DOI:
10.1039/D4FO04561K
(Paper)
Food Funct., 2025,
16, 314-328
Optimization of sulforaphane bioavailability from a glucoraphanin-rich broccoli seed extract in a model of dynamic gastric digestion and absorption by Caco-2 cell monolayers†
Received
19th September 2024
, Accepted 22nd November 2024
First published on 5th December 2024
Abstract
Broccoli is recognized for its health benefits, attributed to the high concentrations of glucoraphanin (GR). GR must be hydrolyzed by myrosinase (Myr) to form the bioactive sulforaphane (SF). The primary challenge in delivering SF in the upper gastrointestinal (GI) tract- is improving hydrolysis of GR to SF. Here, we optimized the formulation and delivery methods to improve GR conversion and SF bioavailability. We investigated whether the combination of GR-rich broccoli seed extract powder (BSE[GR]) with Myr-rich mustard seed powder (MSP[Myr]), ± ascorbic acid (AA, a co-factor of Myr), delivered as free powder or encapsulated powder, can: (i) facilitate GR hydrolysis to SF during dynamic in vitro gastric digestion and static in vitro small intestinal digestion, and (ii) increase SF bioavailability in Caco-2 cell monolayers, a model of human intestinal epithelium. Addition of exogenous Myr increased the conversion of GR to SF in free powder during small intestinal digestion, but not during gastric digestion, where Myr activity was inhibited by the acidic environment. Capsule delivery of BSE[GR]/MSP[Myr] (w/w ratio 4
:
1) resulted in a 2.5-fold higher conversion efficiency compared to free powder delivery (72.1% compared to 29.3%, respectively). AA combined with MSP[Myr] further enhanced the conversion efficiency in small intestinal digestion and the bioavailability of SF in Caco-2 cell monolayers. Bioavailability of GR as SF, SF metabolites, and GR was 74.8% in Caco-2 cell monolayers following 30 min gastric digestion and 60 min small intestinal digestion. This study highlights strategies to optimize GR bioconversion in the upper GI tract leading to enhanced SF bioavailability.
1. Introduction
High consumption of cruciferous vegetables (broccoli, cauliflower, cabbage, etc.) is associated with a reduction in risk for various chronic conditions including cancer, cardiovascular disease and type 2 diabetes.1–4 Evidence for their protective effects in health is largely attributed to the high content of glucosinolates (GSLs).5,6 Whereas GSLs do not exhibit bioactivity per se, hydrolysis by the enzyme myrosinase (Myr, thioglucoside glucohydrolase, EC 3.2.3.1), leads to the formation of the bioactive isothiocyanates (ITCs). Myr naturally occurs in several plant sources including broccoli, mustard, and daikon radish. In the case of fresh vegetables, GSLs and Myr are present in separate cellular compartments, preventing their interaction. Food preparation such as chopping and the act of chewing, release both GSLs and Myr allowing them to react to form ITCs prior to ingestion.7,8 In the case of cooking, however, Myr is readily heat denatured resulting in the ingestion of GSLs, rather than ITCs. In this case, exogenous plant Myr, for example, in mustard seed, may be delivered along with GSLs, enhancing the conversion of GSLs to ITCs in the upper gastrointestinal (GI) tract. Alternatively, GSLs may be converted to ITCs in the human GI tract by microbiota that possess Myr-like activity.9 The activity of Myr, both within the fresh plant and following ingestion, is greatly influenced by various intrinsic and extrinsic factors including temperature, pH and concentrations of its cofactor ascorbic acid (AA).10
Broccoli, especially broccoli seeds and young broccoli sprouts, contain the highest levels of the GSL glucoraphanin (GR) among the cruciferous vegetables, about 10–100 μmol per g fresh weight.11 GR can be converted to its highly bioactive hydrolysis product, sulforaphane (SF), an ITC, by Myr present in plants or by the microbiota localized in the small intestine and in the colon. After absorption, SF is rapidly metabolized in enterocytes via the mercapturic acid pathway, forming several SF conjugates including SF-glutathione (SF-GSH), SF-cysteine (SF-CYS) and SF-N-acetylcysteine (SF-NAC).12 SF and all its conjugates can be transported across enterocytes and be quantified in blood and urine by tandem mass spectroscopy.13,14 The metabolic scheme showing GR hydrolysis to SF, and to their metabolites during digestion is shown in ESI Fig. 1.†
Despite the well-established health benefits of GR-derived SF,15,16 there are challenges in improving SF yield during GI digestion and enhancing SF bioavailability in enterocytes, given the reliance on the presence of active Myr to convert GR to SF. The limitation in relying on the gut microbiota is that the average transformation is quite low, on average ∼10%, due to high inter-individual variability in the make-up of the microbiota.17 In contrast, the inclusion of plant sources of exogenous Myr has been demonstrated to increase bioavailability of SF to as much as 30–40%.17 Thermal processing (e.g., boiling, blanching, baking, and steaming) and/or exposure to low pH (stomach acid, acidic ingredients in coingested foods) leads to partial or total inactivation of Myr, limiting conversion of GR into SF in the upper GI tract,18 therefore, reducing the bioconversion and bioavailability of dietary GR as SF. Potential solutions to overcome the loss of Myr activity and therefore enhance SF bioconversion and bioavailability include, but are not limited to: (1) addition of exogenous Myr from plant sources that have not been heat treated, (2) encapsulation of exogenous plant- sources of Myr in order to protect the enzyme from the low pH of the stomach, (3) delivering an abundance of Myr in an effort to compensate for losses of Myr activity due to acid exposure and (4) co-delivering AA, a cofactor and known enhancer of Myr activity.
Increasing the amount of consumed GR is another potential but not highly effective approach to increase SF yield during digestion.13,19–21 Delivering SF directly is also a feasible alternative, for example, by consuming freshly prepared broccoli sprout juice. It has been demonstrated that SF itself is readily bioavailable; on average 70–90% of oral SF is excreted as SF and SF metabolites in humans.22 While the bioavailability of orally delivered SF is high, SF produced from fresh broccoli sprouts is hygroscopic, tastes bitter and degrades rapidly in aqueous solution at room temperature22–24 so the storage and preparation for use of SF in clinical trials is challenging and costly. Moreover, there is wide variability in the content of GR in broccoli seeds depending on the variety of the seed and agronomic practices, making the production of standardized samples for clinical trials from seeds or sprouts challenging. Therefore, orally delivering GR alone or SF alone are not ideal approaches to achieve optimal conversion efficiency of GR to SF for consumers or for clinical trials.
To overcome these limitations, exogenous plant Myr can be combined with GR-rich sources (GR-containing broccoli seed or sprout extracts) to facilitate conversion of GR to SF during digestion. Mustard seeds are an ideal source of Myr due to their abundance of Myr and relative thermal stability.25 Previous studies have reported a 3 to 4-fold increase in the bioavailability of SF when GR is co-delivered with exogenous Myr from mustard seeds.17,25 Despite the substantial increase in conversion efficiency, these human studies still reported high interindividual variability, ranging from 1 to 40% SF bioavailability.17 Moreover, due to the cost and complexity of carrying out these clinical trials, researchers were limited to testing only a single ratio of GR : Myr, rather than investigating several different ratios to determine the most optimal ratio to enhance conversion efficiency. Therefore, to date, the optimum ratio of GR : Myr required to maximize conversion of GR to SF in humans remains unknown. The use of a dynamic in vitro gastric digestion model would allow for characterization of factors that can affect the conversion of GR to SF in the gut, i.e., the impact of dynamic gastric conditions and gastric residence time, the impact of pH, the ratio of GR : Myr, the presence of AA and the concentration of AA. As studies to date have not considered the optimal ratio of GR : Myr to optimize the conversion of GR to SF and given the inactivation of Myr at low pH (pH < 4), it is important to understand if the encapsulation of the GR source and Myr could enhance the transformation of GR to SF during GI digestion by protecting Myr during gastric digestion. Finally, while it is known that AA is a cofactor for Myr that has been well-documented to enhance the activation of Myr,26 little, if any, research has been conducted on the concentrations of AA necessary to optimize Myr activity in humans. Everything done so far to investigate conversion of GR to SF in the presence of AA has been in vitro in an industrial system.27,28 We are not aware of any studies that have considered the optimal amounts of AA to enhance conversion of GR to SF in a physiologically relevant model and this would be the first study to do so.
Therefore, the aim of the present study was to optimize GR to SF conversion and SF bioavailability by using a dynamic in vitro gastric model, the Human Gastric Simulator (HGS) coupled with a static small intestinal digestion model29,30 and Caco-2 cell monolayers as a model of intestinal epithelium absorption.31 We assessed different factors that could influence the production of SF from GR in a GR-rich broccoli seed extract (BSE[GR]) including: (i) combination with an exogenous plant source of Myr (Myr-rich mustard seed powder (MSP[Myr]) at varying ratios in the form of a homogenous free flowing powder, (ii) combination with MSP[Myr] at a ratio of 4
:
1 in the form of a homogenous powder delivered in a hydroxypropyl methylcellulose (HPMC) capsule, and (iii) combination with MSP[Myr] at a ratio of 4
:
1 with the addition of a range of concentrations of the Myr cofactor AA in the form of a homogenous powder delivered in a HPMC capsule. The information from this study will aid in the development of strategies to optimize SF bioconversion and bioavailability in the upper GI tract where SF is readily absorbed.
2. Materials and methods
2.1 Materials
Broccoli (Brassica oleracea L. var. italica) seed extract (BSE[GR], a hot water extract containing 14.5% GR, product number: BSPE-GR-13-MP02; Lot # 20200903) was provided by Brassica Protection Products LLC (Baltimore, MD). In addition to the GR, the extract contained 10.6% maltodextrin used as a carrier for the drying process (Refractance Window Drying) and 1.8% silicon dioxide, an excipient included to control for moisture and enhance stability. The remaining 73.1% is made up of the water-soluble fraction of the broccoli seeds that was extracted along with the GR, primarily carbohydrates, fiber, protein and minor amounts of lipids. The mustard (Sinapis alba aka Brassica hirta) seed powder (MSP[Myr], containing at least 200 units of Myr activity per g, product number: MP-MY-01, Lot #20210219) were provided by Brassica Protection Products LLC (Baltimore, MD). The MSP was a whole seed powder extract free of excipients. Thioglucosidase from Sinapis alba (white mustard) seed was purchased from Millipore Sigma (Burlington, MA). All chemicals used in the in vitro HGS digestion model were purchased from ThermoFisher Scientific (Waltham, MA), except for mucin, CaCl2(H2O)2, pancreatin and ascorbic acid (AA) (Millipore Sigma, Burlington, MA), as well as α-amylase and pepsin (MP Biomedicals, Solon, OH). The vegetarian HPMC capsules, brand name Vcaps®, were a generous gift from Lonza Capsules & Health Ingredients (Morristown, NJ). The capsule filling machine was purchased from Capsule Connection LLC (Prescott, AZ). SF used in the Caco-2 experiments was purchased from Cayman Chemical (Ann Arbor, MI).
