DOI:
10.1039/D4DT02101K
(Paper)
Dalton Trans., 2025,
54, 328-336
Water-soluble photocatalysts based on porphyrin-carbon dot conjugates produce H2 under visible light irradiation†
Received
22nd July 2024
, Accepted 1st November 2024
First published on 4th November 2024
Abstract
Herein, we report visible-light-induced hydrogen generation from aqueous protons utilizing a novel hybrid photocatalytic nanomaterial comprising porphyrin-carbon dot conjugates. Amide coupling between metallated tetra-carboxyphenyl porphyrins (MTCPPs) and nitrogen doped carbon dots (NCDots) was performed to afford M-TCPP-NCDots hybrids, which were applied in hydrogen evolution photocatalysis under visible irradiation. H2 was obtained in the presence of appropriate sacrificial electron donors and with no additional metallic co-catalysts. It is noteworthy that the covalent attachment as well as the zinc-metallation of the porphyrin moiety were proved vital for the efficiency of the present system. The present study constitutes an innovative approach for artificial photosynthesis avoiding the use of costly materials such as noble metals.
Introduction
The escalating demand for energy along with the environmental challenges resulting from the overexploitation of fossil fuels makes the exploitation of renewable energy sources imperative.1 Among these, solar energy stands out as the most promising alternative since it is abundant and environmentally friendly. Efficient and low-cost photocatalytic hydrogen production from water is a major focus of research toward solar energy conversion and storage.2 H2 is considered as a prospective fossil-fuel alternative due to its high energy density and the absence of harmful emissions, since the only product from its combustion is H2O.3 The use of hydrogen as an alternative fuel represents a longstanding strategy for globally reducing carbon dioxide emissions.4 Conventional approaches of hydrogen generation, like steam reforming of fossil fuels or electrolysis of water, involve substantial energy losses, resulting in low efficiency.5 Therefore, solar driven H2 evolution is the most appealing approach.6 In the pursuit of sustainable energy solutions, the exploration of novel molecules and materials for the development of efficient photocatalytic systems has become paramount. Among these materials, carbon dots (CDs), nitrogen-doped carbon dots (NCDots) and porphyrins have emerged as intriguing components in light-driven hydrogen production schemes.7 Most photocatalytic systems consist of three essential components: the photosensitizer (PS), the catalyst and the sacrificial electron donor (SED). Porphyrin derivatives have been employed in several artificial photosynthetic systems due to their easily modifiable structure, various interaction mechanisms with materials, and high molar absorption coefficient in the visible region.8 Owing to their efficient energy and/or charge-transfer capabilities, they are primarily used as PSs in various photocatalytic schemes.9,10 Moreover, porphyrins have also been employed as catalysts for the hydrogen evolution reaction (HER), as their aromatic macrocycle greatly enriches the redox chemistry of metal centers providing improved catalytic performance.11,12
Carbon dots and nitrogen-doped carbon dots represent a promising new class of low-cost carbon nanomaterials, gathering significant attention due to their unique optical, electronic, and surface area properties.13 CDs have recently been functionalized with porphyrin dyes either via non-covalent supramolecular interactions14,15 or through direct covalent linkages, resulting in the formation of hybrid nanomaterials with interesting properties.16,17 Furthermore, CDs can undergo charge-transfer reactions, acting as either electron donors or acceptors in their excited state. Based on this, CDs have shown great potential as PSs in photocatalytic H2 generation, in the presence of molecular catalysts.18–20 Additionally, CDs have been combined with several nanomaterials, such as CdS nanoparticles, acting as electron acceptors and suppressing photoexcited carrier recombination during light driven H2 formation.21 Notably, Guldi and co-workers prepared novel CDs with dual functionality, operating as photocatalysts.7 These CDs absorb light and produce H2 from water, without the need for an external PS or catalyst.
In this report, we covalently connected tetra-carboxy-substituted porphyrin derivatives (as a free base or metalated with Zn) to NCDots and the resulting hybrid nanomaterials (Scheme 1) were investigated for light induced H2 evolution. The present noble metal free photocatalysts demonstrated high activity in H2 production without the need for additional metallic co-catalysts and remained stable for several days under continuous light irradiation. It is important to note that this is the first example of NCDots operating as catalysts, in combination with a molecular PS in H2 evolving systems.
