DOI:
10.1039/D4CS00029C
(Review Article)
Chem. Soc. Rev., 2025,
54, 2054-2090
Influence of π–π interactions on organic photocatalytic materials and their performance†
Received
31st August 2024
First published on 24th January 2025
Abstract
Currently, organic photocatalyst-based photocatalysis has garnered significant attention as an environmentally friendly and sustainable reaction system due to the preferable structural flexibility and adjustable optoelectronic features of organic photocatalysts. In addition, π–π interactions, as one of the common non-bonded interactions, play an important role in the structure and property adjustments of organic photocatalysts due to their unique advantages in modulating the electronic structure, facilitating charge migration, and influencing interfacial reactions. However, studies summarizing the relationship between the π–π interactions of organic photocatalysts and their photocatalytic performance are still rare. Therefore, in this review, we introduced the types of π–π interactions, characterization techniques, and different types of organic photocatalytic materials. Then, the influence of π–π interactions on photocatalysis and the modification strategies of π–π interactions were summarized. Finally, we discussed their influence on photocatalytic performance in different photocatalytic systems and analyzed the challenges and prospects associated with harnessing π–π interactions in photocatalysis. The review provides a clear map for understanding π–π interaction formation mechanism and its application in organic photocatalysts, offering useful guidance for researchers in this field.

Liquan Jing
| Liquan Jing earned his PhD in Environmental Science and Engineering from Jiangsu University in 2021. He is now a postdoctoral researcher in the Department of Chemical and Petroleum Engineering at the University of Calgary, working under the guidance of Prof. Jinguang Hu and Prof. Ian D. Gates. His research primarily focuses on designing and synthesizing functional photocatalysts for sustainable hydrogen production, converting biomass into high-value-added bioproducts, and methane conversion. |

Pandeng Li
| Pandeng Li earned his PhD from the Institut national de la recherche scientifique (INRS) in Canada in 2021. He is currently a postdoctoral researcher in Prof. Jinguang Hu's research group in the Department of Chemical and Petroleum Engineering at the University of Calgary. His research primarily focuses on developing large-scale reactors for biomass photoreforming, aiming to concurrently produce valuable chemicals and sustainable hydrogen. |

Zheng Li
| Zheng Li earned his PhD from the Dalian Institute of Chemical Physics and is currently an Eyes High Postdoctoral Research Fellow in the Department of Chemical and Petroleum Engineering at the University of Calgary, under the guidance of Dr Jinguang Hu and Dr Zhangxin (John) Chen. His research primarily focuses on the photo(electro/piezo/bio)-catalytic conversion of biomass-derived and energy-related molecules for the production of sustainable hydrogen and value-added chemicals. |

Dongling Ma
| Prof. Dongling Ma holds the Canada Research Chair in Advanced Functional Nanocomposites and serves as a full professor at the Center of Energy, Materials and Telecommunications, INRS. She earned her PhD in materials science and engineering from Rensselaer Polytechnic Institute (USA) in 2004. From 2004 to 2006, she was awarded Natural Sciences and Engineering Research Council Visiting Fellowships and worked at the National Research Council of Canada. Her primary research focus is on developing various nanomaterials including semiconductor quantum dots, transition metal catalytic nanoparticles, and plasmonic nanostructures, as well as different types of nanohybrids for applications in the energy, catalysis, and biomedical sectors. |

Jinguang Hu
| Prof. Jinguang Hu is an Associate Professor and Schulich Research Chair at the University of Calgary. His current research focuses on biomass valorization, sustainable energy and bioinspired materials/systems for Energy, Environmental and Biomedical application. He has received the Chemical Institute of Canada's Bao and Zhu Innovation Award, presented annually to a single early-career researcher across Canada for distinguished contributions to chemical engineering; the Schulich Early Research Excellence Award; and the S.C. Trindade Award. He is an External Advisory Board member of the Canada Biomass Energy Network, a member of the “China-Canada Joint Centre for BioEnergy”, a Research Fellow of Grizzly Bear Institute of Canada (GBIC), and serves on the editorial boards of several journals. |
1. Introduction
Photocatalysis driven by solar energy is a promising future technology for energy transformation, storage and environmental restoration, including H2 generation, CO2 reduction, organic pollutant degradation, and N2 fixation.1–4 The key to the development and eventual practical implementation of photocatalysis technology is the design of novel, high-performance photocatalysts, which should possess strong photo-redox ability, efficient charge migration, rapid surface reactions, high selectivity and durability.5–10 Over the last two decades, different types of photocatalytic materials have been developed. Inorganic semiconductor nanomaterials are promising photocatalysts, including oxide-based photocatalysts (TiO2, ZnO, SrTiO3, BiVO4, etc.), chalcogenide-based photocatalysts (ZnS, CdS, CdSe, MoS2, Zn1−xCdxS (0.2 ≤ x ≤ 1), ZnIn2S4, AgInS2, etc.), and nitride-based photocatalysts (GaN and Ta3N5).11–14 To further improve photocatalytic performance, the size, shape, and doping of inorganic photocatalysts are optimized, and a co-catalyst strategy is also applied.13,15–21 Additionally, another type of photocatalyst is based on organic materials with a delocalized π-electron system, such as covalent organic frameworks (COFs), metal–organic frameworks (MOFs), organic small-molecule compounds, conjugated polymers, carbon-based materials, and other aromatic compounds (Fig. 1a).22–38 The conjugated (or aromatic) photocatalytic materials exhibit structural flexibility and precise tuning of optoelectronic features. Fig. 1b shows that the number of publications related to π–π interactions in photocatalysis (mainly photocatalysts) has increased exponentially since 2002, reflecting the increasing importance of applying π–π interactions in photocatalysis.
 |
| Fig. 1 (a) Photocatalysts involving π–π interactions. (b) Yearly publications on π–π interactions (including the catalyst itself, between different catalysts, and between the catalyst and the substrate) for photocatalytic applications based on keyword search of “π–π” and “photocata*” in the Web of Science (as of September 1, 2024). | |
In addition, another important feature of the π–π interactions arises from the strong dispersion and dipole–dipole interactions when the planes of aromatic rings are stacked on one another.39–41 These interactions can be classified into different groups based on the geometric arrangement of stacked aromatic rings, and typically exist in conjugated intermolecular interactions or between conjugated molecules and reactant organic molecules. The former can influence the crystallization properties, optical properties, electronic properties, and stability of organic conjugated photocatalytic materials. In contrast, the latter can alter charge transfer between conjugated photocatalytic molecules and reactant organic molecules, ultimately influencing the corresponding photocatalytic reaction.42–44 Hence, π–π interaction as an important factor must be carefully considered when designing novel conjugated photocatalysts and optimizing the related photocatalytic performance. To date, the related reviews about the influence of π–π interactions on conjugated photocatalysts and photocatalytic performance are still rare. Therefore, it is of great significance to summarize the influences of π–π interactions in different conjugated (or aromatic) photocatalysts on photocatalytic performance in particular, which can provide a direction for researchers in this aspect.
In order to understand the π–π interactions of conjugated (or aromatic) photocatalysts in depth, this article first provides an introduction to the types of π–π interactions and the characterization methods, and then summarizes, in turn, different types of photocatalytic materials with π–π interactions and the influences of π–π interactions on their photocatalytic performance. Further, we discuss the modification strategies of π–π interactions to improve the photocatalytic performance and their applications and advantages in various photocatalytic reactions. Lastly, the challenges of π–π interactions in photocatalytic systems and the potential for subsequent development are discussed.
2. Characteristics of π–π interactions
2.1. Definition and geometric configurations of π–π interactions
In recent years, noncovalent interactions (π–π interactions, hydrogen bonds, van der Waals forces, ionic interactions, etc.) have attracted widespread attention, particularly in chemistry, biology, and materials sciences.45 Specifically, the noncovalent interactions enhance the adsorption capacity, durability and activity of the catalyst by enhancing the interaction between the reactant and the catalyst, while affecting the adsorption configuration of the reactant, optimizing the stability of the catalyst, and enhancing the catalytic efficiency and selectivity.46 Among them, π–π interactions mainly caused by intermolecular orbital overlap are most prominent in aromatic systems. They exist mainly between benzene rings, and their energy magnitudes range from about 1 to 50 kJ mol−1, with the majority around and below 10 kJ mol−1.39 Their magnitude and direction depend on the intermolecular distances and their dihedral angles. Based on the electrostatic interaction of σ backbone and π electrons, Christoph Janiak's research proposed that the two aromatic ring planar spacings around 3.3 Å constitute a strong π–π interaction, while 3.6–3.8 Å is a weak interaction.47 In addition, the common π–π interactions are divided into four types, as shown in Fig. 2:39,48,49 (a) T-shaped stacking (two aromatic systems are perpendicular to each other, with the point of the aromatic ring opposite to the face of the other aromatic ring); (b) edge-to-face stacking (the two aromatic systems are perpendicular to each other, with the side of the aromatic ring facing the face of the other aromatic ring); (c) offset face-to-face stacking (the two aromatic structures are essentially parallel, but there is a mismatch); and (d) face-to-face stacking (the two aromatic structures are basically parallel, with no dislocation and perpendicular to each other).
 |
| Fig. 2 Schematic of different geometries of interactions between aromatic rings and their corresponding electrostatic interaction diagrams (taking benzene rings as an example): (a) T-shaped, (b) edge-to-face, (c) offset and (d) face-to-face. | |
Furthermore, the strength and physicochemical characteristics of π–π interactions are influenced by the molecular structure and geometric arrangement, size and shape of π-electron clouds, molecular spacing, environmental conditions, molecular aromaticity, and multifarious substituents.39,41,48,50 For effective π–π interactions, the spatial arrangement and geometry of molecules must allow for optimal overlap of π-electron clouds, forming stable interactions. Generally, larger π-electron clouds result in stronger interactions due to their increased overlap and attraction capabilities. In addition, the interaction is more robust when the molecular spacing is approximately 3–4 Å, which facilitates optimal π-electron cloud overlap. Moreover, environmental conditions such as solvent polarity, solubility, temperature, and pressure can significantly affect the formation and stability of π–π interactions. Non-polar solvents and lower temperatures are typically favorable for forming π–π interactions.51–53 Additionally, both the molecule aromaticity and the electronic and steric effects of substituents play crucial roles in determining the strength of π–π interactions. These factors collectively determine the characteristics and potential applications of π–π interactions in the photocatalysis field.
2.2. Analytical methods for π–π interactions
The verification of π–π interactions can be accomplished through both theoretical calculations and experimental methods, with single-crystal X-ray diffraction standing out as an effective technique due to its direct and straightforward approach.40 This method, when coupled with theoretical analysis, allows for the precise measurement of dihedral angles and intermolecular distances, which are critical for identifying the stacking modes of molecules within crystals. Through detailed examination of the diffraction data, not only can the strength of π–π interactions be assessed, but also different stacking arrangements, such as face-to-face or face-to-edge, can be distinguished.39 Overall, single-crystal X-ray diffraction serves as a valuable tool in gaining a comprehensive understanding of π–π stacking interactions and their characteristics. For example, Yang et al. synthesized three COFs with varying structures (COF-TAPB-BPDA, TAPB: 1,3,5-tris(4-aminophenyl)benzene; BPDA: 4,4′,4′′-(benzene-1,3,5-triyltris(ethyne-2,1-diyl))tris(2-hydroxybenzaldehyde); COF-TAPT-BPDA, TAPT: 1,3,5-tris(4-aminophenyl)triazine; COF-TAPP-BPDA, TAPP: 5,5′,5′′-(1,3,5-triazine-2,4,6-triyl) tris(pyridin-2-amine)) and characterized their crystal structures through powder X-ray diffraction (PXRD) analysis and theoretical simulations.54 The broad (001) diffraction peak of COF-TAPB-BPDA at 24.78° suggests a typical interlayer π–π stacking distance of less than 3.5 Å, indicative of strong π–π interactions. In contrast, the sharper (001) diffraction peaks of COF-TAPT-BPDA and COF-TAPP-BPDA at 25.96° reflect their higher crystallinity and relatively weak π–π interactions (Fig. 3a–c). Additionally, using the Forcite module of Materials Studio software, they simulated and optimized the stacking modes of these COFs. The experimental PXRD patterns closely matched the simulated ones, confirming that the stacking distance in COF-TAPB-BPDA is smaller than that in the other two COFs (Fig. 3d–f). In addition, the π–π interaction stacking pattern can likewise be determined by a grazing-incidence wide-angle X-ray scattering (GIWAXS) characterization method. Liu et al. explored three molecular structures (C0IPDI, C2IPDI, and C3IPDI) using perylene diimide (PDI) as the building unit, focusing on the effects of varying alkyl chain lengths between imidazole (IMZ) and PDI on molecular and π–π stacking (Fig. 3g).22 GIWAXS images revealed clear π–π stacking in the protonated thin films. The study concentrated on the structure–activity relationship concerning π–π stacking interactions. Directly connected IMZ and PDI resulted in a π–π stacking distance of 3.42 Å; introducing an ethyl group reduced this distance to 3.19 Å, leading to herringbone stacking in C2IPDI, which enhances charge transport. The insertion of an n-propyl group in C3IPDI led to non-parallel molecular alignment, with π–π stacking distances ranging from 3.33 to 3.51 Å, and a zigzag stacking pattern where the IMZ and PDI are further apart. The study concluded that variations in the σ-bond length effectively regulate the molecular stacking patterns and π–π stacking distances in CnIPDI molecules. Furthermore, ultraviolet photoelectron spectroscopy (UV) and fluorescence spectrophotometry are two methods for detecting molecular π–π interactions.39,55,56 The conjugation effect causes a red shift in the UV spectrum because the conjugated system has a lower transition energy, which shifts the absorption peak to a longer wavelength.57,58 The positive ion stacking of planar molecules promotes molecular aggregation. In fluorescence spectrophotometry, the energy transfer caused by molecular stacking leads to fluorescence quenching, reducing the fluorescence intensity.59–61 Overall, with the eventual development of more advanced spectroscopy, it is expected that more characterization methods will emerge that can clearly reveal π–π interactions in materials.
 |
| Fig. 3 (a)–(c) PXRD patterns of several different COF materials, where the experimental results are indicated in red lines, the refined profiles are in black, the computationally simulated patterns are in blue (indicating the overlap (AA) stacking mode), the Bragg position in green color, and the difference between the experimental and refined PXRD patterns in dark cyan color. ((d)–(f) Top and side views corresponding to the above three structures showing perfect overlapping (AA) structures (red, gray, white, and blue spheres) representing O, C, H, and N atoms, respectively). ((a)–(f) Reproduced from ref. 54 with permission from the American Chemistry Society, Copyright 2023). (g) Molecular structural formulae of the three modified PDI materials and their corresponding grazing-incidence wide-angle X-ray scattering (GIWAXS) 2D images of spin-coated protonated films. ((g) Reproduced from ref. 22 with permission from Wiley-VCH, Copyright 2023). | |
3. Conjugated (or aromatic) photocatalytic materials with π–π interactions
To date, various organic photocatalytic materials with π–π interactions have been developed (Fig. 4), such as organic framework materials (COFs and MOFs), π-conjugated organic small molecules (PDI, phthalocyanines, porphyrins, and complexes), polymers (carbon nitride and others) and carbon-based materials (graphenes, graphene oxides, carbon nanotubes, graphite, graphdiyne, biochar, etc.). As mentioned above, ideal photocatalysts need to have strong visible light absorption, high carrier separation efficiency, high stability, large specific surface area, and tunable structure. First, dye molecules (phthalocyanines, porphyrins, etc.) appeared in the spotlight because of their good absorption and π-structures. Traditional polymeric materials with π-structures (polyaniline, polypyrrole, etc.) were also used in photocatalysis because of their good conductivity and light absorption. Afterwards, carbon materials are widely utilized in photocatalytic systems due to their robust sp2 lattice structure, extensive conjugated systems, excellent physicochemical stability, and strong adsorption capacity. Since 2012, traditional MOF-based framework materials have also been utilized, primarily owing to their intricate pore structures and the high capacity for ligand modulation. Following this, g-C3N4 and PDI, because of their unique structures, have attracted attention; their ability to readily create an internal electric field and excellent visible-light responsiveness has gained growing interest. In recent years, the ongoing achievements in computational chemistry, spectroscopic methods, and synthetic techniques have steered researchers' interest towards organic frameworks, including new π-conjugated polymer materials, COFs, and innovative MOFs. This interest stems from their abundant, regularly arranged pores and easily modified structures. Throughout the evolutionary journey of photocatalysts that leverage π–π interactions, there has been a transition from simpler to more complex structures. This progression has been accompanied by increased structural tunability and designability, which facilitates the construction of active sites and charge separation, the fine-tuning of microenvironments, and the optimization of catalysts. Here, we focus on four widely used classes of materials: organic framework materials, organic conjugated small molecules, conjugated polymers, and carbon materials. Leveraging their distinct structural attributes, our ultimate goal is to provide a guideline for designing and fabricating high-performance, ideal photocatalysts that capitalize on π–π interactions. These photocatalysts will be characterized by their tunable absorption and emission spectra, elevated extinction coefficients, superior charge-transport capabilities, facile modifiability, and durability. Such features render them compelling and cutting-edge materials for a broad spectrum of photocatalytic applications. Additionally, Fig. 5 illustrates the distribution of materials featuring π–π interactions within the field of photocatalysis. Conjugated polymers, carbon materials, organic framework materials, and small organic conjugated molecules represent the principal materials utilized in photocatalytic applications. Other materials featuring π–π interactions require further exploration and development to enhance their potential in photocatalysis.
 |
| Fig. 4 Development history of materials with π–π interactions used in photocatalysis. | |
 |
| Fig. 5 Proportional distribution of various photocatalytic materials capable of forming π–π interactions. | |
3.1. Organic framework materials
3.1.1 Covalent organic frameworks (COFs).
COFs are highly crystalline organic polymers formed by specific organic units linked by covalent bonds (e.g., cyclic boroxanes, imines, hydrazines, boronic esters, acridines, or ketene amines), which can be constructed as two- or three-dimensional structures.62 COFs exhibit high thermal and chemical stability, extensive porosity, and large surface areas.62,63 Additionally, their π-conjugated structures and π–π stacked layers enhance their ability to absorb a broad range of visible light and facilitate efficient charge separation.62,64,65 At present, the research on COF materials is primarily focused on two-dimensional structures. These two-dimensional COFs are structured and crystallized via interlayer π–π stacking interactions within a planar network created by rigid building units.66,67 The synthesis of these two-dimensional COFs involves using building blocks with varying symmetries and molecular sizes to create monolayers of diverse macrocyclic shapes and sizes.68,69 These molecular layers stack parallel to each other, guided by the principle of maximum π orbital overlap.66 This involves the overlap of interlayer π–π electron clouds and conjugated connections within each layer, which facilitates a periodic distribution of pores and supports rapid carrier migration within the framework.66 Additionally, the stability of two-dimensional COFs is enhanced by a combination of intralayer covalent bonds and interlayer non-covalent forces such as π–π interactions, hydrogen bonds, and van der Waals forces.70 By adjusting the molecular structure and optoelectronic properties of two-dimensional COFs, the π–π interactions are enhanced, and structurally functionalized photocatalysts with customized properties can be developed.71,72 This is primarily because π–π interactions influence several properties of COFs, such as charge transport properties, structural stability, crystallinity, morphology, adsorption capacity, and reaction selectivity.73–76 These effects can enhance the performance of COF-based photocatalysts. Consequently, researchers can effectively design and fine-tune the π–π interactions within COFs to optimize their specific photocatalytic performance by selecting appropriate building blocks, functional groups, and synthetic conditions. Among the many above-mentioned COF structures, imine COFs have become the most widely used COF materials due to their ultra-high specific surface area, nano-sized pores, high porosity and excellent physical and chemical stability.77 For example, Li et al. created a novel imine COF material through a solvothermal method and combined it with membrane PVDF for photocatalytic organic synthesis reactions.78Fig. 6a demonstrates the combination of a charge acceptor (naphthalene diimide (NDI)) and a charge donor (tetrakis(4-formylphenyl)pyrene (TFPPy)) constructed as DTF-ANDI-COF by Schiff base condensation. It is also demonstrated that DTF-ANDI-COF is a typical D–A-COF structure, which is a molecular structure with obvious H-kin and strong π–π interactions. Subsequently, they designed membrane-modulated catalysis to extend the existing catalytic advantages of stabilized DTF-ANDI-COF. The reaction performance of DTF-ANDI-COF/PVDF membranes selectively promoting amine-coupled catalysis, and from the results, the yields of the products of amine-coupled DTF-ANDI-COF/PVDF were all above 90%, which was mainly owing to the substantial π–π interactions formed within the structure of DTF-ANDI-COF constructing the charge transfer dynamics and the reactive oxygen radical's generation.
