Investigating the impact of climate change on PCB-153 exposure in Arctic seabirds with the nested exposure model†
Received
30th September 2024
, Accepted 1st April 2025
First published on 10th April 2025
Abstract
At the same time Arctic ecosystems experiences rapid climate change, at a rate four times faster than the global average, they remain burdened by long-range transported pollution, notably with legacy polychlorinated biphenyls (PCBs). The present study investigates the potential impact of climate change on seabird exposure to PCB-153 using the established Nested Exposure Model (NEM), here expanded with three seabird species, i.e. common eider (Somateria mollissima), black-legged kittiwake (Rissa tridactyla) and glaucous gull (Larus hyperboreus), as well as the filter feeder blue mussel (Mytulis edulis). The model's performance was evaluated using empirical time trends of the seabird species in Kongsfjorden, Svalbard, and using tissue concentrations from filter feeders along the northern Norwegian coast. NEM successfully replicated empirical PCB-153 concentrations, confirming its ability to simulate PCB-153 bioaccumulation in the studied seabird species within an order of magnitude. Based on global PCB-153 emission estimates, simulations run until the year 2100 predicted seabird blood concentrations 99% lower than in year 2000. Model scenarios with climate change-induced altered dietary composition and lipid dynamics showed to have minimal impact on future PCB-153 exposure, compared to temporal changes in primary emissions of PCB-153. The present study suggests the potential of mechanistic modelling in assessing POP exposure in Arctic seabirds within a multiple stressor context.
Environmental significance
We have expanded, evaluated, and applied the Nested Exposure Model (NEM) to assess the bioaccumulation of PCB-153 in three Arctic seabirds (common eider, black-legged kittiwake, glaucous gull) and a filter feeder (blue mussel). This application demonstrates the utility of NEM in predicting contaminant exposure under a multiple stressor framework, including both primary emissions and climate change-induced changes in ecosystem structure and reproductive investment. The model's ability to simulate bioaccumulation provides opportunities for (i) predicting long-term contaminant exposure trends in Arctic species; (ii) evaluating the relative influence of climate-induced dietary changes and lipid dynamics during egg incubation versus emission reductions on future pollutant exposure; and (iii) supporting regulatory and conservation efforts in mitigating risks to vulnerable Arctic ecosystems from persistent organic pollutants (POPs).
|
1 Introduction
Climate change is happening at a rapid pace in the Arctic at a rate four times faster than the global average,1 which leads to loss of multi-year sea ice and snow cover, glacier retreat, and extreme seasonal weather events.2 Additionally, the Arctic is facing pollution from Persistent Organic Pollutants (POPs),3–5 which undergo long-range transport, are persistent in the environment, bioaccumulate in biota, and pose toxicity risks to wildlife and humans. One historically important group of chemicals among POPs are polychlorinated biphenyls (PCBs), which have been restricted since the late 1970s.6 Yet, they are still present in the Arctic in both the physical environment and in biota.7–9 For instance, PCBs remain quantitatively one of the most abundant groups in the chemical cocktail that Arctic top predators are exposed to, and at concentrations associated with serious health risks.8 The production and use of POPs have been regulated through international agreements, notably the Stockholm Convention,6 leading to declining trends in Arctic biota.4 In recent years the declining trends of some POPs have levelled off or are even reversed.3 Climate change is believed to alter secondary re-emissions of semi-volatile POPs into the environment and ultimately bioavailability to biota.10,11 For instance, higher temperatures may lead to re-volatilization of deposited POPs from secondary sources (such as soil and seawater) to the atmosphere, and melting glaciers, permafrost and sea ice remobilizes stored POPs into freshwater or seawater systems.3,5 Additionally, warmer water masses entering the European Arctic enable Atlantic species to migrate northward,12 affecting the availability of prey for predatory species, and thus changing their dietary composition.13 This may potentially impact accumulation of POPs,11e.g. have climate-induced changes in trophic levels been identified as important drivers for the POP accumulation in Arctic top predators such as polar bears and seabirds.14
In recent years, the Norwegian Arctic has been strongly influenced by the West Spitsbergen Current with increasing temperature, declining sea ice cover and increasing proportion of Atlantic prey species entering fjords.15 These environmental and ecological changes can be expected to alter PCB exposure in seabird populations. Kongsfjorden in Svalbard, Arctic Norway, is a well-known breeding area for several seabird species occupying various ecological niches.16 This includes the benthos feeding sea duck common eider (Somateria mollissima), the small pelagic fish- and crustacean-eating black-legged kittiwake (Rissa tridactyla), and the opportunistic top predator glaucous gull (Larus hyperboreus). Over the last two decades, these species have been under the spotlight of several studies underlying contamination to a complex cocktail of POPs (e.g. PCBs, dichlorodiphenyltrichloroethane (DDT) and hexachlorobenzene (HCB)) and pointing out a wide range of adverse consequences on individual fitness and population dynamics (e.g. ref. 17–27). This makes a relevant case study to investigate the impact of climate change on POPs accumulation in Arctic seabirds.
Fugacity-based models have proven useful to provide a better mechanistic understanding of the fate and behaviour of contaminants in the environment and food webs.28 As summarized in the critical review by Kuo et al.,29 avian bioaccumulation modelling have yet to account for interactions between birds and other biotic and abiotic model compartments and their spatiotemporal variability. When it comes to investigating combined effects of climate change and contaminant loads in seabirds, this has been limited to directly correlating climate variables and measurement data.30 To date, no studies have combined mechanistic modelling with temporal emission estimates to investigate the impact of climate change on contaminant loads in seabirds.
The Nested Exposure Model (NEM), a fugacity-based dynamic and spatially resolved global environmental fate and bioaccumulation model, was introduced in 2021.31 NEM was recently expanded with key species in the Norwegian Arctic marine ecosystem, from lower trophic levels of marine zooplankton and fish, to mammals such as seals and whales.32 Due to its mechanistic nature and extensive utilization of environmental and biological input data,31,32 NEM has a great potential to investigate the influence of alterations in global emissions, the physical environment, or species interactions on PCB-153 dynamics. However, seabirds, which outlined above as a functional group with great potential in unravelling climate change impacts on contaminant exposure, have yet to be included in NEM.
The main objectives in this study were to expand NEM31,32 with seabird species documented to be subjected to climate change: common eider, black-legged kittiwake, and glaucous gull, in addition to one dietary species important for eiders, i.e. the filter feeder blue mussel (Mytilus edulis); secondly, to use empirical time trends of PCB-153 in seabirds in Kongsfjorden to evaluate model simulations building on estimated global emissions; following an investigation of projected contaminant loads in the seabird species in Kongsfjorden up until year 2100; and lastly to examine impacts of selected climate change-driven alterations of diet composition and lipid metabolism on future PCB-153 exposure in the seabirds.
2 Methods
2.1. The Nested Exposure Model (NEM)
The environmental fate module of NEM simulates contaminant partitioning and transport in the physical environment across spatial scales. This allows to target a specific region on the globe by nesting.31 The predicted fugacities in the physical environment are in turn used as input to a bioaccumulation module that simulates contaminant uptake and elimination in an aquatic food web typical for Norwegian Arctic marine areas.32 Hence, NEM enables simulation of the whole continuum of processes linking global emissions of contaminants with ecosystem exposure.
So far, the existing food web of NEM includes three pelagic zooplankton groups with different feeding strategies (amphipods, krill, and copepods), four fish species, i.e. polar cod (Boreogadus saida), capelin (Mallotus villosus), Atlantic herring (Clupea harengus) and Atlantic cod (Gadus morhua), and two marine mammals, i.e. ringed seal (Pusa hispida) and white whale (Delphinapterus leucas). Here, four new species were added to NEM, including the filter feeder blue mussel and three seabird species: common eider, black-legged kittiwake, and glaucous gull. The species are intra-connected through their diet, thus ensuring transfer of contaminants through the trophic levels (Fig. 1). Uptake and elimination of contaminants are calculated from dynamic mass balance equations, except for zooplankton, which are assumed to be in equilibrium with seawater, as described in Krogseth et al.32 The filter feeder module was based on the bentho-pelagic ACC-HUMAN model by Krogseth et al.,33 and the seabird module was based on the extended Arctic ACC-HUMAN model by Binnington et al.34 In NEM, the filter feeder, seabirds and most other organisms are treated as separate compartments, without considering internal distribution (Fig. 12 ESI†). This means that the fractions of lipids and water (and non-lipid organic material in the filter feeder) within each organism is utilized when calculating the fugacity capacities. The only exceptions from this in NEM are marine mammals, where blubber is treated as a separate compartment.32 Marine mammals were not considered in the present study.
