanoparticle |
Gold nanoparticle |
Spherical AuNPs are advantageous because of their large surface-to-volume ratio, excellent biocompatibility, and low toxicity, as well as size- and shape-related optoelectronic characteristics |
Methotrexate |
Lee et al. synthesised MTX-loaded PLGA (MTX-PLGA) nanoparticles and then deposited an Au film (15 nm) on the monolayer of MTX-PLGA nanoparticles to create a half-shell structure (MTX-PLGA-Au) of size 100–115 nm in diameter. In vivo NIR absorbance images showed that the nanoparticles only accumulated in the inflamed area after being intravenously delivered to the CIA mice. The inflamed paw's temperature was raised to 48 °C in response to NIR exposure (1.59 W cm−2 for 10 min), which caused a burst release of MTX from the nanoparticles. The RGD-MTX-PLGA Au nanoparticle-based treatment along with NIR irradiation demonstrated higher therapeutic efficacy with a significantly lower dose of MTX in the nanoparticles than the standard MTX treatment |
84
|
Chitosan nanoparticles |
Chitosan nanoparticles are stable, less toxic, simple, and biocompatible |
Methotrexate and dexamethasone |
Kumar et al. used ionic gelation method to prepare methotrexate and dexamethasone-loaded chitosan nanoparticles. The average particle size was found to be 217.4 nm (PDI 0.275) and 329.8 nm (PDI 0.286), respectively. The zeta potential of methotrexate and dexamethasone-loaded nanoparticles was found to be +26.9 mV and +19.5 mV, respectively. At pH 7.4 methotrexate chitosan nanoparticles followed a controlled zero-order and Fickian diffusion pattern and at pH 5.8 it followed a controlled first-order release, while dexamethasone chitosan nanoparticles followed first-order, Fickian release at both pH values |
85
|
Solid lipid nanoparticle |
Solid lipid nanoparticle manufacturing on a large scale is simple. They have low toxicity, are biocompatible, and biodegradable |
Celecoxib |
Kishor et al. designed celecoxib-loaded tristearin lipid nanoparticles (CEL-TS-LN) using the micro emulsion method. The average particle size was found to be 188 nm. CEL-TS-LN's in vitro drug release indicated sustained drug release of 62 percent for 36 hours, with the drug release being found to follow a Fickian diffusion mechanism and zero order. The serum biochemical characteristics of the CEL-TS-LN-treated animal groups demonstrated a significant difference (p 0.05) from the arthritic control (without treatment) group, with oedema inhibition of 88% |
86
|
There is an option for controlled and altered drug release |
Nanocapsule |
Lipid core nanocapsule |
|
Curcumin and resveratrol |
Coradini et al., prepared curcumin and resveratrol-loaded lipid core nanocapsules by interfacial deposition of a preformed polymer method. The particle size was found to be 200 nm with a PDI of 0.1. In the preclinical studies, the authors observed that the animal group receiving curcumin and resveratrol loaded in lipid core nanocapsules showed 37% to 55% inhibition in paw oedema when compared with the animal group receiving plain curcumin and resveratrol in preclinical studies using the FCA rat model |
87
|
Polymeric nanocapsule |
Targeted drug delivery and ligand conjugation are made possible by polymeric nanocapsules, which offer controlled release to the desired site and stability to unstable molecules (such as proteins) |
Indomethacin |
Bernardi et al. prepared nanocapsules loaded with indomethacin by interfacial deposition of polymer. The particle size and zeta potential values were found to be 240 nm and −6.9, respectively. In the preclinical studies on CFA rat model, they observed that the group receiving nanocapsule-loaded indomethacin showed 33 ± 4% inhibition in sub-chronic oedema while plain indomethacin showed 21 ± 2% inhibition in paw oedema. The serum levels of tumour necrosis factor and IL-6 were also significantly reduced by 84.11% and 83.8%, respectively, by the Indomethacin nanocapsule |
88
|
Lipid core nanocapsule |
Lipid core nanocapsules have excellent ability in preventing the drugs from degradation and high drug loading capacity |
Tacrolimus |
Friedrich et al. developed tacrolimus-loaded lipid core nanocapsules (TAC-LNC) of particle size 212 ± 11 and PDI less than 0.12 using a self-assembling method. At the end of 48 hours, the in vitro drug release was recorded at 57.11 ± 2.15% from TAC-LNC. In the preclinical studies on an FCA-induced arthritic rat model, significant inhibition in paw oedema was observed in the animal group treated with TAC-LNC after intra-peritoneal administration |
89
|
Nanoemulsion |
Labrafil1944CS |
Nanoemulsions are industry scalable. Hydrophobic drugs are effectively loaded, have greater stability |
Meloxicam |
Pathan et al. prepared nano-emulsion loaded with meloxicam. The particle size, PDI, and zeta potential of the optimized batch were found to be 66.6 nm, 0.22 and 0.86 mV, respectively. Transdermal efficacy was also improved and the highest flux 29.69 μg cm−2 h−1 was observed |
90
|
Lipoprotein |
Methotrexate |
Mello et al. formulated methotrexate nanoemulsion using different lipids mixture with high-pressure homogenization. In the in vivo study with an antigen-induced rabbit model, it was observed that LDE (lipidic-nanoemulsion) was taken up 2.5 times more by arthritic joints than by healthy joints. When compared with arthritic non-treated joints, intra-articular LDE-MTX treatment resulted in a clear dosage response pattern by lowering synovial leukocyte infiltration (P = 0.004) and protein leakage (P = 0.032) |
91
|
Nanomicelles |
Polycaprolactone polysialicacid |
Nanomicelles have small particle size with high drug loading capacity. Nanomicelles are soluble in water |
Cyclosporine A |
Wilson et al. prepared PSA-based micelles that were formed via self-assembly of PSA grafted with polycaprolactone (PCL) loaded with cyclosporine A. The particle size was found to be 107.5 ± 9.3 nm and zeta potential was found to be 29.7 ± 8.0. In vitro studies verified that rheumatoid arthritis synovial fibroblasts could internalize the cyclosporine-loaded micelles |
92
|
Polymeric nanomicelles |
Lornoxicam |
Helmy et al. formulated lornoxicam-loaded polymeric nano-micelles by direct equilibrium technique. The particle size of nano-formulation was found to be 169.45 nm with PDI 0.243. In the in vivo studies on a FCA rat model, the observations showed a significant inhibition of circulatory inflammatory mediators in the lornoxicam-nanomicelles-treated group as compared with the group receiving free lornoxicam |
93
|
Polyethylene glycol polycaprolactone |
Dexamethasone |
Wang Q et al. formulate dexamethasone-loaded micelles by film polymer dispersion method. The average particle size, PDI, and zeta potential were found to be 45 nm, 0.209 and −7.52 mV, respectively. The encapsulation efficiency was found to be 94.2%. The in vitro release at 37 °C and pH 7.4 showed that 90.6% of free dexamethasone was released within 6 hours, while there was only a 50% release of dexamethasone from micellar preparation in 72 hours, which showed release was sustained |
94
|
Dissolving microneedles loaded with nanomicellles |
Methotrexate |
Ting Liu et al. looked at the usage of methotrexate-loaded nanomicelles and dissolving microneedles for transdermal therapy of rheumatoid arthritis (RA). Nanomicelles encapsulated in mPEG-PLA and HA targeted inflammatory joint via the HA-CD44 receptor interactions. The dissolving microneedles formed microchannels and released nanomicelles in situ. In vivo investigations demonstrated that this technique significantly reduced the progression of RA without causing systemic impairment |
95
|
Solid lipid nanoparticles |
Glycerol monostearate |
Less chances of contamination and cost effective |
Aceclofenac |
Raj et al., prepared aceclofenac-loaded solid lipid nanoparticles by ultrasonic emulsification method of particle size 189 ± 9.2 nm PDI of 0.162 ± 0.02. The zeta potential was found to be −32.51 ± 0.12 mV and entrapment efficiency was found to be 86.51 ± 2.46%. In preclinical studies, the results showed 81% inhibition of paw oedema in the group receiving aceclofenac-loaded solid lipid nanoparticles |
96
|
Glycerol monostearate |
Piroxicam |
Hua Peng et al. designed solid lipid nanoparticles using a modified solvent evaporation process. The particle size was found to be 102 ± 5.2 nm with a PDI of 0.262. The zeta potential was found to be 30.21 ± 2.05 mV. The prepared SLNs showed high entrapment efficiency of 87.5% for Piroxicam |
97
|
Various nanoparticulate drug delivery techniques are being investigated for the treatment of rheumatoid arthritis. Nanocarrier systems have the ability to modify the pharmacokinetics of drugs, especially absorption and distribution. In recent years, clinical trials of nanocarriers used for the management of rheumatoid arthritis have be reported:98https://clinicaltrials.gov/study/NCT00241982 accessed on 10th September 2024; https://clinicaltrials.gov/study/NCT05117593 accessed on 10th September 2024; https://clinicaltrials.gov/study/NCT00760669 accessed on 10th September 2024.99 However, nanocarriers might not be sufficient on their own to deliver the drug to the target site. Nanocarriers can reach the specific target site when they are coupled with ligands, preventing systemic distribution to non-target locations. Conjugation of the carrier with an appropriate and specific ligand conjugation is a promising method for selective and effective therapy of rheumatoid arthritis. In order to make an accurate selection of ligand, it is of utmost importance to understand the prominent biological targets involved in rheumatoid arthritis. Steroids, non-steroidal anti-inflammatory drugs, and antihistamines, which are the current cornerstones of anti-inflammatory therapy, work primarily by blocking the production or activity of inflammatory mediators. Other biological agents have also been developed to target the recruitment or activation of inflammatory cells that drive the host's response to injury, such as antibodies to integrin-47 and CTLA-4-Ig, which disrupt T-cell activation. These newer biopharmaceuticals include tumor necrosis factor-neutralizing therapies, anti-IgE, and anti-CD20 antibodies, among others. Some of them are discussed in the next part. However, neither the conventional treatments nor the more recent biopharmaceutical methods are without their flaws. As a result, active targeting using a ligand-gated system offers a novel strategy for efficient drug delivery.
5. Biological targets
Inflammatory mediators play a significant role in the course of RA pain and inflammation because these components are key drivers in the inflammatory cascade. Fig. 2 illustrates the various pathways through which inflammation can develop.16 Understanding the pathogenetic relevance of these mediators in RA holds the key to the discovery of several possible biological targets for the disease.100 Cytokines have long been examined and studied as potential targets for RA since they are directly implicated in the RA process, and are classified as pro- or anti-inflammatory cytokines based on their actions against antigen response.101,102 Chemokines attract leukocytes, regulate angiogenesis, and play a vital role in RA etiology, hence are a potential biological target. Chemokines are classified according to their structures as CXC chemokines, CC chemokines, XC chemokines and CX3C chemokines.103 All of these chemokines are involved in RA pathogenesis during different stages of the disease. Several additional essential proteins, such as the cytokine targets discussed earlier, play major roles in the pathophysiology of RA, and related medications have been used in medical settings as part of continued RA research.101 The ongoing research being conducted for a better understanding of the biological targets for the efficient therapy of rheumatoid arthritis is presented in Table 4.
 |
| Fig. 2 Different pathways of inflammation: the above figure shows different pathways leads to the inflammation, bone erosion and cartilage damage. Activation of the Th1, Th17, dendritic cell, macrophages, synovial fibroblast, chondrocytes, B cell and Neutrophil leads to secretion of cytokines responsible for bone erosion, inflammation, cartilage damage and pannus formation. | |
Table 4 Role of inflammatory mediators in pathogenesis of RA
S. no. |
Inflammatory mediators |
Role in pathogenesis |
Current research |
Ref. |
1 |
T cell |
Zang et al. state that T cells contribute to the pathogenesis of RA by stimulating IL17, macrophages, fibroblast |
IgD-Fc-Ig fusion protein was created by Jing Zhang et al. The group discovered that inhibiting T-cell overactivation relieved symptoms of RA in in vivo trials |
104 and 105 |
Similarly, Kremer et al. created a CTLA4Ig fusion protein that inhibited CD28 engagement on T cells, inhibiting T-cell activation, and thereby slowing the progress of RA |
2 |
B cell |
Activates T cell and produce auto-antibodies |
Rituximab is the only available drug to target B cells |
106
|
3 |
NF-kB |
Activation and proliferation of T-cell, activation of macrophages and fibroblast |
Duan et al. combined siRNA and MTX in a calcium phosphate/liposome-based hybrid nano-carrier to block NF-kB signaling pathways and lower pro-inflammatory cytokine production. Xie et al. stated that iguratimod (IGU) can inhibit nuclear factor kappa B (NF-kB) activation by interfering with NF-kB translocation from the cytoplasm to the nucleus |
107 and 108 |
4 |
Macrophages |
Hamilton et al. state that GM-CSFR and macrophages are responsible for secretion of pro-inflammatory cytokines IL1 & IL6 |
The effect of anti-GM-CSFR antibodies on RA was investigated by Greven DE et al. Anti-GM-CSFR antibodies were found to decrease GM-CSFR-dependent macrophage proliferation in an in vivo mouse model |
109 and 110 |
5 |
CX3CL1 |
Tanaka et al. state that CX3CL1 plays a role in the migration of monocytes, T cells, and osteoclast |
E6011 is a new humanized anti-fractalkine monoclonal antibody. It was found that E6011 400 mg was well tolerated in active RA patients with an inadequate response to biologics in phase 2 clinical trials; however, it did not reach the study's primary aim |
111 and 112 |
6. Comparative evaluation of new rheumatoid arthritis treatments and ligand-based nanoparticle approaches
Rheumatoid arthritis is chronic and impacts quality of life significantly. The traditional and conventional therapies have various limitations, such as systemic side effects or the action taking longer to show effect. Ligand-based nanoparticle methods outperform traditional RA medications by providing better targeting, fewer side effects, and increased effectiveness. A comparison between ligand-based nanoparticles and the conventional therapies is given in Table 5.