The standards of GR, SF, SF-GSH, SF-CYS, and SF-NAC for chromatographic analysis were purchased from Toronto Research Chemical (Toronto, Canada). All LC-MS grade solvents were obtained from ThermoFisher Scientific (Waltham, MA). The human Caco-2 cells were purchased from the American Type Culture Collection (ATCC). MEM cell culture media, fetal bovine serum (FBS) and Hanks’ Balanced Salt solution (HBSS) were obtained from Gibco (Waltham, MA). The Millicell cell culture inserts were from EMD Millipore (Hayward, CA).
2.2 Methods
2.2.1 GR and SF extraction from raw materials BSE[GR]and MSP[Myr] powder.
GR and SF in BSE[GR]and MSP[Myr] powder were extracted. Briefly, 1 mg of the BSE[GR] or MSP[Myr] powder was dissolved in 10 mL water/methanol (7/3, v/v), and sonicated for 30 min. The supernatant was collected after centrifugation at 12
000g for 15 min, and the leftover was subjected to two more extractions. The extracts from all three extractions were combined and made up to 50 mL with water, and for further GR/SF analysis.
2.2.2 Myr activity determination.
Myr activity in the pure Myr and MSP[Myr] powder was assessed by measuring the sinigrin disappearance rate as previously described, with modifications.32,33 To determine if the Myr activity was inhibited at pH 2 and its activity was recovered at pH 6, the pure Myr thioglucosidase enzyme from Sinapis alba seed (stock 25 U mL−1) was used for the experiments. Pure Myr (10 μL) was pre-incubated with and without HCl (final concentration of Myr 0.25 U mL−1) at pH 2 for 30 min at 37 °C. Following the pre-incubation, for the Myr activity assessment, 20 μL of Myr–HCl solution (0.25 U mL−1) was added to 765 μL of pH 6 sodium phosphate buffer together with 500 μM AA (10 μL) and 50 μM sinigrin (5 μL). For the MSP[Myr] powder, an aliquot (1 μL) of MSP[Myr] (20 mg powder per mL) was added to a quartz cuvette containing 20 mM sodium phosphate buffer (pH 6.0) (784 μL), 500 μM AA (10 μL) and 50 μM sinigrin (5 μL). The enzymatic reaction was followed and recorded every 2 s for a total of 3 min at 227 nm using a GENESYS 180 UV-VIS spectrophotometer (Thermo Fisher, Walthman, MA). Enzymatic activity (1 unit) was defined as the amount required to catalyze the hydrolysis of 1 μmol sinigrin per min. A molar extinction coefficient of ε = 7254 M−1 cm−1 for sinigrin at 227 nm was used.
2.2.3 Dynamic gastrointestinal digestion studies.
Following the evaluation of BSE[GR] and MSP[Myr] powder, dynamic gastric digestion and static small intestinal digestion of various combinations were investigated: (1) varying ratios of BSE[GR]
:
MSP[Myr] (w/w) delivered as a free (non-encapsulated) homogeneous powder mixture, (2) a 4
:
1 BSE[GR]
:
MSP[Myr] (w/w) homogeneous powder delivered in a HPMC capsule and (3) a 4
:
1 BSE[GR]
:
MSP[Myr] (w/w) homogeneous powder delivered in a HPMC capsule with or without the inclusion of various concentrations of AA (see Table 1).
Table 1 Ratios of BSE[GR] and MSP[Myr] and AA used in the present experiments
|
Free powder experiments |
A |
BSE (mg) |
GR (mg) |
MSP (mg) |
Myr (units) |
BSE[GR] : MSP[Myr] (w/w) |
|
1000 |
145 |
0 |
0 |
1 : 0 |
|
1000 |
145 |
50 |
22 |
20 : 1 |
|
1000 |
145 |
100 |
45 |
10 : 1 |
|
1000 |
145 |
250 |
112 |
4 : 1 |
|
1000 |
145 |
1000 |
448 |
1 : 1 |
|
Encapsulated powder experiments |
B |
BSE (mg) |
GR (mg) |
MSP (mg) |
Myr (units) |
BSE[GR] : MSP[Myr] (w/w) |
|
380 |
55 |
0 |
0 |
1 : 0 |
|
380 |
55 |
95 |
42.6 |
4 : 1 |
C |
BSE (mg) |
GR (mg) |
MSP (mg) |
Myr (units) |
AA (mg) |
BSE[GR] : MSP[Myr] (w/w) |
BSE, broccoli seed extract; GR, glucoraphanin; MSP, mustard seed powder; Myr, myrosinase; AA, ascorbic acid. |
|
380 |
55 |
0 |
0 |
0 |
1 : 0 : 0 |
|
380 |
55 |
95 |
42.6 |
0 |
4 : 1 : 0 |
|
380 |
55 |
95 |
42.6 |
11 |
4 : 1 : 0.1 |
|
380 |
55 |
95 |
42.6 |
44 |
4 : 1 : 0.5 |
|
380 |
55 |
95 |
42.6 |
88 |
4 : 1 : 0.9 |
|
380 |
55 |
95 |
42.6 |
154 |
4 : 1 : 1.6 |
Simulated gastrointestinal fluid preparation.
Simulated salivary and gastric fluids were prepared following the INFOGEST standard protocol with minor modifications in mucin content and pH.34,35 The specific composition of each fluid is shown in ESI Table S1.† All fluids were preheated to 37 °C before experiments. CaCl2(H2O)2, α-amylase, pepsin and pancreatin were added to the warm fluids immediately before each experiment. All fluids were re-adjusted to pH 1.8 (gastric fluids) or 7.0 (saliva and intestinal fluids) before and after enzyme addition.
Dynamic gastric digestion.
Gastric digestion was mimicked using the Human Gastric Simulator v2.0 (HGS).29,30,36 Simulated gastric fluid (35 mL) was preloaded into the HGS to represent the average gastric fluid present in the stomach during fasting.37 The free powder or encapsulated powder was mixed with 1 mL simulated saliva and 250 mL water (representing an approx. 8 oz. glass that may typically be consumed with a supplement) for 1 min to simulate oral digestion and added to the HGS.38 The HGS contractions and the gastric fluid secretion began immediately after the powder mixture or encapsulated powder were introduced into the HGS and were continued at 3.5 mL min−1.30 A sample of powder or capsule mixed with water was collected at time 0 prior to addition of salivary and digestive fluids. Gastric digesta samples were collected after 15, 30, 60, 90, or 120 min of gastric digestion, unless stated otherwise. The emptying rate of the gastric digesta was set as 4.5 mL min−1.30 Aliquots (1 mL) of the gastric digesta at each time point were reserved for analysis of GR and SF. To observe changes in pH of gastric digesta during the dynamic gastric digestion, the initial pH of the mixture of BSE[GR] + MSP[Myr] prepared in 250 mL of water was measured. In the digestion experiments of this treatment, after 15, 30, 60, 90, or 120 min of gastric digestion, the pH of the emptied gastric digesta was measured using a pH meter.
Static small intestinal digestion.
Small intestinal digestion was completed in an orbital shaking water bath at 37 °C and 100 rpm according to previous studies.35,39 Aliquots (3 mL) of gastric digesta from each gastric digestion time point were mixed with simulated small intestinal fluid (4
:
1 v/v small intestinal fluid : gastric digesta) to generate the small intestinal digesta.40 After 60 or 120 min small intestinal digestion, aliquots (1 mL) of the small intestinal digesta were reserved for analysis of GR and SF.
2.2.4 Digestion of BSE[GR]/MSP[Myr] free powder.
A free powder mixture of BSE[GR](1000 mg BSE; 145 mg (332 μmol) GR) and MSP[Myr] (0, 50, 100, 250, 1000 mg) made up of different ratios of BSE[GR]
:
MSP[Myr] (w/w, 1
:
0, 20
:
1, 10
:
1, 4
:
1, 1
:
1), such that the amount of GR remained constant, but the level of Myr activity was increased with each ratio (Table 1A), was subjected to gastric and small intestinal digestion (Fig. 1). Samples of HGS gastric digesta and small intestinal digesta at different time points as described above were collected for GR and SF analysis.
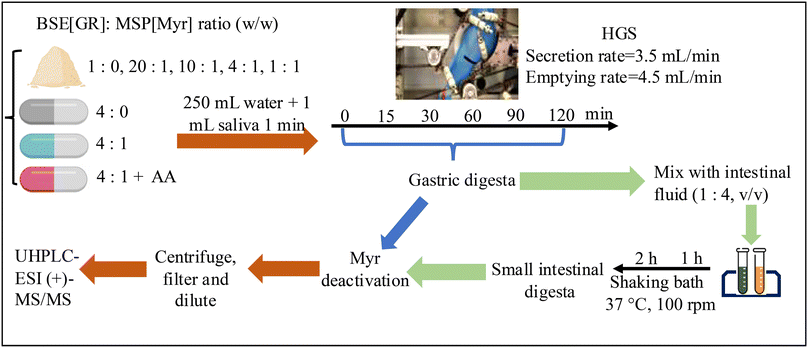 |
| Fig. 1 Experimental design. Processing procedures of dynamic gastric and small intestinal digestion. BSE[GR], broccoli seed extract; MSP[Myr], mustard seed powder; Myr, myrosinase; AA, ascorbic acid; HGS, human gastric simulator. | |
2.2.5 Digestion of BSE[GR]/MSP[Myr] capsule.
Capsules containing BSE[GR] (380 mg BSE; 55 mg (126 μmol) GR) and MSP[Myr] (95 mg MSP, 42.6 units Myr activity) in a ratio of 4
:
1 were prepared using commercial HPMC capsules and a capsule machine (Table 1B). Single capsules were subjected to dynamic gastric digestion in the HGS, and static small intestinal digestion as described above. Samples of HGS gastric digesta and small intestinal digesta at different time points as described above were collected for GR and SF analysis.
2.2.6 Digestion of BSE[GR]/MSP[Myr]/AA capsule.
AA is a co-factor of Myr and has been demonstrated to enhance the activity of Myr in vitro.26 To investigate whether inclusion of AA increased Myr activity in the present model, the impact of varying concentrations of AA on the conversion of GR to SF in the presence of Myr, BSE[GR](380 mg BSE; 55 mg (126 μmol) GR) and MSP[Myr] (95 mg; 42.6 units Myr activity) were combined with 0, 11, 44, 88 or 154 mg (0, 63, 251, 502 or 878 μmol, respectively) AA to form a homogenous mixture (Table 1C), and then encapsulated as previously described. Based on the gastric kinetics obtained for the capsules containing only BSE[GR] + MSP[Myr], HGS gastric samples were taken at only 30 min gastric digestion, and then subjected to 60 or 120 min of small intestinal digestion.
2.2.7 SF bioavailability of the post-digestive capsules assessed in Caco-2 cell monolayers.
Caco-2 cell culture and Caco-2 cell monolayers.