 |
| Scheme 1 Synthetic procedure for the synthesis of TCPP-NCDots and ZnTCPP-NCDots photocatalysts. | |
Results and discussion
Synthesis and characterisation
The porphyrin-NCDots conjugates were synthesized via amide coupling between carboxy-porphyrin derivatives (MTCPPs) and nitrogen-doped carbon dots (Scheme 1). Porphyrin precursor molecules TCPP and ZnTCPP were prepared according to published procedures.22NCDots were synthesized according to a simple bottom-up method using citric acid and ethylenediamine as carbon and nitrogen sources, respectively.18 The corresponding NCDots possess a variety of oxygen and nitrogen functional groups and in particular the presence of the amino groups enables their linkage with the carboxy-porphyrins. The final hybrid materials were fully characterized by NMR, XPS, IR, UV-Vis and fluorescence spectroscopy techniques as well as SEM. The successful covalent functionalization of the NCDots surface with porphyrins was verified initially by 1H NMR spectroscopy (Fig. S1–S5†). The porphyrin-based resonances were all present in the spectra of the hybrids (TCPP-NCDots and ZnTCPP-NCDots), along with signals expected for NCDots. Remarkably, in both hybrids (at around 10 ppm) we observed a signal that corresponds to the amide proton with an integration close to 1 in relation to the porphyrin β-pyrrolic peaks (integral equals 8). This proves the successful amide coupling and suggests that only 1 of the 4 carboxylic peripheral moieties of each porphyrin molecule is used for the coupling. Additional evidence for the successful covalent formation of hybrids was obtained from the Kaiser test (see the ESI for details†).23,24 From these measurements, we calculated the number of free (non-reacted) amino groups in TCPP-NCDots and ZnTCPP-NCDots (Fig. S6a–f and Table S1†) and in both cases a similar degree of functionalization (∼90%) was achieved. Scanning electron microscopy studies were performed to investigate the size and the morphology of the synthesized porphyrin-NCDots conjugates. As illustrated in Fig. 1, both TCPP-NCDots and ZnTCPP-NCDots hybrids aggregate into poorly formed spherical architectures with a diameter of ∼190 nm. This radius is increased compared to pristine NCDots (Fig. S6g†) and previous reports concerning similar materials.18
 |
| Fig. 1 Scanning electron microscopy images of (a) TCPP-NCDots and (b) ZnTCPP-NCDots, from aqueous solution after drop-casting onto a glass substrate. | |
Spectroscopic characterization (FT-IR and UV-visible)
FT-IR spectroscopy was performed to obtain further information about the surface functionalization of TCPP-NCDots and ZnTCPP-NCDots conjugate materials (Fig. S7†). In Fig. S7a,† the spectra of all compounds containing NCDots are presented. The broad bands at 3670–3160 cm−1 correspond to N–H and O–H vibrations, while the peak at 2940 cm−1 is attributed to C–H stretching. This is in accordance with previous reports on CDots prepared using citric acid as a carbon source.25,26 The absorption bands in the range of 1730–1630 cm−1 are attributed to the asymmetric stretching vibration of C
O groups and the peak at 1533 cm−1 originates from the N–H bending vibration. Based on the above observations, we conclude that the hybrid materials retain their characteristic NCDots-based features. Fig. S7b† highlights specific peaks to facilitate the comparison of vibrational peaks between precursor porphyrin molecules and porphyrin-NCDots. The intense peak at 1683 cm−1 in both TCPP and ZnTCPP corresponds to C
O stretching and is also dominant in the TCPP-NCDots and ZnTCPP-NCDots spectra, while the feature at 1636 cm−1 (C
O stretching) is mainly derived from the NCDots moiety. The peak at 1599 cm−1 is attributed to C
C/C
N stretches and is present in both the precursor porphyrins and the hybrid materials, but is absent in the non-functionalized NCDots. The signals in the range of 1425–1340 cm−1 are attributed to the sp3 C–H bending and symmetric stretching vibration of the carboxylate groups.27 Additionally, the evolution of new features in the range of 1230–1140 cm−1 in the spectra of both hybrids can be assigned to the O–H vibration of the carboxylate groups from the porphyrin moiety.
The UV-Vis absorption spectra of the synthesized conjugates demonstrated all characteristic peaks of the NCDots and porphyrin components (Fig. 2). In detail, the pristine NCDots presented an absorption band at 340 nm, which is attributed to the n → π* transition of the C
O bond.28 The absorption spectrum of the free base TCPP revealed the typical Soret-band at 416 nm and four Q-band absorptions at 512, 546, 590 and 646 nm. Similarly, the spectrum of the metalated ZnTCPP derivative exhibited the Soret band at 425 nm and two Q bands at 556 and 597 nm. The free base conjugate, TCPP-NCDots, displayed the broad peak of NCDots at 348 nm (red-shifted by 8 nm), along with the typical Soret band at 416 nm and Q bands at 512, 545, 589 and 646 nm, attributed to the porphyrin ring. In the case of the zinc metalated hybrid ZnTCPP-NCDots, the carbon-dot-based absorption band was observed at 346 nm, which is red-shifted by 6 nm compared to the pristine NCDots. In addition, the Soret and Q-band absorptions were also red-shifted to 430 nm and 561 and 601 nm, respectively. The observed red shift of the porphyrin absorption maxima could be attributed either to the close proximity between NCDots and ZnTCPP and their electronic communication in the ground state or to the formation of J-aggregates (side by side) of the porphyrin macrocycle moieties on the surface of the nanomaterial.29
 |
| Fig. 2 UV-Vis absorption spectra in DMSO of (a) NCDots, ZnTCPP and ZnTCPP-NCDots and (b) NCDots, TCPP and TCPP-NCDots. | |
Fluorescence and X-ray photoelectron spectroscopy studies
Fluorescence studies revealed that the emission of NCDots depends strongly on the excitation wavelength, as shown in Fig. 3a. This excitation–dependent behaviour is a well-known feature of carbon dot materials.30,31 In the pristine NCDots, the emission maximum was observed at 443 nm after excitation at 340 nm. The fluorescence spectra of the two hybrids TCPP-NCDots and ZnTCPP-NCDots displayed the characteristic wavelength-dependent NCDots-based emission, along with the porphyrin fluorescence bands at 651 nm and 715 nm for the free base material and 607 nm and 656 nm for the zinc derivative (Fig. 3b and c). Interestingly, the red shift of the NCDots-based emission maxima as we alter the excitation wavelength from 300 nm to 480 nm is more intense in the case of the two conjugates (111 nm and 120 nm) compared to the pristine NCDots (95 nm) (Fig. S8†). This observation indicates that the covalent coupling affects significantly the photophysical characteristics of the nanomaterial. On the other hand, the porphyrin-based emission is not excitation-dependent, as expected.