Table 5 Comparison between the ligand-based nanoparticles and the conventional therapies
Characteristic |
Ligand-based nanoparticle strategies |
Conventional RA therapies |
Ref. |
Targeted delivery |
Hydrophilic HA was successfully incorporated onto pro-glycosomes for site-specific delivery of Tofacitinib via CD44 receptors in the RA region. The addition of Tofacitinib changed the zeta potential to −8.52 mV, and the Tofacitinib entrapment efficiency was 68.4 ± 3.85% |
Tofacitinib (TOF) is an active arthritis inhibitor that the FDA authorised in 2012. However, long-term oral dosing might result in adverse effects such as increased cholesterol, neutrophil decrease, and systemic effects. Topical application may be a better way to address these concerns and increase patient compliance. Topical pain management necessitates increased concentration in the synovial area and epidermal layers; nevertheless, penetration through the stratum corneum and deeper layers is difficult |
113–116
|
Efficacy |
The study aimed to develop a modified liposome gel coated with chondroitin sulphate (CS) proglycosomes (PG) for treating RA. The gel targets CD44 receptors, penetrates skin layers, and lowers cationic nanocarrier toxicity. When compared with a free drug-loaded gel, PG-incorporated liposomes had better skin deposition and penetration rates. The gel also demonstrated considerable Tofacitinib (TOF) localisation in epidermal layers, which might be attributed to the PG in the nanovesicle structure and the chondroitin sulphate coating. The arthritic score was highest in the DC group (4 ± 0.28) (P < 0.001) and considerably decreased (P < 0.001) in the CS-TOF-PG gel (0.7 ± 0.14), FD gel (3.15 ± 0.14), and TOF oral (3.1 ± 0.42) groups on the 21st day |
|
117–119
|
Controlled release mechanism |
Extracellular vesicle-guided in situ macrophage reprogramming is a unique therapy option for rheumatoid arthritis. This procedure entails restoring M1–M2 macrophage balance in synovial tissue. Intra-articular delivery of M2-EVs produces biodistribution comparable to footpad injection; however, local administration results in increased accumulation and extended EV sequestration in articular tissues. This is owing to delayed distribution dynamics through microvascular or lymphatic channels, as well as increased capillary permeability caused by joint inflammation. Unlike small substances, EVs can provide long-term activity for RA treatment following local injection. EVs, unlike small molecules, can provide sustained action for RA therapy after local injection |
Inflamed synovial joints contain highly active M1 macrophages that release pro-inflammatory cytokines and chemokines, causing bone erosion and cartilage deterioration |
120 and 121 |
Side effects and toxicity |
This study was the first to show that camptothecin (CPT) is effective against CIA at substantially lower concentrations than typical anti-cancer doses. A single subcutaneous injection of camptothecin sterically stabilised micelles-vasoactive intestinal peptide (0.1 mg kg−1) reduced joint inflammation in CIA mice for 32 days without causing systemic harm. CPT alone required at least a tenfold larger dosage to reach the same result, but with considerable vacuolization in liver histology |
The study investigated RA's potential as a new camptothecin indication in an existing animal model, but challenges like low solubility, instability, and systemic toxicity, along with side effects like myelosuppression, haematological toxicity, hemorrhagic renal cystitis, and elevated liver function tests, restricted its clinical applicability |
122–124
|
Pharmacokinetics |
Carrier-free polyglycyrrhetinic acid (PGA)-facilitated celastrol-loaded nanoparticle had a homogeneous particle distribution with an average particle size of 180 nm, good colloidal stability, a celastrol (Cel) loading capacity of about 4.5%, extended blood circulation, and efficient accumulation at inflammatory joints. Regular nanoparticles had a short half-life and low bioavailability, with quick elimination from the circulation. In vivo, PGA@Cel and poly(D,L-lactide-co-glycolide)@Cel NPs had a prolonged circulation duration due to their protective polyethylene glycol corona, lower plasma protein opsonisation, and minimal identification by the reticuloendothelial system. PGA@Cel NPs exhibited longer MRT0–∞ and T1/2 in the bloodstream |
MTX's circulation duration is limited in the bloodstream, leading to a short plasma half-life ranging from 2 to 10 h, necessitating a larger dose regimen to accumulate in inflamed joints and produce significant therapeutic results. This technique, however, has safety issues, such as gastrointestinal discomfort and bone marrow suppression, due to its short circulation time in the bloodstream |
125 and 126 |
7. Ligands for biological targets
Nanoparticles are currently being employed to offset the negative effects of conventional therapy.127 The ideal nanoparticle-based treatments should target pathologic tissues specifically, minimizing or eliminating off-target effects. Surface-modified nanoparticles have the potential for site-specific drug delivery.128 Active and passive targeting are two methods for delivering nanoparticles to specific tissues or organs.129 In active targeting, a suitable ligand is selected for the receptor which is over-expressed on the cell in the diseased condition.130 Targeting ligands specific to cell-surface components that are unique to and are elevated in dysplastic and pathologic tissues have been conjugated to nanoparticle surfaces in a lot of current research studies.131 Small compounds, like polypeptides (long unbranched chain made of amino acid), protein domains (complex structures made up of amino acids), antibodies, and nucleic acid-based aptamers are examples of targeting ligands. In order to improve nanoparticle targeting, ligands from various classes (chimeras) or several ligands from the same class but with diverse targets (multi-valences and multi-specificity) have used intestinal peptides, and beta-3 integrins are over-expressed on the inflammatory cells as compared with normal cells. Targeting approaches employing ligands specific to these receptors can be a promising medication delivery strategy for RA. Different ligands explored for targeting RA are discussed in detail in the following section.132
7.1. Folic acid
Folic acid (FA), chemically (2S)-2-[[4-[(2-amino-4-oxo-1H-pteridine-6-yl)methylamino]benzoyl]amino]pentanedioic acid, is also known as Vitamin B 9, and is water soluble and non-immunogenic. Folic acid has two monodentate carboxylate groups, hence it acts as a bi-dentate ligand. FA is stable over a wide temperature and pH range and is utilized for both diagnostic and therapeutic purposes when combined with drugs.133 When drug-loaded nanoparticles conjugated with folic acid bind to the folic acid receptors, they get internalized by endocytosis. In the acidic pH of endosomes (pH 5.0), the folic acid dissociates from the receptor and releases the drug. Folic acid receptor β (FRβ) is overexpressed on activated macrophages, which are important in the pathogenesis of RA, with a high affinity to folic acid.134,135 Activated macrophages play a critical role in the pathophysiology of RA by secreting TNF-α, IL-6, IL-1, chemokines, metalloproteinase, and reactive oxygen species, which are pro-inflammatory cytokines. The cellular internalization of the conjugated molecule via endocytosis is carried out by clathrin-independent carriers (CLIC) and the GPI-anchored protein-enriched early endosomal compartment (GEEC) pathway. This is followed by reduction in pH of the vesicle lumen by the action of proton pumps localized in the endosome membrane, which leads to a conformational change in the folate receptor protein allowing the release of bound ligand in the cytosol. The final step in the cycle is the return of the folate receptor to the cell surface. Using this mechanism, a folate-conjugated therapeutic or diagnostic agent is one approach applied for the treatment of arthritis.136–138 Folic acid-conjugated nanocarriers have shown encouraging results in several studies. One example is the study by Verma A. et al. on folate-conjugated double liposomes of methotrexate and prednisolone. They used the film casting method to prepare inner liposomes, and the glass bead method to prepare double liposomes. The prepared liposomes had a particle size of 429.3 ± 3.6 nm, a zeta potential of 8.01 ± 0.3 mV, and a PDI of 0.109. The entrapment efficiency of prednisolone and methotrexate was found to be 66.7 ± 3.9% and 45.3 ± 1.7%, respectively. In the preclinical studies on collagen-induced arthritis (CIA) rats, they observed that in comparison with the normal joint, the inflamed joint had a higher drug concentration. When compared with the plain drug, the concentration of unconjugated double liposomes increased up to threefold, while the concentration of folate-conjugated double liposomes increased tenfold.137,139 Another similar study by Yang et al. used folate-conjugated dextran methotrexate (Dex-g-MTX/FA) nanoparticles formulated by a one-step condensation reaction. The particle size of the prepared nanoparticle was found to be 90 nm. The release of methotrexate from a non-conjugated system was observed to be 77.68%, while the release from a conjugated system was observed to be 72.04%. Furthermore, it was observed that the bio-distribution of drugs was improved because of folate conjugation.140 Zang et al. investigated the use of a folic acid-conjugated micellar system to treat RA. They synthesized polysialic acid micelles conjugated with folic acid and natural cholesterol (FA-PSA-CC), which were loaded with dexamethasone and had a size of less than 100 nm and an optimum negative charge. In a pharmacokinetic study, it was discovered that the half-life and AUC of animals treated with FA-PSA-CC were longer than those treated with commercial dexamethasone injection. Because folic acid is well internalized, it improves the anti-inflammatory activity of various first- and second-line drugs.141 Bilthariya et al. developed etoricoxib-loaded bovine serum albumin nanoparticles conjugated with folic acid. The average particle size was found to be 215.8 ± 3.2 nm with a zeta potential of +7.8 mV. In an in vivo pharmacokinetic study, they discovered that free etoricoxib was cleared quickly and was not detected in the blood after 12 hours as compared with the drug-loaded nanoparticles. The residence times of etoricoxib nanoparticles and folate-conjugated etoricoxib nanoparticles were discovered to be 24 and 48 hours, respectively. They also discovered that the conjugated nanoparticle had a longer half-life (12.27 hours) than the non-conjugated nanoparticle (5.83 hours).142 The drug's retention time in the body increased as a result of the conjugation. All of the aforementioned studies emphasize the significance of folic acid's function as a ligand. The rate of drug internalization at the site of inflammation increases as a result of the overexpression of activated macrophages. Hence providing a unique strategy for treating RA involves using a folic acid-conjugated nanoparticle drug delivery method.