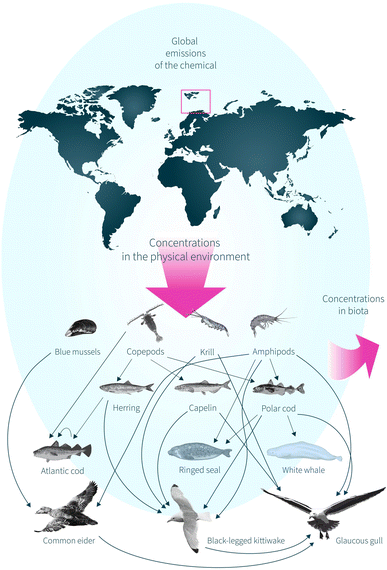 |
| Fig. 1 The Nested Exposure Model (NEM) uses global emissions of the chemical to predict concentrations in the physical environment and biota (pink arrows). The pink square denotes the geographic area of interest in the present study. Species included in NEM (shaded when not applicable in the present study) are shown with arrows illustrating trophic links. Here, NEM was expanded from the established version32 to now also include a filter feeder (blue mussel), and three seabird species with different feeding strategies, i.e. common eider, black-legged kittiwake, and glaucous gull. | |
2.2. Parameterization of new species
The biological parameters implemented in NEM are based on published literature, unpublished data, and extensive field experience. A detailed description of the parameters for the species can be found in the ESI (Sections 1.1–1.5).†
2.2.1. Filter feeder.
In the Norwegian Arctic, blue mussels and other species of mussels represent a large part of the eider's diet,35 and for this reason a filter feeder was included in NEM. The filter feeder was based on a model for a benthic filter feeder in a subarctic lake, which lives partially buried in sediments.33 As eiders feed on mussels living on underwater rocks, the filter feeder here was assumed to only filter seawater and not sediment pore water. In brief, the uptake of contaminants in the filter feeder happens through ingestion and ventilation, and contaminant elimination through metabolism, ventilation, and egestion (Fig. 12 ESI†). These processes are based on life cycle, size, chemical composition, ventilation rate, and dietary composition of the filter feeder. The filter feeder feeds by filtering seawater, and the diet is assumed to consist of 50% suspended particles and 50% phytoplankton.36
2.2.2. Seabirds.
Parameterization of NEM for the targeted seabird species was based on the Canada goose model by Binnington et al.34 While the Canada goose in that study was assumed to solely eat grass, the three new seabird species in NEM feed on various prey species from the Arctic marine food web. In addition, seasonal variation in body mass and lipid content are included into the model, which in turn affect the energy needs and feeding rates throughout the year. Lastly, in NEM, seabirds are assumed to be stationary in Svalbard without any seasonal migration as the model does not yet account for migration.32
In NEM, the modelled seabirds take up contaminants through diet, water, and respiration, and eliminate contaminants through egestion, water excretion, biotransformation, and respiration (Fig. 12 ESI†). Additionally, female seabirds eliminate contaminants through maternal transfer to eggs.37,38 Dietary uptake is regulated by both the dietary composition and the energy needs of the bird. Dietary compositions for each seabird species were assumed to be constant throughout the year, except for the glaucous gull diet, which is assumed to rely on availability of kittiwake and eider eggs and chicks in summer. Energy requirements for all seabird species, ages, seasons and both female and male seabirds in NEM are calculated from the field metabolic rate (FMR), and the lipid energy content is assumed to be constant at 39.3 kJ g−1.39
The eider is a marine duck found in Svalbard during summer and along the coast of Iceland and northern Norway in winter.40 In NEM, the eider's diet is assumed to consist of 80% filter feeders and 20% amphipods35,41 year-round. During reproduction, the female eider experiences large variation in body weight: they feed heavily before nesting and increase their body weight, before starving and drinking minimally during incubation.42 After hatching, they slowly regain their initial body weight. This leads to remobilisation of lipophilic contaminants from their lipid reserves into the blood stream, making the female eiders more susceptible to increased exposure of POPs during incubation.43 In NEM, the female is assumed to fast during incubation, but to maintain water uptake. Only the female incubates, while the male leaves the nesting area after mating, precluding sampling of biological parameters and contaminants from the male. The parameters in NEM are thus only based on measurements from reproducing female eiders.
The kittiwake is a small pelagic surface-feeding gull breeding in colonies on cliffs along the coast. The kittiwake has a circumpolar distribution, and the colonies breeding in Kongsfjorden spend the winter in offshore areas in the South Greenland–Labrador areas.44 Both sexes share incubation and chick-rearing duties, and experience variation in body weight and composition during the reproductive period.45 The parameters are based on both sexes (except for maternal transfer of contaminants, which only affects the female). In NEM, the kittiwakes are assumed to feed on 55% polar cod, 13% capelin, 5% herring, 22% krill, and 5% amphipods,46 constant through the year.
The glaucous gull is a top predator in the Arctic. It has a circumpolar distribution and spends the winters in the north-east areas of the Atlantic Ocean.47 As with kittiwakes, both sexes share the parental investment (incubation and chick-rearing), and the parameters in NEM are based on both females and males. The body mass and composition of the glaucous gull is assumed to be constant through the reproductive period and the rest of the year due to lack of data. In NEM, the glaucous gull's diet is assumed to consist of 28% polar cod, 28% capelin, 22% krill and 22% amphipods,48 constant through the year. An exception for this is made for periods where eggs and chicks of eiders and kittiwakes are available and are assumed to make up 30% of the diet.
2.3. Additional input parameters and model simulations
Except for the newly added species, all other input parameters were kept as previously published.31,32 This includes physicochemical properties for PCB-153 (e.g. partition coefficients), the most recent default global emission scenario for PCB-153,49 and detailed physical and biological parameters of the model.32 Biotransformation of PCB-153 was assumed insignificant in zooplankton and fish,32 and in the filter feeder.33 Biotransformation rates and faeces-blood partition coefficients of PCB-153 of higher-trophic level organisms were utilized as described in Binnington and Wania.50 This gave biotransformation rate constants of 1.36 × 10−5 h−1, 2.05 × 10−5 h−1 and 1.42 × 10−5 h−1 for eiders, kittiwakes, and glaucous gulls, respectively. Additionally, chemical specific mother-egg ratios for PCB-153 of 0.48 were assumed for all seabird species, adopted from the mother-egg ratio from herring gulls (Larus argentatus).34
NEM was run for the global physical environment with a spatial resolution of 5° × 5° latitude/longitude.31,32 Predicted fugacities in lower air and dissolved phase seawater was used as input to the bioaccumulation module run for the Norwegian Arctic covering the coast of northern Norway and Svalbard (65–80°N, 10–35°E). This spatial domain included 15 grid cells, and covered Kongsfjorden (grid cell 1, Fig. 1 ESI†) and the coast of northern Norway where the PCB-153 concentrations in blue mussel were sampled (grid cell 9, 10, 12 and 15, Fig. 1 ESI†). Bioaccumulation simulations were run with a 12-hour time step from 1930 to 2021, and NEM produced lipid normalized concentrations of PCB-153 for all age groups of the species, as well as in seabird eggs. Additionally, NEM produced fugacities and transport rate constants (D-values) for each model compartment and process, respectively, which in turn were used to calculate the transport rates (N-values) for PCB-153 for each uptake and elimination process. Results were stored with a 120-hour (5 days) time step, and stored with a 24-hour time step when more detailed information (such as for egg-laying or incubation) was needed.
2.4. Model evaluation
Estimated concentrations by NEM of PCB-153 in air,31 seawater and dietary species (except the filter feeder) have already successfully been evaluated.32 To assess the efficiency of NEM in estimating the concentrations of PCB-153 in the newly added species, modelled concentrations were compared to measured ones in blood/plasma/muscle tissue within identical grid cells, on the same date, and across corresponding species, sexes, and age groups. For the estimated concentrations in the seabirds, only age groups with reproducing seabirds were included in the evaluation, as the age of the measured individuals was not known, only that they were of reproducing age. The measured data for PCB-153 utilised for evaluating NEM were drawn from various sources. A comprehensive overview of these measured concentrations, along with their respective references, is provided in Table 9 ESI.† All measurement data used in the evaluation were lipid normalized, either with corresponding lipid concentrations collected with the measurement data, or, where lacking, an average lipid concentration for the same species, sex, area, year and/or season.
Filter feeder evaluation used measured data from blue mussels sampled from the coast of northern Norway. In total 89 individual measurements from 1993–1997, 1999–2007, 2009, and 2012–2019 were collected from the Water Environment Database.51 Localities south of 60°N and close to harbours or larger settlements were removed to avoid areas without overwintering Svalbard eiders and areas influenced by local sources, respectively.
All measurements of PCB-153 in seabird blood and eggs were sampled from seabirds in Kongsfjorden. Yearly measurements of PCB-153 in whole blood sampled from female eiders in 2007–2017 (ref. 52) and 2017–2021 (ref. 51) were obtained for the model evaluation. In total, 281 individual concentrations spanning over 14 consecutive years (2007–2021) were used to evaluate the female eiders. Concentrations of PCB-153 in eider eggs sampled in 2015 (ref. 53) and 2017–2021 (ref. 51) were obtained, giving a total of 92 individual measurements for the model evaluation of eider eggs. A total of 347 individual measurements of PCB-153 in whole blood from female and male kittiwakes sampled in 2007–2011, 2015, 2017–2021 (ref. 54) were used for the model evaluation of kittiwakes. For the kittiwake egg evaluation, a total of 36 individual PCB-153 measurements sampled in 1993 (ref. 55), 2008 (ref. 56), 2013–2014 (ref. 51) and 2015 (ref. 53) were used. A total of 228 concentrations of PCB-153 in glaucous gull plasma sampled from female and male gulls in 2008–2021 (ref. 54) were used for the model evaluation of female and male glaucous gulls. PCB-153 concentrations in glaucous gull eggs sampled in 2012–2013, 2020 (ref. 54) and 2015 (ref. 53) were used for the evaluation, giving a total of 20 individual measurements for the model evaluation for glaucous gull eggs.