 |
| Fig. 3 Fluorescence emission spectra in DMSO of (a) NCDots, (b) TCPP-NCDots and (c) ZnTCPP-NCDots. | |
We proceeded to analyze the samples with X-ray photoelectron spectroscopy (XPS). Fig. S9† shows the C 1s peak of all the samples. The spectra were charge-referenced by setting the C–C peak at 248.8 eV. Upon initial examination, we observed a distinct difference in the peak shape between the NCDots and the porphyrins. The C 1s peak of the porphyrins exhibited a highly asymmetric profile, featuring a prominent peak corresponding to C–C bonds, as well as secondary features at higher binding energies attributed to carbon in functional groups containing oxygen and nitrogen. In contrast, the C 1s peak of the NCDots displayed a wider, asymmetric shape, suggesting a greater presence of carbon bonded to oxygen and nitrogen species. To further analyze the XPS data, we fitted the experimental points using peaks assigned to C–C, C–O/C–N, –C
O, –COO, and π–π* transitions at 284.8 eV, 286.4 eV, 288.1 eV, 289.2 and 291.4 eV, respectively. To quantitatively assess the atomic percentage concentration of carbon species, we compiled the fitting results in Table S2.† The C 1s spectra and atomic percentage of carbon–oxygen species for TCPP and ZnTCPP were consistent with expectations and aligned with the existing literature. It is important to note that adventitious carbon was present on the sample surfaces, contributing to a small extent to the C 1s and O 1s signals. Table S2† also reveals a high content of oxygen bonded to carbon in the NCDots, reaching up to 50%,32 while the porphyrin samples exhibited a high concentration of C–C content. The C–C content increases for the ZnTCPP-NCDots and TCPP-NCDots, which indicates the functionalization of NCDots by the porphyrins. Fig. S10† presents the N 1s spectra for all samples. In most spectra, a weak peak originating from the Mo 3p3/2 band of the Mo holder was observed at approximately 395 eV. The N 1s peak of the NCDots displayed a wide, symmetric shape, possibly attributed to different chemical states of N atoms that could not be resolved in our current XPS resolution. Conversely, the N 1s spectrum of TCPP exhibited the typical shape of porphyrins, with two resolved peaks representing different chemical environments of the N atoms in the porphyrin core. The lower binding energy peak at 398.2 eV was assigned to iminic (–N
) nitrogen, while the higher binding energy peak at 400.1 eV corresponded to pyrrolic (–NH) nitrogen.33,34 Notably, the N 1s peaks of the TCPP-NCDots and ZnTCPP-NCDots did not show any significant changes in the position of the peak and spectral shape compared to the N 1s peak of the NCDots, suggesting the presence of a thin film of porphyrins on the surface of the functionalized NCDots. Table S3† reports the binding energies of the N 1s peak for all the samples and of the Zn 2p3/2 peak. The N 1s of ZnTCPP is located at 398.6 eV in excellent agreement with similar Zn porphyrins.35 Fig. S11† displays the characteristic peaks of the metal in the porphyrin core. The peaks observed in the ZnTCPP-NCDots samples exhibit significantly lower intensity. The Zn 2p3/2 peak of ZnTCPP is located at 1022.2 eV, indicating the Zn2+ oxidation state.35,36
Photocatalytic hydrogen generation studies
Photocatalytic experiments were performed under visible light irradiation using 5 mg of each nanomaterial as a photocatalyst in aqueous solution with the use of diverse sacrificial electron donors (SEDs), namely tris(carboxyethyl)-phosphine/ascorbic acid (TCEP/Asc). The concentration of the TCEP/Asc 1
:
1 solution was 0.1 M and the pH was adjusted to 5. This SED mixture has previously presented enhanced catalytic efficiency towards H2 evolution, since TCEP regenerates oxidized ascorbic acid, thereby overcoming the instability of Asc and extending the lifetime of the system.18,19,37,38 Photocatalytic studies demonstrated that both porphyrin-NCDots conjugate nanomaterials are able to produce H2. On the other hand, NCDots alone as well as control experiments lacking the photocatalyst, or the SED, or light irradiation, displayed no H2 production. Moreover, the non-covalent combination (physical mixture) of either ZnTCPP or TCPP with NCDots did not present any H2 generation probably due to the insolubility of the porphyrin molecules in the acidic aqueous buffer solution. The later observation demonstrates the necessity of the covalent attachment to achieve photocatalytic H2 production in the present system. Upon optimization of the system, ZnTCPP-NCDots exhibited a maximum catalytic activity of 9 ± 1 mmol g−1 after 168 h of irradiation but had already reached 8.3 mmol g−1 after 72 h of irradiation (with a rate of 0.115 ± 0.02 mmol g−1 h−1) (Fig. 4). It is noteworthy that the free base conjugate presented negligible H2 evolution (0.2 ± 0.02 mmol g−1) compared to the Zn metalated nanomaterial.