 |
| Fig. 6 (a) Preparation route of the novel COF and its structural features. ((a) Reproduced from ref. 78 with permission from Wiley-VCH, Copyright 2023). (b) Physical roadmap for the preparation of ZIF-8-Pp and the schematic of the large-scale and extensive built-in electric field that will be formed between the two through π–π interaction and the corresponding effect manifestation. ((b) Reproduced from ref. 79 with permission from the American Chemistry Society, Copyright 2022). | |
3.1.2. Metal–organic frameworks (MOFs).
Unlike COF-based photocatalysts, MOFs represent a distinct class of nanoporous inorganic–organic hybrid materials.80 These are formed from inorganic metal ions or clusters interconnected by organic ligands, available in one-dimensional, two-dimensional, or three-dimensional topological structures.80–82 Among these, three-dimensional MOF materials are particularly valued in practical applications due to their versatile and porous nature.83 This is mainly because the spatial 3D network formed by this type of material has the characteristics of high specific surface area, various structures, tunable pore shape and size, and modifiable pore surface.83–85 By varying the structural units and synthesis methods, it is possible to tailor the functional groups, pore dimensions, and overall size of the MOFs to specific needs.83 Furthermore, MOFs enhance the flexibility and practicality through assorted weak interactions (π–π stacking, electrostatics, hydrogen bonding, etc.) and coordination bonds.86 Among them, the interaction formed by π–π stacking has received extensive attention. The conductivity and charge transfer efficiency of MOFs are enhanced by regulating the π–π stacking distance between electron-rich organic linkers (commonly using various planar aromatic cores such as naphthalene, pyrazine, naphthalene diimide, triphenylene, anthracene, and tetrathiafulvalene).86 Moreover, π–π stacking not only alters MOFs' structural features such as porosity and packing density but also creates a continuous pathway for efficient charge transfer.86 By selecting ligands with specific aromatic or conjugated structures and optimizing synthesis conditions, researchers can effectively control and design π–π interactions in MOFs, paving the way for substantial enhancements in photocatalytic performance. Zeolitic imidazolate framework-8 (ZIF-8) is a widely reported MOF composed of 2-methylimidazole and Zn, showcasing the inherent structural benefits of MOFs along with enhanced thermochemical stability.87 Unlike many other MOFs, ZIF-8 is not only straightforward to synthesize but also retains its crystallinity and porosity after exposure to various solutions and remains stable up to 500 °C. Its surface active sites and effective charge separation attributes confer outstanding adsorption capacities and significant photocatalytic potential.87 Furthermore, the π–π stacking interactions between the imidazole rings in ZIF-8 and aromatic ring-containing substances serve to further augment its photocatalytic capabilities.88–90 Shi et al. constructed a complex of porphyrin and ZIF-8 (ZIF-8-Pp) for the photolysis of organic pollutants and transformation of organic molecules by self-assembly.79Fig. 6b demonstrates the assembly process of ZIF-8 and 5,10,15,20-tetrakis(4-pyridyl)porphyrin (Pp), which is mainly owing to the substantial π–π interactions between Pp and ZIF-8, which results in a substantial built-in electric field's constitution, and ultimately, enhanced light absorption (antennae effect of porphyrin), efficient charge migration and high concentration of reactive oxygen radicals. As a result, the ZIF-8-Pp can efficiently convert phenylboronic acid and its congeners to the corresponding phenolics with yields of around 70%, and the study of the reaction process reveals that the photocarriers in Pp are excited under visible light, and then migrate to multiple metal nodes in ZIF-8 to form superoxide radicals, and the photogenerated holes resting on the Pp molecule oxidize the triethylamine molecule (TEA) on the Pp molecule to form TEA+, which subsequently generates intermediates that are hydrolyzed to give the final phenol product. The above-mentioned results show that the substantial π–π interaction between Pp and ZIF-8 creates a built-in electric field that strongly pushes photocarrier migration, ultimately promoting the efficient utilization of solar energy.
3.1.3. Hydrogen-bonded organic frameworks (HOFs).
Hydrogen-bonded organic frames (HOFs), a new type of porous material, are assembled by the non-covalent interaction of organic and organometallic components, and have the characteristics of easy synthesis, high crystallinity, low density, good biocompatibility and high flexibility.91–95 The self-healing properties of HOFs help reduce damage, extend service life, and enhance stability through hydrogen bonding and π–π interactions.94 Their metal-free composition, renewability and recyclability make them environmentally friendly and economic.93 The versatility and designability of HOFs make them excellent candidates in photoelectric applications, and their hydrogen bonding properties make them superior to COFs and MOFs in solution processability and crystallinity.94 Similar to COFs and MOFs, the high crystallinity network of HOFs facilitates electron transport and light utilization efficiency, and its conjugated structure and π–π packing interaction promote charge transfer, regulating energy levels to achieve efficient light absorption and utilization.94 The sp2-π conjugated connection of HOFs enhances π electron delocalization, promotes photoexcited carrier transfer, and expands the range of light absorption.94,96 Currently, HOF-based photocatalysts have attracted increasingly more attention due to their excellent catalytic performance. One interesting HOF example is biohydrogen bond organic framework (HOF-25), which is derived from 2,2′-bipyridine (bpy) and uses a G-quadruplex as a rigid hydrogen bond backbone to further consolidate the hydrogen bond assembly by π–π stacking, and exhibits permanent porosity by co-stabilizing the G-quadruplex and the π–π interaction between layers. After post-modifying it with rhenium (Re) sites, which serve as the active center of photocatalysis, Yu et al. achieved a high visible light-driven carbon dioxide (CO2) reduction efficiency of 1448 mmol g−1 h−1 with a CO selectivity of 93%.97 The photocatalytic mechanism involves the transfer of electrons of the excited [Ru(bpy)3]Cl2·6H2O photosensitizer to HOF-25-Re, triggering the photocatalytic reaction. This study not only provides a new strategy for constructing stable HOFs, but also offers new insights for heterogenizing molecular catalysts to achieve CO2 photoreduction, opening up a new way to engineer functional HOFs.
3.2. Organic small-molecule compounds
3.2.1. Perylene imides (PDIs).
The PDI molecules are the best-known n-type organic semiconductors composed of a planar perylene ring and the lactam units at two ends, and it has two main modification sites, including both end units called “imide positions” and “bay positions” at the middle of the perylene ring, which boosts diversity of PDI derivatives.98 Excitingly, the conjugated π-electron system in the PDI molecule based on the formation between its planar (planar bicyclic structure with alternating single and double bonds) and aromatic structures (stilbene nuclei) allows for π–π stacking interactions between PDI units.99–107 Because of excellent fluorescence quantum yields, excellent photochemical stability, and enhanced donor–acceptor property, the PDI-based materials in organic photocatalytic field have shown great progress through functionalizing a PDI molecular structure, functionalizing the PDI surface with anchoring groups that can bind with semiconductor metal oxides or –NH2 terminal groups of graphitic carbon nitride (g-C3N4), and constructing self-assembled supramolecular architectures.108–114 Among these strategies, π–π interactions play an important role in forming a supramolecular structure with a π–π stacking spacing of 3.4–3.5 Å.115 In addition, the π–π stacking patterns in single PDI supramolecules can be mainly divided into H-aggregate (face-to-face arrangement) and J-aggregate (head-to-tail stacking).116 The former exhibits the compact co-facial stacking structure and strong π–π stacking degree, and these features not only result in blue-shifted absorption and obvious photofluorescence quenching, but also promote rapid electron transfer to form superoxide radicals (˙O2−) and holes. Differently, the J-aggregate of the perylene core structure with partial dislocation shows a lower π–π stacking degree, retaining the photoelectric property similar to that of a single PDI molecule. It not only causes red-shifted absorption and no distinct fluorescence quenching, but also facilitates energy transfer from the excited singlet state to the excited triplet state via intersystem crossing, ultimately generating singlet oxygen species (1O2). Clearly, the H-aggregate PDI supermolecules display more potential in the photocatalysis field because of more excellent mobility and separation capacity of photogenerated carriers, and deeper valence bands.117,118
In the self-assembling process of pure PDI supermolecules, the π–π stacking interactions are affected by side chains or substituents of monomer PDI. For instance, Li et al. found that the amide-modified PDI molecules formed self-assembled supramolecular (sAmi-PDI-HCl) nanofibers with high H-mode π–π interactions and hydrogen-bond networks that are beneficial for photogenerated charge carrier transport, finally enhancing organic pollutants’ photodegradation.119 Kong et al. further introduced a strong electron-withdrawing phosphate unit into two terminal positions of PDI, obtaining a multilayer P-PMPDI supramolecular nanobelt with the improved regularity of coplanar π–π interaction and strong dipole moment. Therefore, the resulting P-PMPDI provided a fast channel for the charge carrier separation and migration, resulting in high photocatalytic hydrogen evolution.120 Additionally, the PDI supramolecules’ π–π interactions further promoted their combination with other π-conjugated organic materials to build up extended π–π composites. For example, because of the π-conjugated structure in PTCDI and 7,7,8,8-tetracyanoquinodimethane (TCNQ), their self-assembling via π–π stacking successfully led to the formation of TCNQ-PTCDI co-catalysts under acid-based conditions. The formed strong electronic coupling and larger π-electron delocalization caused through the extended π–π stacking facilitate carrier transport and decrease carrier recombination, obviously promoting the photodegradation activity of phenol compared with the pure PTCDI.121 Sun et al. synthesized various supramolecular structures by substituting the terminal groups of PDI with different organic acids (6-aminonicotinic acid (NA), benzoic acid (BA), and propionic acid (PA)).122 Among these, the PDI conjugated with NA formed a biplanar π–π conjugated porous supramolecular photocatalyst (hp-PDI-NA). As depicted in the π–π stacking schematic of Fig. 7a, hp-PDI-PA did not form a biplane structure due to the absence of π-orbitals in its short alkyl terminal chains. Similarly, hp-PDI-BA also failed to form a biplane structure despite having a benzene ring at the terminal position. In contrast, hp-PDI-NA achieved double π–π conjugation through its biplanar structure (the primary plane of the PDI molecule and the nicotinic plane of the aminonicotinic acid), which enhances the π–π interactions and shortens the interlayer spacing, thus facilitating the photogenerated carriers' transport and separation. The study reveals that hp-PDI-NA exhibits photocatalytic phenol removal activity that is 3.5 times more excellent than that of standard PDI nanoparticles underneath visible light, along with excellent pollutant mineralization capabilities. This research highlights a novel approach to designing efficient PDI-based supramolecular photocatalysts through strategic end-group substitution and morphological control. Additionally, different drying strategies can also impact π–π interactions. Freeze-drying, in particular, helps preserve the π–π stacking within the PDI structure, which subsequently influences its photocatalytic performance.123 In a word, the π–π stacking interaction not only plays an important role to form self-assembled PDI supramolecules with different shapes (such as nanofiber and nanobelt) but can also be used as an efficient strategy to get composite photocatalysts with other π-conjugated materials, which finally improve the PDI-based photocatalyst performance.
 |
| Fig. 7 (a) Schematic of PDI-BA, PDI-PA and PDI-NA. ((a) Reproduced from ref. 122 with permission from Elsevier, Copyright 2022). (b) UV-Vis absorption spectrum of VOPc/CN photocatalysts and molecular flat model of the π–π interactions formed between them. ((b) Reproduced from ref. 124 with permission from Elsevier, Copyright 2019). (c) π–π stacking structure between O-CN and SA-TCPP. ((c) Reproduced from ref. 125 with permission from Elsevier, Copyright 2021). | |
3.2.2. Phthalocyanine.
Phthalocyanine is a planar cyclic tetrapyrrole compound characterized by π-electron delocalization and an 18-π conjugated network, offering exceptional optical and electrochemical properties.124,126 This includes strong absorption in the Q band between 600 and 800 nm, which imparts a deep blue color.124 Phthalocyanine molecules engage in π–π stacking with π-electron conjugated materials, enhancing the photocatalytic processes.124,127 Metal phthalocyanines, which are derivatives formed by substituting the central hydrogen with metal ions (such as Zn, Fe, Cu etc.), extend absorption into the near-infrared spectrum, boosting the photosensitization effect and photocatalytic performance.28,99,124,128–130 Additionally, their π–π conjugation with π-electron-rich materials enhances molecular attraction, improving photocatalytic efficiency.28,128 Liu et al. examined the photocatalytic H2 production performance of vanadium phthalocyanine oxynitride-modified carbon nitride (VOPc/CN).124 As illustrated in Fig. 7b, VOPc/CN demonstrates significantly higher light absorption in the visible spectrum compared to g-C3N4, with VOPc exhibiting a noticeable red shift within the VOPc/CN composite, indicating π–π stacking interactions between VOPc and g-C3N4. The 4 wt%-VOPc/CN system displayed a hydrogen production efficiency six times more wonderful than that of g-C3N4 alone. This enhancement is imputed primarily to the π–π interactions between VOPc and g-C3N4, which facilitate charge separation and enhance the efficiency of photocatalytic hydrogen evolution.
3.2.3. Porphyrin.
Porphyrin is a tetrapyrrole macromolecule with an 18π electron conjugation system, consisting of four pyrrole units connected by sp2 hybridized carbon bridges to form a planar square structure, which is widely used in the fields of catalysts, photosynthesis, phototherapy and sensors.131–133 As organic semiconductors with large π-conjugated systems, porphyrins have unique photochemical and redox capabilities, and their optical properties and easily tunable characteristics make them important in the field of chemical materials.134–137 They are excellent photocatalysts because of their selective absorption of visible light, and their self-assembly improves carrier mobility through π–π bonding, which further enhances photocatalytic performance.136,137 In addition, planar layer materials with π-electron conjugation can be assembled with the porphyrin via π–π stacking interactions,138–140 and the rigid planar surfaces of the porphyrin and abundant π-electrons can also enhance the adsorption of organic pollutants on the surface of the photocatalysts via π–π stacking interactions.141 Further, the porphyrin's tetrapyrrole macrocyclic core is an idealistic binding site for metals and is easily functionalized by metallization.132 During metallization, the porphyrin loses protons to form a double anionic ligand, and the residual nitrogen atoms are coordinated to the metal ions via a lone pair of electrons. The free-base porphyrin and metalloporphyrin units act as acceptors and influence the type of binding to the guest, where the free-base porphyrin binds the guest mainly through π–π interactions, while the metalloporphyrin forms a coordination bond with the guest through the metal center.132 Compared to porphyrins, metallized porphyrins also have an 18π conjugated planar structure, which effectively absorbs long-wave visible light and exhibits excellent electron transport, and the metal active sites of metalloporphyrins help to reduce charge complexation and further enhance photocatalytic ability.137 Xu et al. synthesized oxidized carbon nitride (O-CN) through thermal exfoliation and hydrothermal treatment, and combined it with porphyrin molecules via supramolecular self-assembly (TCPP) to create SA-TCPP/O-CN composites (Fig. 7c). SA-TCPP/O-CN-40% composites showed enhanced degradation capabilities for organic pollutants, with phenol degradation rates 2.5 times and 3.1 times more excellent than SA-TCPP and O-CN, respectively. Oxygen production rates for the SA-TCPP/O-CN-40% composite were 2.7 times and 4.7 times more excellent than that of SA-TCPP and O-CN, respectively, and the sterilization efficiency increased significantly to 62.5%, surpassing both SA-TCPP and O-CN. These improvements are attributed to the 0D/2D heterostructure formed via π–π interactions between SA-TCPP and O-CN, which generate built-in electric fields and electron departure domain effects. These enhancements promote interfacial charge transfer and the generation of a large amount of reactive oxygen radicals, thereby improving the photocatalytic performance.125
3.2.4. Complexes.
Complex is a type of compound formed by a central atom or ion and a molecule or ion (ligand) containing a lone pair of electrons through a coordination bond. The π–π interaction within a complex is a crucial determinant of its internal chemical structure, material stability, morphology, and functionality.142–145 It modifies the photophysical properties by adjusting the rotational conformations of aromatic ligands.142 This interaction enables the transition from zero-dimensional to one-dimensional,143 one-dimensional to two-dimensional,143,146 two-dimensional to three-dimensional,147 and directly from one-dimensional to three-dimensional,148 forming a versatile supramolecular network. In addition to the inherent changes within complexes, π–π interactions also occur between π-conjugated complexes and other π-conjugated complexes, reaction substrates and semiconductor materials.149–156 These interactions facilitate charge migration and transmission, thereby enhancing photocatalytic performance.149–156 Zhang et al. constructed mononuclear Zn(II) molecular complex-coupled co-catalysts for CO2 photoreduction, in which the introduction of CoCl2 was the most effective, and the CO generation rate was as high as 494.4 μmol g−1 h−1 (100% selectivity), which was mainly attributed to the fact that the catalytic capacity could be extremely boosted by the ordered heterogeneousness of mononuclear Zn(II) molecular complexes via π–π stacking (Fig. 8a).149 Qiu et al. constructed Re[4,4′-di(pyren-1-yl)-2,2′-bipyridine](CO)3Cl (4,4′-dipyrenyl-Re) catalysts with bifunctional photosensitizer pyrenyl moiety (photosensitized and π-structured) units, which can achieve efficient CO2 reduction under visible light, and TONCO can be as high as 1367 ± 32, which is mainly imputed to the π–π interactions originating from the pyrene moiety in the catalyst structure and the extension of the π-conjugated system remarkably improves the electron transport efficiency and visible light trapping ability (Fig. 8b).150
 |
| Fig. 8 (a) Schematic of CO2 reduction based on heterogeneous (π-1) and homogeneous ([Zn(phen)2L]) photocatalysts. ((a) Reproduced from ref. 149 with permission from National Academies Press, Copyright 2022). (b) Design and mechanism of complex catalysts for the photoreduction of CO2. ((b) Reproduced from ref. 150 with permission from Royal Society of Chemistry, Copyright 2020). | |
3.3. Conjugated polymers
3.3.1. Carbon nitride.
To date, various types of carbon nitride materials including graphitic carbon nitride (g-C3N4), poly heptazine imide (PHI), poly triazine imide (PTI), and other synthetic polymeric carbon nitrides have been synthesized and employed across diverse research areas in photocatalysis.157–161 Among them, g-C3N4 is widely favored due to its extensive applications, frequent research focus, and distinct optoelectronic properties.162 It is composed of sp2 hybridized carbon and nitrogen atoms that form a π-conjugated planar structure akin to graphene, notable for its high stability and non-toxicity.162 The π–π conjugated bond structure within g-C3N4 provides active sites for catalytic reactions.163 Furthermore, a π–π stacking structure is formed between the layers of g-C3N4 heptazine units (C6N7).164,165 π–π stacking influences interlayer carrier transport and separation.166 Additionally, g-C3N4 can be combined with various π-conjugated materials to create composites that enhance its photocatalytic performance.167 The other two noteworthy materials, PHI and PTI, are nitrogen-containing polymers characterized by their cyclic nitrogen-containing heptazine and triazine units linked by imine bonds (–NH–).161 This arrangement endows PHI and PTI with a rich π-electron system at the molecular level, facilitating π–π interactions due to their cyclic structures with extended π-electron systems.161,168–170 Researchers can manipulate π–π interactions in carbon nitride materials by adjusting the materials' structure, layer stacking, or surface functionalization, thus leveraging their significant potential in photocatalysis. Tang et al. developed BCN/CNx composite materials for the photoreduction of CO2 by an ultrasonic self-assembly technology to combine two types of carbon nitride materials.32 The process involves synthesizing boron-containing carbon nitride (BCN) and nitrogen-deficient ultrathin carbon nitride nanosheets (CNx), and forming heterojunctions via π–π stacking interactions (Fig. 9a). The BCN/CNx composite exhibits superior performance in converting CO2 into CH4, achieving higher yields than either BCN or CNx alone, thanks to the strong π–π interactions and efficient electron transport within the composite structure. Xu et al. constructed a FePc/g-C3N4(CNFP)/H2O2 photo-Fenton system for the removal of oxytetracycline (OTC) by calcination.171 The OTC removal rate of CNFP60 reached 88.48% at 1 h, which was significantly higher than that of g-C3N4 (41.30%), while the degradation rate of CNFP60 was 7.4 times more excellent than that of g-C3N4. The principal cause for the enhanced photocatalytic performance is the constitution of a fast electron transfer channel by the π–π interaction between FePc and g-C3N4, which realizes the rapid transfer of photogenerated electrons (Fig. 9b).
 |
| Fig. 9 (a) Preparation route of BCN/CNx hybrid materials ((a) Reproduced from ref. 32 with permission from Elsevier, Copyright 2023). (b) Illustration of the photo-Fenton reaction mechanism of FePc/g-C3N4 composites. ((b) Reproduced from ref. 171 with permission from Elsevier, Copyright 2023). | |
3.3.2. Other conjugated polymers.
The π–π interactions in other conjugated polymers (traditional and novel) are influenced by their aromatic and conjugated nature. Traditional conjugated polymers such as polyaniline, polypyrrole, and polythiophene feature π–π conjugated structures within their polymer chains, facilitating the formation of π–π interactions.172–178 These polymers are characterized by extensive π-conjugated skeletons, which impart high electrical conductivity, environmental stability, superior light absorption, and excellent carrier mobility, which contribute to better charge conversion during photocatalysis.174–180 In addition to traditional conjugated polymers, new π-conjugated organic polymers have emerged as efficient visible light-driven photocatalysts. These materials exhibit a large specific surface area, adjustable structures, designable properties, low cost, and high stability.135,181 Their π-delocalized systems respond effectively to sunlight, promoting charge migration and separation.182 π-conjugation along the polymer chains and π-stacking between chains result in hybridized energy levels, which facilitate efficient charge transfer and separation.183 Additionally, the π-conjugated skeleton and surface functional groups can adsorb pollutants, enhancing the photocatalytic performance.182 Furthermore, hybridizing these polymers with other π-conjugated materials uses π–π stacking to improve charge transfer and dissociation, thereby boosting the photocatalytic activity.184,185 In summary, researchers can control and design π–π interactions in conjugated polymers by selecting appropriate polymer structures, side groups, and processing conditions. This is essential for understanding and optimizing the behavior and properties of conjugated polymers in various applications, especially in the field of photocatalysis.