SF bioavailability was evaluated in an in vitro model using human Caco-2 cells grown in permeable inserts, which allows for the differentiation of cells with the typical apical and basolateral characteristics of intestinal epithelial cells.41 Caco-2 cells were cultured at 37 °C and 5% (v/v) CO2 atmosphere in minimum essential medium (MEM) supplemented with 10% (v/v) FBS, antibiotics (50 U ml−1 penicillin and 50 μg mL−1 streptomycin), 1% (v/v) of 100× non-essential amino acids, and 1 mM sodium pyruvate. The medium was replaced every 2 d during cell growth and differentiation. Cells were used between passages 5 and 20. For the experiments, Caco-2 cells were differentiated into polarized monolayers by culture for 21 days on 6-well plate polyester membrane permeable support inserts (30 mm, 0.4 μm pore size) at a density of 1 × 105 cells per well. Before and after experiments, the functionality of the monolayers was assessed by measuring the transepithelial electrical resistance (TEER) using a Millicell-ERS Resistance System (Millipore, Bedford, MA) that includes a dual electrode volt ohmmeter.
SF incubation in Caco-2 cell monolayers.
A kinetic study was performed to determine the optimal incubation time in Caco-2 cell monolayers. Freshly prepared SF (10 μM in HBSS) was added to the upper chamber (which mimics the GI lumen), and 750 μL HBSS was added to the lower chamber (which mimics the circulation) of the Caco-2 cell monolayers. After incubation for 1, 2, 4, and 6 h, the solutions in both chambers were collected. Cells were collected by HBSS, sonicated for 20 min on ice and centrifuged at 12
000g for 20 min at 4 °C to collect the supernatant for analysis of GR, SF and SF metabolites.
Small intestinal digesta incubation in Caco-2 cell monolayers.
To investigate the SF bioavailability from the small intestinal digesta, samples from encapsulated powder experiments were used due to the significantly higher GR to SF conversion as compared to the non-encapsulated powder mixtures. Three different freshly prepared intestinal digesta samples were obtained: (1) B capsule (380 mg BSE[GR]), (2) BM capsule (380 mg BSE[GR] + 95 mg MSP[Myr]), and (3) BMA capsule (380 mg BSE[GR] + 95 mg MSP[Myr] + 154 mg AA). For these experiments, dynamic gastric digestion was conducted for 30 min, followed by 60 min static small intestinal digestion. The small intestinal digesta was placed into the apical side of Caco-2 cell monolayers and experiments were conducted as described above for pure SF solutions. After incubation for 4 h in Caco-2 cell monolayers, solutions in both chambers and cells were all collected and prepared as described above to determine GR, SF and SF metabolites.
2.2.8 UHPLC-ESI (+)-MS/MS determinations.
GR, SF and SF metabolites from BSE[GR] and MSP[Myr] were resolved chromatographically and quantified by ultra high-pressure liquid chromatography (UHPLC) coupled with a 6460 tandem mass spectrometer (MS) with an Agilent Jetstream ESI source (UHPLC-ESI (+)-MS/MS; Agilent Technologies, CA) according to our previous study.14 A poroshell 120 Bonus-RP column (2.1 × 150 mm, 2.7 μm) equipped with a Bonus-RP guard column (2.1 × 5 mm, 2.7 μm) (Agilent Technologies, CA) was used to separate the target compounds. Dynamic multiple reaction monitoring was performed in positive ion mode to characterize and quantify the target compounds.
Gastric and small intestinal digesta samples were immediately treated with DMSO/ACN (1/1 v/v) upon collection to denature the Myr enzyme and prevent artefactual conversion of GR to SF. All digesta samples were measured immediately or stored at −20 °C for less than 1 week when necessary for further analysis of GR, SF and SF metabolites.
Conversion efficiency (CE) of GR to SF in digesta was calculated by:
|  | (1) |
Cell and medium samples were analyzed immediately upon collection. SF bioavailability was calculated by:39
|  | (2) |
2.2.9 Data and statistical analysis.
HPLC peak areas were obtained in triplicate for each of the three independent measurements of each sample. HPLC peak areas were averaged, and the standard deviation (SD) was determined from the average of the three measurements. All other experiments were performed using 3 replicates for each of the 3–5 independent experiments. Data were tested for normality and homogeneity of variance. Statistical differences were analyzed by unpaired t-test, paired t-test, one-way Analysis of Variance (ANOVA), or repeated-measures ANOVA (rm-ANOVA) using GraphPad Prism Ver. 8.0 (La Jolla, CA) or SAS Studio (Cary, North Carolina). Fisher's least significance difference test was used to examine differences between group means. For the rm-ANOVA, Tukey-HSD was used for post-hoc analysis. A p < 0.05 was considered statistically significant.
3. Results and discussion
3.1 Assessment of BSE[GR] and MSP[Myr]
The content of GR in BSE[GR] was 145 mg (332 μmol) per 1000 mg (14.5%, w/w) of fresh powder weight. Given that hot extraction (boiling water) was used to denature Myr during broccoli seed extract production, limited or no conversion of GR to SF was expected in the absence of exogenous Myr. As expected, the measured concentration of SF in the BSE was very low (0.012%, w/w).
The MSP[Myr] was prepared by removing the seed coat and grinding the remaining mustard seed remnant into a homogenous powder. As anticipated, GR and SF were not detected in the MSP. The Myr activity assessed in MSP[Myr] was 448 ± 22 U g−1 powder. The Myr activity delivered in the powder mixtures of different ratios was as follows: 1000 mg MSP = 448 U; 250 mg MSP = 112 U; 100 mg MSP = 44.8 U; 50 mg MSP = 22.4 U. The Myr activity for the encapsulated MSP was 95 mg MSP = 42.6 U.
3.2 Kinetics of dynamic digestion of the BSE[GR]/MSP[Myr] free powder
To investigate the effects of MSP[Myr] activity on conversion of GR to SF in the gastric digesta (stomach) and small intestinal digestion, BSE[GR] and MSP[Myr] mixtures were prepared at different ratios (1
:
0, 1
:
1, 4
:
1, 10
:
1, 20
:
1), such that the same amount of GR was delivered with increasing amounts of Myr activity.
3.2.1 Gastric digestion of the BSE[GR]/MSP[Myr] free powder.
The emptied amount of GR varied at each time point during the entire 120 min gastric digestion period and decreased over time due to continuous secretion of gastric fluids and emptying of gastric digesta (Table 2). Including the GR content in the leftover gastric digesta, the total emptied GR ranged from 132.6–140.1 mg (303.8–322.4 μmol) across all ratios (compared to 145 mg (332 μmol) GR added to the HGS), resulting in over 91% recovery of GR from the HGS. Small amounts of SF (0.10–2.70 mg, 0.6–15.2 μmol) were detected in all treatments (except for the 1
:
0 ratio which did not contain Myr), due to the conversion of GR by Myr. The CE (across all gastric time points) of GR to SF was quite low, ranging from 0.1–4.8% but, predictably, increased with increasing amounts of Myr in the powder mixtures (ratio 1
:
1 > 4
:
1 > 10
:
1 > 20
:
1 > 1
:
0). The CE at the 10
:
1 ratio was significantly higher compared to 20
:
1 (2.9 ± 0.04% vs. 0.1 ± 0.01%, respectively), while the 1
:
1 ratio resulted in the highest CE, significantly higher than all ratios tested other than the 4
:
1 ratio (Table 2). As the CE was not significantly different between the 1
:
1 and 4
:
1 ratios (4.8 ± 0.05% vs. 3.8 ± 1.3%, respectively); this was a major consideration for the selection of the 4
:
1 ratio for testing in the capsule experiments.
Table 2 GR and SF content (mg) emptied from HGS at different gastric digestion times with different ratios of BSE[GR]
:
MSP[Myr] powder (w/w)
Time (min) |
Ratio 1 : 0 |
Ratio 20 : 1 |
Ratio 10 : 1 |
Ratio 4 : 1 |
Ratio 1 : 1 |
GR |
SF |
GR |
SF |
GR |
SF |
GR |
SF |
GR |
SF |
The total amount of broccoli seed extract (BSE) was 1000 mg. GR content in BSE was 14.5% (145 mg (322 μmol)). Mustard seed powder (MSP) was 1000 mg (ratio 1 : 1, 448 Units of Myr activity), 250 mg (ratio 4 : 1, 112 Units of Myr activity), 100 mg (ratio 10 : 1, 44.8 Units of Myr activity) and 50 mg (ratio 20 : 1, 22.4 Units Myr activity). At 0 min, powder mixture was dissolved in 250 mL water. The emptied amount was based on measured concentration by UHPLC-ESI (+)-MS/MS and gastric emptying rate (4.5 mL min−1). Results are shown as mean ± SD of 3 replicates of 3–5 independent experiments. Conversion efficiency (% CE) is based on total produced SF (μmol)/total added GR (μmol) × 100. Means of GR and SF and %CE with different superscript letters in the same row are significantly different at p < 0.05 by one-way ANOVA analysis. ND: Not detected. |
0 |
0.6 ± 0.05 |
ND |
0.6 ± 0.03 |
0.001 ± 0 |
0.6 ± 0.05 |
0.001 ± 0 |
0.6 ± 0.03 |
0.001 ± 0 |
0.6 ± 0.03 |
0.001 ± 0 |
15 |
26.3 ± 1.1 |
ND |
25.3 ± 0.5 |
0.04 ± 0.05 |
28.3 ± 4.9 |
0.3 ± 0.01 |
25.5 ± 2.1 |
0.4 ± 0.1 |
25.6 ± 0.1 |
0.6 ± 0.02 |
30 |
23.9 ± 1.1 |
ND |
19.6 ± 1.8 |
0.05 ± 0.04 |
17.4 ± 3.9 |
0.2 ± 0.01 |
21.0 ± 2.2 |
0.4 ± 0.1 |
24.6 ± 0.8 |
0.5 ± 0.02 |
60 |
34.9 ± 6.4 |
ND |
36.4 ± 0.8 |
ND |
32.0 ± 3.7 |
0.5 ± 0.05 |
31.4 ± 1.7 |
0.6 ± 0.2 |
33.9 ± 0.6 |
0.8 ± 0.02 |
90 |
23.6 ± 1.8 |
ND |
25.3 ± 0.2 |
ND |
23.1 ± 1.5 |
0.4 ± 0.01 |
24.9 ± 1.1 |
0.4 ± 0.1 |
21.8 ± 0.2 |
0.4 ± 0.01 |
120 |
13.5 ± 0.04 |
ND |
16.6 ± 0.3 |
ND |
18.0 ± 3.2 |
0.3 ± 0.02 |
15.7 ± 3.9 |
0.2 ± 0.1 |
11.3 ± 0.1 |
0.2 ± 0.02 |
Leftover |
15.3 ± 0.8 |
ND |
16.4 ± 0.9 |
ND |
17.3 ± 3.0 |
ND |
16.6 ± 1.1 |
0.2 ± 0.1 |
14.6 ± 0.4 |
0.2 ± 0.01 |
Total (mg) |
138.2 ± 9.9a |
ND |
140.1 ± 2.3a |
0.1 ± 0.09a |
139.1 ± 2.8a |
1.7 ± 0.07b |
136.3 ± 5.8a |
2.2 ± 0.7b |
132.6 ± 1.0a |
2.7 ± 0.01b |
Total (μmol) |
316.2 ± 22.7a |
ND |
329.2 ± 7.5a |
0.6 ± 0.01a |
318.4 ± 6.5a |
9.6 ± 0.3b |
310.3 ± 13.6a |
12.5 ± 4.2b |
303.8 ± 2.4a |
15.2 ± 0.1b |
% CE |
0a |
0.1 ± 0.01%a |
2.9 ± 0.04%b |
3.8 ± 1.3%b,c |
4.8 ± 0.05%c |
It is well known that the stability and activity of Myr is influenced by a range of intrinsic and extrinsic factors such as temperature, pH and incubation time.42–45 The optimum temperature and pH for Myr activity from yellow mustard seeds (Sinapis alba aka Brassica hirta) are reported to be 50–60 °C and 6.0, respectively.46,47 Lower pH values (pH < 4) are known to inhibit Myr activity, resulting in as much as a 70% reduction in activity compared to pH 6.0.46 During the process of gastric digestion, the pH of the solution decreased from an initial value of 3.8 which occurred when the BSE[GR] + MSP[Myr] powder mixtures were dissolved in 250 mL of water, to pH 1.8 after 120 min gastric digestion (Fig. 2A). These findings suggest that the CE of GR to SF during gastric digestion is very limited (<5%), likely due to the inhibition of Myr activity by the low pH of the gastric conditions. Unfortunately, it was not possible to measure Myr activity in the gastric samples due to interference of gastric juices with the Myr activity assay. Therefore, the effects of low pH on Myr activity were evaluated using a Myr standard from white mustard powder in a closed system. It was also observed that after only 30 min incubation in HCl at pH 2, Myr activity was completely abolished (data not shown), but when pH was returned to pH 6, Myr activity was recovered by 69%, a significant reduction from baseline (Fig. 2B). Similarly, a recent pilot study also reported that gastric acidity reduced the bioavailability of GR in vivo.48 It is clear from our data that Myr activity is inhibited by exposure to the low pH of the stomach, inhibiting GR conversion to SF in the gastric environment, but that Myr activity may partially recover with increases in pH.