 |
| Fig. 4 Photocatalytic hydrogen production plots of ZnTCPP-NCDots (black line), TCPP-NCDots (red line) and NCDots (blue line). The presented results are the average values of three independent measurements (within 10% error). | |
As demonstrated in Fig. 4, H2 evolution reaches a plateau after several hours of irradiation. The addition of SED does not regenerate the catalytic system but when the photocatalyst (ZnTCPP-NCDots) was added, the catalytic activity was effectively restored. This observation suggests that the porphyrin-NCDots nanomaterial decomposed after about 3 days of visible light irradiation. This observation was further supported by UV-Vis spectroscopy measurements, which showed that all porphyrin-based peaks had vanished after the catalysis (Fig. S12 and S13†).
In order to shed light on the catalytic mechanism of this system, we investigated the excited state of ZnTCPP-NCDots by selectively exciting the NCDots and the porphyrin constituents and comparing them with the NCDots and ZnTCPP references after adjusting to the same optical density (A = 0.1). Upon selective excitation of the NCDots moiety at 345 nm, a quantitative quenching of the NCDots fluorescence at 448 nm was observed in the ZnTCPP-NCDots hybrid (Fig. 5a), indicating a strong electronic interaction between the two components. In addition, when the porphyrin chromophore was selectively excited at 430 nm (S2 excited state), a significant fluorescence quenching was observed in the ZnTCPP-NCDots hybrid (Fig. 5b), possibly due to an electron transfer process from the ZnTCPP to the NCDots. Surprisingly, when the zinc porphyrin was selectively excited at 560 nm (S1 excited state), no significant quenching was revealed between ZnTCPP and ZnTCPP-NCDots (Fig. 5c). These findings are in strong agreement with the work of Arcudi et al.,39 who verified that the charge separated states of the porphyrin-NCDots conjugates are above the S1 and below the S2 porphyrin singlet excited states. Thus, the electron transfer from the zinc porphyrin to NCDots is possible only after excitation of the porphyrin to the S2 excited state. Lifetime decay fluorescence measurements (Fig. S14†) were also performed to study the electronic interactions of the two components in the ZnTCPP-NCDots hybrid. The pristine NCDots presented two lifetimes, τ1 = 3.7 ns (38%) and τ2 = 14.7 ns (62%). These values were calculated by using a double-exponential function for satisfactory data fitting. These measurements are consistent with the literature,40,41 since it is known that carbon dot materials show multiexponential decay of photoluminescence emission. ZnTCPP showed a mono-exponential decay with τ1 = 2.2 (100%) ns, which is a regular value for zinc metalated porphyrins. Interestingly, ZnTCPP-NCDots exhibited double-exponential decay with τ1 = 0.9 (28%) ns and τ2 = 5.1 (72%) ns. The short one (0.9 ns) is porphyrin based and is significantly quenched compared to the ZnTCPP. This finding further supports the strong electronic communication between ZnTCPP and NCDots in the excited state. Based on the above results, we propose that during the photocatalytic H2 evolution, after light excitation of the porphyrin moiety to the S2 excited state, electrons are injected towards the NCDot-moiety and aqueous protons are reduced to H2, while the SED mixture regenerates the oxidized photocatalyst (Fig. 6).
 |
| Fig. 5 Fluorescence emission spectra in DMSO solutions of (a) ZnTCPP-NCDots and NCDots upon excitation at 345 nm, (b) ZnTCPP-NCDots (concentration = 0.003 mg mL−1) and ZnTCPP (concentration = 0.26 × 10−6 M) upon excitation at 430 nm and (c) ZnTCPP-NCDots (concentration = 0.003 mg mL−1) and ZnTCPP (concentration = 0.26 × 10−6 M) upon excitation at 560 nm. | |
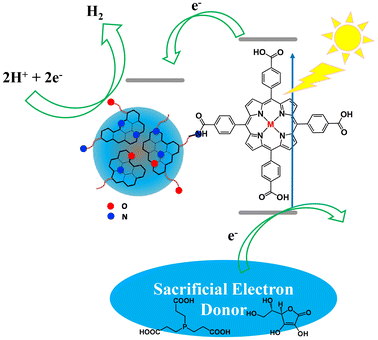 |
| Fig. 6 Proposed mechanism for H2 generation. | |
Conclusions
In summary, we synthesized porphyrin and nitrogen-doped carbon dot conjugates, covalently connected via amide coupling and investigated their photocatalytic ability for visible light driven H2 evolution ability in aqueous media. A mixture of TCEP and Asc was used as the sacrificial reagent, enabling prolonged H2 production. Importantly, the covalent connection between the porphyrin and NCDots was shown to be essential for efficient light driven H2 generation in this system. The ZnTCPP-NCDots photocatalyst, which demonstrated considerable stability, achieved an H2 production of 9 mmol g−1 after 168 h of visible light irradiation without the need for any additional co-catalyst. The highest production rate was 0.115 mmol·g−1·h−1, which, while modest, is comparable to the values reported in the literature for this type of material (Table 1). This work highlights the great potential of carbon-based nanomaterials combined with noble metal-free porphyrin dyes as efficient water-soluble photocatalysts for the HER.