3.4. Carbon materials
Carbon-based materials such as graphenes, graphene oxides (GOs), reduced graphene oxides (RGOs), carbon nanotubes (CNTs), graphite, graphdiyne, biochar, carbon quantum dots (CQDs) and graphene quantum dots (GQDs) are known for their capacity to construct π–π interactions with other π-conjugated materials or aromatic compounds (Fig. 10a–c). Among them, graphenes and their derivatives are the most widely used. Graphene, a two-dimensional material, features a unique honeycomb structure comprising a single layer of carbon atoms arranged in a tightly bound sp2 hybrid configuration.41,186–189 This arrangement endows graphene with outstanding physical and chemical properties (high conductivity and superior electron mobility).41,186–188,190 The sp2 hybridized carbon atoms in graphenes form covalent bonds that contribute to a delocalized π-electron cloud across their surface, creating an extensive π-conjugated structure.186–188,191 This structure facilitates π–π interactions with other molecules or materials via π-electron cloud interactions.41,186–188,192 Particularly, the π–π stacking interactions between graphenes and the aromatic rings of organic compounds in water make them highly effective in absorbing and removing pollutants.186–188 Similarly, graphene derivatives (GOs, RGOs, and GQDs) and CNTs also have such extended π structures, which are extremely easy to form π–π interactions with other materials or pollutants with π conjugated structures.102,127,193–201 Graphyne, an innovative material crafted from a fusion of sp and sp2 hybridized carbon atoms, exhibits distinctive attributes such as an extensively conjugated π-system (easy to form π–π interaction), conformally arrayed pores, and tunable electronic properties, thereby unveiling its burgeoning promise in the realm of photocatalysis.202–204 While the aforementioned carbon materials are widely utilized, their intricate synthesis processes and high costs hinder large-scale application. Consequently, biochar has attracted attention for its easy availability, low cost, porous structure, rich functional groups, excellent adsorption capabilities, and tunable chemical and physical properties.205–209 Notably, biochar also possesses a π-conjugated electron system through π–π stacking and ether bond formation, demonstrating significant potential in the field of photocatalytic degradation.210–212 Currently, carbon materials are primarily utilized in photocatalysis for the degradation of pollutants. This effectiveness is largely due to the π-conjugated systems within their structures, which engage in π–π interactions with pollutants to enhance the photocatalytic activity. Additionally, there is a small but growing body of research focused on combining carbon materials with other π-conjugated semiconductor materials through π–π interactions, broadening their application in various areas of photocatalysis. Tang et al. constructed graphene-like marl biochar-modified g-C3N4 (BC/CN) by calcination, and photocatalytic synergistic persulfate (PMS) removal of tetracycline could be achieved under visible light (1 h, 90% removal rate), which was principally due to the electron-absorbing property of the biochar that could modulate g-C3N4's electronic constitution, and meantime, the formation of the π–π interaction between them can accelerate the photogenerated electron transport channel (Fig. 10d).211 Sun et al. developed a perylene diamide/graphene-g-C3N4 composite photocatalyst (PDI/G-CN) that is responsive across the entire light spectrum. This photocatalyst is capable of photo-reducing CO2 to produce CO and CH4 with an efficiency 16 times more excellent than that of g-C3N4 alone. This enhanced performance is primarily owing to the creation of an S-type heterojunction among the three components via π–π interactions, which markedly improves the efficiency of charge separation (Fig. 10e).101
 |
| Fig. 10 (a) Summary of currently used carbon materials with π–π interactions, the main structural forms of the above materials. (b) Graphite (sp2 hybridized carbon atoms) and (c) graphdiyne (sp2 and sp hybridized carbon atoms). (d) Mechanistic diagram of the photodegradation of pollutants by biochar/g-C3N4 synergized PMS. ((d) Reproduced from ref. 211 with permission from Elsevier, Copyright 2022). (e) S-scheme electron migration pathway of PDI/G-CN composites and mechanistic diagram of photo-reduction of CO2. ((e) Reproduced from ref. 101 with permission from American Chemistry Society, Copyright 2021). | |
3.5. Other materials
In addition to the previously mentioned examples, π–π interactions can occur in a wide variety of materials (BN, dyes, some organic molecules, etc.) in which aromatic rings or conjugated π-electron systems are present, and the principle of their π–π interactions is essentially based on the presence of aromatic or conjugated structures and the ability of these structures to participate in attractive interactions.26,97,213–224 In summary, researchers can control and design π–π interactions in these materials by modifying the chemical structure, adjusting the distance between interacting structures, or introducing functional groups that enhance or inhibit interactions. Thus, π–π interactions can occur in a variety of materials in addition to the examples discussed earlier.
3.6. Summary
The choice of photocatalysts forming π–π interactions hinges on the particular application and requirements. The advantages and disadvantages of different types of photocatalysts that can form π–π interactions are as follows: (a) most of the conjugated polymers, organic small molecules, and organic framework materials have the advantages of excellent charge transport properties, high absorbance in the visible and near-infrared areas, flexible design and synthesis for various applications, and obvious π–π interactions between conjugated structures. Their disadvantages are mainly limited stability in certain environments (e.g., UV and pH) and the need for some additional modification or protection. In addition, the raw materials for synthesizing these materials are very expensive and not suitable for large extent production, and some of them are toxic (potential environmental problem). Nevertheless, among them, there are also special materials that can be stabilized. For instance, g-C3N4 has excellent physical and chemical stability at room temperature, and its layered structure is very easy to modulate. However, because it generally forms a disordered structure, the π–π interactions are difficult to adjust, and its light absorption ability is weak. Therefore, directional modulation of the g-C3N4 structure is ineffective, which limits its charge separation and capability and, consequently, restricts its development. (b) Carbon materials possess unique characteristics making them attractive for π–π interactions, and their advantages are mainly high stability, wonderful electrical conductivity, large specific surface area, dimensional diversification, and excellent compatibility. Their disadvantages are mainly their lack of specificity in forming π–π interactions with various aromatic or conjugated compounds, the ease of aggregation between their layered structures, the difficult control of surface functionalization, the fact that some of the carbon materials are also polluted by the production process, and the high cost of synthesis of advanced carbon materials (single-walled carbon nanotubes, high-quality graphenes, graphyne, etc.). These drawbacks need to be addressed when designing and using these materials in various applications. In addition, Table S1 (ESI†) also shows the advantages and disadvantages of other related materials and the difficulty of regulating the π–π interaction, which is still a great challenge in general. In summary, the selection of the most favorable photocatalyst depends on the specific photocatalytic reaction, environmental conditions, and desired properties. The trade-offs between advantages and disadvantages must be carefully considered and the photocatalytic materials and systems optimized accordingly to meet the desired performance criteria.
4. π–π interaction between photocatalytic materials and reactant organic molecules
Besides the π–π interactions within aromatic photocatalysts themselves that indirectly affect their catalytic performance, the π–π interactions between aromatic photocatalysts (such as carbon nitride, COFs, MOFs, PDIs, and various types of composites modified with carbon materials) and organic conjugated reaction molecules exist in various photochemical and photophysical processes, which directly affect the efficiency and selectivity of photocatalytic reactions. Therefore, it makes sense to investigate the π–π interactions of aromatic photocatalysts and organic conjugated reaction molecules and to discuss the influence on photocatalyst performance.
4.1. Formation of π–π interaction between photocatalysts and organic pollutants
First, π–π interactions were found between aromatic photocatalysts and organic pollutants, which mainly include polycyclic aromatic hydrocarbons (PAHs), dyes, drugs, and aromatic pesticides.206,208,225–235 It significantly affects degradation and removal of organic pollutants.135,236–240 During the photocatalytic process, the π electron clouds between the photocatalyst and the organic pollutant may overlap and result in the π–π interaction. In addition, it further enhances the pollutant's adsorption on the photocatalyst's surface, which is beneficial for the subsequent photocatalytic degradation reaction. Therefore, researchers often use the strategy, enhancing the π–π interaction by exploring new photocatalysts with aromatic structure, towards improving the efficiency of aromatic pollutant molecules. For example, Gao et al. successfully designed the photocatalytic adsorbent MIL-53(Fe) and found that it exhibited a good adsorption capacity (0.57 mmol g−1) of carbamazepine (CBZ) from water, which is mainly due to π–π interactions between MIL-53(Fe) and CBZ.241 Similarly, Du et al. constructed a composite (NHCS/Zn2In2S5) of self-assembled Zn2In2S5 and N-doped hollow carbon spheres (NHCS) with the rich π-conjugated aromatic backbone structure. Therefore, the organic pollutants (phenol or bisphenol A (BPA)) with abundant π bonds can be gathered onto the surface of the composite (NHCS/Zn2In2S5), which leads to reduce activation energies of the phenol*/BPA*.242 Therefore, both phenol (97.6%) and BPA (79.7%) were efficiently removed. In addition, the separated electrons were quickly transferred to the dissolved oxygen, resulting in higher H2O2 yields of 1.31 mmol L−1 from phenol and 2.31 mmol L−1 from BPA, respectively. Zhang et al. further confirmed that the π–π interactions between g-C3N4 (CNN-500) and pollutant molecule (flumequine, FLU) facilitated efficient charge separation and transfer, finally accelerating the photocatalytic degradation rate of FLU. That is, CNN-500 can photocatalytically remove 62.9% of FLU in 30 min.243
4.2. Formation of π–π interactions between photocatalysts and organic reaction substrates
In addition, π–π interactions were confirmed to be able to promote the conversion of organic conjugated reaction substrates. These substrates containing aromatic or conjugated structures, such as aromatics, some biomass molecules or aromatic molecules with π-electron systems, can form π–π interactions with these photocatalysts via van der Waals forces, enhance the adsorption of substrates onto the catalyst surface, and affect the reaction efficiency and selectivity.24,33,244 In the presence of light, the substrate molecules are more likely to participate in photochemical processes, such as electron transfer, and the π–π interaction leads to selective activation of the substrate and controls the reaction outcome. Researchers use these interactions to design and optimize photocatalytic systems such as photoredox, cross-coupling, and photodissociation reactions. Das et al. used a chemically stable two-dimensional microporous COF (PCR-1) with a dangling phenyl group as a photocatalyst for H2O2 generation (adding benzyl alcohol as a sacrificial agent). This induced the strong π–π interactions of PMCR-1 and benzyl alcohol that facilitates efficient hole transfer, obviously increasing the production of H2O2 and a higher selective oxidation of benzyl alcohol to the corresponding aldehyde. Based on these inspired findings, the catalyst (PMCR-1) was highly selective for other benzene ring species (benzylamine and methyl sulfide derivatives).24 Different from the above-mentioned sections, Bai et al. explored the heterojunction Zn0.5Cd0.5S/graphene oxide (ZCS/GO) composites that induced charge separation and then formed reduction and oxidation centers.33 Meanwhile, the π–π interactions of GO and benzene ring promoted charge carriers’ spatial distribution, which improved interfacial charges transfer and photocatalytic hydrogenation/oxidation activities of ZCS/GO. Therefore, ZCS/GO realized the cascade conversion from p-xylene to p-methylbenzaldehyde and finally to selected p-methylbenzyl alcohol. Additionally, other mesoporous 2D organic photocatalysts show a similar property when the π-conjugated material is used as the reaction substrate. Liu et al. found that the mesoporous graphitic carbon nitride (mpg-C3N4) photocatalyst also formed the favorable π–π interactions with a lignin model molecule, finally realizing 90% conversion rate of lignin model molecule via photocatalytic oxidation (cleavage of the C–C structure: β-O-4 and β-1 bonds) under visible light.244
5. Influence of π–π interaction on photocatalysis
The π–π interactions, esteemed as one of the most fascinating and important intermolecular noncovalent interactions, affect greatly the crystal size and crystallinity of conjugated organic photocatalysts, subsequently impacting their multiple physicochemical traits. Moreover, they can directly influence charge transfer, thereby significantly enhancing the efficiency and stability of photocatalytic systems. The mechanisms underlying π–π interactions in photocatalytic reactions are intricate and contingent upon the specific materials and reactions involved. In general, π–π interactions can affect photocatalytic reactions in the following ways (Fig. 11 and Table S2, ESI†).
 |
| Fig. 11 Main influence of π–π interactions on photocatalysis. | |
5.1. Enhancing light absorption
π–π interactions can lead to stacking or close proximity of aromatic or conjugated systems within the photocatalyst material, which enhance the light absorption properties of the material by better π–π interactions.245 Stacked aromatic systems with extended π-electron systems can absorb photons over a wider wavelength range, including UV and visible light. π–π interactions can affect the energy level and electronic structure of photocatalysts. The stacking of π-conjugated systems can lead to changes in energy levels, which is important for matching the band gap of the photocatalyst to the energy of the incident photon.121,122,246 This extended light absorption is essential for triggering photocatalytic reactions.
5.2. Promoting electron–hole pair separation
Photocatalytic reactions implicate the creation of e−–h+ pairs when the photocatalyst absorbs photons. Effective charge separation is essential for these reactions to occur. π–π interactions can play a role in separating these charge carriers.154,166 In materials with π–π stacks, photogenerated electrons and holes may be located in different parts of the stack structure, leading to spatial separation. This spatial separation reduces the likelihood of electron–hole pair complexation, which would otherwise reduce the efficiency of the photocatalytic process.121,155,247 In addition, π–π interactions can facilitate electron transfer between aromatic or conjugated units within the photocatalyst. These units are in close proximity, enabling efficient electron transfer pathways that promote electron mobility and transfer to active sites on the surface of the material, where the chemical reaction occurs.42,248 Due to the presence of π–π stacks, excitons generated by light absorption can move along π-conjugated paths, thus improving the chances of charge separation and reaction at the active site.249 Furthermore, it has been found that only moderate π–π interactions at appropriate distances can balance charge transfer and recombination, thereby optimizing carrier generation and transition processes in photocatalytic systems.23 In some photocatalytic systems, π–π interactions may be convoluted in heterojunctions' constitution, in which different semiconductor materials (organic semiconductors or inorganic semiconductors) are combined together.250–253 These connections can improve charge separation at their interface, so the construction of composites with π–π interactions can advance carrier separation and thus reduce recombination.
5.3. Optimization of stability
π–π interactions also contribute to the stability of the photocatalyst.175 π–π interactions on the surfaces of photocatalysts improve crystalline stability, enhance mechanical stability, and improve charge separation.78,175,254–256 These combined effects lead to improved stability and performance of photocatalysts, ensuring their long-term effectiveness across a broad spectrum of applications.
5.4. Adjusting reaction pathways and selectivity
π–π interactions can significantly affect the selectivity and pathways of photocatalytic reactions by influencing the adsorption, activation, and transformation of reactant molecules on the surface of the photocatalyst.257,258 π–π interactions serve multiple roles in photocatalytic reactions including regulating the adsorption orientation of substrates on the photocatalyst surface, activating and stabilizing reaction intermediates.170,258–260 These effects collectively favor specific reaction pathways while inhibiting others, thereby enhancing product selectivity. Overall, π–π interactions offer a valuable approach for designing and optimizing photocatalysts by precisely controlling various aspects of the photocatalytic process to achieve efficient and selective conversions tailored to particular applications.
It is worth noting that the specific role and impact of π–π interactions on photocatalytic reactions hinge on the nature of photocatalysts, the reactants involved, and the reaction environments. By careful design and engineering of photocatalytic materials, these interactions are used to enhance the performance of various applications such as pollutant removal, hydrogen peroxide production, water splitting, CO2 reduction and synthesis of valuable chemicals.
6. Modulating π–π interaction for photocatalysis
Based on above-mentioned effects of π–π interactions on photocatalysts’ properties, the design and selection of appropriate materials with optimized π–π interactions can improve the efficiency and selectivity of photocatalysis. Therefore, it makes sense to modulate carefully π–π interactions for high-performance photocatalysts. To date, researchers have proposed several effective strategies including side-chain engineering, substituent engineering, and doping of main aromatic structures in photocatalysts. The detailed discussion is shown as follows:
6.1. Side-chain and substituent engineering
Although π–π interactions depend on the main aromatic structure stacking in photocatalysts, different types of side-chains and halogen substituents on main aromatic structure also influence the π–π interactions between aromatic units of photocatalysts, because of the steric hindrance and the electron push–pull property, which can tune the light absorption and crystallinity of related photocatalysts, and enhance their charge separation.254,261,262 For instance, Liu et al. designed three linearly conjugated polymers (ethylenediamine-PDI, oxamide-PDI, and oxamide-NDI) based on two aromatic cores (perylene diimide (PDI) and naphthalene diimide (NDI)) and two different covalently linked sections (ethylenediamine and oxamide) (Fig. 12a).254Fig. 12b demonstrates that highly crystallized oxamide-PDI shows the best photocatalytic oxygen production performance. It was mainly attributed to the fact that oxamide-PDI not only yielded a higher π–π stacking interaction energy and enlarged light absorption from expanded π-conjugation of oxamide, but also produced a strong built-in electric field from a strong internal dipole moment and ordered crystalline structure that facilitates the efficient migration and separation of charge carriers. In addition, the length of the side chain influences its π–π stacking mode in organic aromatic photocatalysts. Wang et al. developed H/J-type aggregated PDI (perylene tetracarboxylic acid diimide) supramolecular nanostructures using two PDI derivatives modified with linear carboxyl-substituted side chains of different lengths.117 H-PDI demonstrated superior efficiency in photocatalytic pollutant removal and oxygen production, particularly for phenol removal. This enhanced performance is attributed to H-PDI's stronger π–π interactions and a narrower band gap, which facilitate more effective photogenerated carrier migration and produce a higher yield of superoxide radicals and potent oxidizing holes. Surprisingly, a few studies have noted that regioisomers also influence π–π interactions in organic aromatic photocatalysts. Wang et al. developed regioisomeric benzotriazole-based COFs, where the symmetrical isomer (BTz-COF-S), due to its more ordered π–π stacking, exhibits stronger light absorption and exciton dissociation capabilities. This results in superior photocatalytic hydrogen production activity to the asymmetric isomer (BTz-COF-AS).263 Different from the above-mentioned side-chain tactics, using halogen substituents (such as F and Cl) represents another efficient way to facilitate π–π interactions of organic aromatic photocatalysts. Wang et al. introduced electronegative halogen atoms (F and Cl) into benzothiadiazole as a acceptor unit, which combine 1,3,5-tricarbonylresorcinol (TP) as a donor unit to successfully synthesize donor–acceptor (D–A) conjugated COFs (COF-Cl and COF-F) (Fig. 12c).261 Through theoretical calculations, COF-F showed the lower charge (1.191) of the TP donor unit and the highest charge (0.286) of the acceptor component compared with those of COF-H and COF-Cl. It indicates that the electronegative F substituent enhances the internal segregation driving force and promotes charge separation. In addition, due to the formation of intramolecular hydrogen bonds between the F atom and the hydrogen atoms of adjacent benzene rings, the smaller torsion angle and center distance of the benzene rings in COF-F not only provide a better planarity of COF-F, but also improve the conjugation degree and π–π interactions between COF-F molecule interlayers. This helps to increase the crystallinity of COF-F, and obtain the faster axial carrier charge transfer and lower the carrier recombination rate based on the maintained beneficial face-to-face stacking (AA′ positive stacking mode). Therefore, COF-F finally exhibits a higher photocatalytic hydrogen production activity than COF-Cl and COF-H (Fig. 12d). In addition, based on the two above-mentioned strategies’ synergistic effects, An et al. constructed a series of benzothiadiazole (BT)-based polymeric materials with adjusting different side chains and substituents for hydrogen production.262 As seen from Fig. 12e, compared with the P1 polymer, the P2 polymer was introduced by a linear oligo (ethylene glycol) (OEG) side chain and the P3 polymer was incorporated by both the OEG side chain and the backbone fluorine substituent. It was found that the hydrophilic OEG side chains not only promoted water uptake but also enhanced intermolecular packing and efficient exciton separation. Additionally, the fluorine substituents on the polymer backbone improved the noncovalent coulombic interactions, resulting in a planar chain conformation in polymer's structure. This enhanced polymer's self-assembling capability. Therefore, the P3 polymer showed the π–π stacking enhancement and the higher long-lived polarons’ yield, ultimately realizing the higher hydrogen production activity, as shown in Fig. 12f. Therefore, the side-chain and substituents are regarded as indirect strategies to change the density of the π-electron cloud and the π–π stacking distance of adjacent main aromatic structures, finally influencing related photocatalysts’ catalytic performance.