7.2. Hyaluronic acid (HA)
Hyaluronic acid is a naturally occurring non-protein glycosamine glycan (GAG).143 Chemically, it is a disaccharide composed of repeating units of −1, 3-N-acetyl glucosamine and −1, 4-glucuronic acid. It is a linear polymer that is water soluble and non-sulfated. HA has high biocompatibility, is biodegradable, is easily chemically modified, and is non-immunogenic. It also has lubricating and cartilage-protecting effects.144 In recent years, hyaluronic acid has attracted a lot of attention for its potential in active ligand targeting for various ailments. The CD44 receptor has a hyaluronic acid binding site on which HA binds at the N terminal of the receptor. These CD44 receptors are over-expressed in RA patients’ synovial fibroblasts, macrophages, and lymphocytes, which act as a selective binding site for hyaluronic acid-conjugated nanocarriers. Drug-loaded nanoparticles are then internalized through the endocytic process, preventing the release of inflammatory mediators that cause pain and inflammation by different mechanisms. This preferential binding to the CD44 receptor, which is abundant in inflamed joints, allows for site-specific delivery of drugs.145 In a study by Zhou et al., prednisolone was encapsulated into solid lipid nanoparticles and then coated with hyaluronic acid (HA-SLNs-PD). The SLNs were prepared by the film ultra-sonication method with an average particle size of 166.86 ± 1.25 nm and 19.8 ± 1.45 mV zeta potential. In vitro release study showed a release-retardant effect for the HA-SLNs-PD system (37.6%) as compared with the solution of free prednisolone (56.52%) at the end of 30 hours. In addition, on LPS-activated Raw 264.7 macrophages, the cellular uptake of the HA-SLNs-PD system was significantly higher than the non-conjugated system. The pharmacokinetic study also showed the AUC0–t, and Cmax of the HA-coated system to be 2.62 and 1.93-fold higher than the free prednisolone solution-treated group of animals. The in vivo studies depicted a lower histopathology score of 4.0 for the animal group treated with only SLN-PD, while the group treated with HA-SLNs-PD showed a lower histopathology score of 2.0 in comparison with the animals treated with saline that showed the highest (>7.0) histopathology score.146 The potential of hyaluronic acid as a ligand was also observed by Lee et al. with a gold nanoparticle encapsulating tocilizumab that was coupled with hyaluronic acid in successful RA treatment. With the lower particle size of 64.83 nm (PDI 0.18) and active targeting, the HUVECs cell line analysis revealed that the cellular uptake of the HA-conjugated system was greater than that of tocilizumab alone.147 Similarly, Jung et al. prepared hydrogel of HA and Pluronic F-127 loaded with piroxicam micelles. The PX-loaded HP hydrogels had AUC, AUMC, and Cmax values that were approximately 2–4 times higher than the control group. They also discovered that, when compared with conventional piroxicam formulations (Tmax = 4 h), the PX-loaded HP hydrogels had a substantially longer PX release (Tmax = 24 h).148
7.3. Arginyl glycyl aspartic acid (RGD)
Vascular endothelial cells (VECs) play an important role in the recruitment of leukocytes throughout the inflammatory process. Angiogenesis and the generation of soluble inflammatory mediators are also induced by VECs, resulting in inflammation.149 Angiogenesis is the process by which the vascular endothelial cell responds to increased oxygen and nutritional demands by generating new blood vessels. Because of the role played by angiogenesis in arthritis progression, anti-angiogenic approaches for arthritis treatment have recently gained attention.150 Targeting the vascular endothelial cell (VEC) in the inflamed region is a unique RA therapeutic strategy that takes into consideration the vascular endothelial cell's role in inflammation. Integrin αvβ3 is overexpressed on the angiogenic endothelium at the site of inflammation. RGD peptide is a site-specific ligand, which has widely been explored for targeting integrin αvβ3.151 When drug-encapsulated nanoparticles are coupled with this RGD peptide, drug-loaded nanoparticles are well internalized in the VEC, which is responsible for the inflammatory cascade.152 This was explored by Koning et al. for the development of dexamethasone-loaded polyethylene glycol liposomes conjugated with the RGD peptide (RGD-PEG-L) to target the angiogenic endothelium cell at the site of inflammation in Adjuvant-Induced Arthritic (AIA) rats. The conjugated system's average particle size was recorded at 100 nm, with a polydispersity index of 0.1. In comparison with PEG liposomes, the RGD-PEG-L showed a three-fold increase in targeting the site of inflammation in in vivo research on AIA rats. They also discovered that the concentration of RGD-PEG-L at inflamed sites was 6–10 times higher than at non-inflamed sites, indicating that RGD is a ligand for Integrin αvβ3 and hence provided site-specific activity. Conjugation with RGD aided in two-fold benefit by dosage reduction and a reduction in the drug's systemic side effects.152
Another application was observed by Wang et al. with the development of nimesulide and methotrexate-loaded RGD-conjugated polymeric micelles (R-M/N-PMs). RGD-conjugated methotrexate polymeric micelles (R-M-PMs) and RGD-conjugated nimesulide polymeric micelles (R-N-PMs) had particle sizes of 34.30 ± 0.61 nm and 60.20 ± 3.21 nm, respectively. The authors noted that the R-M/N-PMs had a potent inhibitory effect on angiogenesis demonstrated by the CAM assay. In an in vivo investigation on arthritic rats, they discovered that the animals that were given R-M/N-PMs had less bone degradation, fewer inflammatory cytokines in their blood, lower immunological organ index, and reduced joint swelling.153
7.4. Mannose
Mannose is an aldohexose type of sugar and it is the C-2 epimer of glucose. On the surface of macrophages and dendritic cells, mannose receptors are present which are involved in mannose receptor (MR)-mediated phagocytosis and endocytosis. Mannose receptors are a subgroup of the C-type lectin superfamily, which has three types of mannose receptor: M-type phospholipase A2 receptor (PLA2R), DEC-205/gp200-MR6 and Endo180/uPARAP.154 Among these, MR receptors participate in phagocytosis.155 Sultana et al. developed mannose-conjugated liposomes that encapsulated withaferin-A (MN-WA) with a particle size of 128.4 nm ±7.4 nm and zeta potential of 34.9 ± 0.34 mV. The study showed that in AIA rats treated with MN-WA, ankle joint inflammation was successfully controlled and that treatment with MN-WA inhibited the production of RANKL, which leads to osteoclast formation in AIA. Another interesting observation was the 9-fold rise in IL10 gene expression (an anti-inflammatory cytokine) in the MN-WA-treated group compared with the control group.156 There are limited data available on the utility of mannose as a ligand for targeting RA. This creates a need and scope for researchers to further study the role and extent of the utility of mannose in RA.
7.5. Chondroitin sulphate
Chondroitin sulphate is chemically classified as a disaccharide, un-branched anionic glycosaminoglycan that is soluble in water and is made up of alternate 4-linked-D-glucuronic acid and 3-linked N-acetyl galactosamine units. The sulphate groups at the fourth and sixth positions on the chondroitin sulphate molecule provide its hydrophilicity.157 Because chondroitin sulphate is structurally similar to hyaluronic acid, both the molecules show an affinity for the CD44 receptor. Similar to hyaluronic acid, chondroitin sulphate also works as an effective active targeting ligand owing to its low toxicity, biodegradability, and biocompatibility.158 In a study by Mahtab et al., the thin film hydration method was used to create teriflunomide-loaded nano-liposomes, which were then coated with chondroitin sulphate via an ionic interaction technique. The particle size was found to be 155.6 ± 2.12 nm with a zeta potential of −10.2 ± 1.4 mV. In 8 hours, the coated system gave a sustained release of 73.21 ± 2.1%, while the uncoated system showed a release of 92.12 ± 2.7%. A cell line investigation proved that the coated systems had higher uptake than uncoated systems, and this was reiterated in the animal study which revealed that, as compared with the traditional TEF-treated group, the chondroitin sulphate coated system significantly reduced inflammation and aided in the regeneration of injured cartilage.159 Another type of nanocarrier was explored in a study by Shilpi et al. where the authors used the solvent injection approach to develop methotrexate and aceclofenac-loaded solid lipid nanoparticles, followed by conjugation with chondroitin sulphate. The developed SLNs showed a zeta potential of 17.3 ± 0.9 mV, and a particle size of 131.2 ± 4.6 nm for the conjugated system. A detailed in vivo investigation showed that edema inhibition began after 1.5 hours and lasted for three hours in the conjugated system group, whereas edema inhibition began after three hours and lasted for eight hours in the unconjugated system group. The recovery rates in the groups also saw drastic differences, with animals who received conjugated–SLN recovering 6 times faster than non-conjugated SLNs. However, the recovery rate for conjugated SLN was 80–140 times higher than plain medication.160
7.6. Vasoactive intestinal peptide
Vasoactive intestinal peptide (VIP) is a 28-amino-acid peptide hormone that belongs to the glucagon/secretin superfamily.161 The receptors for vasoactive intestinal peptide are classified as vasoactive intestinal polypeptide receptor 1 (VPAC1) and vasoactive intestinal polypeptide receptor 2 (VPAC2).162 In proliferative synoviocytes, activated T-lymphocytes, and macrophages, VPAC receptors are overexpressed. As a result, conjugating VIP as a ligand to a drug-loaded nanoparticle successfully delivers the medicine to the site of inflammation. The use of vasoactive intestinal peptide as a ligand for targeting the overexpressed VPAC receptor is a novel approach for rheumatoid arthritis treatment.163 In the study by Yue Koo et al., camptothecin-loaded sterically stabilized micelles (CPT-SSM) were formulated by the co-precipitation method and then conjugated with VIP to obtain particles with an average size of 13 nm. The efficacy of the formulation in CIA-induced arthritic mice was investigated, where camptothecin effectively inhibited angiogenesis, collagenase expression, and proliferation of synoviocytes. The authors also observed that VIP-conjugated self-stabilizing micelles loaded with camptothecin were safe and long-acting, specifically targeting the inflamed site with the least systemic toxicity. As compared with the dose required for treating cancer, a 100-fold lower dose was required for treating inflammation. The linkage of CPT-SSM with the VIP increased the specificity of the nanoformulation and made it more effective for the treatment of inflammation.164 Another interesting study by Deng et al. observed the role played by VIP in the balance of CD4+, CD25+, Treg and Th17 in the CIA rat model, in which they observed that the severity and incidence of RA were decreased in the group treated with VIP.165
7.7. Dextran sulphate
Dextran sulphate is chemically a 1–6 connected glucopyranose polysaccharide, highly soluble in water, which has proven biodegradability, biocompatibility, non-immunogenicity, ease of chemical modification and non-toxicity. Dextran sulphate is a typical ligand for the macrophage scavenger receptor class A.166,167 Kim et al. prepared dextran sulphate-polycaprolactone co-polymer (DS-b-PCL) by using click chemistry. The authors hypothesized that DS-b-PCL nanoparticles would reach inflamed joints via both active and passive targeting using the affinity of dextran sulphate for macrophage scavenger receptor type A. The targeting ability of produced nanoparticles in the inflamed joints of CIA mice was established using live animal imaging. The study proved that the DS-b-PCL nanoparticles had a high target ability because they were actively taken up by macrophages. The observations above led to the conclusion that DS-b-PCL is a good nanocarrier ligand for rheumatoid arthritis therapy.168 Heo et al. prepared self-assembled dextran sulphate nanoparticles loaded with methotrexate by a simple dialysis method of particle size 220.0 ± 5.17 nm. The study examined the in vitro cellular uptake study on RAW264.7 of the dextran sulphate nanoparticles, which showed the effective uptake by activated macrophages through scavenger receptor type A-mediated endocytosis. The in vivo bio-distribution study with the CIA murine model also showed conjugated nanoparticles actively targeting the inflamed tissues. All of the evidence suggests that dextran sulphate functions as a ligand and improves clinical outcomes.169 To improve the therapeutic efficacy of methotrexate, another study was performed where pH-sensitive nanoparticles (NPs) were produced by encapsulating it in acetalated dextran (AcDEX). In vitro, the MTX@pH-AcDEX NPs demonstrated significant drug loading, pH-responsive release, and low cytotoxicity. Adjuvant-induced arthritic rats showed reduced joint oedema, synovial hyperplasia, and bone degradation by inhibiting the JAK-STAT pathway and suppressing pro-inflammatory cytokines such as TNF-α, IL-6, and IL-1β.170
7.8. Sialic acid
Sialic acid is a carboxylated monosaccharide with 9 carbons that is also known as N-acetyl neuraminic acid. This is found in the cell membrane's outermost layer and is linked to Lewis Oligosaccharide X, which is chemically N-acetylgalactosamine.171 This sialyted Lewis’ oligosaccharide X is a natural ligand found on the outermost layer of leukocytes that binds specifically to E-selectin and P-selectin.172 This E-selectin, integrin, and adhesion protein is abundantly expressed over the surface of vascular endothelial cells in inflammatory diseases. Siglecs are sialic acid-binding receptors that are expressed on immune cells and can inhibit immune responses. In RA, levels of sialic acid-binding Ig-like lectin-9 (Siglec-9) are elevated in the serum and synovial fluid.173 While the exact role played by Siglec-9 in RA pathogenesis has not been determined, Wang et al. showed that administration of recombinant human Siglec-9 Fc chimera protein caused the reciprocal regulation of the differentiation of Th17 and Treg cells, which significantly reduced collagen-induced arthritis development, demonstrating its immunosuppressive effect in RA.174 As a result, conjugating drug-loaded nanomaterial to sialic acid, a natural ligand for E-selectin and P-selectin, is a promising RA therapeutic strategy.175 The use of sialic acid as a ligand was demonstrated by Xu et al. with methotrexate-loaded dextran-octadecoic acid (Dex-OA) micelles conjugated with sialic acid. The formulated micelles were found to be 117.33 ± 5.77 nm in size. The cellular uptake study on human umbilical vein endothelial cells (HUVECs) depicted that there was more internalization of sialic acid-conjugated Dex-OA micelles as compared with non-conjugated Dex-OA nanoparticles. To check improved in vivo targeting, the CIA rat model was employed. In the animal study, there was a marked increase in the concentration of sialic acid-conjugated Dex-OA micelles in the inflamed joint as compared with the non-conjugated one. Due to conjugation with sialic acid, the drug encapsulated in micelles remained more at the inflamed site, enhanced bone repair function and protected the RA subject from bone erosion.176 Wang et al. prepared sialic acid-conjugated liposomes for targeting L-selectin, which is overexpressed on neutrophils and is a mediator in inflammatory action. They prepared dexamethasone palmitate-loaded sialic acid-conjugated liposomes of different sizes. The observations showed that the small size Dexamethasone Palmitate-Sialic Acid Liposomes (DP-SALs) were able to efficiently target the peripheral neutrophils and presented in high concentrations at the inflamed sites as compared with large size DP-SALs, and also had an excellent anti-inflammatory effect.177
