Recent advances in encapsulation of pomegranate peel extract and combination of wall materials: a review of encapsulation technologies, characterization and applications in the food industry
Received
25th June 2024
, Accepted 25th October 2024
First published on 18th November 2024
Abstract
Pomegranate peel comprises a vital source of bioactive constituents such as antioxidants, fiber, vitamins, and minerals. This review delivers a comprehensive outline of using pomegranate peel waste as a source of bioactive compounds for encapsulation in the food industry. The encapsulation methods from pomegranate peel studies, including spray drying, coacervation, ionic gelation, and freeze drying, and their characterization are explored from previous studies. The importance of encapsulation in improving the controlled release of anthocyanin and phenolic content, thereby enhancing their functionality in food products is mentioned. Furthermore, the review highlights the potential of encapsulation to reduce waste in the food industry. The applications of a combination of wall materials and encapsulation techniques in preserving pigments, flavors and aromas, enhancing nutritional value, and extending shelf life are also stated. Through a thorough analysis of encapsulation methodologies and applications, this review offers valuable insights into the potential of pomegranate peel encapsulation as a sustainable solution for enhancing food products.
 Rahul P B | Rahul P B is an aspiring food technologist with a Master's degree in Food Science and Technology from Lovely Professional University, Punjab. His research interests focus on food safety, encapsulation techniques, and the application of bioactive components in food systems. Rahul has conducted comprehensive reviews and research projects on encapsulation technologies, particularly utilizing pomegranate peel extract. His academic and practical work aims to advance innovation in food science and contribute to sustainable approaches in food technology. |
 Ravindra Kumar Tiwari | Dr Ravindra Kumar Tiwari is an Assistant Professor at the Department of Food Technology and Nutrition, School of Agriculture, Lovely Professional University, Phagwara, Punjab-144411, India. His research expertise is in Agriculture Engineering. He has completed his PhD from Punjab Agriculture University, Punjab, India. He motivates students towards agro-food processing. |
 Kshirod K. Dash | Dr Kshirod Kumar Dash is a Professor at the Department of Food Processing Technology, Ghani Khan Choudhury Institute of Engineering and Technology, Malda, West Bengal, India. He holds a BTech in Agricultural Engineering from CAET Bhubaneswar and MTech and PhD in Food Process Engineering from IIT Kharagpur and completed his postdoc at Ohio State University. He has published extensively and led three funded projects. He is involved in the ePG Pathshala program as a paper coordinator and content writer. His research focuses on novel food processing technologies and mass transfer in food processing. |
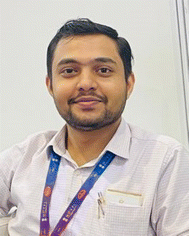 Maanas Sharma | Dr Maanas Sharma is an Assistant Professor and Teacher-in-Charge at the Department of Food Technology and Home Science at Vivekananda College, University of Delhi. He earned a PhD in Food Engineering and Technology from Tezpur University, an MSc in Food Science and Technology from Pondicherry University, and a BSc (Hons.) in Food Technology from the University of Delhi. His research focuses on food science and new technologies, and he has published numerous papers and contributed to book chapters. Dr Sharma is also a life member of the Association of Food Scientists and Technologists (India), where he is honored for his leadership and academic accomplishments. |
Sustainability spotlight
Pomegranate peel waste is transformed into useful functional components rich in antioxidants, fiber, vitamins, and minerals by encapsulation technology. Bioactive chemicals are protected by this mechanism, which also improves their stability, bioavailability, and regulated release. Spray drying and freeze drying are important techniques that enhance the quality and preservation of food. These methods provide a range of uses in food and stable bioactive substances. Encapsulation promotes sustainability in the food business by offering chances for waste reduction, creative product development, and enhanced profitability. By using this method, waste is decreased and functional foods with improved health benefits are produced.
|
1. Introduction
Pomegranates, scientifically referred to as Punica granatum L., belong to the Punicaceae species.1 Pomegranate has been utilised in diverse medicinal traditions for treating and addressing a broad spectrum of illnesses and health issues.2 Since pomegranates are usually eaten raw or juiced, a large amount of peel waste is produced. In addition to providing health advantages including antioxidants and anti-inflammatory qualities, pomegranate peels also include vital bioactive substances such as fiber, vitamins, and minerals that help mitigate and treat chronic metabolic problems.3 Utilizing these bioactive components from pomegranate peels as functional ingredients can enhance the value of the pomegranate industry by effectively utilising by-product resources.4
Pomegranate peel and its extract have important technical functions as well, such as antioxidant, antibacterial, natural colorant, and flavor enhancer, in addition to their nutritional value. They may also be beneficial natural food additives for preserving and improving the quality of food.5 Various techniques, including agglomeration, coacervation, and encapsulation, are employed to enhance stability, provide protection, and achieve controlled release. Among these methods, encapsulation is utilized in this context.
The process by which one material, or a combination of elements, becomes trapped within another material is known as encapsulation. Đorđević et al. (2014)6 stated that the process of encapsulation involves trapping one material inside another, producing particles that are between a few nanometers and a few millimeters in size. The product or material inside is called the payload, internal phase, fill, active agent, or core material; the material surrounding the core is called the wall material, shell, carrier material, external material, or matrix.7,8 The primary materials utilized for food industry encapsulation consist predominantly of biomolecules. Carbohydrates, lipids, proteins, and natural polymer gums serve as wall materials employed for encapsulation purposes. Starch and its derivatives are the materials most frequently used for encapsulation in culinary applications, which include amylose, amylopectin, dextrins, maltodextrins, polydextrose, syrups, cellulose, and their derivatives. Marine extracts such as alginate and carrageenan, as well as exudates from plants and extracts such as gum arabic, gum tragacanth, and gum karaya, are used in a variety of situations.9
Encapsulation improves both the thermal and chemical stability of pigments while also increasing their bioavailability by allowing the regulated release of bioactive substances such as phenolics and anthocyanins at particular times and locations. Encapsulation provides an increased shelf life for bioactive compounds or the core material. It protects the core material against environmental effects such as light, temperature, and water. It also aids in altering the physicochemical properties of the encapsulated product.10,11 The particle size, which may be altered by different manufacturing methods, is a significant aspect of encapsulation technology.12
Encapsulated products can be utilized as functional food additives because they offer so many health benefits. Encapsulated bioactive compounds are added to food for enhanced oxidation and microbial proliferation, as well as fortification, coloring, and enrichment purposes. The incorporation of encapsulated bioactive substances into foods leads to the proliferation of well-known functional foods. Waste from the food industry such as peels, leaves, seeds etc. from fruits, vegetables or any other raw material can be significantly reduced with the help of encapsulation.13 Spray drying, freeze drying, ionic gelation, coextrusion, electrospinning, electrospraying, and coacervation represent a range of encapsulation techniques employed in various applications. Spray-drying, coacervation, and freeze-drying are more widely used encapsulation techniques to produce enclosed bioactive chemicals from fruit and vegetable by-products.3 Flavors and aromas consist of organic compounds with a small molecular size. Notably volatile, these chemicals are very susceptible to air, heat, light, and moisture. In the flavor and fragrance sector, encapsulation is a commonly used approach to protect flavor and aroma properties. When it comes to processed foods, flavor and aroma are lost throughout the production stage and during the product's subsequent storage. It is possible to utilize pre-encapsulated volatile components in a variety of foods and drinks to lessen the severity of this deterioration and maintain the taste and scent.10
Encapsulated aroma and flavor substances are frequently utilized in the baking industry because of their exceptional stability, ease of measuring, and ease of storage. They are usually found in powdered form. Ingredients such as starch, soy flour, or wheat flour are utilized in the baking business as carriers for encapsulated ingredients. As an alternative, liquid forms such as emulsions are also used in many baked goods. The food sector has limitations in using some antioxidants because of their strong pigmentation and unique taste.14
Encapsulation has shown to be a successful method for increasing the availability and bioactive compound stability, in addition to providing control over their release.15 Chezanoglou and Goula (2023)16 stated that co-crystallization of phenolic extracts offers distinct advantages when compared to traditional encapsulation methods. Phenolic compounds are given a solid-state structure by co-crystals, which strengthens their chemical stability and provides protection against deterioration and oxidation. Improved solubility, dissolving rates, and increased bioavailability are made possible by controlled co-crystallization. The addition of other excipients, such as naturally occurring polymers and surfactants, can also enhance the stability and functional properties of phenolic encapsulated materials, which are made possible by this co-crystallization process. This comprehensive review focused its attention on encapsulation techniques, thoroughly analyzing their methodologies and applications. This review also examined how these techniques affect the physicochemical properties of encapsulated compounds.
2. Bioactive compounds
Fruits, vegetables, and plants all contain a variety of bioactive substances, offering a range of health benefits, primarily through their antioxidant properties. Nevertheless, these compounds are vulnerable to environmental factors due to their low stability. As a result, the concept of encapsulation has arisen as a means to enhance their resilience and create a more precise delivery system. The primary bioactive substances commonly discovered in pomegranate peel extract include punicalagin, gallic acid, ellagic acid, anthocyanins, and flavonoids as mentioned in Table 3. Anthocyanins enhance the vibrant tones present in berry-type fruits. Robert and Fredes (2015)17 stated that serving as natural colorants, anthocyanins are associated with diverse biological and pharmacological advantages, such as anti-inflammatory, antioxidant, and chemoprotective properties.18 Punicalagin is among the major compounds found in peels of pomegranate, characterized by its high molecular weight, and is regarded as a distinctive feature of this fruit component. Punicalagin offers various potential therapeutic benefits, including anti-proliferative, anti-inflammatory, hepatoprotective, and antigenotoxic properties.19 Ellagic acid (EA) demonstrates various properties including antioxidative, antihepatotoxic, antisteatosic, anticholestatic, antifibrogenic, antihepatocarcinogenic, and antiviral effects. The characteristics aforementioned contribute to improving the structure and function of the liver, offering resistance to various harmful conditions. The molecular mechanisms responsible for the impacts of EA encompass the elimination of reactive oxygen species, management of both stages I and II biotransformation enzymes, adjustment of the synthesis of inflammatory and fibrotic cytokines, and control of metabolic pathways associated with lipid metabolism, along with preservation of optimal levels of essential trace elements.20,21 Gao et al. (2019)22 discussed the medicinal benefits of gallic acid, including its potential as an antibacterial, anti-allergy, anti-inflammatory, and antioxidant agent. Gallic acid has been observed to possess both prooxidant and antioxidant properties. Its prooxidant effects are particularly noted for its potent anticancer and apoptosis-inducing abilities in their studies. Flavonoids are increasingly recognized as essential ingredients in many pharmacological, medical, cosmetic, and nutraceutical products. The reason for this recognition is their ability to control essential cellular enzyme activities in addition to their antioxidative, anti-inflammatory, anti-mutagenic, and anti-carcinogenic properties, as depicted in Table 3. The significance of flavonoids was further emphasized with the revelation of their role in reducing cardiovascular mortality rates and preventing coronary heart disease.23,24
A range of techniques have been created over time to create food delivery systems. Initially, the most common conventional techniques used to create microcapsules were complicated coacervates, which included proteins and polysaccharides. An illustration of the difficulties encountered in orally delivering bioactive compounds includes the unpalatable taste of substances such as saponins and tannins, which consumers find unsuitable for direct consumption. Additionally, hydrophobic chemicals, such as carotenoids, have restricted solubility, which hinders the body's ability to dissolve and absorb them.25
For the enhancement of accessibility, timing of the release, and accuracy of delivery for bioactive substances micro- and nanoencapsulation can be utilized effectively.26,27 The surface area of micro- and nanosystems is closely correlated with the release rate of bioactives, whereas particle size has an inverse effect on this relationship. Thus, lowering particle size to increase surface area causes an increase in the rate at which bioactives are released. Encapsulating substances for delivery systems can be selected from natural biomaterials that satisfy the Generally Recognized As Safe (GRAS) standards.28 Proteins, lipids, and polysaccharides are food-grade materials that can be utilized to produce biologically derived micro- and nanosystems for the food industry. These materials are used to encapsulate different compounds, as shown in Table 2. These can be used individually or in combination to create intricate delivery systems. A deep awareness of the characteristics of the bioactive and the makeup of the matrix of foods through which these substances will be included are prerequisites for choosing the right bio-based material. Delivery systems based on polysaccharides are generally classified based on their natural sources, which can be microorganisms (dextran and xanthan gum), animals (chitosan), algae (alginate and carrageenan), and plants (pectin, guar gum, starch, and cellulose).29 The most commonly used natural polysaccharides in the creation of micro- and nano-delivery systems encompass gums such as xanthan gum, chitosan, alginate, and carrageenan. Chitosan is a hydrophilic linear polysaccharide that is well-known for improving cell membrane permeability among them all. This feature increases the delivery system's duration of residence in the gastrointestinal tract, which ultimately improves the accessibility of bioactive substances.26
Refining the essential production parameters in a protein-based delivery system relies heavily on a comprehensive understanding of protein molecules' attributes, which encompass chemical degradation processes, isoelectric points, and denaturation temperature. This underscores the significant importance of thoroughly examining protein molecules within this system.30 Compared to alternative delivery systems, the lipid-based delivery system has several benefits, including the capacity to hold onto substances with a wide range of solubilities, especially those with notable hydrophobic qualities. This capability ensures the stability of bioactive compounds during storage, effectively shielding them from both biological and chemical degradation. By increasing the hydrophobic bioactive chemicals' encapsulation efficacy, these systems may increase their availability within the body and lower the risk of toxicity. The microstructure, colloidal stability, rheological properties, and moisture resistance of lipids are influenced by their physicochemical attributes. Lowering the hydrocarbon chain's length linked to the backbone of glycerol or increasing the level of unsaturation in the fatty acid chains results in a lower melting point and less resistance to moisture (de Souza Simões et al., 2017).26 Pomegranate peels are also packed with tannins, gallo tannins, ellagic acid, and other compounds that offer health benefits but can also have negative effects when consumed in large quantities.31 These compounds are valuable in industries such as textile dyeing and waste management.32 They also have antibacterial properties that work by interfering with bacterial enzymes, proteins, and metal ions.4
3. Chemical stability of anthocyanins from pomegranate peel
The chemical resilience of anthocyanins, which allows them to endure several environmental elements, including heat, light, pH, and oxygen without undergoing breakdown or deterioration, is recognized as their capacity for stability. Several factors can impact the stability of anthocyanins, such as pH, temperature, and the existence of metal ions.33,34 Their experiment suggests that employing maltodextrin for encapsulation significantly boosts the stability of anthocyanin at increased concentrations, while concurrently maintaining its antioxidant properties and efficacy. They examined how stable anthocyanin remained in freeze-dried encapsulated pomegranate peel powders compared to a control sample and also tested different storage conditions involving temperature and relative humidity. The anthocyanins found in the powder extracted from pomegranate peel exhibited characteristics consistent with first-order kinetics. Generally, the pomegranate peel's total anthocyanin content (TAC) in extraction-microencapsulated powder declined over extended storage periods, with elevated storage temperatures corresponding to diminished retention rates. Out of the various wall concentrations examined in their research, the combination of 15% MDX with 0.1% calcium alginate showed the most advantageous results in terms of both yield and efficiency. These particles showcased improved anthocyanin retention and a high glass transition temperature (Tg), factors that contributed to the superior physical stability of the microparticles.