 |
| Fig. 12 (a) Preparation routes of several linear conjugated polymers and (b) their corresponding photocatalytic oxygen production performance comparison plots. ((a) and (b) Reproduced from ref. 254 with permission from Wiley-VCH, Copyright 2023). (c) Preparation roadmap of several COFs and (d) comparative photocatalytic hydrogen precipitation performances of the above three COF materials. ((c) and (d) Reproduced from ref. 261 with permission from American Chemistry Society, Copyright 2023). (e) Preparation roadmap of three conjugated polymers. (f) Comparative photocatalytic hydrogen production performance of three conjugated polymers. ((e) and (f) Reproduced from ref. 262 with permission from Wiley-VCH, Copyright 2023). | |
6.2. Doping and extending of main aromatic structures in photocatalysts
Doping the main aromatic structure of organic semiconductors or expanding the π-electron cloud can influence the π–π interactions between the aromatic units of photocatalysts. This modification adjusts the charge distribution in the photocatalysts, impacting their light absorption and charge separation capabilities.122,255,264,265 Miao et al. successfully synthesized two different two-dimensional β-ketoenamine-linked COFs with hexagonal skeleton structures: TpTAPT-COF and TpTAPB-COF by combining different organic units (1,3,5-tris(4-aminophenyl)triazine (TAPT) and 1,3,5-tris(4-aminophenyl)benzene (TAPB)) with 1,3,5-triformylphloroglucinol (Tp) (Fig. 13a), respectively.255 The high rigidity and planarity of the triazine ring in TpTAPT-COF result in better crystallinity and more pronounced π–π interactions, as evidenced by the sharper diffraction peak at around 26° (Fig. 13b). Consequently, TpTAPT-COF exhibits superior catalytic activity and selectivity in the photocatalytic oxidation of benzyl sulfide (Fig. 13c). The minimal steric hindrance of the triazine unit allows TpTAPT-COF to maintain stable planarity and tight π–π stacking, enhancing light absorption and facilitating charge separation and migration. Building on previous research, Han et al. substituted the benzene ring with a pyridine ring on the side chain of the triazine ring, forming a new covalent triazine framework (T3N-CTF).265 This modification expanded the π electron clouds on the triazine ring, enhancing electron delocalization and conjugation length. The resulting increase in π–π stacking contributed to superior carrier separation and transmission efficiency, leading to a higher photocatalytic hydrogen production rate. As a representative organic semiconductor material with π–π interactions, Chen et al. improved the π–π conjugated structure of carbon nitride by doping with 1,3,5-benzenetriol (Fig. 13d).266 This doping replaced the triazine ring with a benzene ring in the π-electron delocalized heptazine ring structure, affecting π–π interactions, broadening light absorption, and improving charge separation. The optimized BCN-0.03% sample exhibited a H2 output rate underneath visible light irradiation 6.6 times more excellent than that of g-C3N4 (Fig. 13e). This increase in efficiency is primarily attributed to the dopant choice, which facilitates the formation of unique coplanar heterojunctions (Fig. 13f). These heterojunctions not only retain the g-C3N4's original network formation but also significantly enhance the separation and transfer of photogenerated carriers by an improved π-conjugated endogenic electric field.
 |
| Fig. 13 (a) Structural diagrams of the two COFs and (b) their corresponding PXRD patterns. (c) Reaction time curves for the conversion of phenylmethyl sulfide by the two COFs. ((a) and (c) Reproduced from ref. 255 with permission from Royal Society of Chemistry, Copyright 2023). (d) Preparation process of introducing a benzene ring structure in g-C3N4. (e) Graph of photocatalytic hydrogen production results of all the materials under visible-light irradiation. (f) Roadmap of photogenerated carriers' charge transport on the novel carbon nitride. ((d)–(f) Reproduced from ref. 266 with permission from Elsevier, Copyright 2022). | |
6.3. Regulation of metal ions
At present, the effect of metal ions on the conjugation and π–π interaction in material structure has not been widely concerned. Metal ions are found to many influence the π–π interactions in MOF materials, and can significantly influence these interactions via coordination with organic ligands, regulating electronic properties, and interfering with π–π packing.86 The coordination between metal ions and organic ligands not only determines the pore structure, conductivity and crystallinity of MOFs, but also affects the π–π stacking pattern between molecules.86 The size, coordination mode and geometry of metal ions affect the distance and arrangement between ligands, thus changing the strength of the π–π interaction.86 However, in the photocatalytic reaction system, no researchers have carried out in-depth research on this yet. In addition, we expect that later work can carry out more studies on the influence of metal ions on π–π interaction in photocatalytic systems; reasonable design and selection of metal ions may significantly improve the photocatalytic performance of materials.
7. Construction of π–π interactions in heterojunction systems
In some photocatalytic systems, π–π interactions may be involved in the formation of heterojunctions, in which different semiconductor materials are combined together. These connections can improve charge separation and promote more efficient photocatalytic reactions.
7.1. Hybridization of different organic photocatalysts
Organic semiconductor molecules are usually composed of macromolecules with π-electrons, which form conjugated molecular orbitals, so that this structure can form a low bandgap and generate photoelectron excitation. The complex electronic structure of organic semiconductors can easily cause scattering and compounding of carriers, and hence, the construction of composites with π–π interactions can promote the separation of carriers and thus reduce compounding, as well as enhance the stability of catalysts.32,185,214,250,251,267 Xu et al. constructed a large π-isolated domain direct Z-type polymer composite structure (g-C3N4/P1Cl-T) by combining a two-dimensional (2D) donor–acceptor conjugated polymer (P1Cl-T) with g-C3N4via the π–π stacking interaction between them, and the various characterization results also showed that the π–π interaction between them successfully formed an extended π network on the whole polymer heterojunction, which facilitated the full-spectrum absorption, formation of the built-in electric field, exciton dissociation and charge transport, and ultimately realized the substantial improvement of the hydrogen production performance.184 The orbital overlap between the triazine unit with a π-electronic structure in the g-C3N4 structure and the polymer with a D–A structure and its π-electronic cloud can be easily realized, which is very conducive to the photogenerated charge migration. Compared with g-C3N4 (2.31 mmol h−1 g−1) or P1Cl-T (0.33 mmol h−1 g−1), the hydrogen production performance of g-C3N4/P1Cl-T photocatalysts was significantly enhanced (g-C3N4/P1Cl-T7 had the best performance, 49.22 mmol h−1 g−1), and the photocatalytic hydrogen production performance of g-C3N4/P1Cl-T7 was further enhanced by the further introduction of Pt, reaching 111.8 mmol h−1 g−1. Therefore, we can conclude that the reconstructed extended π-network on the Z-type heterojunction is responsible for the substantial increase in the hydrogen production activity.
7.2. Hybridization of inorganic photocatalysts and organic photocatalysts
In addition to the above-mentioned considerations, hybrid systems of inorganic and organic semiconductors can combine the many advantages offered by inorganic and organic semiconductors, taking full advantage of the unique properties of each material class. For example, the flexible structure of organic semiconductors can be easily incorporated into the surface of inorganic semiconductors, organic semiconductors can be designed to achieve energy band matching with inorganic semiconductors, organic semiconductors can also increase the light absorption of the hybrid system, and the stability of inorganic semiconductors can enable organic–inorganic hybrid materials to exhibit better long-term stability compared to pure organic semiconductors. Overall, combining inorganic and organic semiconductors in hybrid materials has a wide range of advantages that make them promising candidates for photocatalytic applications. These advantages from the synergistic combination of the strengths of both material classes mitigate their respective weaknesses.104,105,111,113,252 Yang et al. constructed a full-spectrum Ag2S/PDI composite system for photocatalytic removal of phenol and photocatalytic oxygen production performance.253 The construction process of Ag2S/PDI composites is mainly due to the formation of hydrogen bonding and π–π stacking from PDI tightly bound by electrostatic self-assembly. The apparent rate constants for visible light degradation of phenol by Ag2S/PDI composites (1
:
0.6) exhibited the fastest degradation rate (0.0259 min−1), which was 6.93 times higher than that of pure PDI (0.00374 min−1). Further, Ag2S/PDI (1
:
0.6) could remove 94.48% of phenol within 2 h. The degradation rate of Ag2S/PDI (0.0259 min−1) was 6.93 times higher than that of pure PDI (0.00374 min−1). In addition, the oxygen production performance of Ag2S/PDI (1
:
0.6) (34.6256 μmol h−1 g−1) was improved by 1.79-fold compared to that of PDI (19.3312 μmol h−1 g−1). The above-mentioned results indicated that Ag2S and PDI form a built-in electric field, which helps the photogenerated electrons to migrate along the direction of π–π stacking, thus generating more reactive radicals and more powerful oxidizing ability.
8. Application of materials involving π–π interaction during photocatalysis
Fig. 14a and b show the applications and distribution of photocatalysts with π–π interactions in various fields of photocatalysis since 2002, in which we can find that more than half of the papers are related to the photodegradation of pollutants, most of which are related to the degradation of aromatic pollutants. We find that the proportions of reported reactions are, in descending order, pollutant degradation (56.7%), water splitting (19.8%), organic synthesis (9.5%), and CO2 reduction (5.4%) that are the main categories of applications, the details of which will be introduced next. In addition, we will introduce in detail the two popular areas of H2O2 generation and biomass conversion. Other photocatalytic applications are relatively rare, mainly through the built-in electric field generated by π–π interaction to improve their photocatalytic performance.
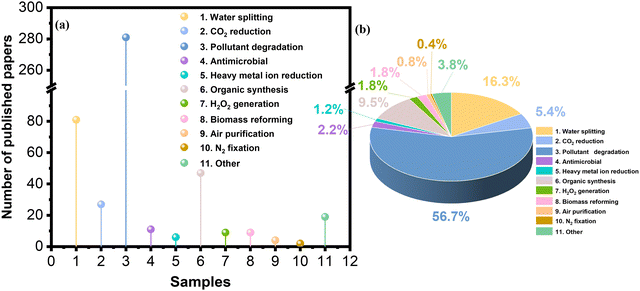 |
| Fig. 14 Publication status (a) and proportion of papers (b) of photocatalysts with π–π interaction based on keyword search of “π–π” and “photocata*” in the Web of Science (as of September 1, 2024). | |
8.1. π–π interactions: synergistic effects of photocatalysts and aromatic organics
While discussing the application of π–π interaction in the field of photocatalysis, the degradation of organic pollutants, aromatic organic conversion and biomass conversion are three major reactions largely relying on the π–π interaction between the photocatalyst and the aromatic organic matter, which has been widely and mostly discussed and studied by researchers.
8.1.1 Pollutant degradation.
In the photocatalytic degradation of pollutants, π–π interactions between different semiconductors and between semiconductors and pollutants, and synergistic effects of these interactions provide various advantages that contribute to the efficiency and effectiveness of pollutant degradation.240–243,268–272 The main advantages of π–π interactions between semiconductors are enhanced charge transfer, increased surface area, and expanded light absorption. The main advantages of π–π interactions between semiconductors and pollutants are enhanced pollutant adsorption, optimized selective adsorption and improved reactivity. The synergistic effect of the two above-mentioned π–π interactions can lead to the efficient removal of difficult-to-degrade organic pollutants (e.g. polycyclic aromatic hydrocarbons, dyes, drugs, and aromatic pesticides).206,208,225–235 For instance, Sun et al. developed a new supramolecular photocatalyst, hp-PDI-NA, by combining PDI (perylene diimide) with nicotinic acid (NA). This structure boosts the transport and separation of photogenerated carriers through reduced steric hindrance and enhanced π–π interaction, thereby resulting in a 3.5 times higher phenol degradation efficiency under the visible illumination than that of nano-PDI (Fig. 15a).122 Li et al. synthesized a new 0D/2D S-type heterojunction photocatalyst via the π–π conjugated self-assembly of nitrogen-doped carbon dots (N-CDs) and sulfur-doped carbon nitride (S-C3N4). This heterojunction, stabilized by π–π interactions, effectively promotes the separation and migration of photogenerated charges, substantially boosting the photocatalytic degradation and hydrogen production from water (Fig. 15b).273 Ning et al. substantially ameliorated the degradation efficiency of 2,4-dichlorophenol by synthesizing a 3D/0D structure Co–N–C/SA-TCPP photocatalyst, achieving a degradation rate 10.9 times higher than that of SA-TCPP alone and enhancing the oxygen production rate by 1.9 times. The enhancement is imputed to the π–π interaction between SA-TCPP and Co–N–C, which facilitates photogenerated charges’ movement. Additionally, the cobalt nanoparticles and nitrogen-doped carbon matrix in Co–N–C serve as electron traps and expedite electron transport (Fig. 15c).274 Tian et al. synthesized a series of dinuclear metal phthalocyanines (M2Pc2(CP)4, M = Fe2+, Co2+, and Zn2+) and encapsulated them on the surface of multi-walled carbon nanotubes (MWCNTs) to enhance the desulfurization performance via a facile “in situ hydrothermal” approach. The π–π interaction plays a fundamental role in the constitution of MWCNTs/M2Pc2(CP)4 composites, which not only enhances the dispersion of M2Pc2(CP)4 on the surface of MWCNTs, but also significantly improves the photocatalytic performance of the composites by increasing the photogenerated charge separation efficiency (Fig. 15d).275
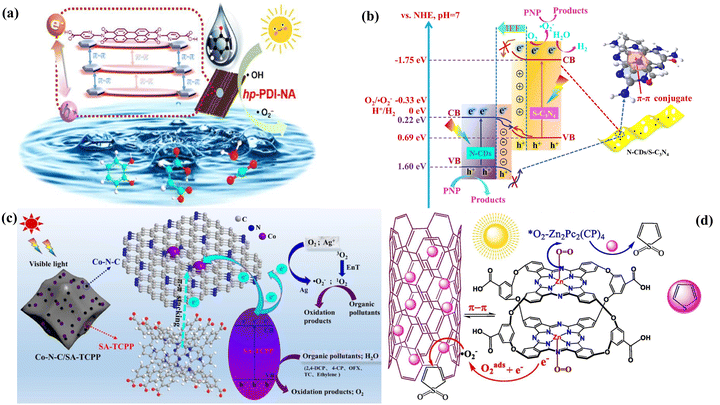 |
| Fig. 15 (a) Mechanism chart of phenol's hp-PDI-NA photocatalytic elimination. ((a) Reproduced from ref. 122 with permission from Elsevier, Copyright 2022). (b) Photo-reactivity mechanism diagram of N-CDs/S-C3N4 constructing S-type heterojunction through π–π interaction. ((b) Reproduced from ref. 273 with permission from Elsevier, Copyright 2022). (c) Schematic of the mechanism of photocatalytic degradation of various pollutants by Co–N–C/SA-TCPP composite materials. ((c) Reproduced from ref. 274 with permission from Elsevier, Copyright 2022). (d) Photo-reactivity mechanism chart of MWCNTs/Zn2Pc2(CP)4. ((d) Reproduced from ref. 275 with permission from Springer, Copyright 2021). | |
The above paragraph mainly describes the constitution of powerful built-in electric fields dictated by π–π interactions between semiconductors in the photocatalytic degradation of pollutants, and the next part of the work is more inclined to describe the significance of π–π interactions between semiconductors and pollutants in the degradation of pollutants.135,236–240 Wang et al. developed an organic–inorganic hybrid heterostructure, NC@PDI/MZO, consisting of a benzimidazole-modified nitrogen-doped carbon skeleton and manganese-doped zinc oxide.276 DFT calculations indicated that the adsorption energy of tetracycline (TC) on this material predominantly originates from hydrogen bonding (−140.0 kJ mol−1) and π–π interactions (−61.5 kJ mol−1), totaling an adsorption energy of −211.2 kJ mol−1 (Fig. 16a). NC@PDI/MZO demonstrated exceptional photocatalytic degradation of TC, achieving a degradation rate of 99.9% for 50 mg L−1 TC within 40 minutes of illumination (Fig. 16b). This high efficiency is largely due to the pivotal role of π–π interactions and hydrogen bonds in forming the composite material, which enhances TC adsorption and thus photocatalytic degradation efficiency via synergistic effects. The formation of the S-scheme heterostructure also aids in the separation and transfer of photogenerated carriers, further boosting photocatalytic performance (Fig. 16c). Zhang et al. prepared a novel donor–acceptor-type graphitic phase carbon nitride (D–A g-C3N4) to boost the photocatalytic degradation of bisphenol A (BPA) by an extended π-conjugation system. DFT calculations revealed that the extended π-conjugation system of D–A g-C3N4 enhances the π–π interactions between the catalyst and BPA molecules, which contributes to the adsorption of BPA and the electron transfer, thereby improving the photocatalytic degradation efficiency (Fig. 16d).233 Song et al. enhanced the adsorption and photocatalytic degradation properties of tetracycline hydrochloride (TC) by modifying TPE-2Py on the surface of the metal–organic framework (MOF) MIL-125(Ti). π–π conjugation is a key factor in the adsorption of TC by TPE-2Py@DSMIL-125(Ti), which helps to enhance the material-TC molecular interactions, thereby improving the photocatalytic performance (Fig. 16e).277 Wang et al. developed a perylene-based amphiphilic micelle for the efficient adsorption and photodecomposition of BPA in aqueous solutions. This micelle system exhibited an eightfold increase in BPA degradation efficiency compared to perylene nanorods. This enhancement is credited to the partially ordered stacking structure of perylene units formed through self-assembly within the micelle. This π–π stacking augments visible light absorption, catalytic ability, and cycling stability. Additionally, the micelle structure facilitates the production and separation of e−–h+ pairs in the photocatalytic reaction. The micelle's adsorption capacity for BPA is bolstered by hydrophobic interactions, hydrogen bonding, and π–π interactions (perylene units and BPA), enriching BPA molecules on the micelle surface and significantly boosting the degradation efficiency (Fig. 16f).232 Zhang et al. investigated the mechanism of simultaneous removal of enoxacin (ENO) and hexavalent chromium (Cr(VI)) using ultrathin g-C3N4 during photocatalysis, highlighting the significant role of the antibiotic's molecular structure. DFT calculations indicated that ENO molecules preferentially adsorbed onto the surface of g-C3N4 by π–π interactions, facilitating crucial electron transfer and enhancing synergistic removal effects. These findings emphasize π–π interactions' decisive function in the photocatalytic elimination of pollutants and provide a theoretical foundation for designing and optimizing photocatalysts (Fig. 16g).278
 |
| Fig. 16 (a) Model diagram of hydrogen bond adsorption, π–π adsorption, hydrogen bond adsorption and π–π interaction co-adsorption of NC@PDI/MZO sample for TC. (b) Performance of synergistic photocatalytic removal of TC by adsorption of different samples. (c) Mechanism diagram of NC@PDI/MZO adsorption synergistic photocatalytic removal of TC. ((a)–(c) Reproduced from ref. 276 with permission from Elsevier, Copyright 2024). (d) Mechanism diagram of D–A g-C3N4 enhancing the π–π adsorption with BPA and efficiently photodegrading BPA. ((d) Reproduced from ref. 233 with permission from Elsevier, Copyright 2022). (e) Mechanism diagram of TPE-2Py@DSMIL-125(Ti) coupling various synergistic effects to achieve efficient photodegradation of TC. ((e) Reproduced from ref. 277 with permission from Elsevier, Copyright 2023). (f) Mechanism diagram of micelles coupling various synergistic effects to achieve efficient photodegradation of BPA. ((f) Reproduced from ref. 232 with permission from Wiley-VCH, Copyright 2022). (g) Mechanism diagram of ultra-thin g-C3N4-coupled Cr(VI) reduction to achieve efficient photodegradation of ENO. ((g) Reproduced from ref. 278 with permission from Elsevier, Copyright 2022). | |
8.1.2. Organic synthesis.
π–π interactions play a vital role in photocatalytic organic synthesis by enhancing the concentration of substrates near the active sites of photocatalysts. This is achieved by promoting the adsorption and activation between substrates and photocatalysts, which, in turn, improve the reaction efficiency and selectivity. These interactions help stabilize the transition state of reactions, reduce activation energy, facilitate electron transfer, accelerate reaction rates, and increase product yields.24,33,257,279–284 Specifically, π–π interactions exhibit high selectivity in the activation of aromatic or conjugated substrates, facilitating the synthesis of complex molecules. Additionally, these interactions enable the efficient conversion of substrates with various functional groups and support a range of photocatalytic reactions, including carbon–carbon bond constitution, carbon–heteroatom bond constitution, and redox reactions.24,33,257,279–284 Shi et al. developed a two-dimensional sp2 carbon-conjugated covalent organic framework (Por-sp2c-COF) and synergistically combined it with a pyridine-anchored TEMPO cocatalyst (Fig. 17a), enhancing photocatalytic performance via π–π stacking. This configuration enables the pyridine moiety of the cocatalyst to intercalate within the Por-sp2c-COF layers, boosting the efficiency of photoinduced hole transport and enhancing stability for selective sulfide to sulfoxide oxidation under 623 nm red LED irradiation (Fig. 17b). The photogenerated holes are effectively transferred to the TEMPO cocatalyst via π–π interactions, enhancing the photocatalytic activity. The HOMO state of the TEMPO portion is situated in the TEMPO moiety, while the LUMO state is focused on the COF, which suggests enhanced spatial charge separation. Electrons move from Por-sp2c-COF to O2 to generate ˙O2−, while holes are injected into pyridine-2-TEMPO to form a reversible transition state, facilitating the oxidation of sulfide to sulfoxide (Fig. 17c).285 Liu et al. developed crystalline triazineimine polymers (PTI) featuring nitrile groups to boost the photocatalytic regeneration of nicotinamide cofactor (NADH) efficiency via π–π interactions.170 The study highlighted that strong π–π interactions between the optimized PTI (D-PTI-350) and NADH facilitated charge transfer, achieving a NADH-to-NAD+ conversion efficiency of 98.2% within 60 minutes. This performance substantially exceeded that of both the original PTI (88.1%) and amorphous poly carbon nitride (PCN, 56.8%) (Fig. 17d). This enhancement is attributed to the π-electron cloud of the aromatic ring in NADH, which promotes directional transport of photogenerated charges and ameliorates the energy conversion efficiency in the photo-enzymatic coupling system (Fig. 17e). The research presents a novel approach to designing photo-enzymatic catalytic systems for biological cofactor regeneration, leveraging extended π-conjugated systems and noncovalent π–π stacking interactions, thus offering fresh perspectives for covalent-dependent photo-enzymatic catalysis development.