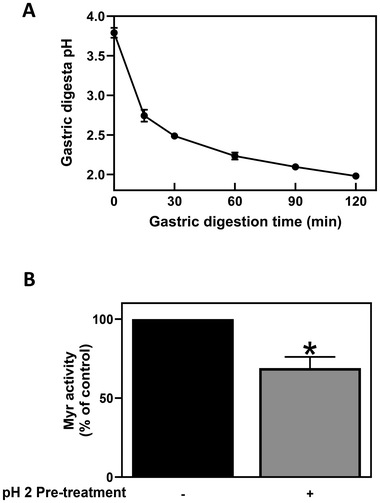 |
| Fig. 2 pH fluctuation during gastric digestion and the effect of pH on Myr activity. (A) Changes in the pH of gastric digesta during the 2 h gastric digestion. Initial pH was measured after the BSE[GR] and MSP[Myr] free powder mixture was dissolved in 250 mL of water. After gastric digestion of 15, 30, 60, 90, 120 min, gastric digesta was collected at each time point and pH was measured separately. (B) Myr activity with or without pretreatment in pH 2 solution. Pure Myr was pre-incubated in pH 2 HCl for 30 min and the Myr activity was measured in pH 6 sodium phosphate buffer. Results are shown as mean ± SD of three independent experiments. *Indicates statistically significant by unpaired t-test, p < 0.05. | |
3.2.2 Small intestinal digestion of the BSE[GR]/MSP[Myr] free powder.
The cumulative production of SF and CE of GR to SF during small intestinal digestion was assessed after 60 and 120 min following gastric digestion for 15, 30, 60, 90 and 120 min (Fig. 3 and ESI Table S2†). The total added GR in each digestion was 332 μmol (1000 mg BSE, 145 mg GR). In the case of the 1
:
0 ratio (no Myr), no SF was detected during 60 or 120 min of small intestinal digestion, as expected. After 120 min gastric digestion and 60 min small intestinal digestion with increasing amounts of Myr, significantly increasing amounts of total SF were produced, with 5.9, 34.2, 60.3 and 127.8 μmol produced for the 20
:
1, 10
:
1, 4
:
1 and 1
:
1 powder mixture ratios, respectively (Fig. 3A). By comparing the amount of total GR added to the HGS, the cumulative CE of GR to SF was 1.8, 13.2, 18.2 and 38.5% for the 20
:
1, 10
:
1, 4
:
1 and 1
:
1 powder mixtures, respectively (Fig. 3C), such that the highest Myr activity led to the highest CE. Similarly, after 120 min gastric digestion and 120 min of small intestinal digestion, the cumulative amount of SF produced was 10.4, 69.0, 97.4 and 132.1 μmol for the 20
:
1, 10
:
1, 4
:
1 and 1
:
1 powder mixtures, respectively (Fig. 3B), where the higher amounts of Myr present elicited significantly higher SF production. This resulted in cumulative CE of 3.1, 20.8, 29.3 and 39.8% for the 20
:
1, 10
:
1, 4
:
1 and 1
:
1 powder mixtures, respectively (Fig. 3D).
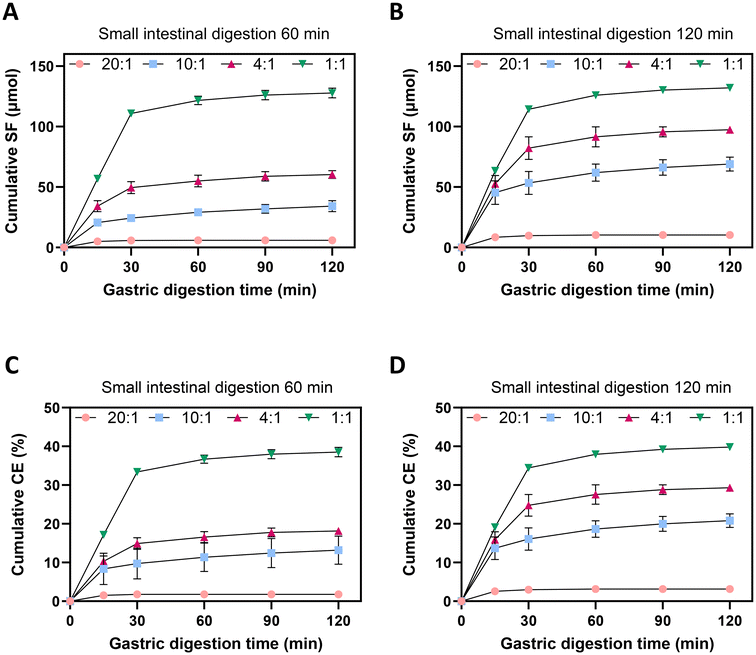 |
| Fig. 3 (A, B) Cumulative SF produced (μmol) and (C, D) conversion efficiency (% CE) during small intestinal digestion compared to total GR added to the HGS. The amount of BSE was 380 mg. GR content in BSE was 14.5% (55 mg (126 μmol)). MSP was 95 mg (42.1 units of Myr activity) (ratio 4 : 1). % CE is based on total produced SF (μmol)/total added GR (μmol) × 100. All capsules were digested in the HGS for 120 min and then in small intestinal digestion for a further 60 or 120 min. Results are shown as mean ± SD of 3 independent experiments. Repeated-measures ANOVA was performed and statistically significant at p < 0.05. | |
Notably, CE of GR to SF was higher during the 120 min small intestinal incubation compared to the 60 min incubation for the 20
:
1, 10
:
1, and 4
:
1 ratios, but not the 1
:
1 ratio of the free powder mixture. It is unknown why the longer intestinal digestion time, 120 min vs. 60 min, led to increased CE in the lower Myr blends, but not in the highest Myr activity blend (1
:
1 ratio). One explanation could be that the amount of Myr activity in the 1
:
1 blend was high enough to be saturating and therefore maximal GR conversion occurred more rapidly, within the first 60 min of small intestinal digestion. Another possibility is that starting with a higher Myr activity resulted in a faster and/or greater recovery of Myr activity following exposure to the low pH of the stomach. In the present study, only one level of Myr activity was evaluated for recovery following 30 min incubation at pH 2 followed by incubation at pH 6 (Fig. 2B). Because of the above, future studies are needed to evaluate if a higher ratio of Myr
:
GR, for example 2
:
1, could improve the CE reported in this study (∼30%), and if the amount of Myr activity could influence enzyme activity recovery from exposure to low pH. Of note, the majority of SF (over 75%) produced in the small intestinal digesta was derived from the emptied digesta from 15 and 30 min gastric digestion suggesting that in our model, GR and Myr were rapidly emptied from the gastric system (ESI Table S2†).
As discussed above, the concentration of GR in gastric digesta decreased due to the continuous gastric fluid secretion, and the emptying of digesta throughout the process. The acidic pH in the gastric fluid inhibited Myr activity, with limited recovery upon pH adjustment (Fig. 2B). The low GR concentration and prolonged low-pH exposure of Myr likely led to the limited conversion of GR to SF in small intestinal digesta after 60–120 min gastric digestion and 60 or 120 min small intestinal digestion (Table 3).