Copigmentation emerges as a promising and economically viable strategy for stabilizing anthocyanins, involving their interaction with copigments. Numerous phenolic substances have been identified as effective copigments in this situation, including gallic acid, ellagic acid, rosmarinic acid, chlorogenic acid, ferulic acid, caffeic acid, quercetin, and rutin. Mazhitova et al.35 investigated the possibility of using powdered mandarin peel, orange peel, and pomegranate peel as co-pigments for anthocyanins extracted from barberry, black hawthorn, dewberry, red hawthorn, and viburnum. The results showed that degradation rate of anthocyanins increased with higher temperatures, but co-pigmentation resulted in enhanced stability. The degradation kinetics were examined across temperatures between 70 and 90 °C, uncovering first-order reactions with rate constants ranging from 0.45 to 2.93 min−1 for reference samples and 0.30 to 2.00 min−1 for co-pigmented samples.
3.1. Alginates and their derivatives
Alginates isolated from brown seaweeds are composed of β-D-mannuronic acid (M) and α-L-guluronic acid (G). They show capacity to create consistent, water-resistant, see-through gels that can't be undone by heat under normal conditions through cross-linking with divalent or trivalent ions, often using solutions of calcium chloride. Alginates are characterized by their wide accessibility, biodegradability, and cost-effectiveness in contrast to organic casings.36,37 Zam et al. (2014)38 achieved an encapsulation rate of 43.90% for total polyphenols and 46.34% for proanthocyanidins utilising 3% alginate in their ionic gelation encapsulation of pomegranate peel polyphenolic extract in alginate beads. Because of its ability to create gels, stabilise surfaces, and hold onto water, alginate is extensively utilised in a variety of sectors, frequently in conjunction with other polymers. Optimising alginate as a gelling agent for controlled release and stability of encapsulated bioactive compounds requires an understanding of parameters such as thickness, porosity, and permeability.39 Rahnemoon et al. (2021)40 utilized alginate nanospheres with PPE integrated through calcium chloride nanoparticle external gelation and water-in-oil (W/O) emulsification as mentioned in Fig. 1. Findings indicated that the nano-encapsulated PPE exhibited a significantly greater inhibitory zone in opposition to the examined bacteria following a 48-hour incubation period. The nanospheres containing PPE demonstrated heightened antimicrobial properties in chicken breast throughout the two-week storage period (4 °C) in comparison to those achieved with PPE-containing and PPE-free alginate.
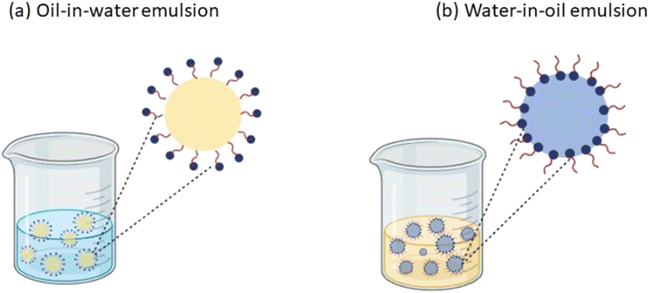 |
| Fig. 1 Different types of emulsion for encapsulation, (a) oil-in-water and (b) water-in-oil. | |
3.2. Chitosan and its derivatives
Chitosan, with its cationic nature from primary amino groups, is a prominent polysaccharide-based polymer. These groups offer various properties such as mucoadhesion for improved pulmonary drug delivery and controlled drug release. However, its solubility is constrained under physiological pH conditions because of the partial protonation of amino acids when proteolytic enzymes are present, which can lead to premature drug metabolism in intestinal and gastric fluids.41 Chitosan's positively charged nature and its ability to encapsulate natural ingredients make it suitable for creating various matrices, including nanoparticles, nanoemulsions, nanofibers, hydrogels, films, and coatings. Its designation as Generally Recognized As Safe (GRAS) underscores several advantages, including its non-toxicity, biocompatibility, and antimicrobial attributes, thereby positioning it favorably for biomedical applications. Soltanzadeh et al. (2021)42 developed chitosan nanoparticles to encapsulate pomegranate peel extract, intending to protect its delicate components. The produced nanoparticles exhibited a spherical morphology, with diameters spanning from 174 to 898 nm. Their zeta potential fell within the range of +3 to +36 mV, while the encapsulation efficiency varied between 26% and 70% and loading capacity varied from varied from 14% to 21%, contingent upon the concentration of the extract. Chitosan nanoparticles, with a ratio of chitosan to pomegranate peel extract at 1
:
0.50 (w/w), demonstrated promising physical stability. Chitosan derivatives such as N-acylated chitosan derivatives demonstrate improved biocompatibility and anticoagulant characteristics. They provoke no inflammatory reactions upon administration into humans, rendering N-acylated chitosan well-suited for pharmaceutical applications, particularly as a carrier or for controlled release functions. N-Acylated chitosan, known for its exceptional solubility, functions effectively as a carrier for hydrophobic drugs.43
3.3. Protein-based encapsulation
Utilizing protein-based encapsulation is a promising strategy for safeguarding and delivering active substances across diverse domains, encompassing but not restricted to delivery of drugs, food technology, and healthcare.44 Protein-based nanocarriers from diverse origins such as plants, animals, and microbes present benefits in encapsulating and preserving bioactive components within the medical, food, and cosmetic sectors.45 Protein-based encapsulation is gaining increasing attention in fields such as food science and medicine. Different techniques are employed to achieve protein encapsulation, with liquid–liquid phase separation and complex coacervation emerging as two commonly employed strategies.46 Using legume proteins as a covering material in microencapsulation delivery systems is explored, emphasizing their functional attributes. Additionally, the integration of high-pressure and ultrasound technologies is examined to enhance encapsulation properties in this context.47 Protein-based nanocarriers, including albumin, gelatin, casein, and whey nanoparticles, are commonly employed in drug delivery applications. Whey protein is renowned for its exceptional functional characteristics, such as enhanced ability to combat radicals and improved properties for emulsification and foaming. Moreover, it is acknowledged for its outstanding digestibility and its capacity to produce bioactive peptides more effectively during digestion. These advantageous qualities have prompted the extensive use of whey proteins in drug delivery applications. Whey protein nanoparticles (WPNs) possess the ability to attach to various bioactive substances that are both hydrophobic and amphiphilic because of functional groups inside the structure of their polypeptides. This feature enables them to hold these compounds in check until their release in desired areas in the body.48
3.4. Polysaccharide-based encapsulation
Polysaccharide-based encapsulation has demonstrated significant potential across various biomedical applications, serving as delivery platforms for therapeutic agents, viscoelastic matrices for cell encapsulation, tissue scaffolds, and wound healing dressings.49 Polysaccharide carriers such as starch, pectin, and cellulose, stand out due to their renewable nature, biocompatibility, and inert properties, making them well-suited for specific release of nutraceuticals.50 Šavikin et al. (2021)24 utilized spray drying technology to encapsulate pomegranate peel, utilizing carbohydrate-based carrier materials, such as maltodextrin, and protein-based carriers such as whey protein (WP) at various concentrations (80%, 100%, and 120%). The study looked at how the nature and level of concentration of the carrier material affected the final encapsulated powder's stability and quality. The microencapsulation of the pomegranate peel (PP) extract at full (100%) concentration using a carbohydrate-based carrier produced the greatest results. This formulation exhibited an encapsulation efficiency of 88.63%, a hygroscopicity of 15.17%, and a water solubility index of 87.04%. Moisture content ranged between 3.69% and 4.60% for maltodextrin (MD) and between 4.21% and 5.84% for whey protein (WP). Nanoscale carriers derived from polysaccharides exhibit the capacity to entrap, safeguard, and release bioactive ingredients, allowing the construction of pH-responsive delivery vehicles tailored for intestinal-targeted delivery. Polysaccharide-based nanopolymers are increasingly utilized in biomaterial fabrication for various purposes, including drug delivery, cell encapsulation, and targeted therapy for tumors. Moreover, these nanofibers derived from polysaccharides can be added to food items to enhance the nutritional value and provide health advantages.51 Soltanzadeh et al.42 successfully encapsulated pomegranate peel extract (PPE) in chitosan nanoparticles (CSNPs) via the ionic gelation method. A wall material to extract a ratio of 1
:
0.50 (w/w) was found to yield the best concentration of PPE. With a diameter of 208 nm and a homogeneous size distribution, the resultant nanoparticles demonstrated a spherical shape and an appropriate level of encapsulation effectiveness.