Table 1 Comparison between the best carbon-dot-based systems in the literature and this work
Photocatalyst |
SED |
H2 production |
Ref. |
ZnTCPP-NCDots
|
TCEP/Asc |
0.115 mmol g−1 h−1 |
This work |
CQD-NiP |
EDTA |
0.398 mmol g−1 h−1 |
20
|
CQD/TiO2 |
TEOA |
0.472 mmol g−1 h−1 |
42
|
CQD/TiO2/Pt |
TEOA |
1.458 mmol g−1 h−1 |
42
|
Au/CQDs |
H2O/MeOH |
0.250 mmol g−1 h−1 |
43
|
Amino-conjugated CQDs |
Na2S/Na2SO3 |
0.273 mmol g−1 h−1 |
44
|
[ZnTMPyP]4+/MoS2/RGO |
TEOA |
2.56 mmol g−1 h−1 |
45
|
Experimental
Materials
All reagents were purchased from common commercial sources and used without any further purification, unless otherwise stated.
Nuclear magnetic resonance (NMR) spectroscopy
The porphyrin moieties were analyzed with 1H NMR spectroscopy using Bruker AMX-500 MHz and Bruker DPX-300 MHz spectrometers. All measurements were carried out at room temperature in a deuterated solvent using residual protons as an internal reference.
Ultraviolet-visible (UV-Vis) absorption spectroscopy
In all studies, a Shimadzu UV-1700 spectrometer was used. The reported experiments were performed using quartz cuvettes with a 0.2 cm path length.
Fluorescence emission spectroscopy
The emission spectra were recorded on a JASCO FP-6500 fluorescence spectrophotometer equipped with a red-sensitive WRE-343 photomultiplier tube (wavelength range: 200–850 nm).
Emission lifetime measurements
The emission lifetimes were determined by the time-correlated single-photon counting (TCSPC) technique using an Edinburgh Instruments mini-tau lifetime spectrophotometer equipped with an EPL 405 pulsed diode laser at 406.0 nm with a pulse width of 71.52 ps and a high-speed red-sensitive photomultiplier tube (H5773-04) as the detector.
X-ray photoelectron spectroscopy (XPS)
The XPS measurements were performed using a SPECS system equipped with a Phoibos 100 1D-DLD hemispherical energy analyzer. A nonmonochromatic Alkα X-Ray line was used to acquire the spectra. The pass energy was set at 30eV, and an electron flood gun was employed for charge compensation during the measurements.
Synthesis
Porphyrin precursor molecules TCPP and ZnTCPP were synthesized according to published experimental procedures.22
Synthesis of TCPP-NCDots.
5,10,15,20-Tetrakis-(4-(carboxy)-phenyl)-porphyrin (TCPP, 0.06 g, 0.076 mmol) was dissolved in dry DMF (6 mL) in a two-necked round-bottomed flask under a nitrogen atmosphere at room temperature. Then, 1-[Bis(dimethylamino)methylene]-1H-1,2,3-triazolo[4,5-b]pyridinium 3-oxide hexafluorophosphate (HATU, 0.12 g, 0.318 mmol) was added and after stirring for 5 minutes, N,N-diisopropylethylamine (DIPEA, 0.12 mL, 0.72 mmol) was introduced into the reaction mixture. At the same time, NCDots (0.12 g) were added to a Schlenk tube and dissolved in dry DMF (6 mL) and left for stirring, until they were fully dissolved. Then, the NCDots solution was added to the two-necked round-bottomed flask with the porphyrin solution and stirred under a nitrogen atmosphere for 72 hours. Then, the solvent was distilled under vacuum and the residue was centrifuged with an ethanol and water solution (1
:
1). The supernatant was collected, and the product was obtained after the distillation of the solvents. Recrystallization with ethanol–petroleum ether afforded the desired hybrid as a brown solid (0.1 g).
UV-Vis λmax, nm 350, 416, 512, 545, 589, 646.
Synthesis of ZnTCPP-NCDots.