Table 3 Cumulative SF produced (μmol) and conversion efficiency (% CE) during small intestinal digestion compared to total GR added in capsule at a ratio of 4
:
1 BSE[GR]
:
MSP[Myr] powder (w/w)
Gastric digestion (min) |
Gastric GR emptied (μmol) |
Intestinal digestion (min) |
Cumulative intestinal SF produced (μmol) |
Cumulative CE (%) |
The amount of BSE was 380 mg. GR content in BSE was 14.50% (55 mg (126 μmol)). MSP was 95 mg (42.1 units of Myr activity). % CE is based on total produced SF (μmol)/total added GR (μmol) × 100. All capsules were digested in the HGS for 120 min and then in small intestinal digestion for a further 60 or 120 min. Results are shown as mean ± SD of 3 replicates of 3 independent experiments. After 60 min, no GR was detected in the emptied gastric digesta, such that measurements were not made on the small intestinal digesta for those samples. Means of gastric GR emptied, cumulative SF produced and % CE with different superscript letters in the same column are significantly different at p < 0.05 by one-way ANOVA analysis. ND: Not detected. |
15 |
ND |
60 |
ND |
ND |
30 |
123.7 ± 8.1a |
60 |
51.5 ± 8.7a |
40.1 ± 6.0a |
60 |
1.5 ± 0.5b |
60 |
52.9 ± 2.3a |
42.0 ± 1.8a |
90 |
ND |
60 |
— |
— |
120 |
ND |
60 |
— |
— |
|
15 |
ND |
120 |
ND |
ND |
30 |
123.7 ± 8.1a |
120 |
89.4 ± 6.2b |
70.9 ± 4.9b |
60 |
1.5 ± 0.5b |
120 |
90.9 ± 7.0b |
72.1 ± 5.6b |
90 |
ND |
120 |
— |
— |
120 |
ND |
120 |
— |
— |
To our knowledge, this is the first study to investigate the effects of different levels of Myr activity relative to GR in a physiologically relevant system, nor are we aware of any human clinical trials that have studied this factor. Based on findings from the present study, higher levels of Myr activity are required to increase CE when ingredients are delivered as a free powder. A ratio of 1
:
1 BSE[GR]
:
MSP[Myr] led to a CE of only 39.8%, suggesting that even higher levels of Myr activity may be required to account for the reduced activity caused by the low pH of the stomach and achieve optimal CE when delivering a free powder, a hypothesis that should be tested in future studies. A tablet delivery, as opposed to a capsule, would be more analogous to the free powder delivery examined here, as the active ingredients are not protected by a capsule and are therefore available to directly interact with the low pH of the gastric juices. Unfortunately, due to cost and other challenges, previous studies only evaluated a single GR
:
Myr ratio, and Myr activity was rarely reported, making it very difficult to directly compare results to the present study. Findings from the present study may inform researchers and developers of supplements for clinical trials or dietary supplements that higher Myr activity is necessary to enhance the CE in free powder or tablet delivery formats.
3.3 Effects of BSE[GR]/MSP[Myr] powder encapsulation on gastric and small intestinal GR to SF conversion
Considering that capsules are the most common delivery format for GR and Myr and the necessity of protecting Myr activity from the low pH of gastric fluids, a commercial HPMC capsule was used to incorporate 380 mg BSE (55 mg (126 μmol) GR) and 95 mg MSP[Myr] (42.6 Units of Myr activity). This combination represents the 4
:
1 ratio of BSE[GR]
:
MSP[Myr] tested as a free powder in the above experiments. This ratio was selected for testing in the capsule format because it allowed for the inclusion of 55 mg GR in a single capsule, a commonly studied dose of GR that has been demonstrated to positively impact inflammatory markers in human clinical trials.49–51 In the simulated gastric fluid, the capsule floated and did not dissolve in gastric fluid within the first 15 min; on average capsules required about 20 min to dissolve in the HGS (ESI Fig. S2†). As shown in Table 3, 98% of the initial encapsulated GR added to the HGS was emptied after 30 min of gastric digestion. The 30 min gastric digesta was then incubated with small intestinal fluids for 60 or 120 min, leading to the production of 51.5 and 89.4 μmol SF, respectively. The CE was 40.1 and 70.9%, respectively, compared to the total GR added in the capsule, indicating that maximal CE occurred following 30 min gastric digesta and 120 min small intestinal digestion.
The cumulative CE of GR to SF in the BSE and MSP (4
:
1) mixture was significantly (p < 0.05) higher when delivered in capsule form (42.0% and 72.1%) compared to the free powder form (16.6% and 27.6%) for 60 min gastric digestion, and 60 or 120 min small intestinal digestion, respectively (Fig. 4). The increased CE observed for the encapsulated compared to the free powder indicates that it is essential to protect Myr from an extended period of incubation in the low gastric pH. Another possibility would be to include substantially higher levels of Myr activity to the free powder blend, though this would need to be confirmed by further experimentation, and it should be considered that this approach may also increase the chances of GI upset. Encapsulation of the BSE[GR] and MSP[Myr] powder mixture protected the Myr activity in the gastric fluid and thus significantly increased SF yield from GR during small intestinal digestion (Fig. 4). A CE of 39.8% is consistent with the literature from human studies that have delivered a combination of GR and Myr in a tablet or a capsule.48 A CE of 72% after 120 min small intestinal digestion is substantially higher than the 30–40% reported in human studies17,48 where 24 h urine collections were used to evaluate CE. One partial explanation for this difference is that the present study utilized a closed system which captures all of the GR and SF, whereas human studies have been limited to 24 h urine excretion and thus could not account for tissue accumulation, losses in the feces, incomplete urine collections, urinary excretion outside of the 24 h time period and other factors. However, a difference of 30–40% CE between the present model and previous human studies likely cannot be entirely explained by these losses. The high amount of Myr activity relative to previous studies delivered in the capsule blend is also a plausible explanation for at least some of the greater CE reported herein. The fact that previous studies evaluated only one Myr activity level and Myr activity was rarely reported, may further explain the higher CE reported here. Moreover, the high variability and lower CE observed in human studies compared to the current study is due to a combination of factors including inter-individual variability in the capsule transit time and in gastric acidity. Another major factor is likely the type of capsule used for the clinical trial compared to what was used in the current study. In our model, the capsule had not dissolved after 15 min gastric digestion, and while dissolved, remained relatively intact when it moved out of the gastric bag at 30 min gastric digestion, such that 98% of the GR was emptied into the small intestinal digesta at that single time point and the GR and Myr remained proximate in the intestinal digesta enhancing the opportunity for interaction. Zawari et al. reported that not all commercial capsules used to deliver GR and Myr actually met United States Pharmacopeia dissolution and disintegration standards.52 Thus, selection of commercial capsules is another critical variable that should be considered in the optimization of CE such that capsules dissolve in the stomach or early in the small intestine allowing for conversion to occur in the upper GI tract where SF is readily absorbed. In vivo, the human microbiota in small intestine and in colon are capable of converting GR to SF, which though limited, appears to be responsible for conversion in the absence of Myr. For example, human studies have demonstrated that directly consumed fresh broccoli sprouts with intact Myr21 or exogenous Myr co-delivered with GR in a tablet, or a capsule17,48 resulted in an average SF bioavailability of 40% compared to an average of only 10% in the absence of Myr. Moreover, human studies have reported a high inter-individual variability in the absence of Myr, ranging from 1–40% most likely due to the interindividual differences of microbiota composition.17,48 Microbiota conversion cannot explain the increased CE in the present in vitro study as live bacterial strains were not present in the model. Thus, the bioavailability in vivo will likely be enhanced further using an optimized capsule formulation containing exogenous Myr that is protected from acidic conditions of the stomach along with the microbial activity of the human gut.
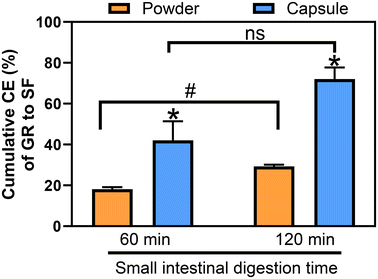 |
| Fig. 4 Comparison of cumulative %CE of GR to SF in form of powder and capsule. BSE[GR] and MSP[Myr] ratio was 4 : 1 (w/w). Powder or capsule was first loaded to the HGS. Gastric digesta at 15, 30, 60, 90, 120 min was collected, and then underwent small intestinal digestion for 60 or 120 min, separately. Cumulative conversion was calculated based on cumulative produced SF (μmol)/total added GR (μmol) × 100, taking into account samples from all gastric digestion times. Results are shown as mean ± SD of three independent experiments. * Indicates that values are significantly different from those for the powder (unpaired t-test, p < 0.05). # Indicates that values are significantly different (paired t-test, p < 0.05). | |
3.4 Effects of AA on the gastric and small intestinal GR to SF conversion in capsules
AA is a Myr co-factor, known to enhance the activity of Myr. However, the amount of AA required to optimize Myr activity in humans has not been previously investigated. Therefore, we tested the effects of adding different concentrations of AA on GR to SF CE in encapsulated BSE[GR]
:
MSP[Myr] (4
:
1 w/w). Capsules containing the same formula as the 4
:
1 capsule blend, 380 mg BSE[GR] (55 mg (126 μmol) GR) and 95 mg MSP[Myr] (42.6 Units of Myr activity), were added with varying amounts of AA: 0, 11, 44, 88, or 154 mg. All capsules underwent 30 min of gastric digestion followed by 60 or 120 min of small intestinal digestion as these time points generated the maximal CE in the capsule experiments above (Table 3). AA significantly increased the CE of GR to SF (Fig. 5A and B) in a dose-dependent manner. After 60 min of small intestinal digestion, 44 mg AA significantly (p < 0.05) increased the CE from 40.1% to 51.0%. Compared to 44 mg AA, addition of 154 mg AA doubled the CE from 40.1% to 78.0% following 60 min of small intestinal digestion (Fig. 5A). After 120 min of small intestinal digestion, the effects of AA on GR to SF conversion were not as important as those observed after 60 min small intestinal digestion, as the capsules without AA had quite a high CE, 70.9%. However, complete conversion (100% CE) was achieved only in the capsules with 88 mg and 154 mg AA after 120 min small intestinal digestion (Fig. 5B). These results suggest that the addition of AA increased the CE of GR to SF dose-dependently, with the most significant difference observed between the control capsules (0 mg AA) and the 154 mg AA concentration, after 60 min of small intestinal digestion. Considering the high CE of GR to SF measured here, 78.0% after 60 min and 100% after 120 min small intestinal digestion, we consider 154 mg AA to be the optimum concentration of the formulations tested in the present study. It is of course possible that 100% CE may be achieved after only 60 min of intestinal digestion with the inclusion of levels of AA above 154 mg, but this would need to be tested further in future studies. Of the AA concentrations tested in the present study, 154 mg, the concentration eliciting the highest CE, was selected for inclusion in the capsule formula to evaluate SF bioavailability in the Caco-2 cell model; 154 mg AA reflects an approximate 3
:
1 ratio of AA to GR.