3.5. Safety and toxicity of wall materials
The wall material serves as a protective barrier which shields core bioactives from environmental conditions such as heat and light during both the encapsulation process and subsequent handling. Ensuring wall material safety is essential for controlled release of bioactive chemicals. Utilizing materials deemed “generally recognized as safe” (GRAS) is vital for creating secure nanocapsules suitable for their intended purposes, while simultaneously preserving the nutritional stability and quality required for food or medicinal applications. These GRAS materials typically demonstrate controlled-release properties, with examples including polysaccharides sourced from plants (such as gum arabic) or microorganisms (such as xanthan gum).52 A wall material should have the capacity to polymerize, withstand mechanical strain, and endure various environmental conditions such as air moisture, temperature fluctuations, and water activity. Moreover, the substance should be safe for human consumption, free from allergens and toxins, sterilizable, and either flavorless or having a mild taste, ensuring its suitability for encapsulating food components.53,54 Wall materials utilized in microencapsulation methods for use in the food and pharmaceutical industries possess the ability to endure the acidic and enzymatic environments found in the stomach and small intestine.55 Polysaccharide based wall materials such as chitosan display numerous therapeutic benefits, including stimulating the immune system, reducing cholesterol levels, lowering blood pressure, inhibiting microbial growth, relieving pain, and enhancing blood clotting and skin cell regeneration. These properties arise from chitosan's advantageous pharmaceutical characteristics, such as its compatibility with the body, cost-effectiveness in production, capability to bind certain organic substances, susceptibility to enzymatic breakdown, and lack of toxicity. The wall material facilitates the precise delivery of bioactive substances to specific locations in the body, thereby decreasing overall systemic exposure and lowering the chance of toxicity to unintended tissues. Wall materials have selective permeability characteristics, permitting the passage of specific substances while obstructing others. This selectivity aids in preventing the ingress of harmful molecules while enabling the release of beneficial compounds. The wall material builds a physical wall around the bioactive, thereby avoiding direct interaction with the surrounding environment. This barrier serves to protect the active ingredient from external factors that could potentially induce toxicity.41
3.6. Encapsulation studies of pomegranate peel extract
Pomegranate peel extract has been the focus of in-depth investigation regarding its ability to encapsulate a variety of bioactive compounds. Scientists have concentrated on the polyphenolic content present in pomegranate peels and have investigated diverse extraction methods.56 Pomegranate peel extract has been encapsulated using a variety of methods, including co-crystallization with sucrose, nanoencapsulation using maltodextrin and Lepidium perfoliatum seed gum, and encapsulation within gelatin/sodium alginate composite “boba balls”.57,58 These encapsulation approaches have displayed promising outcomes in terms of preserving the bioactive components, enhancing antioxidant characteristics, and enhancing the stability and shelf life of encapsulated extracts.59 The utilization of lipid-based nanocarriers, such as nanostructured lipid carriers and solid lipid nanoparticles, has shown significant efficacy in encapsulating bioactive compounds derived from pomegranate peel.60 Encapsulating PPEs in suitable wall materials could improve their solubility and conceal any strong undesirable tastes, such as astringency, which might otherwise restrict their applications.61 Utilizing chitosan nanoparticles for encapsulating extract of pomegranate peel is a beneficial approach to shield the delicate components of extract. The FTIR spectra of pure pomegranate peel extract displayed distinctive absorption bands associated with phenolic compounds such as tannic acid, ellagic acid, and gallic acid. Assessing the total phenolic content (TPC) and antioxidant properties in chosen chitosan nanoparticles indicated a considerable drop in TPC along with antioxidant activity when measured against PPE that has not been encapsulated. This suggests that during the measurements, chitosan nanoparticles successfully shielded PPE against fast release.42 Sanhueza et al.62 investigated if pomegranate peel extract (PPE) could be successfully integrated into double emulsions. At a 70
:
30 ratio (oil
:
PPE), a variety of oils, including castor, soybean, sunflower, miglyol, and orange, were used, emulsifying them using a variety of techniques such as mechanical agitation and direct membrane emulsification. The hydrophilic emulsifier Tween 80 was used, and the lipophilic emulsifier polyglycerol polyricinoleate (PGPR) was used. Notably, direct membrane emulsification (DME) resulted in emulsions with enhanced stability over time. Double emulsions (DEs) produced through direct membrane emulsification (DME) exhibited superior characteristics concerning droplet size, shape, and encapsulation efficiency (EE) compared to those generated via mechanical agitation (MA) as mentioned in Table 7.
4. Colloidal systems used in the encapsulation of pomegranate peel extracts
4.1. Emulsions
Emulsions are colloidal mixtures comprising at least two fluids that do not naturally mix, where one fluid forms small droplets dispersed within the other.63 Emulsion science is extensively used in the food industry to produce goods including dairy products, drinks, butter, mayonnaise, and margarine. Emulsions improve the look, feel, and flavour of these goods and work well as a delivery vehicle for encapsulating bioactive substances such as vitamins and nutraceuticals.64 Sanhueza et al.62 evaluated the viability of encasing pomegranate peel extract (PPE) in double emulsions with two distinct emulsification techniques (mechanical agitation and direct membrane emulsification) and distinct oils in a 70
:
30 oil
:
PPE ratio with Tween 80 and polyglycerol polyricinoleate (PGPR) acting as hydrophilic and lipophilic emulsifiers, respectively. The double emulsions made with oils such as sunflower or miglyol showed improved stability, smaller particle sizes, higher EE, and controlled release. Double emulsions developed with sunflower oil via direct membrane emulsification were identified as appropriate vehicles for encapsulating punicalagin. Nanoemulsions with their reduced droplet size (20–200 nanometres) provide better resistance to gravity separation, improved optical clarity, and improved bioavailability of the bioactive substances they contain.65 Modifying the manufacturing and formulation processes will allow for precise control over droplet size. Hydrophobic bioactive ingredients in food and drink can be efficiently encapsulated and delivered using these nanoemulsions. Tan et al.66 showed that whole-fat milk formulations containing curcumin or vitamin D have demonstrated exceptional chemical and physical stability, even when stored for extended periods of time.
4.2. Liposomes
Liposomes are spherical lipid particles that have been extensively employed for precise drug delivery as mentioned in Fig. 2. However, growing attention has been shown in using them for functional foods. Liposomes possess the ability to entrap substances that exhibit solubility in water, lipids, or both, rendering them attractive vehicles for the food sector. The potential for large-scale production of liposomes, combined with their remarkable targeting capabilities, provides a major benefit for their application in food based industries. Ganjian et al.67 utilized liposomes as a delivery system for pomegranate peel extract. They studied how pomegranate peel extract, both encapsulated and unencapsulated, affected the quality of chilled silver carp fillets. Lower total volatile basic nitrogen and thiobarbituric acid levels showed that the extract was successful in lowering chemical degradation and lipid oxidation in the fillets in contrast to the control. Additionally, pomegranate peel extract resulted in a decrease in the total viable count of the fillets by approximately 2
log
10 CFU g−1 when compared to the control. Furthermore, the efficiency of the extract was enhanced through liposomal encapsulation. Also, the enclosing substances in liposomes offer a protective shield to the enclosed substance, shielding it from variations in environmental conditions such as temperature, humidity, changes in pH, and fluctuations in ionic strength at each stage of processing as well as after processing. Importantly, lipids and taste receptor cells don't interact, making liposomes an effective choice for concealing the unpleasant taste of bitter bioactive compounds such as chloroquine phosphate, quinine, denatonium, and propranolol. Furthermore, liposomes can be used to encapsulate different polyphenolic substances.6 Dundar et al.68 formulated nanophytosomes (NPs) containing pomegranate peel extract (PPE) utilizing phosphatidylcholine (PC) through the thin layer hydration technique. The outcomes exhibited a remarkable encapsulation efficiency of 94.99%, highlighting its distinctive potential as a nano-carrier. The PPE-loaded NPs displayed favorable attributes, including reduced PDI values (p < 0.5), smaller particle size ranging from 166.70 to 144.40 nm, and a spherical microstructure. The least TPC and color deviation over a 28-day storage period were observed at 4 °C, notwithstanding that all NP specimens exhibited enhanced stability under various storage conditions for a maximum of 21 days.
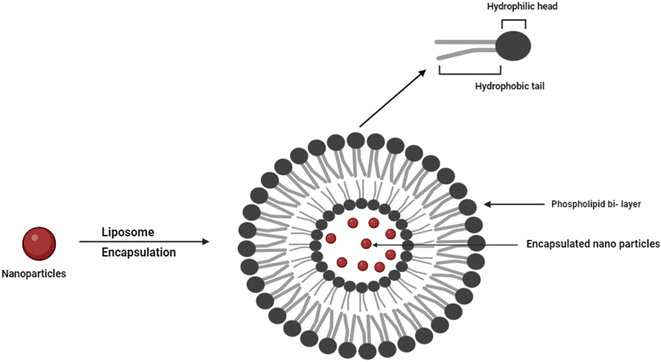 |
| Fig. 2 Schematic representation of liposome encapsulated nanoparticles. | |
5. Encapsulation processes of pomegranate peel
Encapsulation techniques for pomegranate peel involve spray drying, freeze drying, ionic gelation, coextrusion, coacervation, electrospinning and electrospraying. Spray drying involves converting the peel extract into droplets and swiftly drying them to yield powdered particles. Freeze drying protects delicate compounds by freezing the extract and evaporating the frozen solvent under vacuum. Ionic gelation relies on polymer interactions to form a protective gel matrix around the extract. Coextrusion merges the extract with a carrier material to form composite structures. These processes protect and transport bioactive compounds from pomegranate peel extract, improving stability and encapsulation efficiency and enabling controlled release as shown in Table 1.
Table 1 Encapsulation of anthocyanin and phenolic compounds from pomegranate peel using different wall materials and encapsulation techniques
Sources |
Bioactive compounds |
Technique |
Encapsulating material |
Concentration used & particle size (μm) & process conditions |
Encapsulation efficiency (%) |
Properties studied |
Main finding |
Reference |
Pomegranate peel extract |
Anthocyanin |
Nano-encapsulation |
Maltodextrin and lepidium perfoliatum seed gum |
Extract: wall material – 1 : 2, 329 μm. Frozen at −18 °C for 24 h and freeze dried for 48 h |
96.15% |
• Compared anthocyanin content from different extraction techniques |
• Microwave extraction produced the most anthocyanins and phenolics |
69
|
• Evaluated effectiveness of pomegranate peel extract |
• Nanoencapsulation enhanced phenolic retention |
Pomegranate peel |
Anthocyanin |
Freeze drying |
Maltodextrin and β-cyclodextrin |
Extract: wall material – 1 : 5, 39.8 and 47.5 μm. Storage temperature (25 ± 1 °C), centrifuged for 30 minutes at 22 °C at 120 rpm |
The encapsulation efficiency ranged from 86% to 93% |
• Evaluated the physicochemical attributes and morphological characteristics of bioactives |
• Anthocyanin content in a 15% wall material microencapsulated powders decreased by 18% after 42 days of freezing |
34
|
• The powder with a 15% wall material retained the highest anthocyanin content |
Pomegranate peel |
Phenolics |
Spray drying |
Maltodextrin |
With a 50 : 50 ratio, an inlet air temperature of 150 °C, dry air flow rate of 17.5 m3 h−1, a wall-to-core material ratio of 9 : 1, and a feed solid concentration of 30% (w/w) |
The encapsulation efficiency ranged from 69.80% to 99.80% |
• Focuses on optimizing phenolic extraction from pomegranate peel |
• Ultrasound enhanced extraction efficiency significantly, reducing the processing time by more than 20-fold |
70
|
Pomegranate peel |
Phenolics |
Ionic gelation |
Chitosan |
Chitosan to PPE is 1 : 0.50, particle size – 208 nm, pH 5.6, temperature 4 °C, and a loading capacity of 14–21% |
51% |
• Evaluated chitosan nanoparticles for protecting and delivering natural antioxidants |
• PPE loaded nanoparticles were effectively encapsulated and confirmed by lower TPC and DPPH RSA values compared to pure PPE |
42
|
• Novel application in the food industry |
• Physical properties of nanoparticles are influenced by the PPE loading concentration |
Table 2 Different types of wall materials used for encapsulation
Core material |
Wall material |
Results |
Reference |
Phenolic compound |
Whey protein isolate with maltodextrin (50 : 50) |
• Encapsulated phenolic extract has limited solubility in high-lipid environments |
70
|
• Still extends the shelf life of hazelnut paste |
Anthocyanins |
Maltodextrin and β-cyclodextrin |
• Freeze-dried MDX-10% encapsulated powder had the highest TPC at 4 °C |
71
|
• Encapsulated extracts suppressed yeast and mould growth |
Ellagic acid |
Spirulina |
• In vitro digestion results provided an understanding of ellagic acid content and effectiveness |
72
|
• Stimulated intestinal fluid releases ellagic acid faster than stomach fluid |
Anthocyanin |
Maltodextrin and calcium alginate |
• Freeze-dried encapsulated powder with 15% maltodextrin had the highest anthocyanin concentration |
18
|
Table 3 The main bioactive compounds from pomegranate peel extract with their relevant biological activity
Bioactive compound |
Main biological activity/function |
Reference |
Anthocyanins |
• Anti-oxidant |
34
|
• Anti-inflammatory |
• Anti-cancer |
• Chemoprotective |
Punicalagin |
• Scavenging free radical |
19
|
• Anti-proliferative |
• Anti-inflammatory |
• Hepatoprotective and antigenotoxic activity |
Ellagic acid |
• Antioxidant |
72
|
• Antihepatotoxic |
• Antisteatosic |
• Anticholestatic |
• Antifibrogenic |
• Antihepatocarcinogenic |
• Antiviral |
Gallic acid |
• Antibacterial |
24
|
• Antiallergy |
• Anti-inflammatory |
• Antioxidant |
• Scavenging free radical |
Flavonoids |
• Anti-oxidative |
69
|
• Anti-inflammatory |
• Anti-mutagenic |
• Anti-carcinogenic |
5.1. Spray drying
Spray drying is among the most widely utilized techniques for encapsulating bioactive compounds due to its exceptional efficiency. Hu et al.73 through their work achieved a 93% encapsulation efficiency by using the spray drying process to encapsulate cinnamon essential oil. They used whey protein isolate, maltodextrin, and sodium alginate as wall materials (WPI
:
MD
:
sodium alginate = 1
:
3
:
0.01 (w/w)). Within the food sector, the spray drying method converts liquids into stable, conveniently usable powder forms (Fig. 3). This process typically includes dissolving, emulsifying, or dispersing active ingredients, then atomizing and spraying. The core material is initially distributed within an aqueous solution alongside the carrier compound, resulting in the creation of minuscule droplets measuring a few microns in size.53 Innovative nozzle designs blend oil and carrier materials before atomization, eliminating the need for emulsion preparation, as seen in multi-fluid channel nozzles. These droplets are then dehydrated in a heated spray dryer chamber, forming capsules with diameters of 10 to 300 μm. Drying occurs using hot air. This makes it possible to spray dry even thermally sensitive compounds without experiencing significant losses. However, an alternative approach involves initially dissolving bioactive agents in organic solvents. This method is seldom employed due to concerns related to the environment, safety, and expenses. The extensive utilization of spray drying within the food sector can be linked to its convenient manageability, cost efficiency, and capacity to attain elevated production levels. The key to successful spray-dried microencapsulates lies in producing powders with minimal surface oil and maximal retention of bioactive compounds.6 Šavikin et al.24 utilized spray drying as a method for encapsulating pomegranate peel extract. The approach involved employing spray drying with carrier materials based on carbohydrates (maltodextrin, MD) and proteins (whey protein, WP) at varying concentrations (80%, 100%, and 120%). The findings indicated that the microparticles produced using maltodextrin successfully maintained polyphenolic compounds post spray drying. The kind and amount of the carrier material affected the properties of the final powder. Maximum encapsulation efficiency, hygroscopicity, and water solubility index were attained when utilizing a carrier composed entirely of carbohydrates for the extraction of pomegranate peel. Both maltodextrin and whey protein powders had moisture content suitable for long-term storage (3.69% to 5.84%). Maltodextrin was superior to whey protein in enhancing bioavailability and functionality of bioactive compounds from pomegranate peel. The physicochemical attributes of the chosen wall material are critically significant because they directly influence the effectiveness and stability of encapsulation. Essential characteristics of a wall material should include its capacity to form polymers, withstand mechanical pressures, and withstand a range of environmental factors, including temperature, humidity, and water activity. Furthermore, the selected material must be safe for human consumption, free of toxins and allergens, able to undergo sterilization, and either lacking in flavor or having a subtle taste, thereby facilitating its use in the encapsulation of food components. Recently there has been increasing interest in utilizing spray drying technology for substances such as polyphenols, carotenoids, flavonoids, and anthocyanins. This heightened interest arises from the considerable biological potential of these compounds, notably their antioxidant properties, which enhance their attractiveness for incorporation into food items.54
 |
| Fig. 3 Schematic flow chart of comparison between spray drying and freeze drying of anthocyanins. | |
5.2. Freeze drying
Freeze-drying is an excellent method for producing powders with certain characteristics, such as delicate bioactive compounds (Fig. 3). The feed emulsion is frozen, and ice crystals are subsequently sublimated using low pressure. Table 8 illustrates how expensive freeze-drying is because a vacuum pump is required. It takes 24 to 48 hours to complete the procedure, and it uses a lot of energy.74 Nguyen et al.75 achieved a 95.7% encapsulation efficiency by employing wall materials such as gum arabic and maltodextrin to successfully encapsulate anthocyanin by using the freeze-drying process. Depending on the chemical being encapsulated, each encapsulation process offers advantages and disadvantages (Table 7). Freeze-drying works well for microencapsulation and is the best method for heat-sensitive materials. Freezing, sublimation, desorption, and storage are the four processes involved. It protects biological items and foods that are susceptible to heat by employing sublimation. The makeup and form of the encasing material determine how well protection or controlled release works. Whey protein, gum arabic, maltodextrin, and emulsifying starches are examples of common encapsulating agents.76 Azarpazhooh et al.34 used the freeze-drying method to encapsulate the bioactive substances that were extracted from pomegranate peel using ethanol that had been acidified. In this encapsulation technique, maltodextrin was employed at varying concentrations of 5%, 10%, and 15%, in conjunction with calcium alginate at a concentration of 0.1%, at a weight ratio (w/w) of 1
:
5 (extract to wall material). The study's findings demonstrated a strong relationship between the rate of maltodextrin and the process yield, which was explained by the latter's higher blend content.
5.3. Ionic gelation
Ionic gelation is recognized as ionotropic gelation that starts with the blending of an aqueous polymer solution with low molecular weight ions possessing opposite charges to the polyelectrolytes (Fig. 4). These ions interact with the polyelectrolytes, resulting in the creation of an insoluble gel. The fundamental concept behind this encapsulation is to entrap an active substance within this gel and release it upon the gel's phase transitions triggered by external stimuli. Several methodologies are employed to trigger the commencement of the liberation of the encapsulated bioactive compound, including alterations in pH, mechanical agitation, enzymatic processes, and osmotic gradients, leading to subsequent release via diffusion. Ordinarily, a solution comprising polymers or hydrocolloids is introduced into an ionic solution via dripping or atomization while ensuring continuous agitation (Kurozawa and Hubinger, (2017)).77 This process is straightforward and uncomplicated, devoid of the need for specialized equipment, elevated temperatures, or organic solvents, and can be regarded as a cost-effective approach. Commonly employed coating agents such as alginate, low methylation pectin, chitin, and chitosan, along with the calcium ion (Ca2+), serve as prevalent cross-linking agents. These substances can be regarded as highly effective encapsulation systems for food components and controlled release applications due to their non-toxic nature, strong biocompatibility, and robust mechanical properties. Ionic gelation-generated capsules find extensive utility in encapsulating hydrophobic substances. This is because the capsule shell materials, including alginate, gelatin, agar, low methylation pectin, and gellan gum, exhibit hydrophilic properties. Consequently, the method is primarily employed for encapsulating things that are hydrophobic or have very little solubility.77 de Moura et al.78 achieved a remarkable encapsulation effectiveness of 93.9% when encapsulating anthocyanins that were derived from hibiscus by utilizing the ionic gelation method. Pereira Silveira et al.79 created pectin hydrogel beads for carrying pomegranate extract using ionic gelation. Initially, the formation of pectin beads through traditional external ionic gelation resulted in a 41% retention rate. However, through the internal and exterior medium's concentration gradient being optimized, the retention rate was significantly increased to approximately 101%. The study revealed significant advancements in the characteristics of pectin–starch beads generated via adsorption, indicating improved microstructure stability and decreased release rates. Furthermore, these beads exhibited a substantial content of 2960.26 ± 26.92 mg of GAE/100 g sample. Even though the coating technique successfully slowed down the rate of release in every bead, it resulted in a 32% reduction in phenolic compounds in the chitosan solution, which adversely affected the retention as a whole.
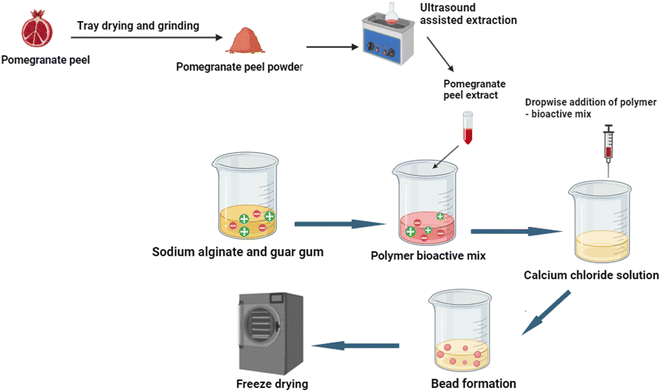 |
| Fig. 4 Schematic flowchart of ionic gelation. | |
5.4. Co-extrusion
Extrusion is described by the bio-based solution being injected into a different solution to foster gelation. This process results in the creation of a sturdy, glassy, and compact structure that functions as an efficient barrier for bioactive substances against oxidation and evaporation during storage, allowing for controlled release. Co-extrusion is the technique of extruding two or more biopolymers together to create the finished product. This technique can be utilized for the generation of spherical configurations featuring a hydrophobic nucleus encircled by either a hydrophilic or hydrophobic covering.26 Pereira Silveira et al. (2023)79 employed an ionic gelation method, utilizing extrusion, to encapsulate pomegranate polyphenols. Remarkable results were noted with pectin–starch beads created via adsorption, exhibiting a content of 2960.26 ± 26.92 mg of GAE/100 g sample, enhanced stability, and reduced rates of release. To attain efficient encapsulation, both the central and outer solutions must exhibit mechanical characteristics that are suitable for the coextrusion procedure. These attributes encompass viscosity levels below 2000 mPa s, interfacial tensions spanning from 10 to 72 mN m−1, and fluid densities within the spectrum of 0.7 to 1.3. Every coextrusion technique provides benefits, including the creation of a protective barrier for encapsulated components, controlled and gradual release of the ingredients, and resistance to oxidation. Nevertheless, it is important to consider certain limitations, including the necessity to monitor specific core and shell fluid rates throughout the process, as well as the requirement for precise and high-quality equipment.80
5.5. Electrospinning and electrospraying
Electrospinning is a non-mechanical technique that uses an outside electric field to constantly create sub-micron or nano-sized fibers. It is a versatile and uncomplicated approach capable of generating electrospun fibers characterized by significant porosity and a notable surface-to-volume ratio. Variables including the polymer concentration, molecular weight, solution viscosity, voltage applied, tip-to-collector distance, and flow rate affect these fibers' properties. Recently, electrospinning has surfaced as a feasible substitute for conventional techniques utilized in microencapsulation. This technique includes using of electrical voltage on the polymer solution, which leads to the accumulation of electrical charges on the liquid's surface.81 Electrospinning offers a range of advantages when compared to other manufacturing methods. First and foremost, it presents a user-friendly, cost-efficient, and direct method for manufacturing nanofibers. Secondly, it enables the smooth integration of bioactive substances into said nanofibers. Additionally, electrospinning diminishes the size criteria for bioactive substances, facilitating their inclusion in food items without impacting sensory attributes. The absence of heat generation throughout the electrospinning process is another important benefit, particularly for preserving the stability of sensitive chemicals. Apart from these benefits, nanofibers generated via electrospinning offer diverse structural and functional advantages. These encompass diameters ranging from submicron to nanoscale, a high ratio of surface area to volume, appropriate porosity, customizable fiber diameters, and a high efficacy in encapsulating bioactive compounds.82
In the electrospraying technique, solid particles are created with a uniaxial electrospraying instrument. Bioactive components and polymers found in food are dissolved in a solvent during processing. This resulting solution is then employed as the electrospraying solution to produce solid particles through the electrospraying method. These electrosprayed solid particles have been investigated for their potential use in encapsulating biological macromolecules from functional food extracts, in an effort to strengthen their stability. Additionally, they have been explored for modifying the release characteristics of these functional food extracts when encapsulating biological macromolecules.83 The electrospraying technique can be adapted for various applications, with a primary focus on its utility in the non-edible food sector. Specifically, it has been employed in the development of smart packaging solutions designed to resist the intrusion of pathogens. Moreover, nanostructures are created with polymeric materials from food for encapsulating bioactive compounds or food ingredients.10,84
5.6. Coacervation
Coacervation is a phrase used to explain how phase separation can be caused by changes in environmental parameters such as pH, ionic strength, temperature, and solubility. During this procedure, the phase enriched with colloidal substances is termed the coacervate phase, while the phase containing minimal colloidal content is referred to as the equilibrium phase. Coacervation, frequently used in the pharmaceutical and culinary industries, is recognized as an extremely effective method for micro/nanoencapsulation. The process of encapsulation through coacervation entails the creation of a polymer coacervate encasing the active ingredient, resulting in the deposition of the enclosed substance. When encapsulating food ingredients, two oppositely charged biopolymers—mostly proteins and polysaccharides—are typically used in the complicated coacervation process to create a complex shell around the core substance. The majority of the proteins used come from plants (soy protein, pea protein, wheat protein, lentil protein, and chia protein) and animals (gelatin, whey proteins, egg albumin, and silk fibroin). Alginate, chitosan, gum arabic, pectin, agar, carrageenans, and carboxymethyl cellulose are among the polysaccharides that are also used.85 Jafarian et al. 2021 (ref. 86) carried out an investigation wherein Prunus armeniaca gum exudates and chitosan were utilized as wall materials for encapsulating pomegranate peel extract through the process of coacervation. The findings indicate that merely 14.2% of phenolic compounds were discharged from Prunus armeniaca gum exudates/chitosan microparticles into the simulated gastric fluid (SGF), implying that the pomegranate peel extract was effectively shielded within the SGF. In their study (B. Wang et al., 2018),87 coacervation is defined as a colloidal phenomenon in which a system splits into two different liquid phases: the coacervate phase, which is dense and polymer-rich, and the continuous phase, which is polymer-poor. These coacervates can be employed as microencapsulation shell materials. Generally speaking, two varieties of coacervation processes exist: simple and complicated, which include one or more polymers, respectively. The polymer is frequently precipitated in simple coacervation by adding electrolytes, such as sodium sulfate, separating it with water-miscible solvents, such as ethanol and methanol, or adjusting the temperature or pH. Simple coacervation is achieved by altering conditions that induce molecular dehydration of macromolecules. This can be accomplished through various means such as the addition of microions and a non-solvent, a change in temperature, or a shift in pH. By adjusting solvent conditions, such as altering the mixing ratios of water and various alcohols in a ternary solution containing a water-soluble macromolecule and two solvent components, phase separation can occur. At specific alcohol/water ratios within this ternary solution, demixing takes place, resulting in two phases. One phase contains a high concentration of the macromolecule, while the other phase, in equilibrium, holds a more diluted concentration of the macromolecule. This process leads to the phenomenon of simple coacervation. Complex coacervation is characterized by the segregation of a solution containing macromolecules into two separate, immiscible liquid phases, consisting of two macroions with opposite charges. This phenomenon is achieved within biopolymer pairs, such as protein–polysaccharide mixtures, through precise control of external parameters that induce electrostatic interactions between oppositely charged macroions. Among these polymer pairs, the coacervation of polysaccharides and proteins has garnered significant interest in recent decades, particularly within the realm of encapsulating active ingredients.88
6. Effects on physicochemical properties
Spray-dried encapsulated food material's moisture content and water activity are the primary variables influencing its shelf life and storage circumstances. Food items are considered acceptable if their moisture level and water activity levels are less than 0.6 and 0.3, respectively, as these circumstances help improve stability.89 Razak et al. (2018)90 investigated to analyze the moisture content changes of encapsulated Orthosiphon stamineus spray-dried powder on a weekly basis. They discovered that the spray-dried powder incorporating Orthosiphon stamineus had a constant initial moisture content of 7.16% in all tests. However, there was a significant increase in moisture content for samples stored in vacuum packaging after one week, at both 10 °C and 25 °C. Subsequently, the moisture content remained relatively stable from weeks 2 to 4 for samples stored at both temperatures. On average, there was a 10.1% increase in moisture content for samples stored at 10 °C and a 46.2% increase for those stored at 25 °C.