Multimedia model performance can be evaluated by comparing modelled concentrations with measured ones. The agreement metrics used in the current study were defined and described by Krogseth et al.32 These are the estimate-measurement ratio (EMR), also called prediction-measurement ratio (PMR) in Krogseth et al.;32 the geometric mean of all EMRs for a group (e.g. all female common eiders) defined as the model bias (MB) for that group; and the root mean square error of the log transformed estimated and measured concentrations (RMSElog). The following criteria evaluations were used:
- EMR: an EMR below 1 implies an underestimation of the modelled concentrations, and vice versa. As a general guideline, a ratio between 0.1 and 10 implies acceptable model performance.57
- MB: the closer MB is to 1, the better model performance. The direction of the agreement is given by MB below 1 (underestimation) or MB above 1 (overestimation).
- RMSElog: a smaller RMSElog equals a better model performance, e.g., when comparing between the different modelled species.
Additionally, assuming first-order kinetics for the measured time trends of PCB-153 in seabird blood, environmental half-lives (t1/2) were obtained, and compared to the equivalent from modelled time trends for the same area, time, sex, and age groups (an approach described in further detail in Krogseth et al.).32
2.5. Model applications
Exposure to POPs in Arctic seabirds has been suggested to be impacted by climate change3 in addition to changes in emissions of POPs into the environment. Here, NEM was used to investigate the future exposure of PCB-153 for three scenarios: changing global primary emissions of PCB-153 (Scenario A); dietary changes for the kittiwake due to “Atlantification” of the Barents Sea (Scenario B); and decreasing lipid loss in the eider during incubation due to increased air temperature (Scenario C). The impact of climate change on distribution or fate of PCB-153 in the physical environment was not accounted for in this study.
2.5.1. Scenario A.
The global primary emissions of PCBs are estimated to continue to decline in the future as they are currently released into the environment from standing stocks and waste piles.49 To predict the future body burdens of PCB-153 in the targeted Arctic seabirds, due to decreasing primary emissions, model simulations were run for the physical environment and the food web (including the three seabird species) in Kongsfjorden (between 80°N, 10°E and 75°N, 15°E). Global emission scenario for PCB-153 from 1930 to 2100 (ref. 49) was used as input, and the bioaccumulation model was run with a 12-hour time step from 1930 to 2100, producing lipid normalized concentrations for all age groups of eiders, kittiwakes and glaucous gulls. The results were stored with a 1752-hour time step (72 days), as more detailed information was deemed redundant.
2.5.2. Scenario B.
The kittiwake's diet is assumed to consist of prey species that are adapted to polar environmental conditions (Section 1.4.5 ESI†), of which several are largely dependent on sea ice. A shift from ice-dependent to Atlantic prey species has been observed for kittiwakes in Kongsfjorden, starting from 2006.46 In the current study, hypothesised future scenarios (FS) for the kittiwake comprised various anticipated changing dietary composition. Firstly, due to on-going Atlantification of the Barents Sea, ice-dependent species (polar cod) can be expected to be substituted with an Atlantic species (Atlantic cod; FS1). Secondly, ice-dependent species (polar cod, capelin, and amphipods) can be expected to disappear from the diet and be substituted by herring and krill (FS2). The assumed current diet composition as well those for the two future scenarios are listed in Table 4 ESI.† The input diet for the kittiwakes was changed to FS1 and FS2, and simulations were run with NEM for female and male kittiwakes in Kongsfjorden in a 12-hour time step from 2006 to 2100. The results were stored with a 1752-hour time step (72 days), and compared to the modelled time trends for the same period under Scenario A.
2.5.3. Scenario C.
The female eider has a complex lipid dynamic during reproduction, due to starvation during incubation under harsh Arctic conditions.42 In NEM, the minimum lipid content after incubation was assumed to be 9.6% of the total body weight58 (Section 1.3.3 ESI†). When the ambient air temperature is <7 °C, eiders increase their energy expenditure in order to maintain an optimal body temperature.59 Female eiders incubating in an average ambient temperature of 8 °C (Tromsø, Norway) lost less of their body weight compared to eiders nesting in an average ambient temperature of 2.5 °C (Kongsfjorden, Svalbard).60 The average temperature in Kongsfjorden in June 2020 was 4 °C (The Norwegian Meteorological Institute, https://www.yr.no/nb, 2024). In June 2100, the average temperature in Kongsfjorden is estimated to be 5.6 °C.61,62 A scenario was hypothesised where higher ambient temperatures in Kongsfjorden would lead to decreased loss of lipids for female eiders during incubation. The input lipid content after incubation was therefore set to 20%, and NEM was run in a 12-hour time step for female eiders in Kongsfjorden from 2020 to 2100. The results were stored in a 24-hour time step to get daily concentrations. Likewise, a simulation with a lipid content of 9.6% after incubation was also run in a 24-hour time step to facilitate comparison between the two. Median concentrations in breeding females from egg-laying (assumed to be June 19th) until the normal body weight was regained after incubation (assumed to be 29 days after hatching, August 12th; see Section 1.3.3 ESI† for details on the eider's breeding periods), meaning a period of declining and increasing body weight, were compared between the two model simulations.
3 Results and discussion
3.1. Model evaluation across species
The median concentrations of both measured and modelled data for filter feeders, common eiders, black-legged kittiwakes and glaucous gulls are illustrated in Fig. 2, together with the earlier reported food web evaluation by Krogseth et al.32 The transport rates (N) in mol h−1 for the uptake and elimination of PCB-153 in the species are given in Fig. 8 ESI.† The importance to the total uptake of PCB-153 from each dietary species in the seabirds' diet is given in Fig. 9 ESI.†
 |
| Fig. 2 Model results for the filter feeder and seabirds in the present study (squares), plotted together with the species included in NEM by Krogseth et al.32 (circles). The plots are the median of the measured concentrations, and the median of the equivalent modelled concentrations. The error bars represent the range in these measured and modelled concentrations. The concentrations are on a logarithmic scale to facilitate comparison between modelled and measured concentrations, and the solid line represents a 1 : 1 fit. The dotted lines represent a fit within one order of magnitude, i.e., acceptable model performance. Note that the kittiwake egg point (purple square) is hidden by the overlapping glaucous gull egg point (light green square), and that the kittiwake male point (light blue square) is hidden by the overlapping glaucous gull female point (dark red square). Figure adapted from Krogseth et al. (2023),32https://doi.org/10.1039/D2EM00439A CC BY 3.0. | |
3.1.1. Filter feeder.
Measured concentrations of PCB-153 in blue mussels ranged from 7.04 to 186 ng/g lw (n = 89).51 The comparison between modelled and measured concentrations are shown in Fig. 2 and 3 ESI.† The EMRs, MBs and RMSElogs for individual grid cells are shown in Table 10 ESI.† The best fit was seen for grid cell 12 in the south-eastern part of the modelling area (EMR range = 0.1–3), the other three grid cells were underestimated (EMR range = 0.01–0.5). Overall, the model underestimated 82% of the individual PCB-153 concentrations across the four grid cells, with EMRs ranging between 0.01 and 3 (median EMR = 0.2, MB = 0.3, RMSElog = 8, see Fig. 2 ESI†). The underestimation could be allocated to a too low ventilation/feeding rate, a too low absorption efficiency from the diet, or unfitting diet composition and lipid content of dietary species. Moreover, the hight spatial resolution of the model run (5° × 5°, latitude/longitude) could contribute to underestimating PCB-153 concentrations in the filter feeders, which were collected in coastal areas (see Fig. 1 ESI†). This is the first evaluation of the filter feeder compartment in NEM for PCB-153. A comparable model assessing concentrations of cyclic volatile methyl siloxanes in a freshwater filter feeder also indicated underestimated concentrations, with EMRs ranging from 0.04 to 0.4.33
The modelled concentrations from the north Norwegian coast were 0.5–1.2 times larger than the equivalent modelled concentrations from Kongsfjorden. Only a few measurements of PCBs in mussels from Kongsfjorden exists.63 Relevant for the common eider's diet, PCB-153 in the mussel species Bathyarca glacialis and Ciliatocardium ciliatum ranged from 22.7 to 115 ng/g lw (n = 6) in 2007.63 Comparing modelled concentrations in the filter feeder to the measured ones in B. glacialis and C. ciliatum (Fig. 2 ESI†), an underestimation with EMRs ranging from 0.04 to 0.2 was seen (median EMR = 0.1, MR = 0.1, RMSElog = 11).