 |
| Fig. 17 (a) Theoretical calculation results of π–π interaction between Por-sp2c-COF and pyrene-2-TEMPO. (b) Synergistic photocatalytic red light-induced selection of Por-sp2c-COF and pyrene-2-TEMPO performance of selective aerobic oxidation of phenyl sulfide in different environments. (c) Reaction mechanism diagram of synergistic photocatalytic selective aerobic oxidation of phenyl sulfide by Por-sp2c-COF and pyrene-2-TEMPO. ((a)–(c) Reproduced from ref. 285 with permission from Wiley-VCH, Copyright 2021). (d) Comparison of the NADH adsorption capacity of different samples under dark conditions. (e) Reaction mechanism diagram of D-PTI-350 photocatalytic oxidation of NADH. ((d) and (e) Reproduced from ref. 170 with permission from the Royal Society of Chemistry, Copyright 2024). | |
8.1.3. Biomass reforming.
In photocatalytic biomass reforming, π–π interactions have several advantages that contribute to the efficiency and selectivity of the process, some of which overlap with the photocatalytic organic synthesis part.258,286 π–π interactions significantly enhance photocatalytic biomass conversion by improving substrate adsorption on the photocatalyst surface and concentrating molecules near active sites, leading to more efficient and selective reactions.29,258 These interactions facilitate electron transfer, enhance hydrogenation or deoxygenation reaction efficiencies, lower activation energy, speed up reaction rates, and boost product yields.244,286 Specifically, for biomass with aromatic or conjugated structures such as lignin, π–π interactions effectively activate these substrates, facilitating selective functionalization or depolymerization.244,286 This capability enables photocatalysis to produce not only biofuels and platform chemicals but also high-value products, positioning π–π interactions as a key mechanism for enhancing biomass conversion under visible light. Xu et al. effectively synthesized S-type heterojunction photocatalysts of p-CN/P-PDI for efficient photocatalytic cleavage of a lignin model, using polyhedral oligomeric sesquisiloxanes (POSS) at both ends of PDI. The incorporation of POSS strengthens the π–π interactions with p-CN, promoting the formation of stable heterojunctions. At the same time, due to the π–π interaction between certain aromatic molecules present in the β-O-4 model and the electron-rich p-CN, this would contribute to molecular activation. As demonstrated in Fig. 18a and b, p-CN/p-PDI-3 accomplished more than 99% conversion of lignin β-O-4 model molecules with 96% C–C bond selectivity. This high efficiency is primarily due to the crucial roles of photogenerated h+ and reactive oxygen species (ROS) in the photocatalytic oxidization process (Fig. 18c). By enhancing the π–π interaction between different materials and the π–π interaction between materials and lignin model molecules, this study provides a new idea for designing efficient photocatalytic polymerization models of activated and depolymerized lignin.107 Li et al. enhanced the photocatalytic efficiency of carbon nitride (g-C3N4-Cl-A5) by means of Cl doping and high-temperature oxidation, significantly boosting the cleavage of lignin's β-O-4 bond and increasing the yield of benzaldehyde (1.74 mg h−1) (Fig. 18d). This enhancement was credited to the superoxide radicals' heightened generation and the improved efficiency of photogenerated electron transport. The π–π conjugated planar constitution of g-C3N4 facilitated the adsorption of benzene rings from lignin by π–π stacking. This noncovalent interaction enhanced the targeted transport of photogenerated charges and increased the energy conversion efficiency, contributing to the superior photocatalytic performance.29 Liu et al. discovered that the mesoporous graphitic carbon nitride (mpg-C3N4) photocatalyst also formed the favorable π–π interactions with the lignin model molecule, finally realizing 90% conversion rate of the lignin model molecule through photocatalytic oxidation under visible light (cleavage of the C–C structure: β-O-4 and β-1 bonds) (Fig. 18e).244
 |
| Fig. 18 (a) Conversion of 1 using p-CN/P-PDI with different POSS-PDI contents in 3 h. (b) Conversion of 1 using p-CN/P-PDI-3 in different irradiation times. Reaction conditions: 10 mg of catalyst, 1 mL of CH3CN, 0.006 mmol of substrate 1, Xe lamp (300 W), O2 (1 atm). (c) Reaction mechanism for the photocatalytic selective cleavage of β-O-4 model compound on p-CN/P-PDI S-type heterojunction. ((a)–(c) Reproduced from ref. 107 with permission from Wiley-VCH, Copyright 2023). (d) Mechanism diagram of g-C3N4-Cl-A5 photocatalytic decomposition of lignin model compounds. ((d) Reproduced from ref. 29 with permission from Elsevier, Copyright 2023). (e) Schematic of the mechanism of selective conversion of lignin model compounds by mesoporous carbon nitride. ((e) Reproduced from ref. 244 with permission from the American Chemistry Society, Copyright 2018). | |
8.2. π–π interaction: the photocatalyst itself and the synergy between different photocatalysts
Although reactants such as water and CO2 do not have aromatic structures, the aromatic or conjugated structures contained in the photocatalyst can stabilize the active center of the catalyst through π–π interaction and improve the catalytic efficiency. Therefore, even if the reactants themselves do not participate in the π–π interaction, this interaction still plays a key role in the photocatalysis process, mainly by regulating the properties of the catalyst to affect the reaction efficiency and selectivity.
8.2.1 Water splitting.
In the photocatalytic water splitting reaction, which employs sunshine to convert water into hydrogen and oxygen, π–π interactions contribute to the efficiency and effectiveness of the process.42,287 π–π interactions boost photocatalytic water splitting primarily by establishing a built-in electric field, modifying the interfacial electron density of composite materials to enhance charge separation, lowering the reaction energy barriers associated with hydrogen evolution, and facilitating proton transfer.155,162,194,249,273,288 Through π–π interactions, PTCDA and PTA formed a close-contact heterostructure by face-to-face arrangement at the molecular level, which strengthened the electron communication between PTA and PTCDA. Fig. 19a demonstrates that the PTCDA/PTA heterostructure exhibits much higher photocatalytic hydrogen production rate (45.06 mmol g−1 h−1) than PTA and PTCDA alone. The calculation results in Fig. 19b show that the LUMO of PTCDA/PTA at the interface is distributed on the PTA, while the HOMO is principally located on the PTCDA, indicating that PTA and PTCDA serve as an electron donor and electron acceptor, respectively. Fig. 19c reveals that the particular asymmetric planar structure formed via π–π stacking increases the dipole moment to generate a giant internal electric field, which significantly facilitates the separation and migration of photogenerated charges.249 π–π interactions also promote the transport of photogenerated electrons from ZnCd-ZIF-8 to GO, enhancing the charge separation efficiency and thereby significantly improving the photocatalytic hydrogen production efficiency (Fig. 19d and e).193 Additionally, π–π interactions help the self-organization of the thiophene-based trimers (TBTs) to form ordered structures and tight stacking, which increases the π–π orbital overlap and thus affects the size and crystallinity of the aggregates. TBTs with tuned aggregation via methyl side-chain engineering with small size and higher crystallinity have more surface-active sites, shortened charge transfer distance, and fewer charge recombination centers, exhibiting remarkably improved photocatalytic activity for hydrogen generation (Fig. 19f–h).289
 |
| Fig. 19 (a) Photocatalytic H2 evolution performance of distinct samples underneath simulated sunlight irradiation. (b) Frontier molecular orbital distribution and dipole moment within different materials and within stacked units between each other. (c) Schematic of the charge transfer mechanism on PTCDA/PTA photocatalyst. ((a)–(c) Reproduced from ref. 249 with permission from Wiley-VCH, Copyright 2023). (d) Performance of photocatalytic hydrogen production of different GO/ZnCd-ZIF-8 samples. (e) Mechanism diagram of GO/ZnCd-ZIF-8 improving photocatalytic hydrogen production through π–π interaction. ((d) and (e) Reproduced from ref. 193 with permission from the American Chemistry Society, Copyright 2024). (f) Performance of photocatalytic hydrogen production from aggregates under UV-visible light and visible light irradiation. (g) Comparison of photocatalytic hydrogen production rates of different aggregates under UV-visible light and visible light. (h) Microaggregate synthesis process and possible photocatalytic hydrogen production mechanism. ((f)–(h) Reproduced from ref. 289 with permission from Wiley-VCH, Copyright 2024). | |
In addition to hydrogen production reactions, π–π interactions have also demonstrated promising applications in photocatalytic oxygen evolution and total water splitting. Strong interfacial π–π interactions can push the spontaneous adsorption of perylene diimide polymer (PDIP) onto carbon paper (CP), resulting in the redistribution of interfacial electrons, constitution of built-in electric field, narrowing of the band gap, downward shift of valence band edges, and prolongation of photogenerated charge carrier lifetime. The constructed PDIP/CP photocatalyst displays considerable boosted photocatalytic water oxidization activity (Fig. 20a and b).290 A conceivable mechanism of the PDIP/CP photocatalyst sheet was recommended (Fig. 20c): the PDIP generates photogenerated e− and h+ through visible light excitation; the photogenerated e− move to the CP that is forced through the built-in electric field and are eaten through Ag+ ions’ reduction; meantime, photogenerated h+ immediately oxidizes adsorbed water molecules to generate oxygen molecules. Moreover, π–π interactions can collaborate with metal ligands to construct via self-assembly nanorods (NRs) of peroxydimethylene diimide (PDI), which demonstrates an excellent photocatalytic oxygen production performance of CuPDI-Hm (25
900 μmol g−1 h−1), owing to the optimized molecular alignment, built-in electric fields, and improved charge separation efficiency (Fig. 20d and e).291 However, excessively strong π–π interactions may be detrimental to photocatalytic water splitting because it may lead to close packing of molecules or layers, resulting in strong intermolecular forces that hinder the separation and transport of photogenerated charge carriers. By optimizing the π–π stacking interactions by changing the conjugation system of g-C3N4 and providing more channels for charge and mass transfer by forming a large number of in-plane holes, alkali treatment can enhance the activity of photocatalytic water decomposition over Pt/CQDs/CN (B) by 24.6 times (Fig. 20f–h). This means that a suitable π–π interaction strength is critical for photocatalysis in these π–π conjugated systems.292
 |
| Fig. 20 (a) Schematic of the preparation process of PDIP/CP photocatalyst. (b) Comparative effect of photocatalytic oxygen production performance of PDIP and PDIP/CP. (c) Photocatalytic oxygen production mechanism diagram of PDIP/CP composite material. ((a)–(c) Reproduced from ref. 290 with permission from Elsevier, Copyright 2024). (d) Schematic of PDI-Hm assembly connected through Cu-imidazole coordination and SEM image of crystallized CuPDI-Hm nanorods, (e) photocatalytic production of CuPDI-Hm nanorods at different AgNO3 concentrations. ((d) and (e) Reproduced from ref. 291 with permission from Wiley-VCH, Copyright 2023). (f) Energy band structure diagrams of different samples. (g) Synthetic routes of different CN samples and mechanism diagrams of their photoreaction processes. (h) Performance of photocatalytic total water splitting of different CN and CQDs/CN composite materials. ((f)–(h) Reproduced from ref. 292 with permission from Elsevier, Copyright 2018). | |
8.2.2. H2O2 generation.
In photocatalytic hydrogen peroxide (H2O2) generation, π–π interactions offer several advantages that contribute to the efficiency and effectiveness of the process.90,293 In addition to the general benefits of π–π interactions in photocatalytic systems (such as enhancing charge separation, promoting electron transfer, increasing the utilization of visible light, and improving catalyst stability), these interactions offer unique advantages including the selective adsorption and activation of oxygen molecules.294,295 Essentially, this interaction helps to align the reactant molecules more effectively at the photocatalyst surface, optimizing the reaction conditions for H2O2 production. Shan et al. substantially ameliorated the photocatalytic H2O2 generation performance through introducing a small amount of dopamine (PDA) between carboxylated cellulose nanofibers (CNFs) and graphitic carbon nitride (CN) to form a composite material with an extended hydrogen-bond/π-bond network (CN/CNFP, Fig. 21a).294Fig. 21b and d demonstrate that CN/CNFP exhibits excellent photocatalytic H2O2 generation performance, optimally up to 130.7 μmol L−1 h−1, which is a great deal more excellent than that of CN/CNF (75.2 μmol L−1 h−1) and original CN (20.2 μmol L−1 h−1). Such improvement was mainly attributed to the enhanced O2 adsorption capacity of CNFP (a composite of dopamine and CNF) via multiple π–π stacking effect/hydrogen bonding with CN, and the enhanced photogenerated charge transport and separation efficiency, which facilitated the superior photocatalytic H2O2 production (Fig. 21e). Guo et al. developed PDA@BCN S-type heterostructures by incorporating 3,4,9,10-perylene tetracarboxylic dianhydride (PTCDA) into BCN, significantly enhancing light absorption through π–π stacking interactions and achieving a H2O2 yield of up to 285.47 μmol h−1 g−1. The π–π stacking interactions between PTCDA and BCN are the primary driving force for the formation of the PDA@BCN heterostructures, facilitating the generation and efficient separation of photogenerated carriers under the visible light. Additionally, the S-type heterostructure between PTCDA and BCN optimizes charge transport and separation, further boosting the photocatalytic production of H2O2 (Fig. 21f).295 Yang et al. constructed composites of polyaniline (PANI) and g-C3N4 to achieve efficient photocatalytic H2O2 production.296 This was primarily owing to the forceful π–π interactions between the tri-s-triazine units in the g-C3N4 structure and PANI that helped to reduce charge recombination and enhance electron transport, which were crucial for H2O2 generation (Fig. 21g). In conclusion, π–π interactions played a key role in this study, which not only facilitated electron transport and separation, but also contributed to the enhancement of light absorption and conductivity of the photocatalytic materials, which significantly improved the photocatalytic H2O2 generation efficiency.
 |
| Fig. 21 (a) Synthesis process of CN/CNFP composite materials and the types of effects involved. (b) Photocatalytic production of H2O2 from distinct CN/CNF composites. (c) Photocatalytic production of H2O2 from distinct CN/CNFP composites. (d) Comparison of photocatalytic production of H2O2 by CN, CN/CNF and CN/CNFP. (e) Reaction mechanism diagram of photocatalytic production of H2O2 by CN/CNFP. ((a)–(e) Reproduced from ref. 294 with permission from Elsevier, Copyright 2024). (f) Mechanism chart of photocatalytic synthesis of H2O2 by the PDA@BCN composite constructed through π–π interaction. ((f) Reproduced from ref. 295 with permission from Elsevier, Copyright 2023). (g) Mechanism diagram of PANI/CN photocatalytic synthesis of H2O2. ((g) Reproduced from ref. 296 with permission from the Royal Society of Chemistry, Copyright 2020). | |
8.2.3. Carbon dioxide reduction.
Photocatalytic carbon dioxide (CO2) reduction is an effective way to convert solar energy into sustainable energy, wherein the selection of photocatalytic materials plays a crucial role. In recent years, π–π interactions are discovered to play a tremendous role in photocatalytic CO2 reduction.297–304 π–π interactions optimize the electron transfer efficiency and reaction selectivity in the photocatalytic CO2 reduction process primarily by constructing directional built-in electric fields, influencing intramolecular and intermolecular conjugation, forming carrier transmission channels, and accelerating the transfer of photoexcited electrons.150,218,299,305 Hierarchical π–π interactions enable the self-assembly of metal–organic layers to form a quadruple π-bonded metal–organic layer (πMOL) with a tessellated lattice of about 1.0 nm thickness (Fig. 22a), on which the catalytic selectivity at individual metal sites could be controlled for efficient CO2 reduction reactions (CO2RRs) and hydrogenolysis reactions (HERs).306 In saturated CO2 acetonitrile aqueous solutions, Fe-πMOL achieved an efficient CO2RR with a yield of about 3.98 mmol g−1 h−1 and a selectivity of 91.7% towards CO. In contrast, isomeric Co-πMOL as well as mixed-metal FeCo-πMOL exhibited excellent activity toward the HER under similar conditions (Fig. 22b). This was mainly attributed to the π–π interactions contributing to the uniform distribution of monometallic active sites in the 2D MOLs and the facilitated electron transfer via spatial electron transport pathways, which were essential for the improvement of the photocatalytic efficiency (Fig. 22c). The π–π interaction-driven formation of the heterojunction between 2D carbon nitride (2D CN) and a covalent organic framework based on triazine (COF-TD) accelerates charge carriers’ migration and consequently improves the production of CO (Fig. 22d and e).307 π–π interactions, together with H-bonding, led to a close-contact Z-type heterojunction between ZnPc and PDI, which greatly enhanced the photogenerated charge transport and separation for the conversion of carbon dioxide (CO2) into solar fuel (Fig. 22f).99 π–π interactions between 4,4′-diaminostyrene-2,2′-disulfonic acid (DAS) and 4,4′-dinitrostyrene-2,2′-disulfonic acid disodium salt (DNS) in a parallel molecule arrangement promote the transfer of photoexcited electrons to the host anions when co-intercalated in Ni2Mn-LDHs, to participate in CO2 reduction, while the photogenerated holes are transferred to the HOMO of the guest anion to oxidize H2 to release H2O (Fig. 22g).308 Wang et al. constructed a molecular model to study the π–π interaction between the photosensitizer and the catalyst by introducing pyridine groups between them. This design strategy enhanced the interaction between the photosensitizer and the catalyst. The coplanar π–π interaction between IrPPPY and Co-PYN5 enabled the photocatalytic CO2-to-CO conversion with an excellent apparent quantum efficiency of 14.3 ± 0.8% and a high selectivity of 98%. This is mainly due to the speedy transport of the excited-state electrons from photoexcited IrPPPY to Co-PYN5 via coplanar π–π interactions, generating oxidized IrPPPY and one-electron-reduced Co-PYN5. This process significantly improves the performance of photocatalytic CO2 reduction (Fig. 22h).218 In general, the electron transfer channel constructed by π–π interaction is conducive to the efficient and selective photoreduction of CO2.
 |
| Fig. 22 (a) Schematic of the formation of a single layer of πMOL through solvent-assisted exfoliation. (b) Comparison of the experimental results of the photocatalytic CO2/H2O reduction activity of Co-πMOL, Fe-πMOL, and FeCo-πMOL. (c) Photocatalytic reduction of the studied FeCo-πMOL electron transfer pathways for CO2 and H2O. ((a)–(c) Reproduced from ref. 306 with permission from Wiley-VCH, Copyright 2023). (d) Composite material formed by COF-TD and 2D CN through π–π interaction. (e) Comparison of the yield effects of photoreduction of CO2 to CO/CH4 for distinct 2D CN-COF samples. ((d) and (e) Reproduced from ref. 307 with permission from Elsevier, Copyright 2021). (f) Mechanism chart of ZnPc/P-PDI's CO2 photoreduction. ((f) Reproduced from ref. 99 with permission from Elsevier, Copyright 2021). (g) Mechanism diagram of Ni2Mn-LDHs-DAS, DNS constructed through π–π interaction for the photoreduction of CO2. ((g) Reproduced from ref. 308 with permission from Wiley-VCH, Copyright 2021). (h) Mechanism diagram of IrPPPY/Co-PYN5 photoreduction of CO2 to generate CO. ((h) Reproduced from ref. 218 with permission from the American Chemistry Society, Copyright 2022). | |
9. Summary and prospects
This review primarily delineates the utilization of π–π interactions within photocatalytic systems, elucidating the various categories and detection techniques for π–π interactions. It also presents an overview of the prevalent photocatalysts capable of engaging in π–π interactions, detailing their types and operational mechanisms. This review further examines the influence of π–π interactions on the characteristics of photocatalysts and the overall photocatalytic system. Moreover, it highlights numerous strategies that have been engineered to augment photocatalytic efficiency via π–π interactions. Additionally, this review acknowledges the promising outcomes of π–π interactions in diverse photocatalytic application frameworks. While π–π interactions offer significant benefits in the realm of photocatalysis, this review also addresses the challenges encountered in their practical application and looks forward to their prospects. The following are the challenges and prospects of π–π interactions for photocatalysis:
(1) Consistently reproducing the desired π–π interactions at an appropriate level in different batches of photocatalysts may be a challenge. Achieving precise control over the assembly of π-conjugated systems to ensure optimal π–π interactions remains a challenge. Therefore, the standardization of synthetic methods and improved characterization techniques is essential to enhance the reproducibility of π–π interactions in photocatalytic materials aiming to achieve a better control of the arrangement of aromatic units. The design of photocatalysts with specific π–π interactions can be tailored by adjusting the energy level to match with the energy of the incident photon, thus enabling the customized design of photocatalytic materials. Among them, organic/conjugated photocatalysts provide highly controllable π–π interactions due to their strong designability, which can significantly improve the selectivity, efficiency and stability of photocatalytic systems. By adjusting the intensity and arrangement of the π–π interaction, the photocatalytic reaction path can be accurately controlled, the reaction efficiency can be optimized and the stability of the catalyst can be enhanced. Although the regulation of π–π interaction still faces certain challenges, through molecular design and novel synthesis methods, these problems can be overcome and the performance of photocatalytic materials can be further improved, and the development of photocatalytic technology can be promoted.