The degree of hygroscopicity significantly affects how long a product lasts and how it is stored. According to Mohd Nawi et al.91 the powder's hygroscopicity was greatly affected by the addition of gum arabic, maltodextrin, and a combination of the two as wall materials. The least hygroscopic sample was the one made with maltodextrin. Gum arabic microcapsules had the highest hygroscopicity of all the powders, measuring 18.736%. The combination of gum arabic and maltodextrin came in second at 15.181%. On the other hand, the powder that was encased in maltodextrins showed the lowest hygroscopic moisture content (10.028%).
Kaderides et al. (2020)92 reported that the red color component (a*) increased during storage, increasing from 0.11 to 3.73 for the contained extract and from 5.06 to 7.36 for the crude extract. The increased release of anthocyanidins from proanthocyanidins may be the cause of this alteration. Furthermore, the yellow color component (b*) of the crude extract dropped dramatically during storage, going from 16.74 to 8.02, whereas enclosed extract's decline was less severe, going from 31.65 to 25.34. Punicalagin is linked to the distinctive yellow color of pomegranate peels; hence, a decrease in yellowness during storage may be related to a drop in the punicalagin concentration. Furthermore, for the encapsulated and crude extracts, the lightness (L*) decreased slightly at the start and end of storage (41 days), from 73.10 to 66.69 and from 45.99 to 36.57, respectively. This suggests that parameter b* had a stronger impact on lightness than parameter a*.
Nguyen et al. (2022) stated that the encapsulation efficiency (EE) of anthocyanins of freeze-dried konjac glucomannan and spray-dried maltodextrin/konjac glucomannan showed the lowest EE at 43.6% and 55.4%, respectively, showing that konjac glucomannan was ineffective in capturing these compounds in microcapsules. On the other hand, the most effective carrier was found to be maltodextrin/gum arabic which minimized the loss of anthocyanins to the microcapsule surface with an efficiency of 91.8% and 95.7%, respectively, during the spray-drying and freeze-drying processes. Guo et al.,93 through their work, developed alginate–pectin hydrogel nanoparticles containing extracts from blueberries and purple corn by adding solution dropwise to buffer at pH 1.2. When compared to purple corn extract, blueberry extract's encapsulation efficiency was noticeably higher. The difference was attributed to changes in the anthocyanins' (ACN) chemical structures and how well these structures complemented the alginate–pectin hydrogel composition in an acidic pH environment. The encapsulation efficiency was unaffected by the starting anthocyanin content or the particle's shape. Nonetheless, the alginate to pectin ratio, total gum content, anthocyanin supply, and the pH of the curing solution all had a significant effect on the effectiveness of encapsulation. The encapsulation efficiency was increased from 26% to 65% for purple maize anthocyanins and from 48% to 116% for blueberry anthocyanins by adding anthocyanins at different concentrations to the curing bath.
Homayoonfal et al. (2021)94 claimed that the generated nanoliposomes had a restricted distribution of particle sizes, a negative zeta potential, and a size range of 141 to 196 nm. Interestingly, samples with an extract content of 9% had the highest encapsulation efficiency (43%). The findings demonstrated that the nanoparticles' surface charge, encapsulation efficiency (EE), polydispersity index (PDI), and particle size all increased with an increase in anthocyanin content. It was discovered that the anthocyanin (AC) extract's hydrophobic interactions with the fatty acid hydrocarbon chain reduce membrane fluidity. This implies that the colloidal suspension's particle size is directly influenced by the type of encapsulated chemical, such as barberry anthocyanin extract.
6.1. Morphology
Morphology of the structure of the core–shell is determined by a combination of factors, primarily spreading coefficients and the surface polarity.95 When Zhong et al.96 evaluated the morphology of the pomegranate peel powder, they discovered that mechanical damage resulted in the breakdown of intermolecular connections, which transformed the structure from ordered into disordered (amorphous). After milling, there was a noticeable change in the morphology, which may affect the physicochemical parameters. Soltanzadeh et al.42 effectively used the ionic gelation process to encapsulate pomegranate peel extract (PPE) into chitosan nanoparticles (CSNPs). A chitosan
:
PPE ratio of 1
:
0.50 (w/w) was shown to be the most effective concentration of PPE. The resulting nanoparticles had an average particle diameter of 208 nm, a spherical shape, and a narrow, unimodal size range. Azarpazhooh et al.34 found that, in contrast to those with a low quantity of maltodextrin, the particles of enclosed powders with a significant amount of the protein were larger and smoother. Table 5 showed that varying types of wall materials and their concentrations can influence both the morphology and encapsulation efficiency.
6.2. Zeta potential
Zeta potential is the electrokinetic charge exhibited by colloidal particles when suspended within a liquid medium, acting as a gauge for the intensity of these particles’ attraction or repulsion. Zeta-potential stands as a fundamental factor influencing the stability of colloidal solutions. Low-zeta-potential colloids are more likely to coagulate or flocculate, whereas positive or negative colloids with a high zeta-potential are electrically stabilized.40 The methods of encapsulation can impact both the zeta potential and particle size as shown in Table 4. A greater zeta-potential value indicates a stronger surface charge on the nano-particles, signifying enhanced repulsive interactions among them. Soltanzadeh et al. (2021)42 developed chitosan nanoparticles encapsulating pomegranate peel extract which showed a zeta potential (ZP) between +3 and +36 mV and a mean diameter that varied between 174 and 898 nm. The ZP of these nanoparticles serves as an indicator of their surface charge and, consequently, their overall stability. The introduction of PPE into the nanoparticles resulted in a reduction of their ZP values, signifying a decline in their physical stability. The increase in the PPE concentration led to a notable (p < 0.05) decline in ZP values, with measurements dropping from 26.5 mV for a chitosan
:
PPE ratio of 1
:
0.50. Notably, there was no substantial variance in ZP values observed between CSNPs with loading ratios of 1
:
0.25 and 1
:
0.50.
Table 4 Zeta potential of encapsulated PPE bio-actives using different encapsulation techniques
Method of encapsulation |
Zeta potential and particle size |
Discussion |
References |
Ionic gelation |
35.60 ± 0.60–66.38 ± 0.22 |
• Calcium alginate nanospheres containing pomegranate peel extract enhanced antimicrobial efficacy |
40
|
205.1 ± 0.1 nm |
• The zeta-potential ranged from 35.60 ± 0.60 to 66.38 ± 0.22 indicating a strong stability |
Double emulsion |
−19.41 to −45.81 mV |
• PPE-formulated emulsions with or without carboxymethyl cellulose showed greater stability with lower particle size |
97
|
259.2–304.1 nm |
• A high encapsulation efficiency of 95% and storage-related phenolic retention were observed |
• The most stable emulsion was the one with carboxymethyl cellulose in it |
Ionic gelation |
+3–+36 mV |
• Chitosan nanoparticles with chitosan : PPE at a ratio of 1 : 0.50 (w/w) showed good physical stability with a zeta potential of 27 mV |
42
|
174–898 nm |
• They also showed the highest loading capacity of 20% and encapsulation efficiency of 51% |
Table 5 Morphology of micro/nanoparticles containing pomegranate peel extract bioactives manufactured using different wall materials
Wall material |
Morphology |
Discussion |
References |
Maltodextrin and calcium alginate |
• β-Cyclodextrin-containing microcapsules showed increased porosity, trachea development, and indentation |
• The morphological difference is due to the variations in superficial power and spatial organization of maltodextrin and β-cyclodextrin |
18
|
• Maltodextrin-containing microcapsules had thin, homogeneous, non-porous walls without any indentation |
Maltodextrin |
• Microcapsule size: 10 μm, mostly spherical |
• Uniform surface tension forces during encapsulation lead to the formation of spherical forms |
98
|
• Irregular shapes without agglomeration |
• Particle agglomeration affects the bulk density, moisture and particle size |
Tween 80 with polyglycerol polyricinoleate (PGPR) |
• Double emulsion with castor oil: considerable droplet size increase over time |
• Castor oil double emulsions: high viscosity and droplet formation energy requirements lead to an increase in droplet size |
62
|
• Sunflower and soybean oils: a lower droplet size increase over time |
• Orange oil double emulsions: low viscosity causes a reduction in droplet size |
• Orange oil: droplet size decreases, particularly when agitated mechanically |
Chitosan |
• SEM micrographs: both chitosan nanoparticles have a homogeneous size distribution and spherical shape |
• Incorporating pomegranate peel into chitosan nanoparticles increases the mean particle size diameter |
42
|
• Empty chitosan nanoparticles: smaller than those containing pomegranate peel extract |
• High homogeneity, size range of 3.6–188 μm for encapsulated extract powder |
• At a 15% concentration of maltodextrin, the mean diameter was 47.53 μm |
7. Release profile
The release profile focuses on the way in which the encapsulated substance is gradually released over time. It demonstrates both the speed and extent of the release of the active component from the encapsulating structure, whether it is a matrix or a shell.99 Jafarian et al. (2021)86 studied the PPE release kinetics from a modern encapsulating method under simulated gastrointestinal conditions. Initially, a rapid release of phenolic compounds was observed within the initial 30-minute period, attributed to their surface presence on the particles. Subsequently, a consistent release plateau ensued. Under simulated stomach conditions, a mere 14.2% of phenolic compounds were discharged from Prunus armeniaca gum exudates/chitosan microparticles, owing to the formidable barrier of the polysaccharide network that impeded release. Conversely, the release rate under simulated intestinal conditions exceeded that observed in the stomach. In their work, Yağmur et al.72 contained ellagic acid, which was taken off of pomegranate peels, within microalgae. The findings showed that after 120 minutes, the amount of phenolic substances released from the microalgae was higher in the simulated intestinal fluid (SIF, pH 6.8) than that in the simulated stomach fluid (SGF, pH 1.2). The gradual release of phenolic substances under alkaline conditions (pH 6.8) may be responsible for the little decrease in the amount of phenolic compounds seen following 120 minutes of digestion in the intestines. On the other hand, the encapsulated phenolic content in the SIF trial was higher than that in its SGF, suggesting that the acidic SGF circumstances affected the phenolic compounds' release. According to this research, wall materials such as maltodextrin or maltodextrin/gum arabic (9
:
1) released more phenolics under SGF circumstances than under SIF settings. Andishmand et al.100 examined the release pattern of PPE in simulated gastrointestinal environments and it showed a gradual increase initially. However, after 2 hours in both settings, the rate of release slowed down. This phenomenon was connected to the detachment of phenolic compounds loaded on the vesicle surfaces after 2 hours of agitation. When compared with simulated intestinal fluid (SIF), the cumulative release of pomegranate peel extract was less in simulated gastric fluid (SGF). 22.66 ± 2.51% of the originally entrapped PPE were released after two hours of stirring in SGF, indicating that the small intestine could be reached by the intended nanodelivery mechanism with little harm. In contrast, SIF had a quick release of PPE, releasing 69.33 ± 4.50% from the originally loaded PPE in under 4 h.