3.1.2. Eiders, kittiwakes and glaucous gulls.
The evaluation metrics (EMR, MB and RMSElog) are listed in Table 11 ESI.† Measured whole blood concentrations of PCB-153 in female common eiders ranged from 8.5 to 1700 ng/g lw (n = 281).51,52 The EMRs ranged from 0.030 to 7.3, with a median of 0.87 (MB = 0.79, RMSElog = 4.2) for the eiders (Fig. 4 ESI†). The EMRs for kittiwakes ranged from 0.48 to 1.4 with a median of 0.85 for females (MB = 0.85 and RMSElog = 7.2), and from 0.60 to 1.2 with a median of 0.87 for males (MB = 0.87 and RMSElog = 6.0, Fig. 3 ESI†). The measured whole blood PCB-153 concentrations54 ranged from 140 to 470
000 ng/g lw (n = 158), and from 310 to 40
000 ng/g lw (n = 186) for female and male kittiwakes, respectively. Lower modelled concentrations in females than in males is explained by egg-laying, which is an important contaminant elimination pathway for female seabirds. In the mechanistic modelling study by Borgå et al.,30 PCB-153 in kittiwakes was underestimated with a ratio of 0.12 (factor 8) when modelling based on measured concentrations in seawater as input and investigating the impact of climate change on bioaccumulation in an Arctic marine food web. As highlighted by Borgå and Di Guardo,64 using measured concentrations as input to models entail some uncertainties. For example, using measurement data from areas with low concentrations is highly affected by the sensitivity of analysis. Additionally, measured PCB concentrations in seawater are impacted by differences in sampling methods between studies.64 Utilizing chemical emissions and dynamic mechanistic modelling with spatial resolution can be a complementary approach to using measured seawater concentrations as input. NEM has previously shown to simulate seawater concentrations with EMRs ranging from 0.10 to 31, where 64% of estimated concentrations were within a factor 4 of measurements based on spatial resolution of 1° × 1° latitude/longitude.32
Measured plasma concentrations of PCB-153 in glaucous gulls from Kongsfjorden in 2008–2021 (ref. 54) ranged from 390 to 25
000 ng/g lw (n = 151) in females and from 1200 to 32
000 ng/g lw (n = 77) in males. The female glaucous gull's EMR ranged from 0.039 to 3.8 (median EMR = 0.36, MB = 0.36, RMSElog = 6.0) and the males' EMR ranged from 0.061 to 1.6 (median EMR = 0.23, MB = 0.25, RMSElog = 6.9, Fig. 4 ESI†). Baert et al.65 used mechanistic and statistical modelling to simulate the effects of migration and opportunistic feeding on the accumulation of PCBs in several Arctic seabirds, including the three species included in the present study. The authors found that migration had a significant impact on PCB accumulation, highlighting the importance of considering both traits when assessing contaminant exposure in Arctic seabirds. Additionally, opportunistic feeding was highlighted as important for the contaminant exposure in glaucous gulls, as they are opportunistic scavengers, which makes for a complex parameterization of complete dietary compositions.
When comparing the root mean square error (RMSElog) among the modelled species in the current study, the female eider exhibited the closest agreement between modelled and measured concentrations. Sorting all evaluated compartments from lowest RMSElog (and thus best fit between modelled and measured concentrations) to the highest RMSElog gave the following order: female eider < male kittiwake < female glaucous gull < male glaucous gull < female kittiwake < filter feeder.
Modelled time trends from 1930 to 2023 are plotted together with measured concentrations (median, error bars represent max and min values) in Fig. 3. The linear regressions and environmental half-lives are given in Fig. 7 and Table 12 ESI,† respectively. Note that this time trend analysis does not consider interannual variations in biological factors and environmental conditions and should be only interpreted as an evaluation of the model's performance.
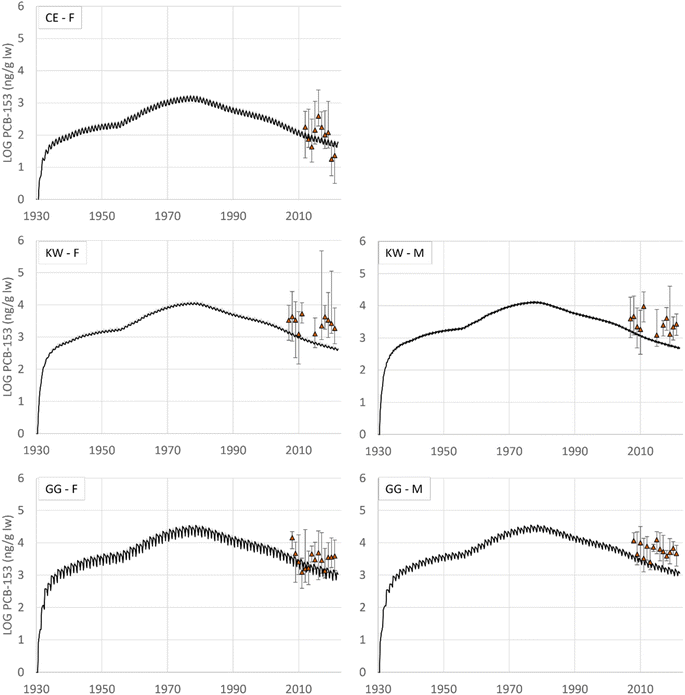 |
| Fig. 3 Modelled (solid line) and measured (triangle) concentrations of PCB-153 (log ng/g lw) included in the present study to evaluate NEM for female common eider (CE – F), female and male black-legged kittiwake (KW – F and KW – M), and female and male glaucous gull (GG – F and GG – M). The black line represent the median (across the age groups of breeding seabirds) of the modelled time series, while the triangles represent the yearly median measured concentrations, with error bars representing min and max concentrations. | |
All modelled time trends showed a consistent decline for all the seabird species, with t1/2 ranging from 8.6 to 9.1 years (p < 0.05). Similarly, the measured concentrations showed a decline over 14 years (2007–2021) for female eiders, with a t1/2 of 12 years (95% confidence interval: 8.4–22 years, p < 0.05). A more in-depth time trend analysis considering biological factors and environmental conditions was conducted for the same measurement data from 2007 to 2017 by Bustnes et al.,52 which did not report a significant temporal trend for PCB-153. The measured time trends for male kittiwakes over 14 years (2007–2021) also showed a decline with t1/2 of 21 years (95% confidence interval: 11–97 years, p < 0.05). For the female glaucous gull on the other hand, the measured time trend showed an increase over the measuring period, with an environmental doubling time of 19 years, but with large uncertainties (95% confidence interval: 790–9.3 years, p < 0.05). The measured time trend for female kittiwakes and male glaucous gulls were inconclusive (p > 0.05). Empirical time trends of POPs (including PCB-153) in Arctic biota show declines across several groups of species (marine mammals, seabirds including black-legged kittiwakes and glaucous gulls, and marine and freshwater fish). This study allocated recent levelling off in declines to climate change.4
Krogseth et al.32 conducted a similar temporal trend analysis for Norwegian fish as in the present study, and found that NEM modelled time trends had a steeper decline than observed in the measured time trends. This bias of NEM is underscored in the present study as well, while the model performances are in closer agreement with the measured time-trends for female eiders and male kittiwakes.
Some of the biological input parameters and assumptions that could influence the performance of NEM for seabirds include (but are not limited to) biotransformation, maternal transfer to eggs and migration. Chemical specific biotransformation rates for PCB-153 have to the best of our knowledge not been reported for the seabirds in the current study. The biotransformation rates used in the current study was based on biotransformation in humans and adapted to the seabirds (see Section 2.3). For kittiwakes, the assumed biotransformation rate for PCB-153 (2.05 × 10−5 h−1, half-life of 3.9 years) was slower than what Borgå et al.30 assumed for biotransformation of PCB-153 in kittiwakes (6.94 × 10−5 h−1, half-life of 1.14 years), based on experimental studies on American kestrels (Falco sparverius). Thus, kittiwakes may metabolize PCB-153 at a higher rate than currently assumed, which is also hypothesized in the study by Borgå et al.30
For the maternal transfer of contaminants between the female seabird and the egg in NEM, a mother-egg ratio for PCB-153 in herring gull was used (see Section 2.3). Verreault et al.37 reported concentrations of polybrominated diphenyl ethers (PBDEs) in breeding female glaucous gulls and their eggs, with ratios around 1. To our knowledge, chemical-specific mother-egg ratios for PCB-153 has not been reported for the seagulls included in the current study.