7.9. Aryl hydrocarbon receptor ligand
The aryl hydrocarbon receptor is particularly significant in rheumatoid arthritis. It is a ligand-dependent transcription factor that controls cell differentiation, activation, and death in rheumatoid arthritis cells. The AhR receptor ligands Tetrachloro-dibenzop-dioxin, benzo[a]pyrene and 3,3′-diindolylmetheane have been reported to suppress osteoclastogenesis in arthritic mice. However, prolonged use of these substances has severe side effects, limiting their therapeutic usage in people and animals, therefore natural ligands are a preferable option for treating rheumatoid arthritis.178,179 Some phytoconstituents that function as ligands for this aryl hydrocarbon receptor are tetrandrine, norisoboldine, berberine, sinomenine, resveratrol, curcumin, and green tea. In the CIA mouse model, Yuan X et al. showed that oral formulations of tetrandrine exhibited anti-arthritis activity through restoring Th17/Treg balance via AhR. In the in vivo study, it was observed that tetrandrine decreases the number of CD4+ IL-17A+ T cells which are responsible for inflammation and increased the numbers of CD4+ CD25+ Foxp3+ T cells which are responsible for the induction of anti-inflammatory cytokines in the mesenteric lymph node.180
8. Latest advancement in rheumatoid arthritis research
Recent study provides fresh insights into RA's molecular underpinnings, highlighting critical pathways and opportunities for therapeutic intervention.181 New pharmacological therapies for RA are being developed, including targeted biologics and small-molecule inhibitors that precisely affect particular inflammatory pathways.182 Ying Chen et al. proposed a unique approach for treating rheumatoid arthritis (RA) by developing extremely efficient and safe DNA scavengers. Scavengers, such as cationic nanoparticles, can bind negatively charged cell-free DNA (cfDNA), which reduces inflammation and joint swelling in RA rats. The scavengers modified with dimethylamino groups (DPs) demonstrated better binding affinity and less cytotoxicity, returning conditions to normal in treated rats. This method provided a novel strategy for treating cfDNA-associated diseases, opening the path for future RA therapies.183 Another research investigation discovered that si-S1A3-Chol, a chemically modified siRNA, significantly restored the malfunction of RA-fibroblast-like synoviocytes (FLSs) and improved arthritis by targeting the Hedgehog signaling system. This technique addressed the constraints of small-molecule antagonists while increasing cellular absorption and silencing efficiency. The study also found that si-S1A3-Chol delayed arthritis development, reduced inflammation, and enhanced motor function in a CIA animal model.184 Linfu Yang et al. investigated Melittin (MLT) as a promising natural anti-rheumatic drug, regulating transcription levels of cytokines, immune responses, and metabolic biomarkers. qRT-PCR determined MLT's downregulation of inflammation-related genes, such as Il6, Jak2, Stat3, and Ptx3. Molecular docking simulations indicated MLT's stable binding to TNF-α and IL-1β.185,186
Nida Meednu et al.'s study indicated a particular abundance of NR4A-expressing B cells in the RA synovium, as well as their status as an antigen-experienced B cell in the synovial Ectopic lymphoid structures capable of developing into a memory B cell in situ in response to additional signals. NR4A might be used as a biomarker for chronic auto-antigen activation in the synovial milieu, as well as a new therapeutic target.187 Another study explored the role played by Casein Kinase 2 (CK2) in the pathological behaviours of Fibroblast-like Synoviocytes (FLS) in Rheumatoid Arthritis (RA). CK2 plays a crucial role in RA by promoting the aggressive phenotypes of FLS, and inhibiting CK2 with CX-4945 could potentially alleviate RA symptoms by targeting FLS-driven inflammation and tissue destruction. CX-4945 is a potential option for rheumatoid arthritis therapy, providing an alternative to standard medications such as NSAIDs, glucocorticoids, DMARDs, and biologics, but it has hazards such as immune suppression.188 The PTPN22 gene signature is seen in patients with active rheumatoid arthritis and could be reversed with adequate therapy in one of the studies. This gene signature can be partially replicated by TNFα or CD28 signalling pathways and abolished in vitro with adalimumab, etanercept or tocilizumab. This in vitro reversal may be utilised to predict clinical responsiveness to targeted therapy.189,190 A disease site-targeted RNA interference system was established by creating a non-viral compound between shRNA against TACE (shTNF-α converting enzyme) and a bone resorption site-specific peptide carrier made up of aspartate and arginine repeating sequences.191 The shTACE/peptide carrier combination reduced arthritic symptoms in collagen-induced arthritis (CIA) animals by improving anti-inflammatory and anti-osteoclastogenic properties. RNAi targeting TACE provides a new therapy option for RA that has fewer side effects.191 FDA approvals for shTACE-based therapies point to further study with better accuracy in targeting genetic and molecular factors.192
9. Future perspective
Targeted drug delivery to inflammatory cells can increase inflammatory cell selectivity, minimize peripheral/systemic toxicity, and allow for dose reduction. The two basic hurdles inherent to nano-medicine are the accomplishment of reproducible and large-scale manufacturing, as well as the inadequacy of present pre-clinical models in effectively mimicking human biology. Indeed, not only for preclinical and clinical applications but also for the pharmaceutical industry's acceptance of nano-medicine, generating mono-disperse systems with the necessary physicochemical features is essential. Furthermore, despite the positive outcomes of various in vitro and in vivo investigations, these models are still unable to accurately mimic rheumatoid arthritis in humans; while cell culture and designed animal models can aid in better understanding of system interactions and consequences, they cannot offer a complete picture of the human body's complexity. The discovery of diverse drug delivery techniques for targeting inflammatory mediators and immune system mediators has increased expectations for the development of a viable targeted drug delivery modality for RA treatment. The development of systems that can not only distinguish specific targets for RA but also efficiently internalize into cells will be a major focus in the coming years. Some of these issues could be solved by using a combination of targeting strategies. Furthermore, employing precise molecular addresses on the vascular endothelium, magnetic field targeting, and ultrasound are some of the developing medication targeting techniques in RA treatment that show a lot of promise. Targeting strategies utilizing nanotechnology and bio-conjugation chemistry that can alter a drug's bio-distribution to reduce toxicity while enhancing efficacy could improve the chances of new anti-arthritic therapies reaching patients.
10. Conclusion
Despite recent breakthroughs in pharmacological treatments for rheumatoid arthritis, cases of resistance to conventional medications are not uncommon, especially with frequent and long-term use of the drugs; this also results in adverse effects and non-specific organ damage. As a result, implementing alternative drug delivery strategies based on receptor targeting, as described in this review, has the potential to enhance patient outcomes by reducing the unwanted off-target effects of conventional drugs, and lowering the frequency and dose of drug administration. More thorough investigation into novel carriers and drug-targeted delivery to arthritic joints, as well as a better understanding of RA, could considerably improve the clinical treatment of RA patients. Apart from the above-mentioned cellular targets and pathways, there are plenty of new cellular targets available which have not been adequately explored yet. Toll-like receptors, NOD-like receptors, Fc-γ receptors (FcγRs) and TAM receptors are some receptors which play a vital role in the pathogenesis of RA but have not been explored as a target for the effective treatment of RA. Exploring these targets could create opportunities for researchers to explore newer ligands to target them. This ultimately increases site-specific delivery of the drugs and avoids side effects of drugs. Recently, the discovery of new receptors has opened new horizons for designing novel ligands for effective treatments.
Abbreviations
RA | Rheumatoid arthritis |
IL-2 | Interleukin 2 |
INF α | Interferon-α |
IL-17 | Interleukin 17 |
RANKL | Receptor activator for nuclear factor κ B ligand |
NF-KB | Nuclear factor kappa of activated B cells |
MAPK | Mitogen-activated protein kinase |
NSAIDs | Non-steroidal anti-inflammatory drugs |
DMARDs | Disease-modifying anti-rheumatoid drugs |
bDMARDs | Biological disease-modifying anti-rheumatoid drug |
IL-6 | Interleukin 6 |
TB | Tuberculosis |
JAK | Janus kinase |
TNF | Tissue necrosis factor |
IL-1 | Interleukin 1 |
WBC | White blood cells |
SLN | Solid lipid nanoparticles |
MTX | Methotrexate |
IL-17 | Interleukin 17 |
siRNA | Silencing ribonucleic acid |
GM-CSFR | Granulocyte-macrophage colony-stimulating factor |
FA | Folic acid |
HA | Hyaluronic acid |
GAG | Glycosaminoglycan |
VEC | Vascular endothelial cell |
VPAC1 | Vasoactive intestinal polypeptide receptor 1 |
CIA | Collagen-induced arthritis |
VIP | Vasoactive intestinal peptide |
AhR | Aryl hydrocarbon receptor |
Author contributions
The authors confirm their contribution to the paper as follows: Sujata P Sawarkar: study conception and design. Trinette Fernandes: data collection, analysis, and interpretation of results; draft manuscript preparation. Shriya Karmarkar: data collection, analysis, and interpretation of results; draft manuscript preparation, Zainab Choonia: data collection, analysis, and interpretation of results; draft manuscript preparation. Sankalp Gharat: data collection, analysis, and interpretation of results; draft manuscript preparation. All authors reviewed and approved the final version of the manuscript.
Ethics approval
No animal or human studies were carried out by the authors for this article.
Consent for publication
All authors have read the final manuscript and approved the submission of the same.
Data availability
No primary research results, software or code have been included and no new data were generated or analysed as part of this review.
Conflicts of interest
On behalf of all authors, the corresponding author states that there is no conflict of interest.
References
- Q. Guo, Y. Wang, D. Xu, J. Nossent, N. J. Pavlos and J. Xu, Rheumatoid arthritis: pathological mechanisms and modern pharmacologic therapies, Bone Res., 2018, 6(1), 15 Search PubMed . Available from: https://pubmed.ncbi.nlm.nih.gov/29736302/.
- P. Jacobs, R. Bissonnette and L. C. Guenther, Socioeconomic Burden of Immune-Mediated Inflammatory Diseases – Focusing on Work Productivity and Disability, J. Rheumatol. Suppl., 2011, 88, 55–61 CrossRef.
- M. Dey, A. Busby, H. Elwell, H. Lempp, A. Pratt and A. Young,
et al., Association between social deprivation and disease activity in rheumatoid arthritis: a systematic literature review, RMD Open, 2022, 8(1), e002058 CrossRef.
- B. Batko, P. Rolska-Wójcik and M. Władysiuk, Indirect Costs of Rheumatoid Arthritis Depending on Type of Treatment—A Systematic Literature Review, Int. J. Environ. Res. Public Health, 2019, 16(16), 2966 CrossRef PubMed.
- R. Handa, U. R. K. Rao, J. F. M. Lewis, G. Rambhad, S. Shiff and C. J. Ghia, Literature review of rheumatoid arthritis in India, Int. J. Rheum. Dis., 2016, 19(5), 440–451 CrossRef . Available from: https://pubmed.ncbi.nlm.nih.gov/26171649/.
- Q. Cheng, H. Wu and Y. Du, The roles of small-molecule inflammatory mediators in rheumatoid arthritis, Scand. J. Immunol., 2021, 93(3), e12982, DOI:10.1111/SJI.12982.
- B. Becher, T. Derfuss and R. Liblau, Targeting cytokine networks in neuroinflammatory diseases, Nat. Rev. Drug Discovery, 2024, 23(11), 862–879, DOI:10.1038/s41573-024-01026-y.
- D. Yuan, J. Hu, X. Ju, E. M. Putz, S. Zheng and S. Koda,
et al., NMDAR antagonists suppress tumor progression by regulating tumor-associated macrophages, Proc. Natl. Acad. Sci. U. S. A., 2023, 120(47), e2302126120, DOI:10.1073/pnas.2302126120.
- Q. Fang, C. Zhou and K. S. Nandakumar, Molecular and Cellular Pathways Contributing to Joint Damage in Rheumatoid Arthritis, Mediators Inflammation, 2020, 2020, 3830212 Search PubMed . Available from: https://pmc/articles/PMC7103059/.
- M. Chetta, P. B. Burns, H. M. Kim, F. D. Burke, E. F. S. Wilgis and D. A. Fox,
et al., The Effect of Swan-neck and Boutonniere Deformities on the Outcome of Silicone Metacarpophalangeal Joint Arthroplasty in Rheumatoid Arthritis, Plast. Reconstr. Surg., 2013, 132(3), 597 CrossRef CAS . Available from: https://pmc/articles/PMC4153347/.
- P. C. Taylor, F. Atzeni, A. Balsa, L. Gossec, U. Müller-Ladner and J. Pope, The Key Comorbidities in Patients with Rheumatoid Arthritis: A Narrative Review, J. Clin. Med., 2021, 10(3), 1–28 Search PubMed . Available from: https://pubmed.ncbi.nlm.nih.gov/33535498/.
- B. M. Köhler, J. Günther, D. Kaudewitz and H. M. Lorenz, Current Therapeutic Options in the Treatment of Rheumatoid Arthritis, J. Clin. Med., 2019, 8(7), 938 CrossRef PubMed . Available from: https://pubmed.ncbi.nlm.nih.gov/31261785/.
- J. R. Curtis and J. A. Singh, The Use of Biologics in Rheumatoid Arthritis: Current and Emerging Paradigms of Care, Clin. Ther., 2011, 33(6), 679 CrossRef CAS PubMed . Available from: https://pmc/articles/PMC3707489/.
- S. Gelperina, K. Kisich, M. D. Iseman and L. Heifets, The potential advantages of nanoparticle drug delivery systems in chemotherapy of tuberculosis, Am. J. Respir. Crit. Care Med., 2005, 172(12), 1487–1490 CrossRef PubMed . Available from: https://pubmed.ncbi.nlm.nih.gov/16151040/.
- L. Serhal and C. J. Edwards, Upadacitinib for the treatment of rheumatoid arthritis, Expert Rev. Clin. Immunol., 2019, 15(1), 13–25 CrossRef CAS . Available from: https://pubmed.ncbi.nlm.nih.gov/30394138/.
- M. Kukar, O. Petryna and P. Efthimiou, Biological targets in the treatment of rheumatoid
arthritis: a comprehensive review of current and in-development biological disease modifying anti-rheumatic drugs, Biologics, 2009, 3, 443 CAS . Available from: https://pmc/articles/PMC2763315/.
- P. C. Taylor, M. E. Weinblatt, G. R. Burmester, T. P. Rooney, S. Witt and C. D. Walls,
et al., Cardiovascular Safety During Treatment With Baricitinib in Rheumatoid Arthritis, Arthritis Rheumatol., 2019, 71(7), 1042–1055 CrossRef CAS . Available from: https://onlinelibrary.wiley.com/doi/full/10.1002/art.40841.
- Y. N. Lamb and E. D. Deeks, Sarilumab: A Review in Moderate to Severe Rheumatoid Arthritis, Drugs, 2018, 78(9), 929–940 CAS . Available from: https://pubmed.ncbi.nlm.nih.gov/29931592/.
- M. A. d. Á. Machado, C. d. Moura, S. F. Guerra, J. R. Curtis, M. Abrahamowicz and S. Bernatsky, Effectiveness and safety of tofacitinib in rheumatoid arthritis: A cohort study, Arthritis Res. Ther., 2018, 20(1), 1–9 Search PubMed . Available from: https://arthritis-research.biomedcentral.com/articles/10.1186/s13075-018-1539-6.