8. Storage stability
Storage stability refers to the ability of encapsulated substances, such as active ingredients, to maintain their quality, effectiveness, and physical attributes over a designated period when exposed to specific storage conditions. Common factors impacting the storage stability of encapsulated compounds include temperature, humidity, the type of encapsulating material, encapsulation efficiency, particle size, the chemical composition of the core material, and the presence of antioxidants or other stabilizing agents. Below Table 6 discussed the storage stability of encapsulated pomegranate peel bioactives stored for a number of days at different temperature. In their study, Hady et al. (2022)97 effectively employed a double emulsion method with carboxymethyl cellulose to encapsulate pomegranate peel extract, resulting in high encapsulation rates of pomegranate polyphenols surpassing 95%. The results showed enhanced encapsulation stability of emulsions containing carboxymethyl cellulose during storage durations. In their study, Akhavan Mahdavi et al.104 studied how long encapsulated pigments would last in storage at three different temperatures (25, 35, and 42 °C), four different levels of relative humidity (20%, 30%, 40%, and 50%), and up to ninety days of light exposure. Comparing enclosed pigments with non-encapsulated anthocyanins, it was discovered that the enclosed pigments had a much longer shelf life in all wall materials during storage. The choice of wall material type affected the anthocyanin-containing barberry's stability and effectiveness during storage. Among all the wall materials examined in this study, the combination of maltodextrin and arabic gum showed the highest anthocyanin stability under all assessed conditions, offering the best protection for the anthocyanin pigments. In a 42-day storage study, Azarpazhooh et al.18 examined the reliability and half-life of enclosed pomegranate peel powders at 4 °C and 25 °C with relative humidity values of 52% and 75%. The degradation of anthocyanins was shown to follow first-order kinetics in storage studies. After 42 days at 4 °C, the total anthocyanin amount of the microencapsulated powders made from pomegranate peel extract with a 15% wall coating and the control fell to 18% and 33%, respectively. These decreases reached 24% and 38% at 25 °C, respectively. The powder that had been freeze-dried and encapsulated with 15% MDX was found to have the greatest anthocyanin concentration. It was kept at 4 °C and 75% relative humidity, with an average half-life of 115 days with a reaction rate constant (k) of 0.64 × 10−2 min−1. According to Moser et al.105 flavanols are unable to form copigment structures with anthocyanins because of the competitive stabilising impact of maltodextrin and the existence of aromatic amino compound residues, which can also function as copigments. The researchers evaluated the stability of storage of phenolic substances in enclosed red grape concentrate using various combinations of soy protein as well as whey protein with maltodextrin. Different anthocyanidins responded differently to temperature elevation; cyanidins were more vulnerable to heat degradation than delphinidin.
Table 6 Experimentations of storage stability of encapsulated pomegranate peel extract bioactive
Storage temperature (°C) |
Storage days |
Results and discussion |
References |
4 and 25 °C |
42 days |
• Anthocyanin degradation followed predictable patterns over 42 days |
18
|
• Pomegranate peel extract-microencapsulated powders: lost 18% anthocyanin at 4 °C, 24% at 25 °C |
• Microencapsulation: improved anthocyanin stability compared to controls |
4 °C |
14 days |
• PPE encapsulated in alginate nanospheres showed increased antibacterial activity |
40
|
• This novel coating improved microbiological stability of chicken flesh, especially during long-term storage |
For high moisture contents – below −33.4 °C |
180 days |
• Antioxidant activity of extracts with higher moisture content remains maintained at temperatures below 33.4 °C |
5
|
For dried extracts – ≥ 1.2 °C |
• Completely dried extracts can preserve functional properties of pomegranate peel extract at temperatures as low as 1.2 °C |
60 °C |
45 days |
• Encapsulated and unencapsulated extracts showed minimal reduction in phenolic content (0.56%) after 45 days of storage at 60 °C |
16
|
• Antioxidative capacity remained above 84% after storage |
Table 7 Comparison of the advantages and disadvantages of different encapsulation techniques for encapsulated pomegranate peel extract
Encapsulation method |
Advantages |
Disadvantages |
References |
Spray drying |
An economical and adaptable technique that yields superior-quality and long-lasting particles |
High temperatures during the procedure cause certain delicate bioactive elements to evaporate. It impacts product integrity, especially for heat-sensitive substances |
101
|
Double emulsion |
The multi-compartment structure of the system controls the outward release of bioactive chemicals. Additionally, it shields sensitive bioactive compounds from degradation and successfully hides any undesirable sensory characteristics |
It's difficult to stabilize double emulsions for extended product life. Loss of the inner-phase material is frequently caused by instability, particularly in production. The huge interfacial area of double emulsions makes their creation difficult |
62
|
Co-crystallization |
Phenolic compounds are given a solid-state structure via co-crystals, which improves their chemical stability and shields them from oxidation and damage. Carefully forming co-crystals can improve bioavailability, solubility, and dissolution rates. This procedure further enhances the durability and usefulness of phenolic encapsulates by permitting the addition of other excipients, such as organic polymers or surfactants |
Attaining the desired co-crystal formation often necessitates careful control of multiple variables, such as temperature, solvent composition, and stoichiometry, which can pose challenges and require significant time investment. The applicability of this method may be limited for specific compounds, as not all active ingredients readily undergo co-crystallization |
16
|
Ionic gelation |
It facilitates precise control over the release rates of encapsulated substances, allowing for targeted delivery and prolonged therapeutic effects. Its adaptability and scalability make it suitable for large-scale manufacturing across various sectors such as pharmaceuticals, cosmetics, and biotechnology |
It might show inconsistency across batches due to its sensitivity to variables such as temperature and pH, potentially affecting the uniformity of particle sizes and, consequently, the overall product quality. Some substances may not be compatible with the gelation agents used in the process, limiting their effectiveness for encapsulation via ionic gelation |
42
|
Table 8 Comparison of encapsulation techniques
Encapsulation techniques |
Encapsulation efficiency |
Cost |
Scalability |
Reference |
Spray drying |
88.68% |
Moderate |
Used frequently on a large scale |
24
|
Freeze drying |
95.7% |
High |
Not used frequently on a large scale |
75
|
Ionic gelation |
95.76% |
Low |
Not used frequently on a large scale |
102
|
Coacervation |
85.88% |
High |
Not used frequently on a large scale |
103
|
9. Challenges faced during encapsulation of PPE
The bioactive extracted from pomegranate peel displays a tendency towards instability, making it prone to various chemical reactions such as oxidation, polymerization, and condensation. The presence of phenolic compounds within the extract makes it particularly vulnerable to degradation when exposed to external elements such as elevated temperatures, oxygen, and light throughout the stages of processing and storage. Their sensitivity to gastrointestinal conditions further limits their effectiveness and diminishes their potential health benefits. When pomegranate peel phenolics are added to food products, they may impart an astringent flavor.97 Sanhueza et al.62 succeeded in encapsulating pomegranate peel extract effectively by utilizing double emulsions. However, they encountered challenges concerning the stability control of these double emulsions (DEs) concerning the product's shelf life. This variation in stability often results in the early shedding of a substantial portion of the inner phase, often starting as early as the double emulsion production stage. Typically, achieving stable DE formulations poses difficulties due to their expansive interfacial area, necessitating the utilization of a minimum of two types of stabilizers. Kaderides et al.101 encapsulated the extract of pomegranate peel utilizing spray drying. Spray drying demands considerable energy usage, predominantly for heating and evaporating the liquid feed, potentially leading to increased operational costs. When high temperatures are used during the spray drying process, sensitive bioactive components evaporate, thereby impacting the overall integrity of the product, especially concerning heat-sensitive substances. There is a chance of losing the product during spray drying, as fine particles or fines may form, making it challenging to collect them efficiently and ultimately reducing the overall yield.
10. Future perspectives toward the use of encapsulated PPE bioactives
In recent times, an increasing need has been observed for natural additives such as antioxidants and preservatives, which are being pursued as alternatives to traditional choices associated with possible harmful effects. The interest of consumers in functional foods has grown rapidly, perceived to fortify the immune system and bolster resistance against viruses. This heightened interest has been largely driven by the COVID-19 pandemic and is anticipated to persist in the foreseeable future. Increasing demands have sparked new possibilities for creating a wide range of innovative functional foods. This trend also facilitates the utilization of valuable ingredients extracted from by-products and waste materials. Within this framework, the emergence of novel functional commodities integrating immune-enhancing components obtained from extracts of pomegranate peel shows potential in diminishing wastage produced from residual pomegranate materials and promoting ongoing creativity.61
11. Applications of encapsulated extracts of pomegranate peel in food matrices
The encapsulated extract derived from the peel of the pomegranate fruit exhibits promising attributes to function as an innovative and organic supplement, enriching the operational characteristics and prolonging the durability of food products. The initiation of lipid oxidation sets off a series of chemical reactions that have the potential to alter the physicochemical properties, sensory characteristics (such as aroma, appearance, and taste), and longevity of meat-based products. There has been notable interest within the scientific community regarding the effectiveness of plant polyphenols as powerful natural antioxidants for different meat products. The application of plant polyphenol extracts in these goods leads to positive results by efficiently hindering both lipid oxidation and microbial decay, thereby extending their shelf life.61 The efficacy of pomegranate peel extract against Salmonella Kentucky in chicken meat was examined in a recent study by Wafa et al.106 During a 21-day period of refrigeration at 4 °C, the administration of the extract at doses of 0.8 and 1.6 mg g−1 in chicken breast led to a reduction in the rate of Salmonella growth. Nevertheless, it is important to highlight that the incorporation of the extract led to a modification in the color of the chicken meat, transitioning from a pink hue to a yellow shade. In the experiment conducted by Turgut et al.,107 following enhancement with 0.5% and 1.0% aqueous PPE, beef meatballs were contrasted with control meatballs and meatballs fortified with 0.01% BHT. It was observed that both PPE and BHT-enriched meatballs demonstrated notably lower peroxide values than the control meatballs throughout the storage period. The meatballs containing 1% PPE exhibited the least increment in the peroxide value, followed by those with BHT and 0.5% peel extract. A similar trend was noted for thiobarbituric acid-reactive substances (TBARSs), with the order being 1% extract < BHT < 0.5% extract < control. Also the investigation revealed that the incorporation of antioxidants led to a reduction in protein oxidation within the beef meatballs. At the end of an 8-day storage duration, carbonyl levels were measured at 0.91, 0.67, 0.80, and 0.68 nmol mg−1 protein for the control, BHT, 0.5% PPE, and 1% PPE samples, respectively. Importantly, it was found that the sensory attributes of the meatballs remained unaffected by the presence of pomegranate peel extract.