Simplified migration scenarios for Norwegian spring-spawning herring and Northeast Atlantic cod have previously been investigated using NEM, but did not show a significant impact on modelled PCB-153 concentrations in these species.32 Migration has been identified as important driving factors for PCB-153 bioaccumulation in Arctic seabirds, including common eiders, black-legged kittiwakes and glaucous gulls.65 A recent study in Arctic kittiwakes showed that wintering areas are influencing the blood concentrations of perfluoroalkyl substances (PFAS) measured during the following breeding season in Kongsfjorden.66 In the modelling study by Binnington et al.,34 migration was included for the Canada goose, which occupies a low trophic level and is exposed to contaminants through its surroundings (e.g. grass, water, air). Modelling species from higher trophic levels, such as eiders, kittiwakes and glaucous gulls, requires taking into account the seasonal variation of the characteristics of its prey species (e.g. dietary composition, lipid dynamics, or reproduction). Knowledge on dietary ecology and chemical parameters from the seabirds outside of the breeding period is currently limited. The seabird species from the current study are known to migrate in various wintering areas of the northern hemisphere (see Section 2.2.2). Regional differences in contaminant loads in prey species may lead to varying concentrations of PCBs in migratory species compared to resident.65,67
3.1.3. Seabird eggs.
Measured concentrations of PCB-153 in eider eggs ranged from 12 to 86 ng/g lw (n = 92) sampled in Kongsfjorden in 2015 and 2017–2021. The EMR between measured and modelled concentrations ranged from 0.18 to 1.6 (median EMR = 0.56, MB = 0.53, RMSElog = 3.3). Measured PCB-153 concentrations in kittiwake eggs ranged from 820 to 4900 ng/g lw (n = 36), sampled in Kongsfjorden in 1993, 2008, and 2013–2015. The EMRs ranged from 0.12 to 1.4 (median EMR = 0.45, MB = 0.44, RMSElog = 4.5). Measured PCB-153 concentrations in glaucous gull eggs from Kongsfjorden ranged from 700 to 3200 ng/g lw (n = 20), sampled in 2012, 2013, 2015 and 2020. The EMRs for the glaucous gull eggs ranged from 0.085 to 0.57 (median EMR = 0.23, MB = 0.22, RMSElog = 6.8). The fit between measured and modelled concentrations of PCB-153 in seabird eggs are shown in Fig. 4.
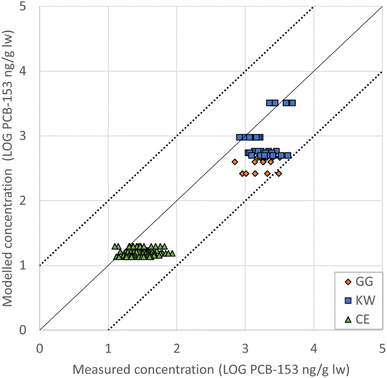 |
| Fig. 4 Measured concentrations of PCB-153 in eggs from common eiders (CE, triangle), black-legged kittiwakes (KW, square) and glaucous gulls (GG, diamond) plotted against the corresponding NEM modelled concentrations. Modelled and measured concentrations are on a logarithmic scale to facilitate comparison, and the solid line represents a 1 : 1 fit between modelled and measured concentrations, meaning data point closer to this line is better. The stippled line represents a fit within one order of magnitude. The modelled concentrations in the kittiwake eggs appear to be divided into three groups due to hiatus in the sampling period between 1993, 2008 and 2013. | |
The EMRs reported for eggs in the current study are comparable to that reported in a study on model-estimated concentrations of POPs in seabird eggs, where EMRs between 0.7 and 1.5 were reported for POPs (dichlorodiphenyldichloroethylene, dieldrin, Mirex and hexachlorobenzene) in herring gull (Larus argentatus) eggs in Lake Ontario in 1985–92.39 In that study however, measured environmental and biotic concentrations of POPs were used as input to the model, in contrast to the current study where global emissions of PCB-153 were used. The potential problems using measured concentrations as input is discussed further under Section 3.1.2.
3.2. Impact of primary emissions and climate change on PCB-153 exposure in Arctic seabirds
Three future scenarios were tested, where (A) global emission estimates of PCB-153 up until 2100 were used as input to the bioaccumulation module in NEM; (B) climate induced “Atlantification” of Kongsfjorden led to changes in the dietary exposure of PCB-153 in black-legged kittiwakes; and (C) higher ambient temperatures led to changes in lipid dynamics during incubation for the female common eider. Modelled concentrations of PCB-153 in common eiders, black-legged kittiwakes, and glaucous gulls for 2000 to 2100 from implementing the three scenarios are shown in Fig. 5 (female seabirds only for simplicity) and Fig. 10 ESI† (male kittiwakes and glaucous gulls).
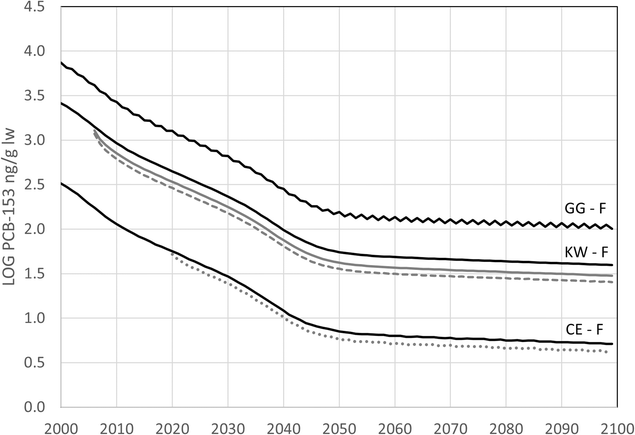 |
| Fig. 5 Modelled concentrations of PCB-153 in female (F) common eider (CE), black-legged kittiwake (KW) and glaucous gull (GG), for Kongsfjorden, Svalbard from 2000 to 2100. For simplicity, only females are included in the figure, and the figure showing modelled concentrations in male seabirds can be found in ESI, Fig. 10.† The concentrations are the median of all age groups. Underlying grey lines for KW and CE are the modelled time trends given two dietary scenarios for kittiwakes starting in 2006,46 where polar cod has been replaced by Atlantic cod (FS1, grey stippled line), and all ice-dependent species have been replaced (FS2, grey unbroken line); and a scenario for the female eider, where climate change has led to changed lipid dynamics during reproduction (grey dotted line). The concentrations are log-scaled to facilitate comparison among species. | |
3.2.1. Scenario A: decreased global primary emissions.
The modelled concentrations of PCB-153 in seabirds between 1930 and 2023 (Section 3.1, Fig. 3) indicate an increasing trend until around 1980, followed by a decline in concentrations until 2023. This reflects the global emission scenarios, with a time lag of approximately 10 years between peak emissions (ca. 1970)49 and maximum bioaccumulation in these seabirds (ca. 1980, Fig. 3). The global emission scenario was characterised by a production peak around 1970, followed by a peak in exports of PCB-containing electronic products just before year 2000. The subsequent decline is attributed to international restrictions and the estimated lifespan of these products (approximately 60 years after production). Continued emissions from standing stocks and waste piles are expected to contribute to trends up to 2100.49
The projected concentrations of PCB-153 in common eiders, kittiwakes, and glaucous gulls from 2000 to 2100, are shown in Fig. 5 (median of all age groups). This scenario takes into account estimated global emissions and shows a continuing declining trend, with concentrations decreasing by 99% by 2100 across the seabird species (female eider: 330 to 5.1 ng/g lw, female kittiwake: 2600 to 40 ng/g lw, male kittiwake: 3000 to 47 ng/g lw, female glaucous gull: 7400 to 100 ng/g lw, male glaucous gull: 7600 to 100 ng/g lw). Empirical time trends indicate that the decline in PCB-153 concentrations in fish and seabirds over the past decades may be slower than currently predicted by NEM (see Section 2.4). This suggests that future declines in PCB-153 concentrations may also be slower than in the current model predictions. Moreover, this scenario does not account for confounding effects related to changing climate conditions.
The presence of PCB-153 in Arctic biota highlights the long-lasting nature of this compound. In fact, the effect of regulations such as the SC on global emissions of POPs (including PCBs) is being discussed.68
Previous estimates from NEM indicate that approximately 50% of PCB-153 in the Svalbard region originates from regions outside Europe and Russia.32 This finding, combined with the future projections in the current study, underscores the critical need for long term and global regulations on POPs, including for unintentional production of these compounds.
3.2.2. Scenario B: Atlantification of dietary sources for the black-legged kittiwake.
Introducing Atlantification of the black-legged kittiwake's diet composition up until 2100 resulted in PCB-153 concentrations in the same range as in Scenario A (Fig. 5 and 10 ESI†). In Fig. 5 and 10 ESI,† an increasing distance between the time trends for the current diet and the scenarios is seen, which is allocated that the scenarios were run from 2006 building on the same time trends as the current diet. Using linear regression on the concentrations between 2006 and 2100, the concentrations declined by 8.2 ng/g lw, 5.6 ng/g lw and 6.4 ng/g lw yearly for females considering the current diet, FS1 and FS2, respectively. Similar patterns were predicted for males, where concentrations declined by 9.6 ng/g lw, 6.7 ng/g lw and 7.6 ng/g lw yearly for the current diet, FS1 or FS2, respectively. FS2 (no sea ice dependent species in the diet) yielded the lowest concentrations, which were up to 23% and 24% lower than for the scenario with the current diet for females and males, respectively. The importance of each specie to the total uptake of PCB-153 through the diet is shown in Fig. 11 ESI.† In FS2, the main contribution for PCB-153 uptake through the diet comes from krill (90%), and the rest from herring (10%). This matches the dietary composition in FS2 (Table 4 ESI†). The concentrations from FS1 (Atlantic cod replacing polar cod) were 18% and 19% lower than for the current diet of female and male kittiwakes, respectively. As shown in Fig. 11 ESI,† polar cod has the highest importance for the total dietary uptake of PCB-153 in the current diet (62%), followed by krill (22%), herring (6%), capelin (5%), and amphipods (5%). In FS1, the main importance for the total uptake of PCB-153 is shifted over to Atlantic cod (41%) and krill (36%), followed by herring (9%), capelin (7%) and amphipods (7%). Considering these fractions and the fractions of the prey species in the diets (Table 4 ESI†) reveals that Atlantic cod accounts for a smaller contribution to the total uptake of PCB-153 than what polar cod has in the current diet. This is because the kittiwakes are assumed to feed on polar cod that are up to 4 years old, but Atlantic cod that are less than 1 year old (Table 5 ESI†), thus having accumulated less contaminants through their lifetime. Also, the polar cod in NEM is assumed to have a higher lipid content than Atlantic cod,32 leading to higher concentrations of PCB-153 in their bodies. Krill contributes to the total uptake of PCB-153 almost as much as Atlantic cod in FS1. Note however, that as NEM omits migration, the Atlantic cod in these simulations are from Kongsfjorden, while known spawning grounds for Atlantic cod includes the North Sea.69 Higher concentrations of PCBs were measured in the Norwegian Sea compared to the Barents Sea.70