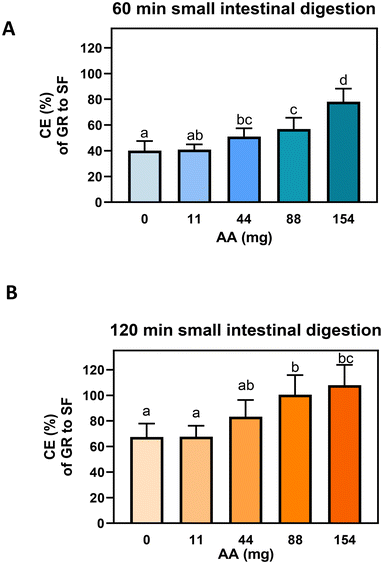 |
| Fig. 5 Conversion of GR to SF in capsules with different amounts of ascorbic acid (AA) after 30 min gastric digestion and further (A) 60 min small intestinal digestion and (B) 120 min small intestinal digestion. The capsule had BSE[GR] 380 mg and MSP[Myr] 95 mg and different amounts of AA. Capsules were first loaded into the HGS, then the gastric digesta at 30 min was collected and was further incubated in intestinal fluid for 60 and 120 min, respectively. Conversion of GR to SF was calculated based on produced SF (μmol)/total added GR (μmol) × 100. Results are shown as mean ± SD of three independent experiments. Different letters are significantly different by one-way ANOVA analysis, p < 0.05. | |
The achievement of 100% CE with the combination of GR, exogenous Myr and ascorbic acid is particularly relevant considering that clinical trials to date have reported dose-dependent relationships between SF and its health benefits. For example, Chen et al.53 observed differential effects of three different doses of a combination of GR and SF derived from broccoli sprouts on the urinary excretion of S-phenylmercapturic acid, a glutathione conjugate of the environmental pollutant benzene. The highest dose studied (600 μmol GR + 40 μmol SF) for 10 days led to a 63.2% in urinary benzene excretion compared to the placebo group. In contrast, the half dose (300 μmol GR + 20 μmol SF) increased urinary benzene excretion by only 11.3% and the one-fifth dose (125 μmol GR + 8 μmol GR) did not exhibit any increase in pollutant excretion. In a similar model studying smokers,54 GR (296 μmol) supplementation for 2 weeks significantly increased urinary excretion of benzene and conjugates of two other environmental pollutants, acrolein and crotonaldehyde whereas a half-dose (148 μmol GR) only increased excretion of benzene. At either dose, the increased excretion of benzene was less than that of the higher doses in the Chen et al. study.53 These data suggest that rather than a dose response, specific thresholds of dose may need to be met in order for GR and/or SF to elicit some health benefits. Notably, exogenous Myr was not included in any of the formulations, therefore, GR conversion was fully reliant on gut microbiota, which is consistently variable and inefficient as noted previously.
To our knowledge, this is the first study to investigate the influence of varying concentrations of AA on the CE of GR to SF in a physiologically relevant in vitro digestion model. The role of AA in enhancing CE has the potential to improve SF bioconversion and bioavailability in the upper GI tract and should be considered in the development of formulations for human clinical trials and dietary supplements.
3.5 SF bioavailability derived from BSE[GR] in Caco-2 cell monolayers
The bioavailability of SF was evaluated in differentiated human Caco-2 cells which are an accepted model for exploring drug/compound uptake, metabolism and transport.41 The kinetics of SF absorption using an SF standard (10 μM) at 1, 2, 4, and 6 h incubation, were initially established to determine the optimal incubation time. After 4 h incubation, the maximum amount of SF and SF metabolites (72% of total SF added) were measured in cells and in the lower chamber.14 In agreement with this result, and at a similar initial SF concentration (11 μM), 74 ± 29% (mean ± SD) SF was absorbed in an in vivo jejunal perfusion system.55 This result is also consistent with human clinical trials supplemented with SF.22 The SF absorption was next evaluated in samples of the small intestinal digesta corresponding to three different encapsulated formulas: B capsule (380 mg BSE[GR] alone), BM capsule (380 mg BSE[GR] + 95 mg MSP[Myr]; 4
:
1 BSE[GR]
:
MSP[Myr] ratio) and BMA capsule (380 mg BSE[GR] + 95 mg MSP[Myr] + 154 mg AA; 4
:
1 BSE[GR]
:
MSP[Myr] ratio + AA). Capsules underwent gastric digestion for 30 min and further small intestinal digestion for 60 min. We selected this timepoint and AA concentration as these reflected the highest CE conditions and the biggest difference of CE between BM and BMA after 60 min small intestinal digestion (Fig. 5). The potential cytotoxicity of the small intestinal digesta from these capsules was assessed by measuring cell viability using the MTT method,56 and by assessing monolayers permeability via measuring TEER.57 Exposure of the Caco-2 cell monolayers to the small intestinal digesta derived from these three capsules did not affect cell viability nor the integrity of monolayers after 4 h incubation (ESI Fig. S3†).
As shown in Table 4, after 4 h incubation of the cell monolayers in the presence of the B capsule (containing only BSE[GR]) digesta added to the upper layer showed a minimal absorption of GR, with 86.8 nmol of GR detected (94.7% of total added GR) observed in the upper chamber, confirming a poor intestinal absorption of GR in the absence of Myr. Only a small amount of SF, 0.4 nmol (0.4% of GR content), was measured in cells and the lower chamber, with no SF metabolites detected. In contrast, the small intestinal digesta of the BM capsule (containing BSE[GR] + MSP[Myr]) showed an efficient conversion of GR to SF during both intestinal digestion and upon incubation with the Caco-2 cell monolayers. The converted SF was absorbed and metabolized through its mercapturic acid pathway to SF-GSH, SF-CYS, and SF-NAC. Digesta added to cells derived from the BM capsule contained 54.1 nmol GR and 36.3 nmol SF. After 4 h incubation with cells, in the upper chamber (mimicking the intestinal lumen), residual 26.1 nmol of GR was observed, while a total 61.6 nmol of SF and SF metabolites was detected. SF metabolites were present in the cell monolayers and in the upper and lower chambers. The bioavailability of SF derived from the BM capsules was approximately 52.7%. The BMA capsule containing BSE[GR] + MSP[Myr] + AA showed the highest SF bioavailability of 74.0% (Table 4). These findings indicate that the combination of Myr and AA significantly increase the SF yield from BSE[GR] in small intestinal digestion compared to GR alone and GR + Myr.
Table 4 GR and SF absorption and metabolism in small intestinal digesta derived from three encapsulated formulas by Caco-2 cell monolayers
Treatment |
Analytes |
Added (nmol) |
Apical (residual, nmol) |
Cell (retention, nmol) |
Basolateral (transport, nmol) |
Bioavailability (%) |
B capsule: BSE[GR] 380 mg; BM capsule: BSE[GR] 380 mg and MSP[Myr] 95 mg; BMA: BSE[GR] 380 mg, MSP[Myr] 95 mg and AA 154 mg. All capsules were digested in the HGS for 30 min and then in a static small intestinal model for a further 60 min. Small intestinal digesta of 250 μL was added and incubated with Caco-2 cells for 4 h. Apical residual digesta, cells and basolateral medium were collected for analysis. Results are shown as mean ± SD of 3 replicates of 3 independent experiments. Statistical analysis was performed between B, BM, and BMA capsules. Means with different superscript letters or symbols in the same column of the same compounds are significantly different at p < 0.05 by one-way ANOVA analysis. ND: Not detected. Glucoraphanin (GR), sulforaphane (SF), SF-glutathione (SF-GSH), SF-cysteine (SF-CYS) and SF-N-acetylcysteine (SF-NAC). |
B |
GR |
91.7 ± 2.4a |
86.8 ± 6.9a |
2.7 ± 1.3a |
1.7 ± 0.6a |
|
SF |
0.4 ± 0.08* |
0.15 ± 0.02* |
0.05 ± 0.03* |
0.05 ± 0.01* |
4.9 ± 2.0A |
SF-CYS |
ND |
ND |
ND |
ND |
|
SF-GSH |
ND |
ND |
ND |
ND |
|
SF-NAC |
ND |
ND |
ND |
ND |
|
|
BM |
GR |
54.1 ± 1.8b |
26.1 ± 1.9b |
1.6 ± 0.1b |
1.7 ± 0.3a |
|
SF |
36.3 ± 1.2# |
12.4 ± 2.8# |
3.4 ± 1.1# |
21.7 ± 3.2# |
56.2 ± 7.3B |
SF-CYS |
ND |
0.9 ± 0.31 |
3.6 ± 0.71 |
12.5 ± 1.41 |
|
SF-GSH |
ND |
0.8 ± 0.1a |
1.2 ± 0.3a |
3.8 ± 1.1a |
|
SF-NAC |
ND |
0.01 ± 0.0021 |
ND |
0.01 ± 0.0081 |
|
|
BMA |
GR |
20.1 ± 1.1c |
3.7 ± 1.4c |
0.3 ± 0.04c |
0.4 ± 0.04b |
|
SF |
71.5 ± 4.0$ |
8.9 ± 0.9$ |
4.5 ± 0.7# |
30.4 ± 3.1$ |
74.8 ± 8.2C |
SF-CYS |
ND |
8.5 ± 1.52 |
4.4 ± 0.62 |
19.0 ± 1.21 |
|
SF-GSH |
ND |
2.8 ± 0.8b |
1.8 ± 0.6b |
6.8 ± 1.2b |
|
SF-NAC |
ND |
0.01 ± 0.0051 |
0.01 ± 0.0041 |
0.03 ± 0.021 |
|
It is known from the literature and confirmed in the present study that, in enterocytes, SF is easily absorbed, transported, and metabolized. A low concentration of GR was detected (∼4%) in both cells and the basolateral medium, indicating minimal absorption of GR. This finding was supported by a previous investigation in F344 rats, where administration of 150 μmol per kg body weight of GR via gavage resulted in a 4.9% intact absorption after 36 h.58 On the other hand, we previously observed that only 29% of SF present in a Kale digesta was absorbed and transported across Caco-2 cell monolayers after 6 h incubation.39 This is relatively low as compared with this study. The lower bioavailability is possibly due to the low amount of SF present in the Kale digesta (0.014 nmol), to a negative impact of the kale food matrix on SF absorption and/or the lack of Myr and AA in the kale (neither of which were assessed). The low concentration of SF-NAC detected under the current experimental conditions may be due to a limited conversion of SF to SF-NAC by Caco-2 cells. Consistently, another study reported that after 60 min incubation of SF (100 μM) on Caco-2 cell monolayers, no detectable peaks of SF-NAC were detected.59 This is consistent with previous evidence showing that SF-NAC is mainly generated in liver and kidneys, but not in the small intestine,60,61 consistent with higher levels of SF-NAC in the urine in human clinical trials.12,53
4. Conclusion
BSE[GR] + MSP[Myr] delivered as a free (non-encapsulated) powder resulted in impairments in Myr activity and subsequent reductions in SF bioconversion and bioavailability. One solution to this issue would be to deliver an abundance of Myr to compensate for losses of Myr activity due to acid exposure within the stomach, whereas an alternative would be to deliver the powder within a capsule. In the present study, we identified that delivering the BSE[GR] + MSP[Myr] within a commercial HPMC capsule protected Myr and significantly increased GR bioconversion to SF, ∼70% conversion after 30 min gastric digestion and 120 min intestinal digestion. Moreover, co-delivering AA, a cofactor and known enhancer of Myr activity as an encapsulated combination of BSE[GR] + MSP[Myr], optimized GR to SF bioconversion and bioavailability. Inclusion of 154 mg of AA (1
:
3 ratio of AA to GR) to the capsule blend which included 4
:
1 BSE[GR]
:
MSP[Myr] ratio, led to 100% bioconversion of GR to SF after 30 min gastric digestion and 120 min small intestinal digestion. This combination subsequently enhanced SF bioavailability in Caco-2 monolayers. Encapsulated BSE[GR] combined with MSP[Myr], and 154 mg of AA led to GR bioavailability as SF of 74.8%, after 30 min gastric digestion, 60 min intestinal digestion and 4 h incubation in Caco-2 monolayers. Protection of Myr activity against low pH during gastric transit and addition of its cofactor, AA, emerged as major strategies to improve SF production and absorption in the upper GI tract. This study identifies development strategies to optimize GR conversion to SF to increase SF bioavailability via formulated supplements in the upper GI tract.