[(5,10,15,20-Tetrakis-(4-(carboxy)-phenyl))-porphyrinato]-Zn (0.063 g, 0.07 mmol) was dissolved in dry DMF (6 mL) in a two-necked round-bottomed flask under a nitrogen atmosphere at room temperature. Then, HATU (0.12 g, 0.318 mmol) was added and after stirring for 5 minutes, DIPEA (0.12 mL, 0.72 mmol) was introduced into the reaction mixture. At the same time, NCDots (0.12 g) were added to a Schlenk tube and were dissolved in dry DMF (6 mL) and were left stirring, until they were fully dissolved. Then, the NCDots solution was added to the two-necked round-bottomed flask and stirred under a nitrogen atmosphere for 72 hours, and then the solvent was distilled. Then, the residue was centrifuged with an ethanol and water solution (1
:
1). The product that was present in the supernatant was collected and after the distillation of the solvents, it was recrystallized with ethanol and petroleum ether, dried and isolated as a brown solid (0.108 g).
UV-Vis λmax, nm 346, 430, 561, 602.
Quantitative Kaiser test protocol
The Kaiser test is a sensitive method for the qualitative and quantitative detection of free primary amino groups in non-functionalized NCDots along with the free-base and Zn-metalated hybrids (TCPP-NCDots and ZnTCPP-NCDots).24,46 The detection of primary free amino groups is based on their reaction with ninhydrin, which gives an intense blue colour to the solution with an absorption band at 570 nm.47 When the coupling between NCDots and porphyrins is successful, the colour of the solution remains light yellow and there is no significant change. This happens because the reaction between ninhydrin and secondary amines cannot take place.
The procedure involves the preparation of 3 solutions:
1. Solution 1: 10 g of phenol is dissolved in 20 mL of absolute ethanol.
2. Solution 2: 2 mL of KCN aqueous solution (1 mM) is added to 98 mL of pyridine.
3. Solution 3: 1 g of ninhydrin is dissolved in 20 mL of absolute ethanol.
Initially, 200 μg of NCDots, TCPP-NCDots and ZnTCPP-NCDots were added to three separate vials. Afterwards, 75 μL of solution 1, 100 μL of solution 2 and 75 μL of solution 3 were added to each vial. Then, each vial was heated in an oil bath at 120 °C for 5 minutes. Furthermore, 4.75 mL of ultrapure ethanol was added to each vial and as a result the final volume of each one was 5 mL. Finally, the UV-Vis spectrum of the supernatant from each one was recorded, with absorption at 570 nm related to the free primary amino groups.
The result is expressed in μmol of amino groups per g of material as follows:
where dilution is 5 mL, the extinction coefficient is 15
![[thin space (1/6-em)]](https://www.rsc.org/images/entities/char_2009.gif)
000 M
−1 cm
−1 and Abs
blank is the absorbance of the corresponding sample without ninhydrin.
Photocatalytic measurements
The photocatalytic H2 evolution studies were performed in glass vials (14 mL) sealed with a rubber septum, at ambient temperature and pressure. Before each experiment, a fresh buffer solution was prepared. More precisely, the buffer solution was a 0.1 M aqueous solution of tris(carboxyethyl)-phosphine/ascorbic acid (TCEP/Asc) in a 1
:
1 ratio and the pH was regulated to 5. The porphyrin-NCDots nanomaterial (5 mg) was added to a glass vial together with 3 mL of the buffer solution. In order to achieve anaerobic conditions, the suspensions were degassed using nitrogen for 5 min (in an ice/water bath). Finally, the samples were sealed with a silicon septum and were irradiated under continuous stirring with a low power white LED lamp ring of 40 W with a colour temperature of 6400 K and a lumen of 3800 LM (Fig. S15†). This set-up provided defined positions and a certain amount (50 W cm−2) of emitted light for all the vials simultaneously (Fig. S16†).
The amount of H2 produced in each sample vial was determined using a Shimadzu GC 2010 Plus chromatograph with a TCD detector and a molecular sieve 5 Å column (30 m–0.53 mm). For every measurement, 100 μL were taken from the headspace of the vial and were instantly injected into the GC. In all cases, the reported H2 production values are the averages of three independent experiments.
Calculation of H2 evolution μmol (H2) g−1 h−1
In every photocatalytic experiment, 0.005 g of porphyrin-NCDots photocatalyst were used. The H2 evolution was calculated according to the following equation:
where n(H2) is the total amount of the produced H2 (in μmol), m(photocatalyst) is 0.005 g, and t is the irradiation time in hours (t = 24 h).
Data availability
The data supporting this article have been included as part of the ESI.†
Conflicts of interest
There are no conflicts to declare.
Acknowledgements
This research has been co-financed by the European Union and Greek national funds through the Regional Operational Program “Crete 2014–2020”, project code OPS:5029187. Moreover, the European Union's Horizon Europe research and innovation programme under Grant Agreement No. 101119286 (Project: GIANCE) and the Special Research Account of the University of Crete are gratefully acknowledged for the financial support. E. N. gratefully acknowledges the Bodossaki Foundation for its financial support.
References
- M. S. Reza, N. B. H. Ahmad, S. Afroze, J. Taweekun, M. Sharifpur and A. K. Azad, Chem. Eng. Technol., 2022, 46, 420–434 CrossRef.
- U. M. Dankawu, H. Y. Hafeez, C. E. Ndikilar, J. Mohammed, A. B. Suleiman and A. S. Shuaibu, Int. J. Hydrogen Energy, 2024, 67, 1218–1242 CrossRef CAS.