- E. Pelechas, P. V. Voulgari and A. A. Drosos, Golimumab for Rheumatoid Arthritis, J. Clin. Med., 2019, 8(3), 387 CrossRef CAS PubMed . Available from: https://www.mdpi.com/2077-0383/8/3/387/htm.
- S. Xie, S. Li, J. Tian and F. Li, Iguratimod as a New Drug for Rheumatoid Arthritis: Current Landscape, Front. Pharmacol., 2020, 11, 506946 Search PubMed . Available from: https://www.frontiersin.org.
- I. Navarro-Millán, J. A. Singh and J. R. Curtis, Systematic Review of Tocilizumab for Rheumatoid Arthritis: A New Biologic Agent Targeting the Interleukin-6 Receptor, Clin. Ther., 2012, 34(4), 788–802 Search PubMed . Available from: https://www.clinicaltherapeutics.com/article/S0149291812000938/fulltext.
- J. S. Smolen, P. Emery, G. F. Ferraccioli, W. Samborski, F. Berenbaum and O. R. Davies,
et al., Certolizumab pegol in rheumatoid arthritis patients with low to moderate activity: the CERTAIN double-blind, randomised, placebo-controlled trial, Ann. Rheum. Dis., 2015, 74(5), 843–850 CAS . Available from: https://pubmed.ncbi.nlm.nih.gov/24431394/.
- M. A. Lopez-Olivo, M. Amezaga Urruela, L. Mcgahan, E. N. Pollono and M. E. Suarez-Almazor, Rituximab for rheumatoid arthritis, Cochrane Database Syst. Rev., 2015, 1(1) DOI:10.1002/14651858.CD007356.PUB2.
- M. C. Genovese, J. C. Becker, M. Schiff, M. Luggen, Y. Sherrer and J. Kremer,
et al., Abatacept for rheumatoid arthritis refractory to tumor necrosis factor alpha inhibition, N. Engl. J. Med., 2005, 353(11), 1114–1123 CAS . Available from: https://pubmed.ncbi.nlm.nih.gov/16162882/.
- P. J. Mease, Adalimumab in the treatment of arthritis, Ther. Clin. Risk Manag., 2007, 3(1), 133 CAS . Available from: https://pmc/articles/PMC1936294/.
- M. Mertens and J. A. Singh, Anakinra for rheumatoid arthritis: A systematic review, J. Rheumatol., 2009, 36(6), 1118–1125 CrossRef CAS PubMed.
- M. Noack and P. Miossec, Selected cytokine pathways in rheumatoid arthritis, Semin. Immunopathol., 2017, 39(4), 365–383 CrossRef CAS.
- J. Huang, X. Fu, X. Chen, Z. Li, Y. Huang and C. Liang, Promising Therapeutic Targets for Treatment of Rheumatoid Arthritis, Front. Immunol., 2021, 12, 686155 CrossRef CAS.
- Monu, P. Agnihotri, M. Saquib and S. Biswas, Targeting TNF-α-induced expression of TTR and RAGE in rheumatoid arthritis: Apigenin's mediated therapeutic approach, Cytokine, 2024, 179, 156616, DOI:10.1016/j.cyto.2024.156616.
- M. Barghbani, M. R. Sarookhani, M. Abbasi, A. Maali, M. Hajiaghaei and S. Keshavarz Shahbaz,
et al., Evaluation of serum level of miR-155 and TNF-α in rheumatoid arthritis patients, Gene Rep., 2022, 26, 101447 CrossRef CAS.
- S. Chakraborty, R. Gupta, K. F. Kubatzky, S. Kar, F. V. Kraus and M. M. Souto-Carneiro,
et al., Negative impact of Interleukin-9 on synovial regulatory T cells in rheumatoid arthritis, Clin. Immunol., 2023, 257, 109814 CAS.
- N. M. Abdel Baki, F. T. Elgengehy, A. M. Zahran, S. Ghoniem, E. Elsayed and A. Medhat,
et al., Galectin-3 and interleukin-7 as potential serologic markers in rheumatoid arthritis patients, Egypt. Rheumatol., 2022, 44(4), 319–324 CrossRef CAS.
- T. Liang, L. Cen, J. Wang, M. Cheng, W. Guo and W. Wang,
et al., Discovery of novel dual Bruton's tyrosine kinase (BTK) and Janus kinase 3 (JAK3) inhibitors as a promising strategy for rheumatoid arthritis, Bioorg. Med. Chem., 2023, 96, 117354 CAS.
- T. Liang, L. Cen, J. Wang, M. Cheng, W. Guo and W. Wang,
et al., Discovery of novel dual Bruton's tyrosine kinase (BTK) and Janus kinase 3 (JAK3) inhibitors as a promising strategy for rheumatoid arthritis, Bioorg. Med. Chem., 2023, 96, 117354 CAS.
- T. Liang, L. Cen, J. Wang, M. Cheng, W. Guo and W. Wang,
et al., Discovery of novel dual Bruton's tyrosine kinase (BTK) and Janus kinase 3 (JAK3) inhibitors as a promising strategy for rheumatoid arthritis, Bioorg. Med. Chem., 2023, 96, 117354 CAS.
- C. M. Weyand, B. Wu and J. J. Goronzy, The metabolic signature of T cells in rheumatoid arthritis, Curr. Opin. Rheumatol., 2020, 32(2), 159–167 CAS.
- J. Li, P. Liu, Y. Huang, Y. Wang, J. Zhao and Z. Xiong,
et al., Immunophenotypic Landscape of synovial tissue in rheumatoid arthritis: Insights from ACPA status, Heliyon, 2024, 10(13), e34088 CAS.
- S. Fillatreau, B cells and their cytokine activities implications in human diseases, Clin. Immunol., 2018, 186, 26–31 CAS.
- H. Fujita, M. B. Soyka, M. Akdis and C. A. Akdis, Mechanisms of allergen–specific immunotherapy, Clin. Transl. Allergy, 2012, 2(1), 18–27 CrossRef PubMed.
- Y. Araki and T. Mimura, Matrix Metalloproteinase Gene Activation Resulting from Disordred Epigenetic Mechanisms in Rheumatoid Arthritis, Int. J. Mol. Sci., 2017, 18(5), 905 CrossRef PubMed.
- B. J. Heard, L. Martin, J. B. Rattner, C. B. Frank, D. A. Hart and R. Krawetz, Matrix metalloproteinase protein expression profiles cannot distinguish between normal and early osteoarthritic synovial fluid, BMC Musculoskelet. Disord., 2012, 13(1), 126 CrossRef CAS PubMed.
- Ł. Pulik, P. Łęgosz and G. Motyl, Matrix metalloproteinases in rheumatoid arthritis and osteoarthritis: a state of the art review, Rheumatology, 2023, 61(3), 191–201 CrossRef.
-
J. Barnes, Biologic Agents to Control Autoimmune Inflammation, in Translational Inflammation, Elsevier, 2019, pp. 177–191 Search PubMed.
-
J. Barnes, Biologic Agents to Control Autoimmune Inflammation, in Translational Inflammation, Elsevier, 2019, pp. 177–191 Search PubMed.
- M. Lyu, P. Jiang, B. Li, Z. Hu and N. Guo, CD305 participates in abnormal activation of memory CD4+ T cells in patients with RA and attenuates collagen-induced arthritis, Mol. Immunol., 2024, 173, 80–87 CAS.
- M. Lyu, P. Jiang, B. Li, Z. Hu and N. Guo, CD305 participates in abnormal activation of memory CD4+ T cells in patients with RA and attenuates collagen-induced arthritis, Mol. Immunol., 2024, 173, 80–87 CrossRef CAS PubMed.
- F. Ajam, M. Aghaei, S. Mohammadi, H. Samiei, N. Behnampour and A. Memarian, PD-1 Expression on CD8+ CD28- T cells within inflammatory synovium is associated with Relapse: A cohort of Rheumatoid Arthritis, Immunol. Lett., 2020, 228, 76–82 Search PubMed.
- S. S. Makarov, NF-κB in rheumatoid arthritis: a pivotal regulator of inflammation, hyperplasia, and tissue destruction, Arthritis Res., 2001, 3(4), 200 CAS.
- A. V. Miagkov, D. V. Kovalenko, C. E. Brown, J. R. Didsbury, J. P. Cogswell and S. A. Stimpson,
et al., NF-κB activation provides the potential link between inflammation and hyperplasia in the arthritic joint, Proc. Natl. Acad. Sci. U. S. A., 1998, 95(23), 13859–13864 CAS.
- R. M. Bakry, S. M. Hassan, R. A. Mohammed and E. A. Ablelaleem, Significance of vascular endothelial growth factor (VEGFA)-1154 G/A gene polymorphism (rs1570360) in rheumatoid arthritis patients, Egypt. Rheumatol., 2022, 44(3), 197–201 Search PubMed.
- J. Wang, S. Yan, H. Lu, S. Wang and D. Xu, METTL3 Attenuates LPS-Induced Inflammatory Response in Macrophages via NF- κ B Signaling Pathway, Mediators Inflammation, 2019, 2019, 1–8 Search PubMed.
- T. Miao, Y. Qiu, J. Chen, P. Li, H. Li and W. Zhou,
et al., METTL3 knockdown suppresses RA-FLS activation through m6A-YTHDC2-mediated regulation of AMIGO2, Biochim. Biophys. Acta, Mol. Basis Dis., 2024, 1870(4), 167112 CrossRef CAS.
- W. Shi, Y. Zheng, S. Luo, X. Li, Y. Zhang and X. Meng,
et al., METTL3 Promotes Activation and Inflammation of FLSs Through the NF-κB Signaling Pathway in Rheumatoid Arthritis, Front. Med., 2021, 8, 607585, DOI:10.3389/fmed.2021.607585.
- Y. H. Wang, P. Gao, Y. Q. Wang, L. Z. Xu, K. W. Zeng and P. F. Tu, Small-molecule targeting PKM2 provides a molecular basis of lactylation-dependent fibroblast-like synoviocytes proliferation inhibition against rheumatoid arthritis, Eur. J. Pharmacol., 2024, 972, 176551 CrossRef CAS.
- S. S. Ahn, H. M. Kim and Y. Park, Assessment of disease activity in patients with rheumatoid arthritis using plasma tumour M2-pyruvate kinase test, Front. Immunol., 2022, 13, 901555 Search PubMed.
- R. Garcia-Carbonell, A. S. Divakaruni, A. Lodi, I. Vicente-Suarez, A. Saha and H. Cheroutre,
et al., Critical Role of Glucose Metabolism in Rheumatoid Arthritis Fibroblast–like Synoviocytes, Arthritis Rheumatol., 2016, 68(7), 1614–1626 CrossRef CAS.
- X. F. Li, Y. Y. Sun, J. Bao, X. Chen, Y. H. Li and Y. Yang,
et al., Functional role of PPAR-γ on the proliferation and migration of fibroblast-like synoviocytes in rheumatoid arthritis, Sci. Rep., 2017, 7(1), 12671 Search PubMed.
- C. Ma, J. Wu, H. Lei, H. Huang and Y. Li, Significance of m6A in subtype identification, immunological evolution, and therapeutic sensitivity of RA, Immunobiology, 2024, 229(1), 152781 CrossRef CAS PubMed.
- J. Xiao, X. Cai, R. Wang, W. Zhou and Z. Ye, Identification of Synovial Fibroblast-Associated Neuropeptide Genes and m6A Factors in Rheumatoid Arthritis Using Single-Cell Analysis and Machine Learning, Dis. Markers, 2022, 2022, 1–12 Search PubMed.
- R. Ben Mrid, N. Bouchmaa, H. Ainani, R. El Fatimy, G. Malka and L. Mazini, Anti-rheumatoid drugs advancements: New insights into the molecular treatment of rheumatoid arthritis, Biomed. Pharmacother., 2022, 151, 113126 CrossRef CAS.
- S. Maity, A. Misra and S. Wairkar, Novel injectable carrier based corticosteroid therapy for treatment of rheumatoid arthritis and osteoarthritis, J. Drug Delivery Sci. Technol., 2021, 61, 102309 CrossRef CAS.
- M. Aslani, A. Ahmadzadeh, Z. Rezaieyazdi, S. S. Mortazavi-Jahromi, A. Barati and M. Hosseini,
et al., The Situation of Chemokine Ligands and Receptors Gene Expression, Following the Oral Administration of Drug Mannuronic Acid in Rheumatoid Arthritis Patients, Recent Pat. Inflamm. Allergy Drug Discovery, 2020, 14(1), 69–77 CrossRef CAS.
- M. Popovtzer, J. Robinette, C. Halgrimson and T. Starzl, Acute effect of prednisolone on renal handling of sodium, Am. J. Physiol., 1973, 224(3), 651–658 CrossRef CAS.
- M. Chester Wasko, A. Dasgupta, G. Ilse Sears, J. F. Fries and M. M. Ward, Prednisone Use and Risk of Mortality in Patients With Rheumatoid Arthritis: Moderation by Use of Disease–Modifying Antirheumatic Drugs, Arthritis Care Res., 2016, 68(5), 706–710 CrossRef CAS PubMed.
- C. L. Keller, N. T. Jones, R. B. Abadie, W. Barham, R. Behara and S. Patil,
et al., Non-steroidal Anti-inflammatory Drug (NSAID)-, Potassium Supplement-, Bisphosphonate-, and Doxycycline-Mediated Peptic Ulcer Effects: A Narrative Review, Cureus, 2024, e51894 Search PubMed.