Abbreviations
GR | Glucoraphanin |
Myr | Myrosinase |
SF | Sulforaphane |
BSE[GR] | GR-rich broccoli extract powder |
MSP[Myr] | Myr-rich mustard seed powder |
AA | Ascorbic acid |
GSLs | Glucosinolates |
ITCs | Isothiocyanates |
GI | Gastrointestinal |
SF-GSH | SF-glutathione |
SF-CYS | SF-cysteine |
SF-NAC | SF-N-acetylcysteine |
HPMC | Hydroxypropyl methylcellulose |
FBS | Fetal bovine serum |
HBSS | Hanks’ balanced salt solution |
HGS | Human gastric simulator |
MRM | Multiple reaction monitoring |
CE | Conversion efficiency |
TEER | Transepithelial electrical resistance |
MEM | Minimum essential medium |
UHPLC-MS | Ultra high-pressure liquid chromatography coupled with a mass spectrometer |
DMSO | Dimethyl sulfoxide |
ACN | Acetonitrile. |
Data availability
The data supporting this article are available upon request.
Conflicts of interest
At the time this study was conducted, Angela F. Mastaloudis was an employee of Brassica Protection Products LLC, who provided funding for the study. All other authors have no conflict of interest to declare.
Acknowledgements
This work was supported by funding from Brassica Protection Products LLC to A. E. M., G. M. B, E. C. and P. O., and NIFA-USDA (CA-D*-NTR-7244-H) to P. O. and NIFA-USDA (CAD-FST-6975-H) to A. E. M. Thanks to Christina Shelton at Lonza Capsules & Health Ingredients for donating the capsules for this project. Special thanks to Jed W. Fahey, ScD, for his support in various technical aspects of the study, particularly the myrosinase activity testing.
References
- I. Herr and M. W. Büchler, Dietary constituents of broccoli and other cruciferous vegetables: Implications for prevention and therapy of cancer, Cancer Treat. Rev., 2010, 36, 377–383 CrossRef CAS.
- Y. Z. Li, Z. Y. Yang, T. T. Gong, Y. S. Liu, F. H. Liu, Z. Y. Wen, X. Y. Li, C. Gao, M. Luan, Y. H. Zhao and Q. J. Wu, Cruciferous vegetable consumption and multiple health outcomes: an umbrella review of 41 systematic reviews and meta-analyses of 303 observational studies, Food Funct., 2022, 13, 4247–4259 RSC.
- L. Ma, G. Liu, L. Sampson, W. C. Willett, F. B. Hu and Q. Sun, Dietary glucosinolates and risk of type 2 diabetes in 3 prospective cohort studies, Am. J. Clin. Nutr., 2018, 107, 617–625 CrossRef PubMed.
- X. Zhang, X. O. Shu, Y. B. Xiang, G. Yang, H. Li, J. Gao, H. Cai, Y. T. Gao and W. Zheng, Cruciferous vegetable consumption is associated with a reduced risk of total and cardiovascular disease mortality, Am. J. Clin. Nutr., 2011, 94, 240–246 CrossRef CAS PubMed.
- E. L. Connolly, M. Sim, N. Travica, W. Marx, G. Beasy, G. S. Lynch, C. P. Bondonno, J. R. Lewis, J. M. Hodgson and L. C. Blekkenhorst, Glucosinolates From Cruciferous Vegetables and Their Potential Role in Chronic Disease: Investigating the Preclinical and Clinical Evidence, Front. Pharmacol., 2021, 12, 767975 CrossRef CAS PubMed.
- P. Soundararajan and J. S. Kim, Anti-Carcinogenic Glucosinolates in Cruciferous Vegetables and Their Antagonistic Effects on Prevention of Cancers, Molecules, 2018, 23, 2983 CrossRef PubMed.
- I. Winde and U. Wittstock, Insect herbivore counteradaptations to the plant glucosinolate–myrosinase system, Phytochemistry, 2011, 72, 1566–1575 CrossRef CAS PubMed.
- S. Chhajed, I. Mostafa, Y. He, M. Abou-Hashem, M. El-Domiaty and S. Chen, Glucosinolate Biosynthesis and the Glucosinolate–Myrosinase System in Plant Defense, Agronomy, 2020, 10, 1786 CrossRef CAS.
- K. Sikorska-Zimny and L. Beneduce, The metabolism of glucosinolates by gut microbiota, Nutrients, 2021, 13, 2750 CrossRef CAS.
- W. P. Burmeister, S. Cottaz, P. Rollin, A. Vasella and B. Henrissat, High resolution X-ray crystallography shows that ascorbate is a cofactor for myrosinase and substitutes for the function of the catalytic base, J. Biol. Chem., 2000, 275, 39385–39393 CrossRef CAS PubMed.
- J. W. Fahey, Y. Zhang and P. Talalay, Broccoli sprouts: An exceptionally rich source of inducers of enzymes that protect against chemical carcinogens, Proc. Natl. Acad. Sci. U. S. A., 1997, 94, 10367–10372 CrossRef CAS PubMed.
- P. A. Egner, T. W. Kensler, J. G. Chen, S. J. Gange, J. D. Groopman and M. D. Friesen, Quantification of Sulforaphane Mercapturic Acid Pathway Conjugates in Human Urine by High-Performance Liquid Chromatography and Isotope-Dilution Tandem Mass Spectrometry, Chem. Res. Toxicol., 2008, 21, 1991–1996 Search PubMed.
- P. A. Egner, J. G. Chen, J. B. Wang, Y. Wu, Y. Sun, J. H. Lu, J. Zhu, Y. H. Zhang, Y. S. Chen, M. D. Friesen, L. P. Jacobson, A. Munoz, D. Ng, G. S. Qian, Y. R. Zhu, T. Y. Chen, N. P. Botting, Q. Zhang, J. W. Fahey, P. Talalay, J. D. Groopman and T. W. Kensler, Bioavailability of Sulforaphane from two broccoli sprout beverages: results of a short-term, cross-over clinical trial in Qidong, China, Cancer Prev. Res., 2011, 4, 384–395 Search PubMed.
- W. Zhu, L. A. Lerno, E. Cremonini, P. I. Oteiza, A. Mastaloudis, G. M. Bornhorst and A. E. Mitchell, Robust UHPLC-(ESI+)-MS/MS Method for Simultaneous Analysis of Glucoraphanin, Sulforaphane, and Sulforaphane Metabolites in Biological
Samples, ACS Food Sci. Technol., 2023, 3, 1300–1310 Search PubMed.
- H. F. Gu, X. Y. Mao and M. Du, Metabolism, absorption, and anti-cancer effects of sulforaphane: an update, Crit. Rev. Food Sci. Nutr., 2022, 62, 3437–3452 CrossRef CAS PubMed.
- R. T. Ruhee and K. Suzuki, The Integrative Role of Sulforaphane in Preventing Inflammation, Oxidative Stress and Fatigue: A Review of a Potential Protective Phytochemical, Antioxidants, 2020, 9, 521 CrossRef CAS.
- J. W. Fahey, W. D. Holtzclaw, S. L. Wehage, K. L. Wade, K. K. Stephenson and P. Talalay, Sulforaphane Bioavailability from Glucoraphanin-Rich Broccoli: Control by Active Endogenous Myrosinase, PLoS One, 2015, 10, e0140963 CrossRef PubMed.
- G. C. Wang, M. Farnham and E. H. Jeffery, Impact of Thermal Processing on Sulforaphane Yield from Broccoli (Brassica oleracea L. ssp. italica), J. Agric. Food Chem., 2012, 60, 6743–6748 CrossRef CAS.
- T. W. Kensler, J. G. Chen, P. A. Egner, J. W. Fahey, L. P. Jacobson, K. K. Stephenson, L. Ye, J. L. Coady, J. B. Wang, Y. Wu, Y. Sun, Q. N. Zhang, B. C. Zhang, Y. R. Zhu, G. S. Qian, S. G. Carmella, S. S. Hecht, L. Benning, S. J. Gange, J. D. Groopman and P. Talalay, Effects of glucosinolate-rich broccoli sprouts on urinary levels of aflatoxin-DNA adducts and phenanthrene tetraols in a randomized clinical trial in He Zuo township, Qidong, People's Republic of China, Cancer Epidemiol. Biomarkers Prev., 2005, 14, 2605–2613 CrossRef CAS PubMed.
- T. A. Shapiro, J. W. Fahey, K. L. Wade, K. K. Stephenson and P. Talalay, Chemoprotective glucosinolates and isothiocyanates of broccoli sprouts: metabolism and excretion in humans, Cancer Epidemiol. Biomarkers Prev., 2001, 10, 501–508 CAS.
- R. Dominguez-Perles, S. Medina, D. Á. Moreno, C. García-Viguera, F. Ferreres and Á. Gil-Izquierdo, A new ultra-rapid UHPLC/MS/MS method for assessing glucoraphanin and sulforaphane bioavailability in human urine, Food Chem., 2014, 143, 132–138 CrossRef CAS PubMed.
- J. W. Fahey, K. L. Wade, S. L. Wehage, W. D. Holtzclaw, H. Liu, P. Talalay, E. Fuchs and K. K. Stephenson, Stabilized sulforaphane for clinical use: Phytochemical delivery efficiency, Mol. Nutr. Food Res., 2017, 61, 1600766 CrossRef PubMed.
- M. W. Yi Jin, R. T. Rosen and C.-T. Ho, Thermal Degradation of Sulforaphane in Aqueous Solution, J. Agric. Food Chem., 1999, 47, 3121–3123 CrossRef PubMed.
- S. J. Franklin, S. E. Dickinson, K. L. Karlage, G. T. Bowden and P. B. Myrdal, Stability of sulforaphane for topical formulation, Drug Dev. Ind. Pharm., 2014, 40, 494–502 CrossRef CAS PubMed.
- O. Okunade, K. Niranjan, S. K. Ghawi, G. Kuhnle and L. Methven, Supplementation of the Diet by Exogenous Myrosinase via Mustard Seeds to Increase the Bioavailability of Sulforaphane in Healthy Human Subjects after the Consumption of Cooked Broccoli, Mol. Nutr. Food Res., 2018, 62, e1700980 CrossRef.
- M. Ohtsuru and T. Hata, The interaction of L-ascorbic acid with the active center of myrosinase, Biochim. Biophys. Acta, 1979, 567, 384–391 CrossRef CAS.
- D. Van Eylen, I. Oey, M. Hendrickx and A. Van Loey, Behavior of mustard seed (Sinapis alba L.) myrosinase during temperature/pressure treatments: A case study on enzyme activity and stability, Eur. Food Res. Technol., 2008, 226, 545–553 CrossRef CAS.