(2) Tuning the strength of π–π interactions to achieve the desired balance between stability and reactivity is a challenge. We need to understand the factors that affect the strength of the interactions and employ computational methods that can help fine-tune the π–π interactions for specific applications. Prolonged exposure to light and harsh reaction conditions may lead to degradation of the π-conjugated system and affect the stability of the photocatalyst. The introduction of stabilizing groups or protective layers can improve the photostability of π–π interactions in photocatalytic materials. The rational construction of cocatalysts is an effective strategy to swiftly transfer photogenerated charge carriers from the bulk to the surface and then to the reactants, thus reducing the photo-corrosion. Moreover, appropriate choice of the reaction medium including the solvent, pH and gas atmosphere as well as suitable sacrificial reagents will benefit the stability of these photocatalysts.
(3) Optimizing π–π interactions enables the development of photocatalysts that exhibit tailored selectivity and enhanced efficiency, facilitating precise manipulation of intricate organic transformations, including selective oxidation, reduction, and isomerization. In multi-stage photocatalytic processes, fine-tuning these interactions can steer reaction trajectories towards achieving high selectivity and product yields, exemplified by the targeted synthesis of specific compounds in photocatalytic systems. The rational design of photocatalytic systems can improve the selectivity by precisely controlling the molecular structure and electronic properties, which allows us to optimize the redox ability of the catalyst, free radical generation, and adsorption configuration of the reactants. It is very promising to design photocatalysts for different applications to realize the versatility of π–π interactions in photocatalysis. Combining π–π interactions with other types of interactions (e.g., hydrogen bonding or metal–ligand interactions) may also be an effective strategy.
(4) In situ and operando characterization techniques such as infrared spectroscopy, Raman spectroscopy, nuclear magnetic resonance, transient absorption spectroscopy, X-ray diffraction, and electron microscopy (TEM and SEM) are poised to significantly advance our understanding of π–π interactions in materials science. These techniques allow for real-time monitoring and detailed analysis at the molecular level, which is crucial for revealing the characteristics, vibrational modes, electron transfers, interaction strengths, and changes in crystal structure associated with π–π stacking in organic semiconductors, carbon-based materials, MOFs, and COFs. As these methods evolve, they will provide a robust scientific basis for the comprehensive study of π–π interactions, furthering material design and optimization.
(5) In future research, the combined use of density functional theory (DFT) and machine learning (ML) is expected to significantly accelerate new photocatalyst discovery and also enhance the exploration of the mechanisms underlying π–π interactions. DFT will provide essential insights into the energy, geometric configuration, and electronic distribution of π–π interactions by accurately simulating the electronic structures of molecules. It will also optimize the geometric structures of stacked assemblies to elucidate electronic mechanisms. Concurrently, ML will expedite DFT calculations through data-driven predictive models, identify the characteristics of π–π interactions, and facilitate the screening of potential molecules from extensive molecular libraries. This integrated application of DFT and ML will profoundly advance the understanding of π–π interactions, accelerate the development of novel materials and catalysts, and bolster research in materials science and chemistry.
In conclusion, while there are challenges in exploiting and optimizing π–π interactions in photocatalysis, ongoing research and advances in materials science, synthesis techniques, and characterization methods offer promising prospects for addressing these challenges and unlocking the full potential of π–π interactions in photocatalysis.
Data availability
No primary research results, software or code have been included and no new data were generated or analysed as part of this review.
Conflicts of interest
There are no conflicts to declare.
Acknowledgements
This research was financially sustained through the Natural Sciences and Engineering Research Council of Canada-Discovery Grant (Canada).
Notes and references
- W. Zhang, W. Huang, B. Wu, J. Yang, J. Jin and S. Zhang, Coord. Chem. Rev., 2023, 491, 215235 CrossRef CAS.
- A. Chawla, A. Sudhaik Sonu, P. Raizada, T. Ahamad, Q. V. Le, V.-H. Nguyen, S. Thakur, A. K. Mishra, R. Selvasembian and P. Singh, Coord. Chem. Rev., 2023, 491, 215246 CrossRef CAS.
- V. Thakur, S. Singh, P. Kumar, S. Rawat, V. Chandra Srivastava, S.-L. Lo and U. Lavrenčič Štangar, Chem. Eng. J., 2023, 475, 146100 CrossRef CAS.
- M. Wang, H. Yu and K. Yu, Chem. Eng. J., 2023, 470, 144100 CrossRef CAS.
- L. Jing, Y. Xu, M. Xie, Z. Li, C. Wu, H. Zhao, N. Zhong, J. Wang, H. Wang, Y. Yan, H. Li and J. Hu, Small, 2024, 20, 2304404 CrossRef CAS PubMed.
- I. Ahmad, Y. Zou, J. Yan, Y. Liu, S. Shukrullah, M. Y. Naz, H. Hussain, W. Q. Khan and N. R. Khalid, Adv. Colloid Interface Sci., 2023, 311, 102830 CrossRef CAS PubMed.
- H. Huang, L. Jiang, J. Yang, S. Zhou, X. Yuan, J. Liang, H. Wang, H. Wang, Y. Bu and H. Li, Renewable Sustainable Energy Rev., 2023, 173, 113110 CrossRef CAS.
- Y. Sun, Y. Ahmadi, K.-H. Kim and J. Lee, Renewable Sustainable Energy Rev., 2022, 170, 112967 CrossRef CAS.
- S. Naghdi, M. M. Shahrestani, M. Zendehbad, H. Djahaniani, H. Kazemian and D. Eder, J. Hazard. Mater., 2023, 442, 130127 CrossRef CAS PubMed.
- F. Deng, J. Peng, X. Li, X. Luo, P. Ganguly, S. C. Pillai, B. Ren, L. Ding and D. D. Dionysiou, J. Clean. Prod., 2023, 416, 137957 CrossRef CAS.
- P. Bhavani, D. Praveen Kumar, J. Suk Yoo, M. Hussain, S. Weon, W. Kim and Y.-K. Park, Chem. Eng. J., 2023, 467, 143429 CrossRef CAS.
- I. Ahmad, A. Idrees, N. S. Alatawi, S. B. Ahmed, M. Shaban and Y. Y. Ghadi, Adv. Colloid Interface Sci., 2023, 321, 103032 CrossRef CAS PubMed.
- L. Jing, Y. Xu, M. Xie, Z. Li, C. Wu, H. Zhao, J. Wang, H. Wang, Y. Yan, N. Zhong, H. Li and J. Hu, Nano Energy, 2023, 112, 108508 CrossRef CAS.
- Z. Li, L. Zhang, Y. Liu, C. Shao, Y. Gao, F. Fan, J. Wang, J. Li, J. Yan, R. Li and C. Li, Angew. Chem., Int. Ed., 2020, 59, 935–942 CrossRef CAS PubMed.
- Z. Li, R. Li, H. Jing, J. Xiao, H. Xie, F. Hong, N. Ta, X. Zhang, J. Zhu and C. Li, Nat. Catal., 2023, 6, 80–88 CrossRef CAS.
- J. Yang, D. Wang, H. Han and C. Li, Acc. Chem. Res., 2013, 46, 1900–1909 CrossRef CAS PubMed.
- R. Yang, Y. Fan, Y. Zhang, L. Mei, R. Zhu, J. Qin, J. Hu, Z. Chen, Y. Hau Ng, D. Voiry, S. Li, Q. Lu, Q. Wang, J. C. Yu and Z. Zeng, Angew. Chem., Int. Ed., 2023, 62, e202218016 CrossRef CAS PubMed.
- J. Li, L. Zhu, C.-H. Tung and L.-Z. Wu, Angew. Chem., Int. Ed., 2023, 62, e202301384 CrossRef CAS PubMed.
- M. Z. Rahman, F. Raziq, H. Zhang and J. Gascon, Angew. Chem., Int. Ed., 2023, 62, e202305385 CrossRef CAS PubMed.
- Y. Shi, Z. Zhao, D. Yang, J. Tan, X. Xin, Y. Liu and Z. Jiang, Chem. Soc. Rev., 2023, 52, 6938–6956 RSC.
- X. Tao, Y. Zhao, S. Wang, C. Li and R. Li, Chem. Soc. Rev., 2022, 51, 3561–3608 RSC.
- W. Liu, C. He, S. Huang, K. Zhang, W. Zhu, L. Liu, Z. Zhang, E. Zhu, Y. Chen, C. Chen and Y. Zhu, Angew. Chem., Int. Ed., 2023, 62, e202304773 CrossRef CAS PubMed.
- S. Liu, Q. Chen, Y. Chen, P. Lin, H. Zhuzhang, M. Han, Z.-A. Lan, X. Chen, X. Wang, Q. Li and Z. Li, J. Mater. Chem. A, 2023, 11, 14682–14689 RSC.
- P. Das, J. Roeser and A. Thomas, Angew. Chem., Int. Ed., 2023, 62, e202304349 CrossRef CAS PubMed.
- P.-D. Liu, Y. Chen, A.-G. Liu, Z.-T. Chen and B. Li, J. Mater. Chem. A, 2023, 11, 25322–25331 RSC.
- X. Gong, F. Ma, Y. Zhang, Y. Li, Z. Wang, Y. Liu, P. Wang, H. Cheng, Y. Dai, Y. Fan, B. Huang and Z. Zheng, ACS Catal., 2023, 13, 12338–12349 CrossRef CAS.
- K. Zhang, L. Wang, Y. Hong, X. Duan, C. Ai, L. Zhang, T. Zhang, Y. Chen, X. Lin, W. Shi and F. Guo, J. Alloys Compd., 2023, 966, 171580 CrossRef CAS.
- M. K. T. Chee, B.-J. Ng, W.-K. Chong, L.-L. Tan, W. S. Chang and S.-P. Chai, ACS Appl. Energy Mater., 2023, 6, 7935–7943 CrossRef CAS.
- X. Li, G. Fang, T. Wu, Q. Tian, Q. Yang and Z. Chen, Appl. Surf. Sci., 2023, 635, 157717 CrossRef CAS.
- S. S. Zhao, J. Liang, D.-H. Si, M.-J. Mao, Y. B. Huang and R. Cao, Appl. Catal., B, 2023, 333, 122782 CrossRef CAS.
- Z. Liu, S. Yao, A. Zhang, Y. Li, Y. Fu and Q. Zhou, Appl. Catal., B, 2023, 338, 123023 CrossRef CAS.
- J.-Y. Tang, C.-C. Er, X. Y. Kong, B.-J. Ng, Y.-H. Chew, L.-L. Tan, A. R. Mohamed and S.-P. Chai, Chem. Eng. J., 2023, 466, 143287 CrossRef CAS.
- X. Bai, M. She, Y. Ji, Z. Zhang, W. Xue, E. Liu, K. Wan, P. Liu, S. Zhang and J. Li, Adv. Sci., 2023, 10, 2207250 CrossRef CAS PubMed.
- M. A. Hamza, S. A. Rizk, E.-E. M. Ezz-Elregal, S. A. A. El-Rahman, S. K. Ramadan and Z. M. Abou-Gamra, Sci. Rep., 2023, 13, 12929 CrossRef CAS PubMed.
- Z. Mou, Y. Dong, S. Li, Y. Du, X. Wang, P. Yang and S. Wang, Int. J. Hydrogen Energy, 2011, 36, 8885–8893 CrossRef CAS.
- Y.-Q. Xing and S.-Y. Liu, Chin. J. Struct. Chem., 2022, 41, 2209056–2209068 CAS.
- F. Jin, B. Yang, X. Wang, T. Li, N. Tsubaki and Z. Jin, Chin. J. Struct. Chem., 2023, 42, 100198 CrossRef CAS.
- J. Wang, Z. Wang, J. Zhang and K. Dai, Chin. J. Struct. Chem., 2023, 42, 100202 CrossRef CAS.
- W.-R. Zhuang, Y. Wang, P.-F. Cui, L. Xing, J. Lee, D. Kim, H.-L. Jiang and Y.-K. Oh, J. Controlled Release, 2019, 294, 311–326 CrossRef CAS PubMed.
- T. Chen, M. Li and J. Liu, Cryst. Growth Des., 2018, 18, 2765–2783 CrossRef CAS.
- E. M. Pérez and N. Martín, Chem. Soc. Rev., 2015, 44, 6425–6433 RSC.
- Y. Liu, H. Wu and Q. Wang, J. Mater. Chem. A, 2023, 11, 21470–21497 RSC.
- J. Ji, X. Wei, W. Wu and C. Yang, Acc. Chem. Res., 2023, 56, 1896–1907 CrossRef CAS PubMed.
- S. J. Olusegun, T. G. F. Souza, G. D. O. Souza, M. Osial, N. D. S. Mohallem, V. S. T. Ciminelli and P. Krysinski, J. Water Process Eng., 2023, 51, 103457 CrossRef.
- Y. Hao, Y.-L. Lu, Z. Jiao and C.-Y. Su, Angew. Chem., Int. Ed., 2024, 63, e202317808 CrossRef CAS PubMed.
- S. Hu, M.-L. Gao, J. Huang, H. Wang, Q. Wang, W. Yang, Z. Sun, X. Zheng and H.-L. Jiang, J. Am. Chem. Soc., 2024, 146, 20391–20400 CrossRef CAS PubMed.
- C. Janiak, J. Chem. Soc., Dalton Trans., 2000, 21, 3885–3896 RSC.
- R. Thakuria, N. K. Nath and B. K. Saha, Cryst. Growth Des., 2019, 19, 523–528 CrossRef CAS.
- T. F. Headen, C. A. Howard, N. T. Skipper, M. A. Wilkinson, D. T. Bowron and A. K. Soper, J. Am. Chem. Soc., 2010, 132, 5735–5742 CrossRef CAS PubMed.
- R. Zhao and R.-Q. Zhang, Phys. Chem. Chem. Phys., 2016, 18, 25452–25457 RSC.
- X. Xu, K. Jia, Q. Qi, G. Tian and D. Xiang, Phys. Chem. Chem. Phys., 2024, 26, 14607–14612 RSC.
- S. Samanta, S. Sanyal, M. Maity, M. Chaudhury and S. Ghosh, J. Lumin., 2017, 190, 403–412 CrossRef CAS.
- B. J. J. Timmer and T. J. Mooibroek, J. Chem. Educ., 2021, 98, 540–545 CrossRef CAS PubMed.
- T. Yang, Y. Chen, Y. Wang, X. Peng and A. Kong, ACS Appl. Mater. Interfaces, 2023, 15, 8066–8075 CrossRef CAS PubMed.
- J. Zhang, Y. Xu, L. Cui, A. Fu, W. Yang, C. Barrow and J. Liu, Composites, Part A, 2015, 71, 1–8 CrossRef.
- F. C.-M. Leung, S. Y.-L. Leung, C. Y.-S. Chung and V. W.-W. Yam, J. Am. Chem. Soc., 2016, 138, 2989–2992 CrossRef CAS PubMed.
- M.-M. Shi, Y. Chen, Y.-X. Nan, J. Ling, L.-J. Zuo, W.-M. Qiu, M. Wang and H.-Z. Chen, J. Phys. Chem. B, 2011, 115, 618–623 CrossRef CAS PubMed.
- Y. Shao, G.-Z. Yin, X. Ren, X. Zhang, J. Wang, K. Guo, X. Li, C. Wesdemiotis, W.-B. Zhang and S. Yang, RSC Adv., 2017, 7, 6530–6537 RSC.
- L. Chen, H. Wang, J. Liu, R. Xing, X. Yu and Y. Han, J. Polym. Sci., Part B:Polym. Phys., 2016, 54, 838–847 CrossRef CAS.
- S. Yao, X. Zhang, H. Wang, H. Wang, X. Gan, Z. Wu, Y. Tian, Z. Pan and H. Zhou, Dyes Pigm., 2017, 145, 152–159 CrossRef CAS.
- L. Liu, J. Hao, Y. Shi, J. Qiu and C. Hao, RSC Adv., 2015, 5, 3045–3053 RSC.
- Q. Yang, M. Luo, K. Liu, H. Cao and H. Yan, Appl. Catal., B, 2020, 276, 119174 CrossRef CAS.
- E. Asayesh-Ardakani, M. Rahmani, A. Hosseinian, S.-B. Ghaffari and M.-H. Sarrafzadeh, Coord. Chem. Rev., 2024, 518, 216087 CrossRef CAS.
- J. Tan, S. Namuangruk, W. Kong, N. Kungwan, J. Guo and C. Wang, Angew. Chem., Int. Ed., 2016, 55, 13979–13984 CrossRef CAS PubMed.
- T. Wang, Y. Zhang, Z. Wang, Y. Chen, P. Cheng and Z. Zhang, Dalton Trans., 2023, 52, 15178–15192 RSC.
- Y. Zhu, P. Shao, L. Hu, C. Sun, J. Li, X. Feng and B. Wang, J. Am. Chem. Soc., 2021, 143, 7897–7902 CrossRef CAS PubMed.
- X. Guan, Q. Fang, Y. Yan and S. Qiu, Acc. Chem. Res., 2022, 55, 1912–1927 CrossRef CAS PubMed.
- Z. Ting, G. Yi-fan and G. Jia, J. Funct. Polym., 2018, 31, 189–215 Search PubMed.
- C. Liu, Z. Wang, L. Zhang and Z. Dong, J. Am. Chem. Soc., 2022, 144, 18784–18789 CrossRef CAS PubMed.
- A. Zhang and Y. Ai, Prog. Chem., 2020, 32, 1564–1581 CAS.
- W. H. X. Yu and Y. Li, Acta Chim. Sin., 2022, 80, 1494–1506 CrossRef.
- F. Guo, W. Zhang, S. Yang, L. Wang and G. Yu, Small, 2023, 19, 2207876 CrossRef CAS PubMed.
- W. Liu, X. Li, P. He, B. Li, N. Liu, Y. Li and L. Ma, Small, 2024, 20, 2403684 CrossRef CAS PubMed.
- Y. Li, J. Sui, L.-S. Cui and H.-L. Jiang, J. Am. Chem. Soc., 2023, 145, 1359–1366 CrossRef CAS PubMed.
- M. Guo, S. Jayakumar, M. Luo, X. Kong, C. Li, H. Li, J. Chen and Q. Yang, Nat. Commun., 2022, 13, 1770 CrossRef CAS PubMed.
- S. Kandambeth, K. Dey and R. Banerjee, J. Am. Chem. Soc., 2019, 141, 1807–1822 CrossRef CAS PubMed.
- H. Yuan, Z. Lu, Y. Li, C. Zhang and G. Li, Chin. J. Chromatogr., 2022, 40, 109–122 CrossRef CAS PubMed.
- J. Li, S.-Y. Gao, J. Liu, S. Ye, Y. Feng, D.-H. Si and R. Cao, Adv. Funct. Mater., 2023, 33, 2305735 CrossRef CAS.
- T. Shi, L. Li, Z. Li, G. Chen, H. Wang, X. Zhang and Y. Xie, J. Phys. Chem. Lett., 2022, 13, 10364–10369 CrossRef CAS PubMed.
- S. Yu, H. Pang, S. Huang, H. Tang, S. Wang, M. Qiu, Z. Chen, H. Yang, G. Song, D. Fu, B. Hu and X. Wang, Sci. Total Environ, 2021, 800, 149662 CrossRef CAS PubMed.
- J. Li, Q. Lv, L. Bi, F. Fang, J. Hou, G. Di, J. Wei, X. Wu and X. Li, Coord. Chem. Rev., 2023, 493, 215303 CrossRef CAS.
- S. Sağlam, F. N. Türk and H. Arslanoğlu, J. Environ. Chem. Eng., 2023, 11, 110568 CrossRef.
- Y. Chai, Y. Zhang, L. Wang, Y. Du, B. Wang, N. Li, M. Chen and L. Ou, Sep. Purif. Technol., 2022, 295, 121225 CrossRef CAS.
- G. Xu, C. Zhu and G. Gao, Small, 2022, 18, 2203140 CrossRef CAS PubMed.
- S. A. A. Razavi, W. Chen, H.-C. Zhou and A. Morsali, Coord. Chem. Rev., 2024, 517, 216004 CrossRef CAS.
- P. Thanasekaran, C.-H. Su, Y.-H. Liu and K.-L. Lu, Coord. Chem. Rev., 2021, 442, 213987 CrossRef CAS.
- H. Dai, X. Yuan, L. Jiang, H. Wang, J. Zhang, J. Zhang and T. Xiong, Coord. Chem. Rev., 2021, 441, 213985 CrossRef CAS.
- V. Anh Tran, K. B. Vu, T.-T. Thi Vo, V. Thuan Le, H. H. Do, L. G. Bach and S.-W. Lee, Appl. Surf. Sci., 2021, 538, 148065 CrossRef CAS.
- Y. Wang, A. Liu, Y. Zheng, J. Song, H. Xian and T. Jiang, Surf. Interfaces, 2024, 46, 104069 CrossRef CAS.
- N. A. Nordin, M. A. Mohamed, N. S. N. Hasnan, S. F. M. Yusoff, M. S. Mastuli, T. Sugiura and K. Manseki, J. Sol-Gel Sci. Technol., 2024 DOI:10.1007/s10971-024-06331-x.
- Y. Sun, J. Wei, Z. Fu, M. Zhang, S. Zhao, G. Xu, C. Li, J. Zhang and T. Zhou, Adv. Mater., 2023, 35, 2208625 CrossRef CAS PubMed.