- A. Le Goff, V. Artero, B. Jousselme, P. D. Tran, N. Guillet, R. Métayé, A. Fihri, S. Palacin and M. Fontecave, Science, 2009, 326, 1384–1387 CrossRef CAS PubMed.
- S. Cao and J. Yu, J. Photochem. Photobiol., C, 2016, 27, 72–99 CrossRef CAS.
- M. Aravindan and G. P. Kumar, Results Eng., 2023, 20, 101456 CrossRef.
- H. Song, S. Luo, H. Huang, B. Deng and J. Ye, ACS Energy Lett., 2022, 7, 1043–1065 CrossRef CAS.
- B. Jana, Y. Reva, T. Scharl, V. Strauss, A. Cadranel and D. M. Guldi, J. Am. Chem. Soc., 2021, 143, 20122–20132 CrossRef CAS PubMed.
- A. Charisiadis, E. Glymenaki, A. Planchat, S. Margiola, A.-C. Lavergne-Bril, E. Nikoloudakis, V. Nikolaou, G. Charalambidis, A. G. Coutsolelos and F. Odobel, Dyes Pigm., 2021, 185, 108908 CrossRef CAS.
- E. Nikoloudakis, I. López-Duarte, G. Charalambidis, K. Ladomenou, M. Ince and A. G. Coutsolelos, Chem. Soc. Rev., 2022, 51, 6965–7045 RSC.
- K. Ladomenou, M. Natali, E. Iengo, G. Charalampidis, F. Scandola and A. G. Coutsolelos, Coord. Chem. Rev., 2015, 304, 38–54 CrossRef.
- W. Zhang, W. Lai and R. Cao, Chem. Rev., 2017, 117, 3717–3797 CrossRef CAS PubMed.
- B. B. Beyene and C.-H. Hung, Coord. Chem. Rev., 2020, 410, 213234 CrossRef CAS.
- J. Liu, R. Li and B. Yang, ACS Cent. Sci., 2020, 6, 2179–2195 CrossRef CAS.
- V. Villari, M. Gaeta, A. D'Urso and N. Micali, Colloids Surf., A, 2022, 648, 129436 CrossRef CAS.
- A. Cadranel, V. Strauss, J. T. Margraf, K. A. Winterfeld, C. Vogl, L. Đorđević, F. Arcudi, H. Hoelzel, N. Jux, M. Prato and D. M. Guldi, J. Am. Chem. Soc., 2018, 140, 904–907 CrossRef CAS.
- J. R. A. Cosme, H. E. Bryant and F. Claeyssens, PLoS One, 2019, 14, e0220210 CrossRef.
- C. I. M. Santos, L. Rodríguez-Pérez, G. Gonçalves, S. N. Pinto, M. Melle-Franco, P. A. A. P. Marques, M. A. F. Faustino, M.Á Herranz, N. Martin, M. G. P. M. S. Neves, J. M. G. Martinho and E. M. S. Maçôas, Carbon, 2020, 166, 164–174 CrossRef CAS.
- K. Ladomenou, G. Landrou, G. Charalambidis, E. Nikoloudakis and A. G. Coutsolelos, Sustainable Energy Fuels, 2021, 5, 449–458 RSC.
- K. Ladomenou, M. Papadakis, G. Landrou, M. Giorgi, C. Drivas, S. Kennou, R. Hardré, J. Massin, A. G. Coutsolelos and M. Orio, Eur. J. Inorg. Chem., 2021, 2021, 3097–3103 CrossRef CAS.
- B. C. M. Martindale, G. A. M. Hutton, C. A. Caputo and E. Reisner, J. Am. Chem. Soc., 2015, 137, 6018–6025 CrossRef CAS PubMed.
- Y. Yu, Q. Zeng, S. Tao, C. Xia, C. Liu, P. Liu and B. Yang, Adv. Sci., 2023, 10, 2207621 CrossRef CAS.
- V. Nikolaou, E. Agapaki, E. Nikoloudakis, K. Achilleos, K. Ladomenou, G. Charalambidis, E. Triantafyllou and A. G. Coutsolelos, Chem. Commun., 2023, 59, 11256–11259 RSC.
- E. J. Pratt, E. I. Mancera-Andrade and K. L. Bicker, ACS Omega, 2022, 7, 36663–36671 CrossRef CAS PubMed.
- E. Kaiser, R. L. Colescott, C. D. Bossinger and P. I. Cook, Anal. Biochem., 1970, 34, 595–598 CrossRef CAS.
- S. N. Baker and G. A. Baker, Angew. Chem., Int. Ed., 2010, 49, 6726–6744 CrossRef CAS PubMed.
- S.-T. Yang, L. Cao, P. G. Luo, F. Lu, X. Wang, H. Wang, M. J. Meziani, Y. Liu, G. Qi and Y.-P. Sun, J. Am. Chem. Soc., 2009, 131, 11308–11309 CrossRef CAS PubMed.