- M. G. Ettlinger, G. P. Dateo Jr, B. W. Harrison, T. J. Mabry and C. P. Thompson, Vitamin C as a coenzyme: the hydrolysis of mustard oil glucosides, Proc. Natl. Acad. Sci. U. S. A., 1961, 47, 1875–1880 CrossRef CAS PubMed.
- S. Keppler, S. O'Meara, S. Bakalis, P. J. Fryer and G. M. Bornhorst, Characterization of individual particle movement during in vitro gastric digestion in the Human Gastric Simulator (HGS), J. Food Eng., 2020, 264, 109674 CrossRef CAS.
- Y. A. Mennah-Govela and G. M. Bornhorst, Breakdown mechanisms of whey protein gels during dynamic in vitro gastric digestion, Food Funct., 2021, 12, 2112–2125 RSC.
- A. R. Hilgers, R. A. Conradi and P. S. J. P. r. Burton, Caco-2 cell monolayers as a model for drug transport across the intestinal mucosa, Pharm. Res., 1990, 7, 902–910 CrossRef CAS.
- M. Shikita, J. W. Fahey, T. R. Golden, W. David Holtzclaw and P. Talalay, An unusual case of ‘uncompetitive activation’ by ascorbic acid: purification and kinetic properties of a myrosinase from Raphanus sativus seedlings, Biochem. J., 1999, 341, 725–732 CrossRef CAS.
- K. L. Wade, Y. Ito, A. Ramarathnam, W. D. Holtzclaw and J. W. Fahey, Purification of Active Myrosinase from Plants by Aqueous Two-Phase Counter-Current Chromatography, Phytochem. Anal., 2015, 26, 47–53 CrossRef CAS PubMed.
- M. Minekus, M. Alminger, P. Alvito, S. Ballance, T. Bohn, C. Bourlieu, F. Carrière, R. Boutrou, M. Corredig, D. Dupont, C. Dufour, L. Egger, M. Golding, S. Karakaya, B. Kirkhus, S. Le Feunteun, U. Lesmes, A. Macierzanka, A. Mackie, S. Marze, D. J. McClements, O. Ménard, I. Recio, C. N. Santos, R. P. Singh, G. E. Vegarud, M. S. J. Wickham, W. Weitschies and A. Brodkorb, A standardised static in vitro digestion method suitable for food – an international consensus, Food Funct., 2014, 5, 1113–1124 RSC.
- M. J. Roman, B. J. Burri and R. P. Singh, Release and Bioaccessibility of β-Carotene from Fortified Almond Butter during in Vitro Digestion, J. Agric. Food Chem., 2012, 60, 9659–9666 CrossRef CAS.
- A. M. R. Hayes, C. Swackhamer, Y. A. Mennah-Govela, M. M. Martinez, A. Diatta, G. M. Bornhorst and B. R. Hamaker, Pearl millet (Pennisetum glaucum) couscous breaks down faster than wheat couscous in the Human Gastric Simulator, though has slower starch hydrolysis, Food Funct., 2020, 11, 111–122 RSC.
- H. Vrbanac, J. Trontelj, S. Berglez, B. Petek, J. Opara, R. Jereb, D. Krajcar and I. Legen, The biorelevant simulation of gastric emptying and its impact on model drug dissolution and absorption kinetics, Eur. J. Pharm. Biopharm., 2020, 149, 113–120 CrossRef CAS PubMed.
- Q. Guo, A. Ye, M. Lad, D. Dalgleish and H. Singh, Effect of gel structure on the gastric digestion of whey protein emulsion gels, Soft Matter, 2014, 10, 1214–1223 RSC.
- E. S. Hwang, G. M. Bornhorst, P. I. Oteiza and A. E. Mitchell, Assessing the Fate and Bioavailability of Glucosinolates in Kale (Brassica oleracea) Using Simulated Human Digestion and Caco-2 Cell Uptake Models, J. Agric. Food Chem., 2019, 67, 9492–9500 CrossRef CAS.
- B. Gong, L. Cheng, R. G. Gilbert and C. Li, Distribution of short to medium amylose chains are major controllers of in vitro digestion of retrograded rice starch, Food Hydrocolloids, 2019, 96, 634–643 CrossRef CAS.
- V. Meunier, M. Bourrié, Y. Berger and G. Fabre, The human intestinal epithelial cell line Caco-2; pharmacological and pharmacokinetic applications, Cell Biol. Toxicol., 1995, 11, 187–194 CrossRef CAS PubMed.
- D. Van Eylen, Indrawati, M. Hendrickx and A. Van Loey, Temperature and pressure stability of mustard seed (Sinapis alba L.) myrosinase, Food Chem., 2006, 97, 263–271 CrossRef CAS.
- G.-C. Yen and Q.-K. Wei, Myrosinase activity and total glucosinolate content of cruciferous vegetables and some Properties of Cabbage Myrosinase in Taiwan, J. Sci. Food Agric., 1993, 61, 471–475 CrossRef CAS.
- D. Stoin, P. Pirsan, F. Radu, M.-A. Poiana, E. Alexa and D. Dogaru, Studies regarding the myrosinase enzymatic activity from black mustard seeds, J. Food, Agric. Environ., 2009, 7(1), 44–47 CAS.
- S. Pihakaski and K. Pihakaski, Myrosinase in Brassicaceae (Cruciferae), J. Exp. Bot., 1978, 29, 1363–1369 CrossRef CAS.
- R. Björkman and B. Lönnerdal, Studies on myrosinases III. Enzymatic properties of myrosinases from Sinapis alba and Brassica napus seeds, Biochim. Biophys. Acta, Enzymol., 1973, 327, 121–131 CrossRef.
- Z. Pan, Z. Meng, M. Tan, H. Duan, H. S. Ramaswamy, X. Qiu and C. Wang, Optimization the conversion of glucosinolate to isothiocyanate in yellow mustard seeds (Sinapis alba) by response surface methodology, Appl. Food Res., 2022, 2, 100207 CrossRef CAS.
- J. W. Fahey, K. L. Wade, K. K. Stephenson, A. A. Panjwani, H. Liu, G. Cornblatt, B. S. Cornblatt, S. L. Ownby, E. Fuchs, W. D. Holtzclaw and L. J. Cheskin, Bioavailability of Sulforaphane Following Ingestion of Glucoraphanin-Rich Broccoli Sprout and Seed Extracts with Active Myrosinase: A Pilot Study of the Effects of Proton Pump Inhibitor Administration, Nutrients, 2019, 11, 1489 CrossRef CAS PubMed.
- S. Medina, R. Dominguez-Perles, D. A. Moreno, C. Garcia-Viguera, F. Ferreres, J. I. Gil and A. Gil-Izquierdo, The intake of broccoli sprouts modulates the inflammatory and vascular prostanoids but not the oxidative stress-related isoprostanes in healthy humans, Food Chem., 2015, 173, 1187–1194 CrossRef CAS.
- Y. Ushida, H. Suganuma and A. Yanaka, Low-dose of the sulforaphane precursor glucoraphanin as a dietary supplement induces chemoprotective enzymes in humans, Food Sci. Nutr., 2015, 6, 1603–1612 CAS.
- M. T. Lopez-Chillon, C. Carazo-Diaz, D. Prieto-Merino, P. Zafrilla, D. A. Moreno and D. Villano, Effects of long-term consumption of broccoli sprouts on inflammatory markers in overweight subjects, Clin. Nutr., 2019, 38, 745–752 CrossRef CAS.
- M. Zawari, B. Poller, G. Walker, A. Pearson, M. Hampton and A. C. Carr, Formulation of Broccoli Sprout Powder in Gastro-Resistant Capsules Protects against the Acidic pH of the Stomach In Vitro but Does Not Increase Isothiocyanate Bioavailability In Vivo, Antioxidants, 2019, 8, 359 CrossRef CAS.
- J. G. Chen, J. Johnson, P. Egner, D. Ng, J. Zhu, J. B. Wang, X. F. Xue, Y. Sun, Y. H. Zhang, L. L. Lu and Y. S. Chen, Dose-dependent detoxication of the airborne pollutant benzene in a randomized trial of broccoli sprout beverage in Qidong, China, Am. J. Clin., 2019, 110(3), 675–684 CrossRef PubMed.
- J. E. Bauman, C. H. Hsu, S. Centuori, J. Guillen-Rodriguez, L. L. Garland, E. Ho, M. Padi, V. Bageerathan, L. Bengtson, M. Wojtowicz and E. Szabo, Randomized crossover trial evaluating detoxification of tobacco carcinogens by broccoli seed and sprout extract in current smokers, Cancers, 2022, 14(9), 2129 CrossRef CAS.
- N. Petri, C. Tannergren, B. Holst, F. A. Mellon, Y. Bao, G. W. Plumb, J. Bacon, K. A. Leary, P. A. Kroon, L. Knutson, P. Forsell, T. Eriksson, H. Lennernas and G. Williamson, Absorption/metabolism of sulforaphane and quercetin, and regulation of phase II enzymes, in human jejunum in vivo, Drug Metab. Dispos., 2003, 31, 805 CrossRef CAS PubMed.
- A. Bahuguna, I. Khan, V. K. Bajpai and S. C. Kang, MTT assay to evaluate the cytotoxic potential of a drug, Bangladesh J. Pharmacol., 2017, 12, 115–118 Search PubMed.
- O. Y. Henry, R. Villenave, M. J. Cronce, W. D. Leineweber, M. A. Benz and D. E. Ingber, Organs-on-chips with integrated electrodes for trans-epithelial electrical resistance
(TEER) measurements of human epithelial barrier function, Lab Chip, 2017, 17, 2264–2271 RSC.
- R. M. Bheemreddy and E. H. Jeffery, The Metabolic Fate of Purified Glucoraphanin in F344 Rats, J. Agric. Food Chem., 2007, 55, 2861–2866 CrossRef CAS PubMed.
- Y. Ushida, C. Boonyapichest, H. Suganuma, M. Tanaka and T. Matsui, Paracellular Transport of Sulforaphane across Caco-2 Cell Monolayers, Food Sci. Technol. Res., 2016, 22, 127–134 CrossRef CAS.
- N. Baenas, J. M. Silván, S. Medina, S. de Pascual-Teresa, C. García-Viguera and D. A. Moreno, Metabolism and antiproliferative effects of sulforaphane and broccoli sprouts in human intestinal (Caco-2) and hepatic (HepG2) cells, Phytochem. Rev., 2015, 14, 1035–1044 CrossRef CAS.
- D. Angelino and E. Jeffery, Glucosinolate hydrolysis and bioavailability of resulting isothiocyanates: Focus on glucoraphanin, J. Funct. Foods, 2014, 7, 67–76 CrossRef CAS.
|
This journal is © The Royal Society of Chemistry 2025 |
Click here to see how this site uses Cookies. View our privacy policy here.