- Y. Sun, Y. Li, X. Song, S. Zhao, T. Zhou and J. Zhang, Adv. Funct. Mater., 2024, 2413107 Search PubMed.
- W.-H. Deng, X. Wang, K. Xiao, Y. Li, C. Liu, Z. Yao, L. Wang, Z. Cheng, Y. Lv, S. Xiang and Z. Zhang, Chem. Eng. J., 2024, 497, 154457 CrossRef CAS.
- C. Ma, L. Qin, T. Zhou and J. Zhang, Energy Environ. Sci., 2024, 17, 8992–9026 RSC.
- W.-K. Qin, C.-H. Tung and L.-Z. Wu, J. Mater. Chem. A, 2023, 11, 12521–12538 RSC.
- A. A. Zhang, Z. X. Wang, Z. B. Fang, J. L. Li and T. F. Liu, Angew. Chem., Int. Ed., 2024, 63, e202412777 CrossRef CAS PubMed.
- B. Yu, L. Li, S. Liu, H. Wang, H. Liu, C. Lin, C. Liu, H. Wu, W. Zhou, X. Li, T. Wang, B. Chen and J. Jiang, Angew. Chem., Int. Ed., 2021, 60, 8983–8989 CrossRef CAS PubMed.
- C. Huang, S. Barlow and S. R. Marder, J. Org. Chem., 2011, 76, 2386–2407 CrossRef CAS PubMed.
- R. Sun, Y. Wang, Z. Zhang, Y. Qu, Z. Li, B. Li, H. Wu, X. Hua, S. Zhang, F. Zhang and L. Jing, Chem. Eng. J., 2021, 426, 131266 CrossRef CAS.
- J. Lu, Z. Li, H. Gao and W. Cui, Appl. Surf. Sci., 2021, 537, 148003 CrossRef CAS.
- R. Sun, H. Yin, Z. Zhang, Y. Wang, T. Liang, S. Zhang and L. Jing, J. Phys. Chem. C, 2021, 125, 23830–23839 CrossRef CAS.
- X. Bai, L. Guo, T. Jia, D. Hao, C. Wang, H. Li and R. Zong, J. Hazard. Mater., 2022, 435, 128992 CrossRef CAS PubMed.
- P. Liu, T. Liang, J. Bian, Z. Li, Z. Zhang and L. Jing, Mater. Res. Bull., 2023, 167, 112370 CrossRef CAS.
- X. Zhang, L. Shi and Y. Zhang, J. Taiwan Inst. Chem. Eng., 2022, 132, 104111 CrossRef CAS.
- L. Yan, W. Wang, Q. Zhao, Z. Zhu, B. Liu and C. Hu, J. Colloid Interface Sci., 2022, 606, 898–911 CrossRef CAS PubMed.
- S. Dong, Y. Zhao, J. Yang, X. Liu, W. Li, L. Zhang, Y. Wu, J. Sun, J. Feng and Y. Zhu, Appl. Catal., B, 2021, 291, 120127 CrossRef CAS.
- X. Xu, S. Dai, S. Xu, Q. Zhu and Y. Li, Angew. Chem., Int. Ed., 2023, 62, e202309066 CrossRef CAS PubMed.
- R. Wang, J. Liu, B. Wang, R. Yang, S. Zhu, Y. Song, Y. Hua, J. Yan, M. Cheng, H. Xu and H. Li, J. Alloys Compd., 2022, 925, 166370 CrossRef CAS.
- R. Tang, D. Gong, Y. Deng, S. Xiong, J. Deng, L. Li, Z. Zhou, J. Zheng, L. Su and L. Yang, Chem. Eng. J., 2022, 427, 131809 CrossRef CAS.
- J. Yang, H. Miao, J. Jing, Y. Zhu and W. Choi, Appl. Catal., B, 2021, 281, 119547 CrossRef CAS.
- Y. Wang, Z. Zhao, R. Sun, J. Bian, Z. Zhang and L. Jing, Nanoscale, 2022, 14, 8041–8049 RSC.
- S. Dai, Y. Xu, W. Zhang, S. Li, Q.-Y. Guo, J. Cui, Y. Song, J. Yuan, W. Peng and M. Huang, J. Mater. Chem. A, 2022, 10, 20248–20253 RSC.
- R. Yang, G. Song, L. Wang, Z. Yang, J. Zhang, X. Zhang, S. Wang, L. Ding, N. Ren, A. Wang and X. Yu, Small, 2021, 17, 2102744 CrossRef CAS PubMed.
- A. Kumari and S. Sengupta, ChemCatChem, 2024, 16, e202301033 CrossRef CAS.
- F. Würthner, Chem. Commun., 2004, 1564–1579 RSC.
- H. Wu, L. Xue, Y. Shi, Y. Chen and X. Li, Langmuir, 2011, 27, 3074–3082 CrossRef CAS PubMed.
- J. Wang, D. Liu, Y. Zhu, S. Zhou and S. Guan, Appl. Catal., B, 2018, 231, 251–261 CrossRef CAS.
- K. Balakrishnan, A. Datar, R. Oitker, H. Chen, J. Zuo and L. Zang, J. Am. Chem. Soc., 2005, 127, 10496–10497 CrossRef CAS PubMed.
- H. Li, C. Wang, X. Bai, X. Wang, B. Sun, D. Li, L. Zhao, R. Zong and D. Hao, Mater. Chem. Front., 2020, 4, 2673–2687 RSC.
- K. Kong, S. Zhang, Y. Chu, Y. Hu, F. Yu, H. Ye, H. Ding and J. Hua, Chem. Commun., 2019, 55, 8090–8093 RSC.
- Z. Zhang, J. Wang, D. Liu, W. Luo, M. Zhang, W. Jiang and Y. Zhu, ACS Appl. Mater. Interfaces, 2016, 8, 30225–30231 CrossRef CAS PubMed.
- Y. Sun, D. Wang and Y. Zhu, Chem. Eng. J., 2022, 438, 135667 CrossRef CAS.
- G. Aimaiti, Y. Zou, Y. Ma, Y. Shi, K. Qi, W. Zhan, Z. Qian, Z. Liu and Y. Dong, Chem. Eng. J., 2024, 496, 153852 CrossRef CAS.
- Y. Liu, L. Ma, C. Shen, X. Wang, X. Zhou, Z. Zhao and A. Xu, Chin. J. Catal., 2019, 40, 168–176 CrossRef CAS.
- J. Xu, Q. Gao, Z. Wang and Y. Zhu, Appl. Catal., B, 2021, 291, 120059 CrossRef CAS.
- S. Bettini, L. Valli and G. Giancane, Molecules, 2020, 25, 3742 CrossRef CAS PubMed.
- D. M. Mafukidze and T. Nyokong, J. Mol. Struct., 2019, 1180, 307–317 CrossRef CAS.
- K. Shi, M. Zhou, F. Wang, X. Li, W. Huang, K. Lu, K. Yang and C. Yu, Chemosphere, 2023, 329, 138617 CrossRef CAS PubMed.
- M. Pei, K. Li, X. Li, C. Song and X. Guo, Ind. Eng. Chem. Res., 2023, 62, 2698–2709 CrossRef CAS.
- S. Xu, W. Sun, X. Meng, Y. Dong and Y. Ding, J. Phys. Chem. C, 2021, 125, 24413–24421 CrossRef CAS.
- T. Ikeda, J. M. Lintuluoto, N. Aratani, Z. S. Yoon, D. Kim and A. Osuka, Eur. J. Org. Chem., 2006, 3193–3204 CrossRef CAS.
- V. Valderrey, G. Aragay and P. Ballester, Coord. Chem. Rev., 2014, 258–259, 137–156 CrossRef CAS.
- K. Yamasumi and H. Maeda, Bull. Chem. Soc. Jpn., 2021, 94, 2252–2262 CrossRef CAS.
- S. Chen, X. Ren, S. Tian, J. Sun and F. Bai, MRS Adv., 2020, 5, 2147–2155 CrossRef CAS.
- J. Han, Z. Zhu, N. Li, D. Chen, Q. Xu, H. Li, J. He and J. Lu, Appl. Catal., B, 2021, 291, 120108 CrossRef CAS.
- B. Zhao, D. Lu, K. K. Kondamareddy, W. Gu, J. Li, T. Tian, L. Li, H. Fan and W. Ho, J. Alloys Compd., 2024, 984, 174004 CrossRef CAS.
- B. Das, L. P. R. Pala, M. K. Mohanta, M. Devi, D. Chakraborty, N. R. Peela, M. Qureshi and S. S. Dhar, J. Mater. Chem. A, 2022, 10, 23691–23703 RSC.
- D. Chen, K. Wang, W. Hong, R. Zong, W. Yao and Y. Zhu, Appl. Catal., B, 2015, 166–167, 366–373 CrossRef CAS.
- R. Bera, B. Jana, B. Mondal and A. Patra, ACS Sustainable Chem. Eng., 2017, 5, 3002–3010 CrossRef CAS.
- W. Li, X. He, R. Ge, M. Zhu, L. Feng and Y. Li, Sustainable Mater. Technol., 2019, 22, e00114 CrossRef CAS.
- S. Liu, S. Zhou, C. Hu, M. Duan, M. Song, F. Huang and J. Cai, J. Mater. Sci.: Mater. Electron., 2020, 31, 10677–10688 CrossRef CAS.
- T. Morimoto, C. Nishiura, M. Tanaka, J. Rohacova, Y. Nakagawa, Y. Funada, K. Koike, Y. Yamamoto, S. Shishido, T. Kojima, T. Saeki, T. Ozeki and O. Ishitani, J. Am. Chem. Soc., 2013, 135, 13266–13269 CrossRef CAS PubMed.
- Y.-J. Huang and J. Zhang, Inorg. Chim. Acta, 2020, 501, 119244 CrossRef CAS.
- T.-T. Zhu, Y.-T. Tao, Y. Sun, X. Wang, X.-W. Zhang, J.-L. Chai, J. Han, X.-L. Zhao and X.-D. Chen, J. Mol. Struct., 2021, 1236, 130289 CrossRef CAS.
- S. Adhikari, A. H. Sheikh, S. Kansız, N. Dege, N. Baildya, G. Mahmoudi, N. A. Choudhury, R. J. Butcher, W. Kaminsky, S. Talledo, E. M. Lopato, S. Bernhard and J. Kłak, J. Mol. Struct., 2023, 1285, 135481 CrossRef CAS.
- X.-Y. Zhang, R.-X. Wu, C.-F. Bi, X. Zhang and Y.-H. Fan, Inorg. Chim. Acta, 2018, 483, 129–135 CrossRef CAS.
- X.-L. Hao, Y.-Y. Ma, Y.-H. Wang, W.-Z. Zhou and Y.-G. Li, Inorg. Chem. Commun., 2014, 41, 19–24 CrossRef CAS.
- Z. Xu, X. Mao, P. Zhang, H. Li, Y. Wang, M. Liu and L. Jia, J. Mol. Struct., 2017, 1128, 665–673 CrossRef CAS.
- J.-H. Zhang, Y.-N. Gong, H.-J. Wang, Y.-C. Wang, W. Yang, J.-H. Mei, D.-C. Zhong and T.-B. Lu, Proc. Natl. Acad. Sci. U. S. A., 2022, 119, e2118278119 CrossRef CAS PubMed.
- L.-Q. Qiu, K.-H. Chen, Z.-W. Yang and L.-N. He, Green Chem., 2020, 22, 8614–8622 RSC.
- J. Li, Y. Wei, X.-Y. Chen, Z.-L. Zhu and Y. Gao, Inorg. Chem. Commun., 2017, 86, 10–13 CrossRef CAS.
- S. Scoditti, E. Dabbish, G. E. Pieslinger, E. Rezabal, X. Lopez, E. Sicilia and L. Salassa, Phys. Chem. Chem. Phys., 2022, 24, 5323–5329 RSC.
- S. Tang, Z. Fu, Y. Li and Y. Li, Appl. Catal., A, 2020, 590, 117342 CrossRef CAS.
- M. Kombo, H.-B. Chong, L.-B. Ma, S. Sahar, X.-X. Fang, T. Zhao, C. Ling, X.-J. Lu and A.-W. Xu, ACS Appl. Nano Mater., 2020, 3, 10659–10667 CrossRef CAS.
- Y. Zhang, Q. Cao, X. Wu, Y. Xiao, A. Meng, Q. Zhang, Y. Yu and W.-D. Zhang, Chem. Eng. J., 2022, 427, 132042 CrossRef CAS.
- J. Wei, D. Luo, M. Shi, Q. Yuan, M. Wang, Y. Huang and Y. Ni, Inorg. Chem., 2023, 62, 10973–10983 CrossRef CAS PubMed.
- S. Cao, J. Low, J. Yu and M. Jaroniec, Adv. Mater., 2015, 27, 2150–2176 CrossRef CAS PubMed.
- X.-L. Song, L. Chen, J.-T. Ren, L.-J. Gao and Z.-Y. Yuan, Coord. Chem. Rev., 2024, 507, 215752 CrossRef CAS.
- M. Chen, M. Sun, X. Cao, H. Wang, L. Xia, W. Jiang, M. Huang, L. He, X. Zhao and Y. Zhou, Coord. Chem. Rev., 2024, 510, 215849 CrossRef CAS.
- H. Li, B. Cheng, J. Xu, J. Yu and S. Cao, EES Catal., 2024, 2, 411–447 RSC.
- H. Song, L. Luo, S. Wang, G. Zhang and B. Jiang, Chin. Chem. Lett., 2024, 35, 109347 CrossRef CAS.
- H. Che, C. Liu, H. Dong, C. Li, X. Liu and G. Che, Int. J. Hydrogen Energy, 2019, 44, 20029–20041 CrossRef CAS.
- W. Wang, Y. Huang and Z. Wang, Acta Phys. Chim. Sin., 2020, 2011073 Search PubMed.
- J. Huang, M. Klahn, X. Tian, X. Dai, J. Rabeah, V. Aladin, B. Corzilius, S. Bartling, H. Lund, N. Steinfeldt, T. Peppel, A. J. Logsdail, H. Jiao and J. Strunk, ACS Appl. Nano Mater., 2024, 7, 7442–7452 CrossRef CAS.
- F. Fina, S. K. Callear, G. M. Carins and J. T. S. Irvine, Chem. Mater., 2015, 27, 2612–2618 CrossRef CAS.
- X. Cai, Y. Li, Y. Zhang and W. Lin, ACS Catal., 2023, 13, 15877–15885 CrossRef CAS.
- M. Majdoub, Z. Anfar and A. Amedlous, ACS Nano, 2020, 14, 12390–12469 CrossRef CAS PubMed.
- T. Banerjee, F. Podjaski, J. Kröger, B. P. Biswal and B. V. Lotsch, Nat. Rev. Mater., 2021, 6, 168–190 CrossRef CAS.
- S. Wang, H. Zhang, R. Nie, Y. Ning, C. Zhao, Z. Xia, P. Niu, L. Li and S. Wang, Dalton Trans., 2022, 51, 13015–13021 RSC.
- J. Liu, J. Li, F. Xing and J. Liu, Catal. Sci. Technol., 2024, 14, 903–911 RSC.
- F. Xu, C. Lai, M. Zhang, D. Ma, L. Li, S. Liu, X. Zhou, H. Yan, N. Wang, M. Xu, L. Qin and H. Yi, Sep. Purif. Technol., 2023, 308, 122829 CrossRef CAS.
- L. Liu, L. Ding, Y. Liu, W. An, S. Lin, Y. Liang and W. Cui, Appl. Catal., B, 2017, 201, 92–104 CrossRef CAS.
- X. Wang, J. Zhu, X. Yu, X. Fu, Y. Zhu and Y. Zhang, J. Mater. Sci. Technol., 2021, 77, 19–27 CrossRef CAS.
- Y. Qing, Y. Li, L. Cao, Y. Yang, L. Han, P. Dansawad, H. Gao and W. Li, Sep. Purif. Technol., 2023, 314, 123545 CrossRef CAS.
- S. Yan, H. Song, Y. Li, J. Yang, X. Jia, S. Wang and X. Yang, Appl. Catal., B, 2022, 301, 120820 CrossRef CAS.
- N. A. Chopan and A. H. Bhat, New J. Chem., 2023, 47, 15922–15941 RSC.
- Y. Yu, Q. Yang, X. Yu, Q. Lu and X. Hong, ChemistrySelect, 2017, 2, 5578–5586 CrossRef CAS.
- S. Wang, C. T. Nai, X.-F. Jiang, Y. Pan, C.-H. Tan, M. Nesladek, Q.-H. Xu and K. P. Loh, J. Phys. Chem. Lett., 2012, 3, 2332–2336 CrossRef CAS PubMed.
- Y. Liang, X. Wang, W. An, Y. Li, J. Hu and W. Cui, Appl. Surf. Sci., 2019, 466, 666–672 CrossRef CAS.
- L. Ding, H. Zhou, S. Li, X. Lan, X. Chen and S. Zeng, Colloids Surf., A, 2022, 648, 129296 CrossRef CAS.
- X. Yuan, N. Sunyer-Pons, A. Terrado, J. L. León, G. Hadziioannou, E. Cloutet and K. Villa, ChemSusChem, 2023, 16, e202202228 CrossRef CAS PubMed.
- C. Lu, J. Han, N. Li, D. Chen, Q. Xu, H. Li and J. Lu, J. Colloid Interface Sci., 2024, 668, 59–67 CrossRef CAS PubMed.
- H. Yang, H. Hou, Y. Jiang, Z. Zhang, L. Wang, R. Wang, X. Zhan, Y. Luo and W. Yang, Opt. Mater., 2024, 149, 115015 CrossRef CAS.
- L. Xu, B. Tian, T. Wang, Y. Yu, Y. Wu, J. Cui, Z. Cao, J. Wu, W. Zhang, Q. Zhang, J. Liu, Z. Li and Y. Tian, Energy Environ. Sci., 2022, 15, 5059–5068 RSC.
- X. Zhu, M. Guan, R. Gong, X. Gong, C. Dai and J. Tang, Sustainable Energy Fuels, 2023, 7, 1537–1543 RSC.
- Q. Zhu, J. Wang, L. Zhang, D. Yan, H. Yang, L. Zhang and X. Miao, Bull. Mater. Sci., 2023, 46, 17 CrossRef CAS.
- K. Thakur and B. Kandasubramanian, J. Chem. Eng. Data, 2019, 64, 833–867 CrossRef CAS.
- V. Georgakilas, J. N. Tiwari, K. C. Kemp, J. A. Perman, A. B. Bourlinos, K. S. Kim and R. Zboril, Chem. Rev., 2016, 116, 5464–5519 CrossRef CAS PubMed.
- G. Sheng, Y. Shao, W. Ye, C. Sun, C. Chen, J. C. Crittenden and C. Liu, ACS Sustainable Chem. Eng., 2018, 6, 6711–6717 CrossRef CAS.
- Q. Wang, H. Li, J.-H. Yang, Q. Sun, Q. Li and J. Yang, Appl. Catal., B, 2016, 192, 182–192 CrossRef CAS.
- F. Li, M. Tang, T. Li, L. Zhang and C. Hu, Appl. Catal., B, 2020, 268, 118397 CrossRef CAS.
- Q. Hao, S. Hao, X. Niu, X. Li, D. Chen and H. Ding, Chin. J. Catal., 2017, 38, 278–286 CrossRef CAS.
- S. Gonuguntla, C. S. Vennapoosa, B. M. Abraham, A. V. S. Sainath and U. Pal, ACS Appl. Nano Mater., 2024, 7, 18146–18156 CrossRef CAS.
- P. Karthik, R. Vinoth, P. Zhang, W. Choi, E. Balaraman and B. Neppolian, ACS Appl. Energy Mater., 2018, 1, 1913–1923 CrossRef CAS.
- M. P. Croxall, R. T. Lawrence, R. Ghosh Biswas, R. Soong, A. J. Simpson and M. C. Goh, J. Phys. Chem. Lett., 2024, 15, 3653–3657 CrossRef CAS PubMed.
- Z. Luo, D. Zhang, C. Ma, M. Zhu, B. Li, L. Song and S. Yang, ACS Omega, 2023, 8, 4072–4080 CrossRef CAS PubMed.
- T. Li, M. Wang, Q. Hou, Y. Hou, K. Xuan and Y. Hao, Appl. Surf. Sci., 2022, 605, 154672 CrossRef CAS.
- A. Jilani, S. Z. Hussain, M. O. Ansari, R. Kumar, M. R. Dustgeer, M. H. D. Othman, M. A. Barakat and A. A. Melaibari, J. Mater. Sci., 2021, 56, 7434–7450 CrossRef CAS.
- S. J. Park, S. Kim, T. F. Anjong, S. E. Lee and J. Kim, Carbon, 2015, 94, 448–454 CrossRef CAS.
- G. Jenita Rani, M. A. Jothi Rajan and G. Gnana Kumar, Res. Chem. Intermed., 2017, 43, 2669–2690 CrossRef CAS.
- Z. Zhu, L. Pan, Z. Liu, J. Zhao, Z. Tao and Y. He, RSC Adv., 2021, 11, 33408–33415 RSC.
- W. Zhou, H. Shen, Y. Zeng, Y. Yi, Z. Zuo, Y. Li and Y. Li, Angew. Chem., Int. Ed., 2020, 59, 4908–4913 CrossRef CAS PubMed.