3.2.3. Scenario C: decrease in lipid loss in common eider during incubation due to increased ambient air temperature.
Comparing concentrations in breeding female common eiders with decreased loss of lipids to concentrations in females with a normal loss of lipids, revealed that NEM estimated down to 2.4% lower concentrations in 2100 after introducing the changed lipid dynamics (Fig. 5). This indicates that changes in lipid dynamics during incubation will likely not be a key driver in the future exposure of PCB-153 in breeding female common eiders. Predicted lower concentrations in PCB-153 in response to a decrease in lipids loss in the eiders during incubation due to increased air temperature supports the hypothesis by Bustnes et al.60 The authors utilized statistical modelling to investigate the correlation between POP concentrations and breeding investment, and hypothesised that contaminant concentrations in incubating eiders would be negatively related to ambient air temperature in the future. However, the change is marginal, and other variables might have a stronger impact on the contaminant exposure. Indeed, a recent statistical modelling study by Bustnes et al. actually showed a positive relationship between PCB-153 concentrations in common eider and ambient temperature between 2007–2017, which does not support the former hypothesis.52 The authors hypothesized that a warmer climate could lead to higher levels of organic contaminants in the breeding female eiders by altered reproductive investments. A warmer climate could allow the female eider to cease incubation to feed, and thus being more exposed to contaminants through the diet.
4 Conclusions
This study demonstrated that NEM successfully simulated PCB-153 concentrations in Arctic seabirds. Generally, the concentrations in the seabirds were underestimated by the model. The female common eiders had median EMR of 0.87, and the kittiwakes were underestimated with median EMRs of 0.85 and 0.87 for females and males, respectively. The glaucous gulls were underestimated with median EMRs of 0.36 and 0.23 for females and males, respectively. PCB-153 concentrations in filter feeders along the Norwegian coast were also underestimated in NEM, with a median EMR of 0.2. NEM predicted a steeper decline in contaminant concentrations than observed for the measured concentrations. Future simulations implied continuing declining trends of PCB-153 in the seabirds, and minor effects of climate-induced changes in dietary exposure and lipid dynamics on PCB-153 concentrations were observed for kittiwakes and eiders, respectively.
Refinements to NEM that are recommended, includes incorporating empirical chemical- and species-specific biotransformation rates, empirical mother-egg contaminant transfer ratios, and migratory behaviours. The latter is dependent on assessed seasonal variations in diet composition, as geographical location naturally influences the availability of prey species. As international agreements and restrictions are implemented, new chemicals are continuously being synthesized, some of which may pose environmental risks. The distribution and bioaccumulation of semi-volatile organic contaminants in Arctic food webs can be explored using environmental models like NEM, pending known physical–chemical properties of the chemical. NEM can also be adapted, evaluated, and applied to other Arctic species of concern. Additionally, NEM can be used to investigate differences in contaminant concentrations across various Arctic regions.
Data availability
The data supporting this article have been included as part of the ESI.†
Author contributions
Lovise P. Skogeng: conceptualization, data curation, formal analysis, investigation, methodology, visualization, writing – original draft. Pierre Blévin: data curation, writing – review and editing. Knut Breivik: data curation, writing – review and editing. Jan Ove Bustnes: Conceptualization, writing – review and editing. Igor Eulaers: conceptualization, data curation, writing – review and editing. Kjetil Sagerup: data curation, writing – review and editing. Ingjerd Sunde Krogseth: conceptualization, data curation, funding acquisition, methodology, project administration, supervision, writing – review and editing.
Conflicts of interest
There are no conflicts to declare.
Acknowledgements
The present study was funded by the Research Council of Norway (RCN) (The COPE project, #287114), the Fram Centre Flagship for Hazardous Substances – effects on ecosystems and human health (#142018), and the Fram Centre research programme CLEAN. The authors thank Dorte Herzke for supplying PCB-153 measurement data, Sabine Eckhardt for generating temperature data using Copernicus Climate Change Service information 2024 (https://doi.org/10.24381/cds.c866074c), and Christine F. Solbakken for contributing with the illustrations.
References
- M. Rantanen, A. Y. Karpechko, A. Lipponen, K. Nordling, O. Hyvärinen, K. Ruosteenoja, T. Vihma and A. Laaksonen, The Arctic has warmed nearly four times faster than the globe since 1979, Commun. Earth Environ., 2022, 3, 168 CrossRef.
- J. C. Deb and S. A. Bailey, Arctic marine ecosystems face increasing climate stress, Environ. Rev., 2023, 31, 403–451 CrossRef.
-
AMAP, AMAP Assessment 2020: POPs and Chemicals of Emerging Arcic Concern: Influence of Climate Change, Arctic Monitoring and Assessment Programme (AMAP), Tromsø, Norway, 2021, vol. 8, pp. 134 Search PubMed.
- F. Rigét, A. Bignert, B. Braune, M. Dam, R. Dietz, M. Evans, N. Green, H. Gunnlaugsdóttir, K. S. Hoydal, J. Kucklick, R. Letcher, D. Muir, S. Schuur, C. Sonne, G. Stern, G. Tomy, K. Vorkamp and S. Wilson, Temporal trends of persistent organic pollutants in Arctic marine and freshwater biota, Sci. Total Environ., 2019, 649, 99–110 CrossRef.
- H. Hung, C. Halsall, H. Ball, T. Bidleman, J. Dachs, A. De Silva, M. Hermanson, R. Kallenborn, D. Muir, R. Sühring, X. Wang and S. Wilson, Climate change influence on the levels and trends of persistent organic pollutants (POPs) and chemicals of emerging Arctic concern (CEACs) in the Arctic physical environment – a review, Environ. Sci.: Processes Impacts, 2022, 24, 1577–1615 RSC.
-
Secretariat of the Stockholm Convention (SSC), Stockholm Convention on Persistent Organic Pollutants (POPs), 2023.
-
AMAP, AMAP Assessment 2021: Human Health in the Arctic, Arctic Monitoring and Assessment Programme (AMAP), Tromsø, Norway, 2021, vol. 10, pp. 240 Search PubMed.
-
AMAP, AMAP Assessment 2018: Biological Eff ects of Contaminants on Arctic Wildlife and Fish, Arctic Monitoring and Assessment Programme (AMAP), Tromsø, Norway, 2018, vol. 7, pp. 84 Search PubMed.
- F. Wong, H. Hung, H. Dryfhout-Clark, W. Aas, P. Bohlin-Nizzetto, K. Breivik, M. N. Mastromanco, E. B. Lundén, K. Ólafsdóttir, A. Sigurðsson, K. Vorkamp, R. Bossi, H. Skov, H. Hakola, E. Barresi, E. Sverko, P. Fellin, H. Li, A. Vlasenko, M. Zapevalov, D. Samsonov and S. Wilson, Time trends of persistent organic pollutants (POPs) and Chemicals of Emerging Arctic Concern (CEAC) in Arctic air from 25 years of monitoring, Sci. Total Environ., 2021, 775, 145109 CrossRef PubMed.
-
AMAP, AMAP Arctic Climate Change Update 2021: Key Trends and Impacts, Arctic Monitoring and Assessment Programme (AMAP), Tromsø, Norway, 2021, vol. 8, pp. 148 Search PubMed.
- K. Borgå, M. A. McKinney, H. Routti, K. J. Fernie, J. Giebichenstein, I. G. Hallanger and D. C. G. Muir, The influence of global climate change on accumulation and toxicity of persistent organic pollutants and chemicals of emerging concern in Arctic food webs, Environ. Sci.: Processes Impacts, 2022, 24, 1544 RSC.
- A. Frainer, R. Primicerio, S. Kortsch, M. Aune, A. V. Dolgov, M. Fossheim and M. M. Aschan, Climate-driven changes in functional biogeography of Arctic marine fish communities, Proc. Natl. Acad. Sci. U.S.A., 2017, 46, 12202–12207 CrossRef.
- M. Fossheim, R. Primicerio, E. Johannesen, R. B. Ingvaldsen, M. M. Aschan and A. V. Dolgov, Recent warming leads to a rapid borealization of fish communities in the Arctic, Nat. Clim. Change, 2015, 5, 673–677 CrossRef.