- S. Thakur, B. Riyaz, A. Patil, A. Kaur, B. Kapoor and V. Mishra, Novel drug delivery systems for NSAIDs in management of rheumatoid arthritis: An overview, Biomed. Pharmacother., 2018, 106, 1011–1023 CrossRef CAS.
- W. Wang, H. Zhou and L. Liu, Side effects of methotrexate therapy for rheumatoid arthritis: A systematic review, Eur. J. Med. Chem., 2018, 158, 502–516 CAS.
- X. Zhang, H. Yang, X. Zuo, L. Wu, J. Peng and Z. Li,
et al., Efficacy and safety of tripterygium wilfordii Hook F plus TNF inhibitor for active rheumatoid arthritis: A multicentre, randomized, double-blind, triple-dummy controlled trial, Clin. Immunol., 2023, 255, 109749 CAS.
- J. W. Kim, Y. Y. Kim, H. Lee, S. H. Park, S. K. Kim and J. Y. Choe, Risk of Retinal Toxicity in Longterm Users of Hydroxychloroquine, J. Rheumatol., 2017, 44(11), 1674–1679 CAS.
- H. Niknahad, R. Heidari, R. Mohammadzadeh, M. M. Ommati, F. Khodaei and N. Azarpira,
et al., Sulfasalazine induces mitochondrial dysfunction and renal injury, Ren. Fail., 2017, 39(1), 745–753 CAS.
- S. Zhao, E. Mysler and R. J. Moots, Etanercept for the Treatment of Rheumatoid Arthritis, Immunotherapy, 2018, 10(6), 433–445 CAS.
- F. Zamri and T. J. de Vries, Use of TNF Inhibitors in Rheumatoid Arthritis and Implications for the Periodontal Status: For the Benefit of Both?, Front. Immunol., 2020, 11, 591365 CAS.
- T. S. Athni and S. Barmettler, Hypogammaglobulinemia, late-onset neutropenia, and infections following rituximab, Ann. Allergy, Asthma Immunol., 2023, 130(6), 699–712 CrossRef CAS.
- J. Zhang, H. Liu, Y. Chen, H. Liu, S. Zhang and G. Yin,
et al., Augmenting regulatory T cells: new therapeutic strategy for rheumatoid arthritis, Front. Immunol., 2024, 15, 1312919 CrossRef CAS.
- C. Zenobia and G. Hajishengallis, Basic biology and role of interleukin–17 in immunity and inflammation, Periodontology, 2015, 69(1), 142–159 CrossRef PubMed.
- P. Sarzi-Puttini, A. Ceribelli, D. Marotto, A. Batticciotto and F. Atzeni, Systemic rheumatic diseases: From biological agents to small molecules, Autoimmun. Rev., 2019, 18(6), 583–592 Search PubMed.
- G. Jones and E. Panova, New insights and long-term safety of tocilizumab in rheumatoid arthritis, Ther. Adv. Musculoskelet. Dis., 2018, 10(10), 195–199 CAS.
- C. J. Wruck, A. Fragoulis, A. Gurzynski, L. O. Brandenburg, Y. W. Kan and K. Chan,
et al., Role of oxidative stress in rheumatoid arthritis: insights from the Nrf2-knockout mice, Ann. Rheum. Dis., 2011, 70(5), 844–850 CAS.
- G. Manda, E. Milanesi, S. Genc, C. M. Niculite, I. V. Neagoe and B. Tastan,
et al., Pros and cons of NRF2 activation as adjunctive therapy in rheumatoid arthritis, Free Radicals Biol. Med., 2022, 190, 179–201 Search PubMed.
- J. Zhang, W. Li, M. Gong, Y. Gu, H. Zhang and B. Dong,
et al., Risk of venous thromboembolism with janus kinase inhibitors in inflammatory immune diseases: a systematic review and meta-analysis, Front. Pharmacol., 2023, 14, 1189389 Search PubMed.
- E. O. Major, Progressive Multifocal Leukoencephalopathy
in Patients on Immunomodulatory Therapies, Annu. Rev. Med., 2010, 61(1), 35–47 Search PubMed.
- T. Lowin and R. H. Straub, Integrins and their ligands in rheumatoid arthritis, Arthritis Res. Ther., 2011, 13(5), 244 CrossRef CAS PubMed.
- S. M. Lee, H. J. Kim, Y. J. Ha, Y. N. Park, S. K. Lee and Y. B. Park,
et al., Targeted chemo-photothermal treatments of rheumatoid arthritis using gold half-shell multifunctional nanoparticles, ACS Nano, 2013, 7(1), 50–57 CAS . Available from: https://pubmed.ncbi.nlm.nih.gov/23194301/.
- V. Kumar, A. Leekha, A. Tyagi, A. Kaul, A. K. Mishra and A. K. Verma, Preparation and evaluation of biopolymeric nanoparticles as drug delivery system in effective treatment of rheumatoid arthritis, Pharm. Res., 2017, 34(3), 654–667 CrossRef CAS . Available from: https://pubmed.ncbi.nlm.nih.gov/28097508/.
- N. Kishore, M. D. Raja, C. S. Kumar, U. Dhanalekshmi and R. Srinivasan, Research Article, Eur. J. Lipid Sci. Technol., 2016, 6(118), 949–958 Search PubMed . Available from: https://www.infona.pl//resource/bwmeta1.element.wiley-ejlt-v-118-i-6-ejlt201400658.
- K. Coradini, R. B. Friedrich, F. N. Fonseca, M. S. Vencato, D. F. Andrade and C. M. Oliveira,
et al., A novel approach to arthritis treatment based on resveratrol and curcumin co-encapsulated in lipid-core nanocapsules: In vivo studies, Eur. J. Pharm. Sci., 2015, 78, 163–170 CAS . Available from: https://pubmed.ncbi.nlm.nih.gov/26206297/.
- A. Bernardi, A. A. C. C. V. Zilberstein, E. Jäger, M. M. Campos, F. B. Morrone and J. B. Calixto,
et al., Effects of indomethacin-loaded nanocapsules in experimental models of inflammation in rats, Br. J. Pharmacol., 2009, 158(4), 1104–1111 CAS . Available from: https://onlinelibrary.wiley.com/doi/full/10.1111/j.1476-5381.2009.00244.x.
- R. B. Friedrich, K. Coradini, F. N. Fonseca, S. S. Guterres, R. C. R. Beck and A. R. Pohlmann, Lipid-Core Nanocapsules Improved Antiedematogenic Activity of Tacrolimus in Adjuvant-Induced Arthritis Model, J. Nanosci. Nanotechnol., 2016, 16(2), 1265–1274 CAS . Available from: https://pubmed.ncbi.nlm.nih.gov/27433576/.
- I. Pathan, M. Mangle and S. Bairagi, Design and Characterization of Nanoemulsion for Transdermal Delivery of Meloxicam, Anal. Chem. Lett., 2016, 6(3), 286–295 CAS . Available from: https://www.researchgate.net/publication/305741476_Design_and_Characterization_of_Nanoemulsion_for_Transdermal_Delivery_of_Meloxicam.
- S. B. V. Mello, E. R. Tavares, M. C. Guido, E. Bonfá and R. C. Maranhão, Anti-inflammatory effects of intravenous methotrexate associated with lipid nanoemulsions on antigen-induced arthritis, Clinics, 2016, 71(1), 54 CrossRef PubMed . Available from: https://pmc/articles/PMC4732406/.
- D. R. Wilson, N. Zhang, A. L. Silvers, M. B. Forstner and R. A. Bader, Synthesis and evaluation of cyclosporine A-loaded polysialic acid-polycaprolactone micelles for rheumatoid arthritis, Eur. J. Pharm. Sci., 2014, 51(1), 146–156 CrossRef CAS PubMed . Available from: https://pubmed.ncbi.nlm.nih.gov/24075961/.
- H. S. Helmy, A. E. El-Sahar, R. H. Sayed, R. N. Shamma, A. H. Salama and E. M. Elbaz, Therapeutic effects of lornoxicam-loaded nanomicellar formula in experimental models of rheumatoid arthritis, Int. J. Nanomed., 2017, 12, 7015–7023 CrossRef CAS . Available from: https://pubmed.ncbi.nlm.nih.gov/29026298/.
- Q. Wang, J. Jiang, W. Chen, H. Jiang, Z. Zhang and X. Sun, Targeted delivery of low-dose dexamethasone using PCL-PEG micelles for effective treatment of rheumatoid arthritis, J. Controlled Release, 2016, 230, 64–72 CrossRef CAS . Available from: https://pubmed.ncbi.nlm.nih.gov/27057749/.
- T. Liu, J. Fu, M. Chen, Q. Wu, G. Quan and C. Wu,
et al., In situ polymeric nanomicelle-generating dissolving microneedle patch for enhanced transdermal methotrexate delivery in rheumatoid arthritis treatment, Eur. Polym. J., 2024, 210, 113008 CrossRef CAS.
- R. Raj, P. Mongia, A. Ram and N. K. Jain, Enhanced skin delivery of aceclofenac via hydrogel-based solid lipid nanoparticles, Artif. Cells, Nanomed., Biotechnol., 2016, 44(6), 1434–1439 CAS . Available from: https://pubmed.ncbi.nlm.nih.gov/25919063/.
- L. H. Peng, W. Wei, Y. H. Shan, Y. S. Chong, L. Yu and J. Q. Gao, Sustained release of piroxicam from solid lipid nanoparticle as an effective anti-inflammatory therapeutics in vivo, Drug Dev. Ind. Pharm., 2017, 43(1), 55–66 CAS . Available from: https://pubmed.ncbi.nlm.nih.gov/27498809/.
- J. M. Metselaar, L. M. Middelink, C. H. Wortel, R. Bos, J. M. van Laar and H. E. Vonkeman,
et al., Intravenous pegylated liposomal prednisolone outperforms intramuscular methylprednisolone in treating rheumatoid arthritis flares: A randomized controlled clinical trial, J. Controlled Release, 2022, 341, 548–554 CAS.
- V. P. Bykerk, P. Nash, D. Nicholls, Y. Tanaka, K. Winthrop and C. Popova,
et al., Long-Term Durability of Certolizumab Pegol in Patients with Rheumatoid Arthritis Over 5 Years: An Analysis of Pooled Clinical Trial Data, Rheumatol. Ther., 2023, 10(3), 693–706 CrossRef.
- H. Y. Yap, S. Z. Y. Tee, M. M. T. Wong, S. K. Chow, S. C. Peh and S. Y. Teow, Pathogenic Role of Immune Cells in Rheumatoid Arthritis: Implications in Clinical Treatment and Biomarker Development, Cells, 2018, 7(10), 161, DOI:10.3390/CELLS7100161.
- J. Huang, X. Fu, X. Chen, Z. Li, Y. Huang and C. Liang, Promising Therapeutic Targets for Treatment of Rheumatoid Arthritis, Front. Immunol., 2021, 12, 686155 CAS.
- S. Siebert, A. Tsoukas, J. Robertson and I. McInnes, Cytokines as therapeutic targets in rheumatoid arthritis and other inflammatory diseases, Pharmacol. Rev., 2015, 67(2), 280–309 CAS . Available from: https://pubmed.ncbi.nlm.nih.gov/25697599/.
- M. A. Khan, N. Khurana, R. S. Ahmed, S. Umar, G. Md, A. H. Sarwar and Q. Alam,
et al., Chemokines: A Potential Therapeutic Target to Suppress Autoimmune Arthritis, Curr. Pharm. Des., 2019, 25(27), 2937–2946 CAS . Available from: https://pubmed.ncbi.nlm.nih.gov/31580792/.
- J. Zhang, X. Hu, X. Dong, W. Chen, L. Zhang and Y. Chang,
et al., Regulation of T Cell Activities in Rheumatoid Arthritis by the Novel Fusion Protein IgD-Fc-Ig, Front. Immunol., 2020, 11, 755 CAS . Available from: https://pmc/articles/PMC7243948/.
- J. M. Kremer, R. Westhovens, M. Leon, E. Di Giorgio, R. Alten and S. Steinfeld,
et al., Treatment of Rheumatoid Arthritis by Selective Inhibition of T-Cell Activation with Fusion Protein CTLA4Ig, N. Engl. J. Med., 2003, 349(20), 1907–1915 CAS . Available from: https://www.nejm.org/doi/full/10.1056/NEJMoa035075.
- R. J. Looney, B cell-targeted therapy for rheumatoid arthritis: An update on the evidence, Drugs, 2006, 66(5), 625–639 CAS . Available from: https://link.springer.com/article/10.2165/00003495-200666050-00004.
- W. Duan and H. Li, Combination of NF-kB targeted siRNA and methotrexate in a hybrid nanocarrier towards the effective treatment in rheumatoid arthritis, J. Nanobiotechnol., 2018, 16(1), 58 Search PubMed . Available from: https://www.researchgate.net/publication/326691990_Combination_of_NF-kB_targeted_siRNA_and_methotrexate_in_a_hybrid_nanocarrier_towards_the_effective_treatment_in_rheumatoid_arthritis.
- S. Xie, S. Li, J. Tian and F. Li, Iguratimod as a New Drug for Rheumatoid Arthritis: Current Landscape, Front. Pharmacol., 2020, 11, 73, DOI:10.3389/FPHAR.2020.00073.
- J. A. Hamilton, GM-CSF-Dependent Inflammatory Pathways, Front. Immunol., 2019, 10, 457651 Search PubMed . Available from: https://www.frontiersin.org.