- S. L. D'Souza, B. Deshmukh, J. R. Bhamore, K. A. Rawat, N. Lenka and S. K. Kailasa, RSC Adv., 2016, 6, 12169–12179 RSC.
- Z. Liang, L. Zeng, X. Cao, Q. Wang, X. Wang and R. Sun, J. Mater. Chem. C, 2014, 2, 9760–9766 RSC.
- E. Nikoloudakis, M. Pigiaki, M. N. Polychronaki, A. Margaritopoulou, G. Charalambidis, E. Serpetzoglou, A. Mitraki, P. A. Loukakos and A. G. Coutsolelos, ACS Sustainable Chem. Eng., 2021, 9, 7781–7791 CrossRef CAS.
- P. G. Luo, S. Sahu, S.-T. Yang, S. K. Sonkar, J. Wang, H. Wang, G. E. LeCroy, L. Cao and Y.-P. Sun, J. Mater. Chem. B, 2013, 1, 2116–2127 RSC.
- A. B. Bourlinos, A. Stassinopoulos, D. Anglos, R. Zboril, M. Karakassides and E. P. Giannelis, Small, 2008, 4, 455–458 CrossRef CAS.
- S. Zhu, Q. Meng, L. Wang, J. Zhang, Y. Song, H. Jin, K. Zhang, H. Sun, H. Wang and B. Yang, Angew. Chem., Int. Ed., 2013, 52, 3953–3957 CrossRef CAS.
- T. E. Shubina, H. Marbach, K. Flechtner, A. Kretschmann, N. Jux, F. Buchner, H.-P. Steinrück, T. Clark and J. M. Gottfried, J. Am. Chem. Soc., 2007, 129, 9476–9483 CrossRef CAS PubMed.
- R. Zanoni, A. Aurora, F. Cattaruzza, F. Decker, P. Fastiggi, V. Menichetti, P. Tagliatesta, A. L. Capodilupo and A. Lembo, Mater. Sci. Eng., C, 2007, 27, 1351–1354 CrossRef CAS.
- M. Tountas, A. Verykios, E. Polydorou, A. Kaltzoglou, A. Soultati, N. Balis, P. A. Angaridis, M. Papadakis, V. Nikolaou, F. Auras, L. C. Palilis, D. Tsikritzis, E. K. Evangelou, S. Gardelis, M. Koutsoureli, G. Papaioannou, I. D. Petsalakis, S. Kennou, D. Davazoglou, P. Argitis, P. Falaras, A. G. Coutsolelos and M. Vasilopoulou, ACS Appl. Mater. Interfaces, 2018, 10, 20728–20739 CrossRef CAS.
- K. Flechtner, A. Kretschmann, L. R. Bradshaw, M.-M. Walz, H.-P. Steinrück and J. M. Gottfried, J. Phys. Chem. C, 2007, 111, 5821–5824 CrossRef CAS.
- B. C. M. Martindale, E. Joliat, C. Bachmann, R. Alberto and E. Reisner, Angew. Chem., Int. Ed., 2016, 55, 9402–9406 CrossRef CAS.
- S. Schnidrig, C. Bachmann, P. Müller, N. Weder, B. Spingler, E. Joliat-Wick, M. Mosberger, J. Windisch, R. Alberto and B. Probst, ChemSusChem, 2017, 10, 4570–4580 CrossRef CAS PubMed.
- F. Arcudi, V. Strauss, L. Đorđević, A. Cadranel, D. M. Guldi and M. Prato, Angew. Chem., Int. Ed., 2017, 56, 12097–12101 CrossRef CAS.
- F. D'Souza, S. Gadde, M. E. Zandler, K. Arkady, M. E. El-Khouly, M. Fujitsuka and O. Ito, J. Phys. Chem. A, 2002, 106, 12393–12404 CrossRef.
- P. M. Gharat, J. M. Chethodil, A. P. Srivastava, P. K. Praseetha, H. Pal and S. D. Choudhury, Photochem. Photobiol. Sci., 2019, 18, 110–119 CrossRef CAS.
- I. Sargin, G. Yanalak, G. Arslan and I. H. Patir, Int. J. Hydrogen Energy, 2019, 44, 21781–21789 CrossRef CAS.
- A. Mehta, D. Pooja, A. Thakur and S. Basu, New J. Chem., 2017, 41, 4573–4581 RSC.
- X. Xu, Z. Bao, G. Zhou, H. Zeng and J. Hu, ACS Appl. Mater. Interfaces, 2016, 8, 14118–14124 CrossRef CAS.
- Y. J. Yuan, D. Chen, J. Zhong, L. X. Yang, J. J. Wang, Z. T. Yu and Z. G. Zou, J. Phys. Chem. C, 2017, 121, 24452–24462 CrossRef CAS.
- C. Ménard-Moyon, C. Fabbro, M. Prato and A. Bianco, Chem. – Eur. J., 2011, 17, 3222–3227 CrossRef.
- S. L. Pilicer and C. Wolf, J. Org. Chem., 2020, 85, 11560–11565 CrossRef CAS.
|
This journal is © The Royal Society of Chemistry 2025 |
Click here to see how this site uses Cookies. View our privacy policy here.