- K. Vorkamp, P. Carlsson, S. Corsolini, C. A. De Wit, R. Dietz, M. O. Gribble, M. Houde, V. Kalia, R. J. Letcher, A. Morris, F. F. Riget, H. Routti and D. C. G. Muir, Influences of climate change on long-term time series of persistent organic pollutants (POPs) in Arctic and Antarctic biota, Environ. Sci.: Processes Impacts, 2022, 24, 1643–1660 RSC.
- T. Divya David and K. P. Krishnan, Recent variability in the Atlantic water intrusion and water masses in Kongsfjorden, an Arctic fjord, Polar Sci., 2017, 11, 30–41 CrossRef.
- H. Hop, T. Pearson, E. N. Hegseth, K. M. Kovacs, C. Wiencke, S. Kwasniewski, K. Eiane, F. Mehlum, B. Gulliksen, M. Wlodarska-Kowalczuk, C. Lydersen, J. M. Weslawski, S. Cochrane, G. W. Gabrielsen, R. J. G. Leakey, O. J. Lønne, M. Zajaczkowski, S. Falk-Petersen, M. Kendall, S. Å. Wängberg, K. Bischof, A. Y. Voronkov, N. A. Kovaltchouk, J. Wiktor, M. Poltermann, G. Di Prisco, C. Papucci and S. Gerland, The marine ecosystem of Kongsfjorden, Svalbard, Polar Res., 2002, 21, 167–208 CrossRef.
- P. Blévin, S. A. Shaffer, P. Bustamante, F. Angelier, B. Picard, D. Herzke, B. Moe, G. W. Gabrielsen, J. O. Bustnes and O. Chastel, Organochlorines, perfluoroalkyl substances, mercury, and egg incubation temperature in an Arctic seabird: Insights from data loggers, Environ. Toxicol. Chem., 2018, 37, 2881–2894 CrossRef.
- A. Goutte, C. Barbraud, D. Herzke, P. Bustamante, F. Angelier, S. Tartu, C. Clément-Chastel, B. Moe, C. Bech, G. W. Gabrielsen, J. O. Bustnes and O. Chastel, Survival rate and breeding outputs in a high Arctic seabird exposed to legacy persistent organic pollutants and mercury, Environ. Pollut., 2015, 200, 1–9 CrossRef CAS.
- M. Melnes, G. W. Gabrielsen, D. Herzke, K. Sagerup and B. M. Jenssen, Dissimilar effects of organohalogenated compounds on thyroid hormones in glaucous gulls, Environ. Res., 2017, 158, 350–357 CrossRef CAS.
- J. O. Bustnes, O. Miland, M. Fjeld, K. E. Erikstad and J. U. Skaare, Relationships between ecological variables and four organochlorine pollutants in an artic glaucous gull (Larus hyperboreus) population, Environ. Pollut., 2005, 136, 175–185 CrossRef CAS.
- T. Nordstad, B. Moe, J. O. Bustnes, C. Bech, O. Chastel, A. Goutte, K. Sagerup, C. Trouvé, D. Herzke and G. W. Gabrielsen, Relationships between POPs and baseline corticosterone levels in black-legged kittiwakes (Rissa tridactyla) across their breeding cycle, Environ. Pollut., 2012, 164, 219–226 CrossRef CAS PubMed.
- K. Sagerup, K. Åsbakk, A. Polder, J. U. Skaåre, G. W. Gabrielsen and R. T. Barrett, Relationships Between Persistent Organic Pollutants and Circulating Immunoglobulin-Y in Black-Legged Kittiwakes and Atlantic Puffins, J. Toxicol. Environ. Health, 2014, 77, 481–494 CrossRef CAS.
- S. Tartu, F. Angelier, D. Herzke, B. Moe, C. Bech, G. W. Gabrielsen, J. O. Bustnes and O. Chastel, The stress of being contaminated? Adrenocortical function and reproduction in relation to persistent organic pollutants in female black legged kittiwakes, Sci. Total Environ., 2014, 476–477, 533–560 Search PubMed.
- K. M. Murvoll, J. U. Skaare, H. Jensen and B. M. Jenssen, Associations between persistent organic pollutants and vitamin status in Brünnich's guillemot and common eider hatchlings, Sci. Total Environ., 2007, 381, 134–145 CrossRef CAS.
- A. Fenstad, J. O. Bustnes, C. G. Bingham, M. Öst, K. Jaatinen, B. Moe, S. A. Hanssen, A. J. Moody, K. M. Gabrielsen, D. Herzke, S. Lierhagen, B. M. Jenssen and Å. Krøkje, DNA double-strand breaks in incubating female common eiders (Somateria mollissima): Comparison between a low and a high polluted area, Environ. Res., 2016, 151, 297–303 CrossRef CAS.
- N. Verboven, J. Verreault, R. J. Letcher, G. W. Gabrielsen and N. P. Evans, Adrenocortical function of Arctic-breeding glaucous gulls in relation to persistent organic pollutants, Gen. Comp. Endocrinol., 2010, 166, 25–32 CrossRef CAS.
- N. Verboven, J. Verreault, R. J. Letcher, G. W. Gabrielsen and N. P. Evans, Nest temperature and parental behaviour of Arctic-breeding glaucous gulls exposed to persistent organic pollutants, Anim. Behav., 2009, 77, 411–418 CrossRef.
-
D. Mackay and J. M. Parnis, Multimedia Environmental Models: the Fugacity Approach, CRC Press, 3rd edn, 2021 Search PubMed.
- D. T. F. Kuo, B. A. Rattner, S. C. Marteinson, R. Letcher, K. J. Fernie, G. Treu, M. Deutsch, M. S. Johnson, S. Deglin and M. Embry, A Critical Review of Bioaccumulation and Biotransformation of Organic Chemicals in Birds, Rev. Environ. Contam. Toxicol., 2022, 260, 6 CrossRef.
- K. Borgå, T. M. Saloranta and A. Ruus, Simulating climate change-induced alterations in bioaccumulation of organic contaminants in an Arctic marine food web, Environ. Toxicol. Chem., 2010, 29, 1349–1357 CrossRef PubMed.
- K. Breivik, S. Eckhardt, M. S. McLachlan and F. Wania, Introducing a nested multimedia fate and transport model for organic contaminants (NEM), Environ. Sci.: Processes Impacts, 2021, 23, 1146–1157 RSC.
- I. S. Krogseth, K. Breivik, S. Frantzen, B. N. Nilsen, S. Eckhardt, T. H. Nøst and F. Wania, Modelling PCB-153 in northern ecosystems across time, space, and species using the nested exposure model, Environ. Sci.: Processes Impacts, 2023, 25, 1986–2000 RSC.
- I. S. Krogseth, E. Undeman, A. Evenset, G. N. Christensen, M. J. Whelan, K. Breivik and N. A. Warner, Elucidating the Behavior of Cyclic Volatile Methylsiloxanes in a Subarctic Freshwater Food Web: A Modeled and Measured Approach, Environ. Sci. Technol., 2017, 51, 12489–12497 CrossRef CAS PubMed.
- M. J. Binnington, M. S. Curren, C. L. Quinn, J. M. Armitage, J. A. Arnot, H. M. Chan and F. Wania, Mechanistic polychlorinated biphenyl exposure modeling of mothers in the Canadian Arctic: the challenge of reliably establishing dietary composition, Environ. Int., 2016, 92–93, 256–268 CrossRef.
- J. O. Bustnes and K. E. Erikstad, The diets of sympatric wintering populations of Common Eider Somateria mollissima and King Eider S. spectabilis in Northern Norway, Ornis Fenn., 1988, 65, 163–168 Search PubMed.
-
The Institute of Marine Research, Theme: Blue Mussels, 2023, https://www.hi.no/hi/temasider/arter/blaskjell Search PubMed.
- J. Verreault, R. A. Villa, G. W. Gabrielsen, J. U. Skaare and R. J. Letcher, Maternal transfer of organohalogen contaminants and metabolites to eggs of Arctic-breeding glaucous gulls, Environ. Pollut., 2006, 144, 1053–1060 CrossRef.
- W. Jouanneau, D. J. Léandri-Breton, A. Corbeau, D. Herzke, B. Moe, V. A. Nikiforov, G. W. Gabrielsen and O. Chastel, A Bad Start in Life? Maternal Transfer of Legacy and Emerging Polyand Perfluoroalkyl Substances to Eggs in an Arctic Seabird, Environ. Sci. Technol., 2022, 56, 6091–6102 CrossRef.
- R. J. Norstrom, T. P. Clark, M. Enright, B. Leung, K. G. Drouillard and C. R. Macdonald, ABAM, a Model for Bioaccumulation of POPs in Birds: Validation for Adult Herring Gulls and Their Eggs in Lake Ontario, Environ. Sci. Technol., 2007, 41, 4339–4347 CrossRef PubMed.
- S. A. Hanssen, G. W. Gabrielsen, J. O. Bustnes, V. S. Bråthen, E. Skottene, A. A. Fenstad, H. Strøm, V. Bakken, R. A. Phillips and B. Moe, Migration strategies of common eiders from Svalbard: implications for bilateral conservation management, Polar Biol., 2016, 39, 2179–2188 CrossRef.