Various fish products can become contaminated by bacteria, thus posing a potential risk for foodborne illnesses and even fatalities. An investigation into the efficacy of encapsulated or nonencapsulated PPEs in preventing the proliferation of bacterial pathogens in fish products could play a crucial role in prolonging their shelf life.61 Basiri et al.108 examined the effects of 1–2% methanolic PPE and vacuum packaging on the quality and shelf life of Pacific white shrimp stored for 10 days at 4 °C. They measured plate counts, bacterial levels, trimethylamine, pH, TBARS, and melanosis. The sensory evaluation revealed that the shrimps treated with PPE exhibited improved color, odor, texture, and overall similarity scores, without causing any adverse effects on overall acceptability. According to the study's findings, PPE and vacuum packing served as antimicrobial agents to increase the shelf life and quality of shrimp. Zhuang et al.109 examined the impact of ethanolic and aqueous PPE on the microbiota composition and quality of bighead carp (Aristichthys nobilis) fillets while they were stored at 4 °C. The outcomes demonstrated that ethanolic PPE was effective in delaying spoilage microorganism growth (Pseudomonas, Aeromonas, and Shewanella) and avoiding fillet odor and texture deterioration. It also extended shelf life by two days. It also impeded the breakdown of molecules associated with ATP and the production of biogenic amines. Conversely, aqueous PPE exhibited shortcomings with regard to color evaluation and overall acceptability in the sensory assessment conducted by expert evaluators. The researchers indicated that PPE might succeed as a preservative for chilled bighead carp fillets, underscoring the importance of enhancing color retention.
The review discusses current advances in the encapsulation of pomegranate peel extract (PPE), with an emphasis on sustainable wall materials and their applications in the food industry. Critical industry standards, such as FDA and EFSA guidelines, stress the safety and efficacy of bioactive chemicals in capsules. These guidelines set acceptable limits for components such as antioxidants and antimicrobials, assuring food safety. Encapsulation technologies such as spray drying, freeze drying, and nanotechnology improve the stability and bioavailability of PPE, prolonging its shelf life. Practical applications in the food business are explored, with a particular emphasis on sustainable packaging and waste reduction measures.
The FDA prioritizes the Generally Recognized As Safe (GRAS) status of bioactive components such as those found in pomegranate peel extract (PPE) to ensure that the compounds utilized in food items do not cause harm. Furthermore, FDA laws limit the types and quantities of wall materials (such as starches, proteins, or polysaccharides) that can be used as encapsulants, ensuring that they are non-toxic and safe for food contact. To prevent negative effects on human health, the review must take into account the safety, bioactivity, and migratory potential of encapsulating materials. The EFSA regulates active and intelligent packaging materials, improving food safety by limiting the release of active chemicals such as antioxidants from PPE. They establish allowable limits for bioactive substances introduced into food to minimize harm. The EFSA also requires the use of materials that preserve the functional integrity of bioactive chemicals while promoting environmental sustainability, such as biodegradable encapsulants. To avoid contamination and for consumer safety, the wall materials used must adhere to these restrictions. Both regulatory agencies underline the importance of careful characterization and testing of encapsulated goods to ensure they meet food safety standards.
Phenolics from PPEs have an impact on microbial development, antioxidant qualities, gel resilience, interaction, amount of moisture, total pH level, rheological attributes, and sensory aspects of dairy products.61 Çam et al.98 examined the possibility of enhancing the functional qualities of conventional ice cream with pomegranate peel microencapsulated phenolics. When 0.5% and 1.0% (weight/weight) pomegranate peel phenolics were added to enhanced ice creams, their antioxidants and α-glucosidase inhibitory activity were significantly increased in comparison to the control sample. In an investigation by Trigo et al.110 carrot juice that had been heated, high-pressured, and left untreated was mixed with 2.5 mg mL−1 of dried pomegranate peel extract (PPE). In comparison to the control samples, the enriched juices showed lower mesophile and psychrophile counts, as well as larger amounts of total phenol and hydrolysable tannin concentrations and antioxidant activity. Crucially, the flavor and texture of the juices remained unaffected by the incorporation of the extract.
12. Conclusion
In conclusion, this comprehensive review has efficiently examined various encapsulation techniques and wall materials employed in the encapsulation of pomegranate peel extract. Detailed discussions on the physicochemical properties of pomegranate peel extract have provided a solid foundation for understanding its encapsulation process. Through an investigation of techniques such as spray drying, freeze drying, ionic gelation, coacervation, electrospraying, electrospinning, and co-extrusion, the review explained the production of diverse encapsulate materials, each with its own set of advantages and drawbacks. The pivotal role of wall materials in facilitating controlled release mechanisms has been underscored, with combinations of these materials yielding improved outcomes. In addition, the review looked into factors influencing the stability and release profile of encapsulated compounds, addressing challenges such as instability and degradation when exposed to environmental factors. By offering insights into these key aspects, the review lays the groundwork for further advancements in optimizing encapsulation strategies for enhanced delivery and efficacy of pomegranate-derived bioactive compounds.
Funding
No funding was obtained for this study.
Author contributions
RPB: conceptualization, data curation, original draft writing. RKT: supervision, resources. KKD: validation, visualization, writing – review & editing. MS: conceptualization, project administration, supervision, writing – review & editing.
Conflicts of interest
The authors have declared no conflict of interest in this work. The authors also declare that they have no known competing financial interests or personal relationships that could have appeared to influence the work reported in this paper.
References
-
C. Venkitasamy, L. Zhao, R. Zhang and Z. Pan, in Integrated Processing Technologies for Food and Agricultural By-Products, Elsevier, 2019, pp. 181–216 Search PubMed.
- P. Sharma, S. F. McClees and F. Afaq, Molecules, 2017, 22, 1–18 CrossRef.
- K. Kandemir, E. Piskin, J. Xiao, M. Tomas and E. Capanoglu, J. Agric. Food Chem., 2022, 70, 6805–6832 CrossRef CAS PubMed.
- Y. Mo, J. Ma, W. Gao, L. Zhang, J. Li, J. Li and J. Zang, Front. Nutr., 2022, 9, 1–9 Search PubMed.
- S. Akhtar, T. Ismail, D. Fraternale and P. Sestili, Food Chem., 2015, 174, 417–425 CrossRef CAS PubMed.
- V. Đorđević, B. Balanč, A. Belščak-Cvitanović, S. Lević, K. Trifković, A. Kalušević, I. Kostić, D. Komes, B. Bugarski and V. Nedović, Trends in Encapsulation Technologies for Delivery of Food Bioactive Compounds, Food Eng. Rev., 2014, 7, 452–490 CrossRef.
- M. S. Culas, D. G. Popovich and A. Rashidinejad, Food Biosci., 2024, 57, 103470 CrossRef CAS.
- O. P. Bamidele and M. N. Emmambux, Crit. Rev. Food Sci. Nutr., 2021, 61, 3100–3118 CrossRef.
-
J. Pandit, M. Aqil and Y. Sultana, in Encapsulations, Elsevier, 2016, pp. 597–640 Search PubMed.
-
T. J. Gutiérrez Carmona, Polymers for food applications, 2018 Search PubMed.
- S. Ghosh, T. Sarkar, A. Das and R. Chakraborty, Lwt, 2022, 153, 112527 CrossRef CAS.
- I. Alemzadeh, M. Hajiabbas, H. Pakzad, S. Sajadi Dehkordi and A. Vossoughi, Int. J. Eng., Trans. A, 2020, 33, 1–11 Search PubMed.
- V. Marcillo-Parra, D. S. Tupuna-Yerovi, Z. González and J. Ruales, Trends Food Sci. Technol., 2021, 116, 11–23 CrossRef CAS.
- S. Roy and J. W. Rhim, Int. J. Biol. Macromol., 2021, 193, 2038–2046 CrossRef CAS.
-
L. G. Gómez-Mascaraque, Development and characterization of microencapsulation systems for bioactive ingredients of interest in the development of functional foods, 2017 Search PubMed.
- E. Chezanoglou and A. M. Goula, Appl. Sci., 2023 DOI:10.3390/app13158680.
- P. Robert and C. Fredes, Molecules, 2015, 20, 5875–5888 CrossRef CAS.
- E. Azarpazhooh, P. Sharayei, S. Zomorodi and H. S. Ramaswamy, Food Bioprocess Technol., 2019, 12, 199–210 CrossRef CAS.
- B. Oudane, D. Boudemagh, M. Bounekhel, W. Sobhi, M. Vidal and S. Broussy, J. Mol. Struct., 2017 DOI:10.1016/j.molstruc.2017.11.129.
- W.-Z. Liang, C.-T. Chou, J.-S. Cheng, J.-L. Wang, H.-T. Chang, I.-S. Chen, T. Lu, J.-H. Yeh, D.-H. Kuo and P. Shieh, Eur. J. Pharmacol., 2016, 780, 243–251 CrossRef CAS PubMed.
- W. R. García-Niño and C. Zazueta, Pharmacol. Res., 2015, 97, 84–103 CrossRef.
- J. Gao, J. Hu, D. Hu and X. Yang, Nat. Prod. Commun., 2019 DOI:10.1177/1934578X19874174.
- A. N. Panche, A. D. Diwan and S. R. Chandra, J. Nutr. Sci., 2016 DOI:10.1017/jns.2016.41.
- K. Šavikin, N. Nastić, T. Janković, D. Bigović, B. Miličević, S. Vidović, N. Menković and J. Vladić, Foods, 2021 DOI:10.3390/foods10091968.
- C. Bao, P. Jiang, J. Chai, Y. Jiang, D. Li, W. Bao, B. Liu, B. Liu, W. Norde and Y. Li, Food Res. Int., 2019, 120, 130–140 CrossRef CAS.
- L. de Souza Simões, D. A. Madalena, A. C. Pinheiro, J. A. Teixeira, A. A. Vicente and Ó. L. Ramos, Adv. Colloid Interface Sci., 2017, 243, 23–45 CrossRef.
- M. R. I. Shishir, L. Xie, C. Sun, X. Zheng and W. Chen, Trends Food Sci. Technol., 2018, 78, 34–60 CrossRef CAS.
- J. Xue and Y. Luo, ES Food Agrofor., 2021, 5, 1–3 Search PubMed.
- A. Rehman, S. M. Jafari, Q. Tong, T. Riaz, E. Assadpour, R. M. Aadil, S. Niazi, I. M. Khan, Q. Shehzad and A. Ali, Adv. Colloid Interface Sci., 2020, 284, 102251 CrossRef CAS.
- M. Tarhini, H. Greige-Gerges and A. Elaissari, Int. J. Pharm., 2017, 522, 172–197 CrossRef CAS.
- J. Singh, H. P. Kaur, A. Verma, A. S. Chahal, K. Jajoria, P. Rasane, S. Kaur, J. Kaur, M. Gunjal and S. Ercisli, ACS Omega, 2023, 8, 35452–35469 CrossRef CAS PubMed.
- H. B. U. Ain, T. Tufail, S. Bashir, N. Ijaz, M. Hussain, A. Ikram, M. A. Farooq and S. A. Saewan, Food Sci. Nutr., 2023, 11, 2589–2598 CrossRef CAS PubMed.
- F. Sultana, S. Akther and M. Rasheda Begum, Ukr. J. Food Sci., 2020, 8, 251–259 CrossRef CAS.
- E. Azarpazhooh, P. Sharayei, S. Zomorodi and H. S. Ramaswamy, Food Bioprocess Technol., 2019, 12, 199–210 CrossRef CAS.
- A. T. Mazhitova, A. M. Kasymakunova and N. Turker, Int. J. Food Eng., 2022, 18, 537–545 CrossRef CAS.
- C. Feng, M. Zhang, B. Bhandari, Y. Wang and B. Wang, J. Food Process Eng., 2021, 44, e13583 CrossRef CAS.
- J. Comaposada, P. Gou, B. Marcos and J. Arnau, Lwt, 2015, 64, 212–219 CrossRef CAS.
- W. Zam, G. Bashour, W. Abdelwahed and W. Khayata, Braz. J. Pharm. Sci., 2014, 50, 741–748 CrossRef CAS.
- M. Milivojević, A. Popović, I. Pajić-Lijaković, I. Šoštarić, S. Kolašinac and Z. D. Stevanović, Gels, 2023 DOI:10.3390/gels9080620.
- P. Rahnemoon, M. Sarabi-Jamab, A. Bostan and E. Mansouri, Food Biosci., 2021, 43, 101331 CrossRef CAS.