- H.-Y. Si, C.-J. Mao, J.-Y. Zhou, X.-F. Rong, Q.-X. Deng, S.-L. Chen, J.-J. Zhao, X.-G. Sun, Y. M. Shen, W.-J. Feng, P. Gao and J. Zhang, Carbon, 2018, 132, 598–605 CrossRef CAS.
- H. Si, Q. Deng, C. Yin, J. Zhou, S. Zhang, Y. Zhang, Z. Liu, J. Zhang, J. Zhang and J. Kong, J. Alloys Compd., 2020, 833, 155054 CrossRef CAS.
- C. M. Navarathna, N. B. Dewage, A. G. Karunanayake, E. L. Farmer, F. Perez, E. B. Hassan, T. E. Mlsna and C. U. Pittman, J. Inorg. Organomet. Polym. Mater., 2020, 30, 214–229 CrossRef CAS.
- C. X. Yang, Q. Zhu, W. P. Dong, Y. Q. Fan and W. L. Wang, Langmuir, 2021, 37, 9253–9263 CrossRef CAS PubMed.
- F. Guo, C. Shi, W. Sun, Y. Liu, X. Lin and W. Shi, Chin. J. Chem. Eng., 2022, 48, 1–11 CrossRef CAS.
- N. Cheng, B. Wang, M. Chen, Q. Feng, X. Zhang, S. Wang, R. Zhao and T. Jiang, Environ. Pollut., 2023, 336, 122409 CrossRef CAS PubMed.
- Y. Lan, Y. Luo, S. Yu, H. Ye, Y. Zhang, M. Xue, Q. Sun, Z. Yin, X. Li, C. Xie, Z. Hong and B. Gao, Sep. Purif. Technol., 2024, 330, 125543 CrossRef CAS.
- L. Meng, W. Yin, S. Wang, X. Wu, J. Hou, W. Yin, K. Feng, Y. S. Ok and X. Wang, Chemosphere, 2020, 239, 124713 CrossRef CAS PubMed.
- R. Tang, D. Gong, Y. Deng, S. Xiong, J. Zheng, L. Li, Z. Zhou, L. Su and J. Zhao, J. Hazard. Mater., 2022, 423, 126944 CrossRef CAS PubMed.
- J. Yang, L. Wang, J. Yang, C. Li and S. Zhong, J. Environ. Chem. Eng., 2024, 12, 111768 CrossRef CAS.
- X. Ji, X. Liu, Y. Guo and J. Zhang, Chem. Eng. J., 2021, 425, 131260 CrossRef CAS.
- C.-C. Li, I. Ullah, G. Wang and A.-W. Xu, Catal. Sci. Technol., 2023, 13, 5456–5461 RSC.
- J. Wang, H. Li, Y. Zhu, M. Yang, J. Huang, X. Zhu, Z. P. Yu, Z. Lu and H. Zhou, Chem. Sci., 2023, 14, 323–330 RSC.
- X. Peng, J. Ma, Z. Zhou, H. Yang, J. Chen, R. Chen, K. Wu, G. Xi, S. Liu, Y. Shen and Y. Zhang, Chem. Sci., 2023, 14, 4319–4327 RSC.
- X. J. Zhao, Y. Li, M. Li, Y. L. Tian, W. P. Wang, B. S. Zhang and X. C. Wang, Org. Biomol. Chem., 2022, 20, 5139–5144 RSC.
- J.-W. Wang, H.-H. Huang, P. Wang, G. Yang, S. Kupfer, Y. Huang, Z. Li, Z. Ke and G. Ouyang, JACS Au, 2022, 2, 1359–1374 CrossRef CAS PubMed.
- X.-D. Su, B.-B. Zhang, Q. Liu, J.-T. Cheng, Z.-X. Wang and X.-Y. Chen, Org. Lett., 2021, 23, 8262–8266 CrossRef CAS PubMed.
- L.-B. Ma, K. Liang, G. Wang, X.-X. Fang, C. Ling, T. Zhao, M. Kombo, T.-Y. Cheang and A.-W. Xu, Catal. Sci. Technol., 2019, 9, 5441–5446 RSC.
- B. Chandra Garain and S. K. Pati, ChemPhysChem, 2023, 24, e202200753 CrossRef CAS PubMed.
- V. J. K. Lourdusamy, M. R. N. Thomas and S. Subramani, Environ. Sci. Pollut. Res., 2023, 30, 18113–18122 CrossRef CAS PubMed.
- S. Saita, M. Anzai, N. Mori and H. Kawasaki, Colloids Surf., A, 2021, 617, 126360 CrossRef CAS.
- G. Son, J. Kim and C. B. Park, ACS Appl. Energy Mater., 2020, 3, 1215–1221 CrossRef CAS.
- W. Shahzad, A. K. Badawi, Z. A. Rehan, A. M. Khan, R. A. Khan, F. Shah, S. Ali and B. Ismail, Ceram. Int., 2022, 48, 24979–24988 CrossRef CAS.
- W. Yao, M. Li, C. Sun, Y. Liu, M. Ma, F. Chen, L. Zhou and Y. Zheng, J. Clean. Prod., 2022, 372, 133745 CrossRef CAS.
- M. Tang, L. Qin, M. Luo, H. Shen, S.-Z. Kang, T. Zhang and X. Li, J. Environ. Chem. Eng., 2022, 10, 108040 CrossRef CAS.
- K. Karuppasamy, I. Rabani, D. Vikraman, C. Bathula, J. Theerthagiri, R. Bose, C.-J. Yim, A. Kathalingam, Y.-S. Seo and H.-S. Kim, Environ. Pollut., 2021, 272, 116018 CrossRef CAS PubMed.
- L. Yan, H. Gao and Y. Chen, ACS Appl. Nano Mater., 2021, 4, 7746–7757 CrossRef CAS.
- D. Mohanta and M. Ahmaruzzaman, J. Alloys Compd., 2020, 828, 154093 CrossRef CAS.
- V. A. Tran, A. N. Kadam and S.-W. Lee, J. Alloys Compd., 2020, 835, 155414 CrossRef CAS.
- G. Wang, H. Li, N. Li, D. Chen, J. He, Q. Xu and J. Lu, Angew. Chem., Int. Ed., 2022, 61, e202210619 CrossRef CAS PubMed.
- Q. Zhang, J. Chen, X. Gao, H. Che, P. Wang, B. Liu and Y. Ao, Sep. Purif. Technol., 2022, 300, 121947 CrossRef CAS.
- F. Li, T. Li, L. Zhang, Y. Jin and C. Hu, Appl. Catal., B, 2021, 296, 120316 CrossRef CAS.
- Y. Shen, C. Zhu, B. Chen, J. Chen, Q. Fang, J. Wang, Z. He and S. Song, Environ. Sci.: Nano, 2020, 7, 1525–1538 RSC.
- A. Saadati and S. Sheibani, Ceram. Int., 2022, 48, 30294–30306 CrossRef CAS.
- S. Qin, Y. Xiong, J. Li, H. Wan, S. Fang, M. Duan, R. Li and D. Liao, J. Phys. Chem. C, 2021, 125, 4027–4040 CrossRef CAS.
- H. Wang, X. Yuan, Y. Wu, G. Zeng, H. Dong, X. Chen, L. Leng, Z. Wu and L. Peng, Appl. Catal., B, 2016, 186, 19–29 CrossRef CAS.
- L. Yue, S. Wang, G. Shan, W. Wu, L. Qiang and L. Zhu, Appl. Catal., B, 2015, 176–177, 11–19 CrossRef CAS.
- K. L. V. Joseph, J. Lim, A. Anthonysamy, H.-I. Kim, W. Choi and J. K. Kim, J. Mater. Chem. A, 2015, 3, 232–239 RSC.
- Y. Gao, G. Yu, K. Liu, S. Deng, B. Wang, J. Huang and Y. Wang, Chem. Eng. J., 2017, 330, 157–165 CrossRef CAS.
- C. Du, W. Feng, S. Nie, X. Su, H. Liu, J. Feng, J. Sun, C. Hu and S. Dong, Appl. Catal., B, 2022, 310, 121298 CrossRef CAS.
- Q. Zhang, J. Chen, X. Gao, H. Che, Y. Ao and P. Wang, J. Hazard. Mater., 2022, 430, 128386 CrossRef CAS PubMed.
- H. Liu, H. Li, J. Lu, S. Zeng, M. Wang, N. Luo, S. Xu and F. Wang, ACS Catal., 2018, 8, 4761–4771 CrossRef CAS.
- L. Wang, X. Liu, L. Ji, Q. Luo, Y. Duan, J. An, X. Chen, Y. Zhang, J. Ren and D. Wang, Chem. Eng. J., 2022, 433, 134502 CrossRef CAS.
- Y. Wei, M. Ma, W. Li, J. Yang, H. Miao, Z. Zhang and Y. Zhu, Appl. Catal., B, 2018, 238, 302–308 CrossRef CAS.
- H. Yang, C. Li, T. Liu, T. Fellowes, S. Y. Chong, L. Catalano, M. Bahri, W. Zhang, Y. Xu, L. Liu, W. Zhao, A. M. Gardner, R. Clowes, N. D. Browning, X. Li, A. J. Cowan and A. I. Cooper, Nat. Nanotechnol., 2023, 18, 307–315 CrossRef CAS PubMed.
- Y. Shiraishi, K. Miura, M. Jio, S. Tanaka, S. Ichikawa and T. Hirai, ACS Mater. Au, 2022, 2, 709–718 CrossRef CAS PubMed.
- W. Yu, N. Fang, R. Wang, Z. Liu, Y. Chu and C. Huang, Adv. Funct. Mater., 2024, 34, 2314894 CrossRef CAS.
- W. Liu, R. Xu, Y. Wang, N. Huang, T. Shimada and L. Ye, Int. J. Hydrogen Energy, 2022, 47, 16005–16013 CrossRef CAS.
- T. Sun, S. Li, L. Zhang and Y. Xu, Angew. Chem., Int. Ed., 2023, 62, e202301865 CrossRef CAS PubMed.
- W. Wei and Y. Zhu, Small, 2019, 15, 1903933 CrossRef CAS PubMed.
- J. Yang, H. Miao, W. Li, H. Li and Y. Zhu, J. Mater. Chem. A, 2019, 7, 6482–6490 RSC.
- D. Liu, X. Yang, P. Chen, X. Zhang, G. Chen, Q. Guo, H. Hou and Y. Li, Adv. Mater., 2023, 35, 2300655 CrossRef CAS PubMed.
- X. Miao, F. Zhang, Y. Wang, X. Dong and X. Lang, Sustainable Energy Fuels, 2023, 7, 1963–1973 RSC.
- C. Yan, J. Dong, Y. Chen, W. Zhou, Y. Peng, Y. Zhang and L.-N. Wang, Nano Res., 2022, 15, 3835–3858 CrossRef CAS.
- T. Xue, C. Ma, L. Liu, C. Xiao, S.-F. Ni and R. Zeng, Nat. Commun., 2024, 15, 1455 CrossRef CAS PubMed.
- Y. Zhang, W. Zhang, F. Zhang, Y. Xiao, G. Jia, W. Wang, F.-S. Ke and Z. Guo, Angew. Chem., Int. Ed., 2024, 63, e202402694 CrossRef CAS PubMed.
- Z. Zhang, W. Liu, Y. Zhang, J. Bai and J. Liu, ACS Catal., 2021, 11, 313–322 CrossRef CAS.
- Y. Zhang, X. Huang, J. Li, G. Lin, W. Liu, Z. Chen and J. Liu, Chem. Res. Chin. Univ., 2020, 36, 1076–1082 CrossRef CAS.
- M. Wang, Z. Wang, M. Shan, J. Wang, Z. Qiu, J. Song and Z. Li, Chem. Mater., 2023, 35, 5368–5377 CrossRef CAS.
- S. An, Z. Wu, H. Jeong, J. Lee, S. Y. Jeong, W. Lee, S. Kim, J. W. Han, J. Lim, H. Cha, H. Y. Woo and D. S. Chung, Small, 2023, 19, 2204905 CrossRef CAS PubMed.
- T. Wang, M. Li, Y. Chen, X. Che, F. Bi, Y. Yang, R. Yang and C. Li, ACS Catal., 2023, 13, 15439–15447 CrossRef CAS.
- B. Wang, X. Guo, Y. Zhang, Y. Wang, G. Huang, H. Chao, W. Wang, Z. Hu and X. Yan, ACS Appl. Energy Mater., 2022, 5, 7479–7489 CrossRef CAS.
- X. Han, F. Zhao, Q. Shang, J. Zhao, X. Zhong and J. Zhang, ChemSusChem, 2022, 15, e202200828 CrossRef CAS PubMed.
- Z. Chen, B. Chong, N. Wells, G. Yang and L. Wang, Chin. Chem. Lett., 2022, 33, 2579–2584 CrossRef CAS.
- X. Liu, Z. Wang, S. Feng, X. Zhang, H. Xu, G. Wei, X. Gong, X. Wu and J. Hua, Macromolecules, 2023, 56, 8275–8289 CrossRef CAS.
- B. Rabeie and N. M. Mahmoodi, J. Colloid Interface Sci., 2024, 654, 495–522 CrossRef CAS PubMed.
- C. Sun, Z. Wang, H. Zheng, S. Zhao, X. Luo, C. Li, L. Chen and F. Li, Sep. Purif. Technol., 2024, 331, 125586 CrossRef CAS.
- X. Wang, X. Li, G. Che, E. Zhu, H. Guo, P. A. Charpentier, W. Z. Xu and C. Liu, ACS Appl. Mater. Interfaces, 2024, 16, 6367–6381 CrossRef CAS PubMed.
- J. Jin, Z. Liu, W. Wang, X. Cai, Y. Zhang, M. Muddassir, H. Sakiyama, J. Wang and C. Xie, J. Mol. Struct., 2022, 1250, 131867 CrossRef CAS.
- J. Han, Y. Deng, N. Li, D. Chen, Q. Xu, H. Li, J. He and J. Lu, J. Colloid Interface Sci., 2021, 582, 1021–1032 CrossRef CAS PubMed.
- X. Li, Q. Luo, L. Han, F. Deng, Y. Yang and F. Dong, J. Mater. Sci. Technol., 2022, 114, 222–232 CrossRef CAS.
- L. Ning, J. Xu, Y. Lou, C. Pan, Z. Wang and Y. Zhu, J. Mater. Sci. Technol., 2022, 124, 53–64 CrossRef CAS.
- M. Tian, Y. He and G. Zhang, J. Nanopart. Res., 2021, 23, 223 CrossRef CAS.
- Y. Wang, X. Li, W. Xu, D. Chen, N. Li, Q. Xu, H. Li and J. Lu, Appl. Catal., B, 2024, 348, 123839 CrossRef CAS.
- X. Song, J. He, Y. Wang, J. Wang and S. Zhang, J. Colloid Interface Sci., 2023, 645, 918–932 CrossRef CAS PubMed.
- Q. Zhang, J. Chen, X. Gao, H. Che, P. Wang and Y. Ao, Appl. Catal., B, 2022, 313, 121443 CrossRef CAS.
- Z. Khazaee, Y. Ouyang, Y. Zhang, O. Bogojevic, T. Jess Plesner and Z. Guo, Chem. – Eur. J., 2024, 30, e202303615 CrossRef CAS PubMed.
- L. Yang, J. Wang, K. Zhao, Z. Fang, H. Qiao, L. Zhai and L. Mi, ChemPlusChem, 2022, 87, e202200281 CrossRef CAS PubMed.
- J. Jiao, T. Zhang, J. Xu, K. Guo, J. Li and Q. Han, Chem. Commun., 2023, 59, 3114–3117 RSC.
- T. Zhang, J. Vanderghinste, A. Guidetti, S. V. Doorslaer, G. Barcaro, S. Monti and S. Das, Angew. Chem., Int. Ed., 2022, 61, e202212083 CrossRef CAS PubMed.
- N. Sun, D. Qi, Y. Jin, H. Wang, C. Wang, C. Qu, J. Liu, Y. Jin, W. Zhang and J. Jiang, CCS Chem., 2022, 4, 2588–2596 CrossRef CAS.
- L. Zhao, W. Cai, G. Ji, J. Wei, Z. Du, C. He and C. Duan, Inorg. Chem., 2022, 61, 9493–9503 CrossRef CAS PubMed.
- J.-L. Shi, K. Feng, H. Hao, C. Ku, P. H. L. Sit, W. Y. Teoh and X. Lang, Sol. RRL, 2022, 6, 2100608 CrossRef CAS.
- Y.-Y. Lin and S.-Y. Lu, J. Taiwan Inst. Chem. Eng., 2019, 97, 264–271 CrossRef CAS.
- J. Cheng, Y. Wu, W. Zhang, J. Zhang, L. Wang, M. Zhou, F. Fan, X. Wu and H. Xu, Adv. Mater., 2024, 36, 2305313 CrossRef CAS PubMed.
- Y. Liu and Z. Xiang, ACS Appl. Mater. Interfaces, 2019, 11, 41313–41320 CrossRef CAS PubMed.
- X. Yuan, K. Yang, C. Grazon, C. Wang, L. Vallan, J. D. Isasa, P. M. Resende, F. Li, C. Brochon, H. Remita, G. Hadziioannou, E. Cloutet and J. Li, Angew. Chem., Int. Ed., 2024, 63, e202315333 CrossRef CAS PubMed.
- B. Xia, N. Qiu, L. Cheng, A. Shan, H. Ma, J. Yang and J. Liu, Chem. Eng. Sci., 2024, 283, 119427 CrossRef CAS.
- S. Lee, J. Ju, C. Keum, J. Bang, H. Lee, S. Vikneshvaran, H. Yoo, J. Park and S.-Y. Lee, ChemSusChem, 2024, 17, e202301044 CrossRef CAS PubMed.
- T. Song, P. Zhang, T. Wang, A. Ali and H. Zeng, Appl. Catal., B, 2018, 224, 877–885 CrossRef CAS.
- C. Shu, X. Yang, L. Liu, X. Hu, R. Sun, X. Yang, A. I. Cooper, B. Tan and X. Wang, Angew. Chem., Int. Ed., 2024, 136, e202403926 CrossRef.
- T. Shan, Y. Wang, D. Luo, Z. Huang, F. Zhang, H. Wu, L. Huang, J. Li, L. Chen and H. Xiao, Appl. Catal., B, 2024, 349, 123872 CrossRef CAS.
- Y. Guo, X. Miao, X. Zhang, H. Che and Y. Li, Sep. Purif. Technol., 2023, 326, 124807 CrossRef CAS.
- H.-D. Yang, J.-H. Huang, K. Shibata, D. Lu, K. Maeda and C. Hu, Sustainable Energy Fuels, 2020, 4, 4186–4195 RSC.
- Y. Liu, Y. Wang, J. Shang, J. Peng and T. Zhu, Appl. Catal., B, 2024, 350, 123937 CrossRef CAS.
- S. Zhang, Y. Hou, L. Zhang, H. Zhu, J. Xiong, S. Wang and T. Liu, Small, 2024, 20, 2311816 CrossRef CAS PubMed.
- Y. Li, L. Wang, X. Gao, Y. Xue, B. Li and X. Zhu, J. Mater. Chem. A, 2024, 12, 7807–7816 RSC.
- J. Song, S. Wang, L. Wang, Q. Luo, J. An, Y. Duan and D. Wang, Appl. Catal., B, 2024, 343, 123582 CrossRef CAS.
- D. Sun, J. Han, M. Xiao, T. Cao, Y. Li and H. Dong, Sep. Purif. Technol., 2024, 338, 126610 CrossRef CAS.
- P. Zhang, J. Jia, Y. Wang, L. Tong, J. Wang, C. Li and H. Dong, Sep. Purif. Technol., 2024, 330, 125224 CrossRef CAS.
- J.-W. Wang, Z. Li, Z.-M. Luo, Y. Huang, F. Ma, S. Kupfer and G. Ouyang, Proc. Natl. Acad. Sci. U. S. A., 2023, 120, e2221219120 CrossRef CAS PubMed.
- Z. Wu, W. Li, L. Hou, Q. Wei, H. Yang, Y. Jiang and D. Tang, Sep. Purif. Technol., 2023, 311, 123322 CrossRef CAS.
- S. Wang, X. Hai, X. Ding, S. Jin, Y. Xiang, P. Wang, B. Jiang, F. Ichihara, M. Oshikiri, X. Meng, Y. Li, W. Matsuda, J. Ma, S. Seki, X. Wang, H. Huang, Y. Wada, H. Chen and J. Ye, Nat. Commun., 2020, 11, 1149 CrossRef PubMed.
- Z. Liu, Y. Xie, L. Liu, X. Cai, H.-Q. Yin, M. Zuo, Y. Liu, S. Feng, W. Huang and D. Wu, Small, 2024, 20, 2309194 CrossRef CAS PubMed.
- X. Song, Y. Wu, X. Zhang, X. Li, Z. Zhu, C. Ma, Y. Yan, P. Huo and G. Yang, Chem. Eng. J., 2021, 408, 127292 CrossRef CAS.
- Y. Li, J. Shi, L. Wu, J. Zhang and J. Lu, Eur. J. Inorg. Chem., 2021, 620–627 CrossRef.
|
This journal is © The Royal Society of Chemistry 2025 |
Click here to see how this site uses Cookies. View our privacy policy here.