- C. Lydersen, I. Gjertz and J. M. Weslawski, Stomach contents of autumn-feeding marine vertebrates from Hornsund, Svalbard, Polar Rec., 1989, 25, 153 Search PubMed.
- F. Criscuolo, G. W. Gabrielsen, J. P. Gendner and Y. L. Maho, Body mass regulation during incubation in female common eiders Somateria mollissima, J. Avian Biol., 2002, 33, 83–88 CrossRef.
- J. O. Bustnes, B. Moe, D. Herzke, S. A. Hanssen, T. Nordstad, K. Sagerup, G. W. Gabrielsen and K. Borga, Strongly increasing blood concentrations of lipid-soluble organochlorines in high arctic common eiders during incubation fast, Chemosphere, 2010, 79, 320–325 CrossRef.
- M. Frederiksen, B. Moe, F. Daunt, R. A. Phillips, R. T. Barrett, M. I. Bogdanova, T. Boulinier, J. W. Chardine, O. Chastel, L. S. Chivers, S. Chistensen-Dalsgaard, C. Clément-Chastel, K. Colhoun, R. Freeman, A. J. Gaston, J. Gonzáles-Solís, A. Goutte, D. Grémillet, T. Guilford, G. H. Jensen, Y. Krasnov, S. H. Lorentsen, M. L. Mallory, M. Newell, B. Olsen, D. Shaw, H. Steen, H. Strøm, G. H. Systad, T. L. Thórarinsson and T. Anker-Nilssen, Multicolony tracking reveals the winter distribution of a pelagic seabird on an ocean basin scale, Divers. Distrib., 2012, 18, 530–542 CrossRef.
- J. O. Bustnes, B. J. Bardsen, B. Moe, D. Herzke, S. A. Hanssen, K. Sagerup, C. Bech, T. Nordstad, O. Chastel, S. Tartu and G. W. Gabrielsen, Temporal variation in circulating concentrations of organochlorine pollutants in a pelagic seabird breeding in the high Arctic, Environ. Toxicol. Chem., 2017, 36, 442–448 CrossRef.
- M. Vihtakari, J. Welcker, B. Moe, O. Chastel, S. Tartu, H. Hop, C. Bech, S. Descamps and G. W. Gabrielsen, Black-legged kittiwakes as messengers of Atlantification in the Arctic, Sci. Rep., 2018, 8, 1178 CrossRef.
-
NPI, Glaucous Gull (Larus Hyperboreus), 2022, https://www.npolar.no/en/species/glaucous-gull/ Search PubMed.
- J. O. Bustnes, K. E. Erikstad, V. Bakken, F. Mehlum and J. U. Skaare, Feeding Ecology and the Concentration of Organochlorines in Glaucous Gulls, Ecotoxicology, 2000, 9, 179–186 CrossRef.
- K. Breivik, J. M. Armitage, F. Wania, A. J. Sweetman and K. C. Jones, Tracking the Global Distribution of Persistent Organic Pollutants Accounting for E-Waste Exports to Developing Regions, Environ. Sci. Technol., 2016, 50, 798–805 CrossRef PubMed.
- M. J. Binnington and F. Wania, Clarifying relationships between persistent organic pollutant concentrations and age in wildlife biomonitoring: individuals, cross-sections, and the roles of lifespan and sex, Environ. Toxicol. Chem., 2014, 33, 1415–1426 CrossRef PubMed.
-
Norwegian Environment Agency, Water Environment Database, 2023, https://vannmiljo.miljodirektoratet.no/ Search PubMed.
- J. O. Bustnes, B. J. Bårdsen, B. Moe, D. Herzke, M. Ballesteros, A. Fenstad, K. Borgå, I. S. Krogseth, I. Eulaers, L. P. Skogeng, G. W. Gabrielsen and S. A. Hanssen, Impacts of a warming climate on concentrations of organochlorines in a fasting high arctic marine bird: direct vs. indirect effects?, Sci. Total Environ., 2024, 908, 168096 CrossRef PubMed.
-
R. H. Rodvelt, MSc Master's thesis, Norwegian University of Science and Technology, 2018.
-
NPI, Norwegian Polar Data Centre (NPDC), https://data.npolar.no/dataset.
- R. T. Barrett, J. U. Skaare and G. W. Gabrielsen, Recent changes in levels of Persistent Organochlorines and Mercury in eggs of Seabirds from the Barents Sea, Environ. Pollut., 1996, 92, 13–18 CrossRef PubMed.
-
C. Miljeteig and G. W. Gabrielsen, Contaminants in Black-Legged Kittiwake Eggs from Kongsfjorden, Barentsburg and Pyramiden, Report 1504-3215, Norwegian Polar Institute, 2009 Search PubMed.
- L. Li, C. Chen, D. Li, K. Breivik, G. Abbasi and Y. F. Li, What do we know about the production and release of persistent organic pollutants in the global environment?, Environ. Sci.: Adv., 2023, 2, 55 Search PubMed.
- H. Parker and H. Holm, Patterns of nutrient and energy expenditure in female common eiders nesting in the high Arctic, The Auk, 1990, 107, 660–668 CrossRef.
-
G. W. Gabrielsen, F. Mehlum, H. E. Karlsen, Ø. Andresen and H. Parker, Energy Cost during Incubation and Thermoregulation in the Female Common Eider (Somateria mollissima), Report 82-90307-79-9, Norwegian Polar Institute, 1991 Search PubMed.
- J. O. Bustnes, B. Moe, S. A. Hanssen, D. Herzke, A. A. Fenstad, T. Nordstad, K. Borga and G. W. Gabrielsen, Temporal dynamics of circulating persistent organic pollutants in a fasting seabird under different environmental conditions, Environ. Sci. Technol., 2012, 46, 10287–10294 CrossRef CAS PubMed.
-
IPCC, in Climate Change 2021 – the Physical Science Basis: Working Group I Contribution to the Sixth Assessment Report of the Intergovernmental Panel on Climate Change, 2023, pp. 1927–2058, DOI:10.1017/9781009157896.021.
- M. Iturbide, J. Fernández, J. M. Gutiérrez, A. Pirani, D. Huard, A. Al Khourdajie, J. Baño, J. Bedía, A. Casanueva, E. Cimadevilla, A. S. Cofiño, M. De Felize, J. Díez, M. García, J. Goldie, D. A. Herrera, S. Herrera, R. García and J. Milovac, Implementation of FAIR principles in the IPCC: the WGI AR6 Atlas repository, Sci. Data, 2022, 9, 629 CrossRef PubMed.
- A. Evenset, I. G. Hallanger, M. Tessmann, N. Warner, A. Ruus, K. Borga, G. W. Gabrielsen, G. Christensen and P. E. Renaud, Seasonal variation in accumulation of persistent organic pollutants in an Arctic marine benthic food web, Sci. Total Environ., 2016, 542, 108–120 CrossRef CAS.
- K. Borgå and A. Di Guardo, Comparing measured and predicted PCB concentrations in Arctic seawater and marine biota, Sci. Total Environ., 2005, 342, 281–300 CrossRef.
- J. M. Baert, C. R. Janssen, K. Borgå and F. De Laender, Migration and opportunistic feeding increase PCB accumulation in Arctic seabirds, Environ. Sci. Technol., 2013, 47, 11793–11801 CrossRef CAS PubMed.
- D. J. Léandri-Breton, W. Jouanneau, P. Legagneux, A. Tarroux, B. Moe, F. Angelier, P. Blévin, V. S. Bråthen, P. Fauchald, G. W. Gabrielsen, D. Herzke, V. A. Nikiforov, K. H. Elliott and O. Chastel, Winter Tracking Data Suggest that Migratory Seabirds Transport Per- and Polyfluoroalkyl Substances to Their Arctic Nesting Site, Environ. Sci. Technol., 2024, 58, 12909–12920 CrossRef.
- J. E. Baak, J. F. Provencher, M. L. Mallory and K. H. Elliott, Spatial ecotoxicology: What we know about the relationship between avian movements and contaminant levels, Environ. Rev., 2024, 32, 315–333 CrossRef.
- F. Wania and M. McLachlan, The Stockholm Convention at a Crossroads: Questionable Nominations and Inadequate Compliance Threaten Its Acceptance and Utility, Environ. Sci. Technol., 2024, 58, 13587–13593 CrossRef CAS.
- C. J. Fox, M. Taylor, M. Dickey-Collas, P. Fossum, G. Kraus, N. Rohlf, P. Munk, C. J. G. van Damme, L. J. Bolle, D. L. Maxwell and P. J. Wright, Mapping the spawning grounds of North Sea cod (Gadus morhua) by direct and indirect means, Proc. R. Soc. B, 2008, 275, 1642 Search PubMed.
- A. Sobek and Ö. Gustafsson, Latitudinal Fractionation of Polychlorinated Biphenyls in Surface Seawater along a 62° N–89° N Transect from the Southern Norwegian Sea to the North Pole Area, Environ. Sci. Technol., 2004, 38, 2746–2751 CrossRef CAS PubMed.
|
This journal is © The Royal Society of Chemistry 2025 |
Click here to see how this site uses Cookies. View our privacy policy here.