-
S. Vijeth, G. B. Heggannavar and M. Y. Kariduraganavar, Microencapsulation – Processes, Technologies and Industrial Applications, 2019, pp. 1–19 Search PubMed.
- M. Soltanzadeh, S. H. Peighambardoust, B. Ghanbarzadeh, M. Mohammadi and J. M. Lorenzo, Nanomaterials, 2021 DOI:10.3390/nano11061439.
- B. Nanda, A. S. Manjappa, K. Chuttani, N. H. Balasinor, A. K. Mishra and R. S. Ramachandra Murthy, Int. J. Biol. Macromol., 2019, 122, 367–379 CrossRef CAS PubMed.
- R. Ramos, J. Bernard, F. Ganachaud and A. Miserez, Small Sci., 2022 DOI:10.1002/smsc.202100095.
- M. Hadidi, C. Tan, E. Assadpour, M. S. Kharazmi and S. M. Jafari, J. Controlled Release, 2023, 355, 327–342 CrossRef CAS PubMed.
- W. C. Blocher McTigue and S. L. Perry, Small, 2020 DOI:10.1002/smll.201907671.
- K. Sridhar, S. Bouhallab, T. Croguennec, D. Renard and V. Lechevalier, Trends Food Sci. Technol., 2022, 127, 49–62 CrossRef CAS.
- N. Shakoury, M. A. Aliyari, M. Salami, Z. Emam-Djomeh, B. Vardhanabhuti and A. A. Moosavi-Movahedi, Lwt, 2022, 158, 113138 CrossRef CAS.
- P. R. Souza, A. C. de Oliveira, B. H. Vilsinski, M. J. Kipper and A. F. Martins, Pharmaceutics, 2021 DOI:10.3390/pharmaceutics13050621.
- K. Kuperkar, L. I. Atanase, A. Bahadur, I. C. Crivei and P. Bahadur, Polymers, 2024, 16, 206 CrossRef CAS PubMed.
- Y. Meng, C. Qiu, X. Li, D. J. McClements, S. Sang, A. Jiao and Z. Jin, Crit. Rev. Food Sci. Nutr., 2024, 64, 187–201 CrossRef CAS PubMed.
- P. P. dos Santos, S. H. Flôres, A. de Oliveira Rios and R. C. Chisté, Trends Food Sci. Technol., 2016, 53, 23–33 CrossRef CAS.
-
H. Kwak, Nano-and Microencapsulation for Foods, 2014, pp. 1–14 Search PubMed.
- P. P. S. Coimbra, F. de, S. N. Cardoso and É. C. B. de A. Gonçalves, Crit. Rev. Food Sci. Nutr., 2021, 61, 2809–2826 CrossRef CAS.
- J. D. Hoyos-Leyva, L. A. Bello-Pérez, J. Alvarez-Ramirez and H. S. Garcia, Food Rev. Int., 2018, 34, 148–161 CrossRef CAS.
- P. Gullón, G. Astray, B. Gullón, I. Tomasevic and J. M. Lorenzo, Molecules, 2020, 25, 2859 CrossRef.
- A. N. Dundar, K. Uzuner, M. E. Parlak, O. I. Sahin, F. T. Saricaoglu and S. Simsek, Foods, 2022, 11, 3785 CrossRef CAS.
- E. Chezanoglou and A. M. Goula, Appl. Sci., 2023, 13, 8680 CrossRef CAS.
- V. Velazquez-Martinez, D. Valles-Rosales, L. Rodriguez-Uribe, O. Holguin, J. Quintero-Quiroz, D. Reyes-Jaquez, M. I. Rodriguez-Borbon, L. Y. Villagrán-Villegas and E. Delgado, Foods, 2021, 10, 116 CrossRef CAS PubMed.
- A. F. Esfanjani, E. Assadpour and S. M. Jafari, Trends Food Sci. Technol., 2018, 76, 56–66 CrossRef.
- K. Kaderides, A. Kyriakoudi, I. Mourtzinos and A. M. Goula, Trends Food Sci. Technol., 2021, 115, 380–390 CrossRef CAS.
- L. Sanhueza, P. García, B. Giménez, J. M. Benito, M. Matos and G. Gutiérrez, Foods, 2022 DOI:10.3390/foods11030310.
- F. Goodarzi and S. Zendehboudi, Can. J. Chem. Eng., 2019, 97, 281–309 CrossRef CAS.
- C. Tan and D. J. McClements, Foods, 2021, 10, 812 CrossRef CAS.
- L. Salvia-Trujillo and D. J. McClements, Food Biophys., 2016, 11, 1–9 CrossRef.
- C. Tan and D. J. McClements, Foods, 2021 DOI:10.3390/foods10040812.
- S. Ganjian, R. Javadian and M. Keshavarz, Iran. J. Fish. Sci., 2020, 19, 994–1005 Search PubMed.
- A. N. Dundar, S. Ozdemir, K. Uzuner, M. E. Parlak, O. I. Sahin, A. F. Dagdelen and F. T. Saricaoglu, Food Chem., 2023, 398, 133921 CrossRef PubMed.
- N. Zahed, R. Esmaeilzadeh Kenari and R. Farahmandfar, Food Sci. Nutr., 2023, 1–8 Search PubMed.
- K. Kaderides, A. M. Goula and K. G. Adamopoulos, Innovative Food Sci. Emerging Technol., 2015, 31, 204–215 CrossRef CAS.
- P. Sharayei, E. Azarpazhooh and H. S. Ramaswamy, J. Food Sci. Technol., 2020, 57, 723–733 CrossRef CAS.
- N. Yağmur and S. Şahin, J. Food Sci., 2020, 85, 998–1006 CrossRef.
- Q. Hu, X. Li, F. Chen, R. Wan, C. Yu, J. Li, D. J. McClements and Z. Deng, J. Food Process. Preserv., 2020, 44, e14805 CAS.
- A. Rezvankhah, Z. Emam-Djomeh and G. Askari, Drying Technol., 2020, 38, 235–258 CrossRef CAS.
- Q. D. Nguyen, T. T. Dang, T. V. L. Nguyen, T. T. D. Nguyen and N. N. Nguyen, Int. J. Food Prop., 2022, 25, 359–374 CrossRef CAS.
- S. Ray, U. Raychaudhuri and R. Chakraborty, Food Biosci., 2016, 13, 76–83 CrossRef CAS.
- L. E. Kurozawa and M. D. Hubinger, Curr. Opin. Food Sci., 2017, 15, 50–55 CrossRef.
- S. C. S. R. de Moura, C. L. Berling, S. P. M. Germer, I. D. Alvim and M. D. Hubinger, Food Chem., 2018, 241, 317–327 CrossRef CAS.
- M. Pereira Silveira, F. Lucas Chaves Almeida, I. Dutra Alvim and A. Silvia Prata, Food Res. Int., 2023, 174, 113590 CrossRef CAS.
- C. Bennacef, S. Desobry-Banon, L. Probst and S. Desobry, Mar. Drugs, 2023 DOI:10.3390/md21040235.
- S. Castro Coelho, B. Nogueiro Estevinho and F. Rocha, Food Chem., 2021, 339, 127850 CrossRef CAS.
- P. Wen, M. H. Zong, R. J. Linhardt, K. Feng and H. Wu, Trends Food Sci. Technol., 2017, 70, 56–68 CrossRef CAS.
- P. Wang, M. Ding, T. Zhang, T. Wu, R. Qiao, F. Zhang, X. Wang and J. Zhong, Food Rev. Int., 2022, 38, 566–588 CrossRef.
- D. Raval, J. Kabariya, T. Hazra and V. Ramani, Int. J. Chem. Stud., 2019, 51, 227–240 Search PubMed.
- Y. P. Timilsena, T. O. Akanbi, N. Khalid, B. Adhikari and C. J. Barrow, Int. J. Biol. Macromol., 2019, 121, 1276–1286 CrossRef CAS.
- M. Jafarian, P. Taghinia, S. Baeghbali and N. Samadi Ghorbani Dozdozani, J. Food Meas. Charact., 2021, 15, 4992–4999 CrossRef.
-
B. Wang, Coacervation technique as an encapsulation and delivery tool for hydrophobic biofunctional compounds, Role of Materials Science in Food Bioengineering, 2018 Search PubMed.
- N. Devi, M. Sarmah, B. Khatun and T. K. Maji, Adv. Colloid Interface Sci., 2017, 239, 136–145 CrossRef CAS.
- U. Baysan, F. Elmas and M. Koç, J. Food Process Eng., 2019, 42, 1–11 Search PubMed.
- N. A. Razak, N. A. Hamid and A. R. Shaari, AIP Conf. Proc., 2018 DOI:10.1063/1.5066835.
- N. Mohd Nawi, I. I. Muhamad and A. Mohd Marsin, Food Sci. Nutr., 2015, 3, 91–99 CrossRef CAS PubMed.
- K. Kaderides, I. Mourtzinos and A. M. Goula, Food Chem., 2020, 310, 125849 CrossRef CAS.
- J. Guo, M. M. Giusti and G. Kaletunç, Food Res. Int., 2018, 107, 414–422 CrossRef CAS.
- M. Homayoonfal, S. M. Mousavi, H. Kiani, G. Askari, S. Desobry and E. Arab-Tehrany, Foods, 2021 DOI:10.3390/foods10030492.
- A. M. Szczotok, I. Garrido, M. Carmona, A. L. Kjøniksen and J. F. Rodriguez, Colloids Surf., A, 2018, 554, 49–59 CrossRef CAS.
- C. Zhong, Y. Zu, X. Zhao, Y. Li, Y. Ge, W. Wu, Y. Zhang, Y. Li and D. Guo, Int. J. Food Sci. Technol., 2016, 51, 212–221 CrossRef CAS.
- E. Hady, M. Youssef, A. H. Aljahani, H. Aljumayi, K. A. Ismail, E. S. El-Damaty, R. Sami and G. El-Sharnouby, Crystals, 2022 DOI:10.3390/cryst12050622.
- M. Çam, N. C. Içyer and F. Erdoǧan, Lwt, 2014, 55, 117–123 CrossRef.
- I. M. Martins, M. F. Barreiro, M. Coelho and A. E. Rodrigues, Chem. Eng. J., 2014, 245, 191–200 CrossRef CAS.
- H. Andishmand, M. Yousefi, N. Jafari, S. Azadmard-Damirchi, A. Homayouni-Rad, M. Torbati and H. Hamishehkar, Int. J. Biol. Macromol., 2024, 256, 128501 CrossRef CAS PubMed.
- K. Kaderides and A. M. Goula, J. Food Eng., 2019, 253, 1–13 CrossRef CAS.
- M. Sharma, K. K. Dash and L. S. Badwaik, Int. J. Biol. Macromol., 2022, 194, 715–725 CrossRef CAS PubMed.
- Y. Liu, H. Wang, R. Fu, L. Zhang, M. Liu, W. Cao, R. Wu and S. Wang, J. Food Meas. Charact., 2023, 17, 664–673 CrossRef.
- S. Akhavan Mahdavi, S. M. Jafari, E. Assadpour and M. Ghorbani, J. Food Eng., 2016, 181, 59–66 CrossRef CAS.
- P. Moser, V. R. N. Telis, N. de Andrade Neves, E. García-Romero, S. Gómez-Alonso and I. Hermosín-Gutiérrez, Food Chem., 2017, 214, 308–318 CrossRef CAS PubMed.
- B. A. Wafa, M. Makni, S. Ammar, L. Khannous, A. Ben Hassana, M. Bouaziz, N. E. Es-Safi and R. Gdoura, Int. J. Food Microbiol., 2017, 241, 123–131 CrossRef CAS PubMed.
- S. S. Turgut, A. Soyer and F. Işıkçı, Meat Sci., 2016, 116, 126–132 CrossRef CAS.
- S. Basiri, S. S. Shekarforoush, M. Aminlari and S. Akbari, Lwt, 2015, 60, 1025–1033 CrossRef CAS.
- S. Zhuang, Y. Li, S. Jia, H. Hong, Y. Liu and Y. Luo, Food Microbiol., 2019, 82, 445–454 CrossRef CAS PubMed.
- J. P. Trigo, E. M. C. Alexandre, A. Oliveira, J. A. Saraiva and M. Pintado, Int. J. Food Sci. Technol., 2020, 55, 1599–1605 CrossRef CAS.
|
This journal is © The Royal Society of Chemistry 2025 |
Click here to see how this site uses Cookies. View our privacy policy here.