- D. E. A. Greven, E. S. Cohen, D. M. Gerlag, J. Campbell, J. Woods and N. Davis,
et al., Preclinical characterisation of the GM-CSF receptor as a therapeutic target in rheumatoid arthritis, Ann. Rheum. Dis., 2015, 74(10), 1924–1930 CrossRef CAS PubMed . Available from: https://pubmed.ncbi.nlm.nih.gov/24936585/.
- T. Nanki, T. Imai and S. Kawai, Fractalkine/CX3CL1 in rheumatoid arthritis, Mod. Rheumatol., 2017, 27(3), 392–397 CrossRef CAS . Available from: https://www.tandfonline.com/doi/abs/10.1080/14397595.2016.1213481.
- Y. Tanaka, T. Takeuchi, H. Yamanaka, T. Nanki, H. Umehara and N. Yasuda,
et al., A phase 2 study of E6011, an anti-Fractalkine monoclonal antibody, in patients with rheumatoid arthritis inadequately responding to biological disease-modifying antirheumatic drugs, Mod. Rheumatol., 2021, 31(4), 783–789, DOI:10.1080/14397595.2020.1868675.
- J. Yoo, C. Park, G. Yi, D. Lee and H. Koo, Active Targeting Strategies Using Biological Ligands for Nanoparticle Drug Delivery Systems, Cancers, 2019, 11(5), 640 CAS.
- M. Bonelli, A. Kerschbaumer, K. Kastrati, K. Ghoreschi, M. Gadina and L. X. Heinz,
et al., Selectivity, efficacy and safety of JAKinibs: new evidence for a still evolving story, Ann. Rheum. Dis., 2024, 83(2), 139–160 CAS.
- S. Dhillon, Tofacitinib: A Review in Rheumatoid Arthritis, Drugs, 2017, 77(18), 1987–2001 CAS.
- S. Gorantla, E. R. Puppala, V. G. M. Naidu, R. N. Saha and G. Singhvi, Hyaluronic acid-coated proglycosomes for topical delivery of tofacitinib in rheumatoid arthritis condition: Formulation design, in vitro, ex vivo characterization, and in vivo efficacy studies, Int. J. Biol. Macromol., 2023, 224, 207–222 CAS.
- S. Nasra, D. Bhatia and A. Kumar, Recent advances in nanoparticle-based drug delivery systems for rheumatoid arthritis treatment, Nanoscale Adv., 2022, 4(17), 3479–3494 CAS.
- S. Gorantla, E. Rao Puppala, V. G. M. Naidu, R. N. Saha and G. Singhvi, Design of chondroitin sulphate coated proglycosomes for localized delivery of tofacitinib for the treatment of rheumatoid arthritis, Eur. J. Pharm. Biopharm., 2023, 186, 43–54 CrossRef CAS PubMed.
- S. Gorantla, E. Rao Puppala, V. G. M. Naidu, R. N. Saha and G. Singhvi, Design of chondroitin sulphate coated proglycosomes for localized delivery of tofacitinib for the treatment of rheumatoid arthritis, Eur. J. Pharm. Biopharm., 2023, 186, 43–54 CrossRef CAS.
- Y. Bedoui, X. Guillot, J. Sélambarom, P. Guiraud, C. Giry and M. C. Jaffar-Bandjee,
et al., Methotrexate an Old Drug with New Tricks, Int. J. Mol. Sci., 2019, 20(20), 5023 CrossRef CAS.
- J. Pradal, P. Maudens, C. Gabay, C. A. Seemayer, O. Jordan and E. Allémann, Effect of particle size on the biodistribution of nano- and microparticles following intra-articular injection in mice, Int. J. Pharm., 2016, 498(1–2), 119–129 CAS.
- A. Rubbert-Roth and A. Finckh, Treatment options in patients with rheumatoid arthritis failing initial TNF inhibitor therapy: a critical review, Arthritis Res. Ther., 2009, 11(Suppl 1), S1 Search PubMed.
- C. G. Moertel, A. J. Schutt, R. J. Reitemeier and R. G. Hahn, Phase II study of camptothecin (NSC-100880) in the treatment of advanced gastrointestinal cancer, Cancer Chemother. Rep., 1972, 56(1), 95–101 CAS.
- O. M. Y. Koo, I. Rubinstein and H. Önyüksel, Actively Targeted Low-Dose Camptothecin as a Safe, Long-Acting, Disease-Modifying Nanomedicine for Rheumatoid Arthritis, Pharm. Res., 2011, 28(4), 776–787 CAS.
- Z. A. Khan, R. Tripathi and B. Mishra, Methotrexate: a detailed review on drug delivery and clinical aspects, Expert Opin. Drug Delivery, 2012, 9(2), 151–169 CAS.
- W. Zhang, Y. Huang, J. Li, M. Zhou, W. Huang and L. Sun,
et al., Carrier-free poly(glycyrrhetinic acid)-facilitated celastrol-loaded nanoparticle for high-efficiency low-toxicity treatment of rheumatoid arthritis, Mater. Des., 2024, 241, 112951 CrossRef CAS.
- J. K. Patra, G. Das, L. F. Fraceto, E. V. R. Campos, M. D. P. Rodriguez-Torres and L. S. Acosta-Torres,
et al., Nano based drug delivery systems: recent developments and future prospects, J. Nanobiotechnol., 2018, 16(1), 1–33 CrossRef . Available from: https://jnanobiotechnology.biomedcentral.com/articles/10.1186/s12951-018-0392-8.
- G. Sanità, B. Carrese and A. Lamberti, Nanoparticle Surface Functionalization: How to Improve Biocompatibility and Cellular Internalization, Front. Mol. Biosci., 2020, 7, 587012 CrossRef PubMed . Available from: https://www.frontiersin.org.
- T. D. Clemons, R. Singh, A. Sorolla, N. Chaudhari, A. Hubbard and K. S. Iyer, Distinction Between Active and Passive Targeting of Nanoparticles Dictate Their Overall Therapeutic Efficacy, Langmuir, 2018, 34(50), 15343–15349 CrossRef CAS PubMed . Available from: https://pubmed.ncbi.nlm.nih.gov/30441895/.
- R. Bazak, M. Houri, S. E. Achy, W. Hussein and T. Refaat, Passive targeting of nanoparticles to cancer: A comprehensive review of the literature, Mol. Clin. Oncol., 2014, 2(6), 904 CrossRef PubMed . Available from: https://pmc/articles/PMC4179822/.
- J. Yoo, C. Park, G. Yi, D. Lee and H. Koo, Active Targeting Strategies Using Biological Ligands for Nanoparticle Drug Delivery Systems, Cancers, 2019, 11(5), 640 CAS . Available from: https://pubmed.ncbi.nlm.nih.gov/31072061/.
- X. Feng and Y. Chen, Drug delivery targets and systems for targeted treatment of rheumatoid arthritis, J. Drug Targeting, 2018, 26(10), 845–857 CAS . Available from: https://www.tandfonline.com/doi/abs/10.1080/1061186X.2018.1433680.
- M. Chen, K. A. Daddy, Y. Xiao, Q. Ping and L. Zong, Advanced nanomedicine for rheumatoid arthritis treatment: focus on active targeting, Expert Opin. Drug Delivery, 2017, 14(10), 1141–1144 CAS . Available from: https://www.tandfonline.com/doi/abs/10.1080/17425247.2017.1372746.
- H. Y. Yap, S. Z. Y. Tee, M. M. T. Wong, S. K. Chow, S. C. Peh and S. Y. Teow, Pathogenic Role of Immune Cells in Rheumatoid Arthritis: Implications in Clinical Treatment and Biomarker Development, Cells, 2018, 7(10), 161 CAS . Available from: https://www.mdpi.com/2073-4409/7/10/161/htm.
- D. M. S. H. Chandrupatla, C. F. M. Molthoff, A. A. Lammertsma, C. J. van der Laken and G. Jansen, The folate receptor β as a macrophage-mediated imaging and therapeutic target in rheumatoid arthritis, Drug Delivery Transl. Res., 2019, 9(1), 366–378 CrossRef CAS PubMed.
- E. Nogueira, A. C. Gomes, A. Preto and A. Cavaco-Paulo, Folate-targeted nanoparticles for rheumatoid arthritis therapy, Nanomedicine, 2016, 12(4), 1113–1126 CrossRef CAS PubMed.
- G. L. Zwicke, G. A. Mansoori and C. J. Jeffery, Utilizing the folate receptor for active targeting of cancer nanotherapeutics, Nano Rev., 2012, 3(1), 18496 CrossRef CAS . Available from: https://pmc/articles/PMC3521101/.
-
J. Minigh, Folic acid, in xPharm: The Comprehensive Pharmacology Reference, 2007, pp. 1–6. Available from: https://www.researchgate.net/publication/296667311_Folic_Acid Search PubMed.
- A. Verma, A. Jain, A. Tiwari, S. Saraf, P. K. Panda and G. P. Agrawal,
et al., Folate Conjugated Double Liposomes Bearing Prednisolone and Methotrexate for Targeting Rheumatoid Arthritis, Pharm. Res., 2019, 36(8), 1–13 Search PubMed . Available from: https://link.springer.com/article/10.1007/s11095-019-2653-0.
- M. Yang, J. Ding, Y. Zhang, F. Chang, J. Wang and Z. Gao,
et al., Activated macrophage-targeted dextran–methotrexate/folate conjugate prevents deterioration of collagen-induced arthritis in mice, J. Mater. Chem. B, 2016, 4(12), 2102–2113 RSC . Available from: https://pubs.rsc.org/en/content/articlehtml/2016/tb/c5tb02479j.
- N. Zhang, C. Xu, N. Li, S. Zhang, L. Fu and X. Chu,
et al., Folate receptor-targeted mixed polysialic acid micelles for combating rheumatoid arthritis: in vitro and in vivo evaluation, Drug Delivery, 2018, 25(1), 1182–1191 CrossRef CAS . Available from: https://www.tandfonline.com/doi/abs/10.1080/10717544.2018.1472677.
- U. Bilthariya, N. Jain, V. Rajoriya and A. K. Jain, Folate-conjugated albumin nanoparticles for rheumatoid arthritis-targeted delivery of etoricoxib, Drug Dev. Ind. Pharm., 2015, 41(1), 95–104 CrossRef CAS PubMed . Available from: https://pubmed.ncbi.nlm.nih.gov/24164469/.
-
J. W. Kuo and G. D. Prestwich, Hyaluronic Acid, in Comprehensive Biomaterials, 2011, pp. 239–259. Available from: https://www.researchgate.net/publication/281665056_Hyaluronic_Acid Search PubMed.
- D. Uebelhart and J. M. Williams, Effects of hyaluronic acid on cartilage degradation, Curr. Opin. Rheumatol., 1999, 11(5), 427–435 CrossRef CAS PubMed . Available from: https://pubmed.ncbi.nlm.nih.gov/10503666/.
- R. D. Altman, A. Manjoo, A. Fierlinger, F. Niazi and M. Nicholls, The mechanism of action for hyaluronic acid treatment in the osteoarthritic knee: a systematic review, BMC Musculoskelet. Disord., 2015, 16(1), 321, DOI:10.1186/S12891-015-0775-Z.
- M. Zhou, J. Hou, Z. Zhong, N. Hao, Y. Lin and C. Li, Targeted delivery of hyaluronic acid-coated solid lipid nanoparticles for rheumatoid arthritis therapy, Drug Delivery, 2018, 25(1), 716–722 CrossRef PubMed . Available from: https://pubmed.ncbi.nlm.nih.gov/29516758/.
- H. Lee, M. Y. Lee, S. H. Bhang, B. S. Kim, Y. S. Kim and J. H. Ju,
et al., Hyaluronate-gold nanoparticle/tocilizumab complex for the treatment of rheumatoid arthritis, ACS Nano, 2014, 8(5), 4790–4798 CrossRef CAS . Available from: https://pubmed.ncbi.nlm.nih.gov/24730974/.
- Y. s. Jung, W. Park, H. Park, D. K. Lee and K. Na, Thermo-sensitive injectable hydrogel based on the physical mixing of hyaluronic acid and Pluronic F-127 for sustained NSAID delivery, Carbohydr. Polym., 2017, 156, 403–408 CAS.
- H. A. Elshabrawy, Z. Chen, M. V. Volin, S. Ravella, S. Virupannavar and S. Shahrara, The pathogenic role of angiogenesis in rheumatoid arthritis, Angiogenesis, 2015, 18(4), 433–448 CAS . Available from: https://pubmed.ncbi.nlm.nih.gov/26198292/.
- I. V. Seoane, C. Martínez, R. García-Vicuña, A. M. Ortiz, Y. Juarranz and V. C. Talayero,
et al., Vasoactive intestinal peptide gene polymorphisms, associated with its serum levels, predict treatment requirements in early rheumatoid arthritis, Sci. Rep., 2018, 8(1), 2035, DOI:10.1038/S41598-018-20400-6.
- R. L. Wilder, Integrin alpha V beta 3 as a target for treatment of rheumatoid arthritis and related rheumatic diseases, Ann. Rheum. Dis., 2002, 61(Suppl 2), ii96-99, DOI:10.1136/ARD.61.SUPPL_2.II96.
- G. A. Koning, R. M. Schiffelers, M. H. M. Wauben, R. J. Kok, E. Mastrobattista and G. Molema,
et al., Targeting of angiogenic endothelial cells at sites of inflammation by dexamethasone phosphate-containing RGD peptide liposomes inhibits experimental arthritis, Arthritis Rheum., 2006, 54(4), 1198–1208 CrossRef CAS . Available from: https://pubmed.ncbi.nlm.nih.gov/16575845/.
- Y. Wang, Z. Liu, T. Li, L. Chen, J. Lyu and C. Li,
et al., Enhanced Therapeutic Effect of RGD-Modified Polymeric Micelles Loaded With Low-Dose Methotrexate and Nimesulide
on Rheumatoid Arthritis, Theranostics, 2019, 9(3), 708–720 CrossRef CAS PubMed . Available from: https://pubmed.ncbi.nlm.nih.gov/30809303/.
- L. East, The mannose receptor family, Biochim. Biophys. Acta, Gen. Subj., 2002, 1572(2–3), 364–386 CrossRef CAS.
- L. East and C. M. Isacke, The mannose receptor family, Biochim. Biophys. Acta, Gen. Subj., 2002, 1572(2–3), 364–386 CrossRef CAS.
- F. Sultana, M. K. Neog and M. K. Rasool, Withaferin-A, a steroidal lactone encapsulated mannose decorated liposomes ameliorates rheumatoid arthritis by intriguing the macrophage repolarization in adjuvant-induced arthritic rats, Colloids Surf., B, 2017, 155, 349–365 CrossRef CAS PubMed . Available from: https://pubmed.ncbi.nlm.nih.gov/28454064/.
- N. Igarashi, A. Takeguchi, S. Sakai, H. Akiyama, K. Higashi and T. Toida, Effect of Molecular Sizes of Chondroitin Sulfate on Interaction with L-Selectin, Int. J. Carbohydr. Chem., 2013, 2013(1), 856142 Search PubMed . Available from: https://onlinelibrary.wiley.com/doi/full/10.1155/2013/856142.
- D. Naor and S. Nedvetzki, CD44 in rheumatoid arthritis, Arthritis Res. Ther., 2003, 5(3), 105 Search PubMed . Available from: https://pubmed.ncbi.nlm.nih.gov/12723975/.
- A. Mahtab, S. A. Rabbani, Y. R. Neupane, S. Pandey, A. Ahmad and M. A. Khan,
et al., Facile functionalization of Teriflunomide-loaded nanoliposomes with Chondroitin sulphate for the treatment of Rheumatoid arthritis, Carbohydr. Polym., 2020, 250, 116926, DOI:10.1016/J.CARBPOL.2020.116926.
- S. Shilpi, S. Upadhaye, R. Shivvedi, E. Gurnany, P. Chimaniya and A. Singh,
et al., Chondroitin sulphate mediated targeted delivery of methotrexate and aceclofenac to the joints for effective management of rheumatoid arthritis, Asian J. Pharm. Pharmacol., 2019, 5(3), 495–502 CrossRef CAS.
- J. Mullol, J. N. Baraniuk, M. Pitale, T. Benfield, C. Logun and C. Picado,
et al., Vasoactive intestinal peptide (VIP) induces IL-6 and IL-8, but not G-CSF and GM-CSF release from a human bronchial epithelial cell line, Neuropeptides, 1997, 31(2), 119–124 CrossRef CAS.
- L. C. Strother, A. Srikiatkhachorn and W. Supronsinchai, Targeted Orexin and Hypothalamic Neuropeptides for Migraine, Neurotherapeutics, 2018, 15(2), 377–390 CrossRef CAS PubMed.
- R. Villanueva-Romero, I. Gutiérrez-Cañas, M. Carrión, S. Pérez-García, I. V. Seoane and C. Martínez,
et al., The Anti-Inflammatory Mediator, Vasoactive Intestinal Peptide, Modulates the Differentiation and Function of Th Subsets in Rheumatoid Arthritis, J. Immunol. Res., 2018, 2018, 6043710 Search PubMed . Available from: https://pmc/articles/PMC6092975/.
- O. M. Y. Koo, I. Rubinstein and H. Önyüksel, Actively Targeted Low-Dose Camptothecin as a Safe, Long-Acting, Disease-Modifying Nanomedicine for Rheumatoid Arthritis, Pharm. Res., 2011, 28(4), 776 CrossRef CAS . Available from: https://pmc/articles/PMC3789645/.
- S. Deng, Y. Xi, H. Wang, J. Hao, X. Niu and W. Li,
et al., Regulatory effect of vasoactive intestinal peptide on the balance of Treg and Th17 in collagen-induced arthritis, Cell. Immunol., 2010, 265(2), 105–110 CrossRef CAS PubMed . Available from: https://pubmed.ncbi.nlm.nih.gov/20716449/.
- J. Morimoto, M. Sarkar, S. Kenrick and T. Kodadek, Dextran as a generally applicable multivalent scaffold for improving immunoglobulin-binding affinities of peptide and peptidomimetic ligands, Bioconjugate Chem., 2014, 25(8), 1479–1491 Search PubMed . Available from: https://pubs.acs.org/doi/full/10.1021/bc500226j.
- S. Pirmardvand Chegini, J. Varshosaz and S. Taymouri, Recent approaches for targeted drug delivery in rheumatoid arthritis diagnosis and treatment, Artif. Cells, Nanomed., Biotechnol., 2018, 46(sup2), 502–514 CrossRef CAS.
- S. H. Kim, J. H. Kim, D. G. You, G. Saravanakumar, H. Y. Yoon and K. Y. Choi,
et al., Self-assembled dextran sulphate nanoparticles
for targeting rheumatoid arthritis, Chem. Commun., 2013, 49(88), 10349–10351 RSC . Available from: https://pubs.rsc.org/en/content/articlehtml/2013/cc/c3cc44260h.
- R. Heo, D. G. You, W. Um, K. Y. Choi, S. Jeon and J. S. Park,
et al., Dextran sulfate nanoparticles as a theranostic nanomedicine for rheumatoid arthritis, Biomaterials, 2017, 131, 15–26 CrossRef CAS PubMed . Available from: https://pubmed.ncbi.nlm.nih.gov/28371624/.
- X. Wang, W. Cao, C. Sun, Y. Wang, M. Wang and J. Wu, Development of pH-sensitive dextran-based methotrexate nanodrug for rheumatoid arthritis therapy through inhibition of JAK-STAT pathways, Int. J. Pharm., 2022, 622, 121874 CrossRef CAS.
- S. Magesh, H. Ando, T. Tsubata, H. Ishida and M. Kiso, High-affinity ligands of Siglec receptors and their therapeutic potentials, Curr. Med. Chem., 2011, 18(23), 3537–3550 CrossRef CAS PubMed . Available from: https://pubmed.ncbi.nlm.nih.gov/21756229/.
- X. L. Xu, W. S. Li, X. J. Wang, Y. L. Du, X. Q. Kang and J. B. Hu,
et al., Endogenous sialic acid-engineered micelles: a multifunctional platform for on-demand methotrexate delivery and bone repair of rheumatoid arthritis, Nanoscale, 2018, 10(6), 2923–2935 RSC.
- E. Gianchecchi, A. Arena and A. Fierabracci, Sialic Acid-Siglec Axis in Human Immune Regulation, Involvement in Autoimmunity and Cancer and Potential Therapeutic Treatments, Int. J. Mol. Sci., 2021, 22(11), 5774 Search PubMed.
- X. Wang, D. Liu, Y. Ning, J. Liu, X. Wang and R. Tu,
et al., Siglec–9 is upregulated in rheumatoid arthritis and suppresses collagen–induced arthritis through reciprocal regulation of Th17-/Treg-cell differentiation, Scand. J. Immunol., 2017, 85(6), 433–440 CAS.
- B. K. Brandley, M. Kiso, S. Abbas, P. Nikrad, O. Srivasatava and C. Foxall,
et al., Structure-function studies on selectin carbohydrate ligands. Modifications to fucose, sialic acid and sulphate as a sialic acid replacement, Glycobiology, 1993, 3(6), 633–641 Search PubMed . Available from: https://pubmed.ncbi.nlm.nih.gov/7510548/.
- X. L. Xu, W. S. Li, X. J. Wang, Y. L. Du, X. Q. Kang and J. B. Hu,
et al., Endogenous sialic acid-engineered micelles: a multifunctional platform for on-demand methotrexate delivery and bone repair of rheumatoid arthritis, Nanoscale, 2018, 10(6), 2923–2935 Search PubMed . Available from: https://pubs.rsc.org/en/content/articlehtml/2018/nr/c7nr08430g.
- S. Wang, S. Yang, X. Lai, Y. Song, L. Hu and C. Li,
et al., Sialic Acid Conjugate-Modified Liposomal Dexamethasone Palmitate Targeting Neutrophils for Rheumatoid Arthritis Therapy: Influence of Particle Size, AAPS PharmSciTech, 2021, 22(1), 16, DOI:10.1208/S12249-020-01870-2.
- W. Hui and Y. Dai, Therapeutic potential of aryl hydrocarbon receptor ligands derived from natural products in rheumatoid arthritis, Basic Clin. Pharmacol. Toxicol., 2020, 126(6), 469–474 CAS . Available from: https://pubmed.ncbi.nlm.nih.gov/31811747/.
- W. Hui and Y. Dai, Therapeutic potential of aryl hydrocarbon receptor ligands derived from natural products in rheumatoid arthritis, Basic Clin. Pharmacol. Toxicol., 2020, 126(6), 469–474 CAS.
- O. Ciftci, S. Tanyildizi and A. Godekmerdan, Protective effect of curcumin on immune system and body weight gain on rats intoxicated with 2,3,7,8-Tetrachlorodibenzo-p-dioxin (TCDD), Immunopharmacol. Immunotoxicol., 2010, 32(1), 99–104 Search PubMed . Available from: https://pubmed.ncbi.nlm.nih.gov/19821784/.
- R. Ben Mrid, N. Bouchmaa, H. Ainani, R. El Fatimy, G. Malka and L. Mazini, Anti-rheumatoid drugs advancements: New insights into the molecular treatment of rheumatoid arthritis, Biomed. Pharmacother., 2022, 151, 113126 CAS.
-
S. Shams, J. M. Martinez, J. R. D. Dawson, J. Flores, M. Gabriel and G. Garcia, et al., The Therapeutic Landscape of Rheumatoid Arthritis: Current State and Future Directions, in Frontiers in Pharmacology, Frontiers Media S.A., 2021, vol. 12 Search PubMed.
- Y. Chen, Y. Wang, X. Jiang, J. Cai, Y. Chen and H. Huang,
et al., Dimethylamino group modified polydopamine nanoparticles with positive charges to scavenge cell-free DNA for rheumatoid arthritis therapy, Bioact. Mater., 2022, 18, 409–420 CAS.
- L. Lin, S. Zhu, H. Huang, L. P. Wu and J. Huang, Chemically modified small interfering RNA targeting Hedgehog signaling pathway for rheumatoid arthritis therapy, Mol. Ther.–Nucleic Acids, 2023, 31, 88–104 CrossRef CAS PubMed.
- L. Yang, X. He, Y. Xue, D. Zhi, Q. Meng and W. Zhao,
et al., Amelioration of melittin on adjuvant-induced rheumatoid arthritis: Integrated transcriptome and metabolome, Int. J. Biol. Macromol., 2024, 270, 132293 CrossRef CAS.
- L. Yang, X. He, Y. Xue, D. Zhi, Q. Meng and W. Zhao,
et al., Amelioration of melittin on adjuvant-induced rheumatoid arthritis: Integrated transcriptome and metabolome, Int. J. Biol. Macromol., 2024, 270, 132293 CrossRef CAS PubMed.
- N. Meednu, J. Rangel-Moreno, F. Zhang, K. Escalera-Rivera, E. Corsiero and E. Prediletto,
et al., Dynamic spectrum of ectopic lymphoid B cell activation and hypermutation in the RA synovium characterized by NR4A nuclear receptor expression, Cell Rep., 2022, 39(5), 110766 CrossRef CAS.
- Y. Luo, Y. Lei, X. Guo, D. Zhu, H. Zhang and Z. Guo,
et al., CX-4945 inhibits fibroblast-like synoviocytes functions through the CK2-p53 axis to reduce rheumatoid arthritis disease severity, Int. Immunopharmacol., 2023, 119, 110163 CrossRef CAS.
- H. H. Chang, C. H. Ho, B. Tomita, A. A. Silva, J. A. Sparks and E. W. Karlson,
et al., Utilizing a PTPN22 gene signature to predict response to targeted therapies in rheumatoid arthritis, J. Autoimmun., 2019, 101, 121–130 CrossRef CAS PubMed.
- H. H. Chang, C. H. Ho, B. Tomita, A. A. Silva, J. A. Sparks and E. W. Karlson,
et al., Utilizing a PTPN22 gene signature to predict response to targeted therapies in rheumatoid arthritis, J. Autoimmun., 2019, 101, 121–130 CrossRef CAS.
- P. Sun, J. Su, X. Wang, M. Zhou, Y. Zhao and H. Gu, Nucleic Acids for Potential Treatment of Rheumatoid Arthritis, ACS Appl. Bio Mater., 2022, 5(5), 1990–2008 CrossRef CAS PubMed.
- Y. Song, S. Jo, J. Y. Chung, Y. Oh, S. Yoon and Y. L. Lee,
et al., RNA interference-mediated suppression of TNF-α converting enzyme as an alternative anti-TNF-α therapy for rheumatoid arthritis, J. Controlled Release, 2021, 330, 1300–1312 CrossRef CAS.
|
This journal is © The Royal Society of Chemistry 2025 |
Click here to see how this site uses Cookies. View our privacy policy here.