DOI:
10.1039/D4YA00025K
(Review Article)
Energy Adv., 2024,
3, 741-764
Recent trends on the application of phytochemical-based compounds as additives in the fabrication of perovskite solar cells
Received
13th January 2024
, Accepted 23rd February 2024
First published on 11th March 2024
Abstract
Perovskite solar cells (PSCs) are promising photovoltaic systems that hold the capacity to bring about a paradigm shift within the field of renewable energy. They possess exceptional photophysical properties, and their advancement is accelerating because of multidimensional research initiatives. Nevertheless, PSCs are faced with the challenges of non-radiative recombination power losses and rapid degradation in performance. These problems are mainly engendered by defects that form during the perovskite crystallization. Among the many ways to address these issues are compositional engineering, architectural modification, control of deposition parameters, solvent engineering, doping, use of additives and interface engineering. Incorporating additives into the perovskite layer is an easy and effective approach to enhance both their photophysical characteristics and resistance to degradation. As PSC additives, natural products, including phytochemicals, are gaining popularity due to their low cost and environmental friendliness. This review provides a survey on the use of natural products as additives in PSCs with particular emphasis on phytochemicals. It also highlights the roles of phytochemical-based additives in modulating the optoelectronic and degradation resistance properties of perovskite films through control of the crystallization dynamics, defect passivation and structural stabilization. Focus has been given to the functional groups in these phytochemical-based additives and how they interact with the perovskite components through hydrogen-bonding, coordination with uncoordinated ionic/atomic species and scavenging of free radicals. Overall, the use of phytochemical-based additives could enhance the performance and stability of PSCs and accelerate their commercialization.
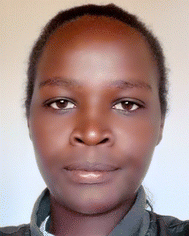
Naomy Chepngetich
| Ms Naomy Chepngetich is an MSc student at the Department of Mathematics, Physics and Computing of Moi University in Kenya. She holds a BEd (Sci) degree in physics and mathematics from University of Eldoret, Kenya. Her research interest is in the development of sustainable photovoltaic systems as renewable energy sources. She is a recipient of the World Bank Group Scholarship through the African Centre of Excellence (ACE II)–Phytochemicals, Textile & Renewable Energy (PTRE)-Moi University. Naomy's long-term goal is to mentor more young girls to become physicists in future. |
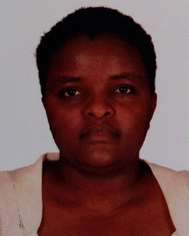
Gloria M. Mumbi
| Ms Gloria M. Mule is an MSc student at the Department of Mathematics, Physics and Computing of Moi University in Kenya. She holds a BSc degree in physics and her research interest is in computational physics and its applications in renewable energy technologies. She is currently studying the effects of additives on the optoelectronic properties of mixed halide perovskites for solar cell applications. Gloria's long-term goal is to contribute to the development of affordable renewable energy systems. |
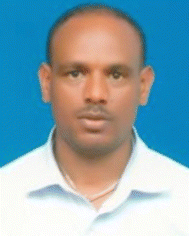
Getnet Meheretu M.
| Mr Getnet Meheretu is an assistant professor and a PhD student in Sustainable Energy Engineering at Bahir Dar University in Ethiopia. He holds an MSc in Physics and Photonics. His research interests include photonics and photovoltaic materials. He is currently working on the effects of additives on the performance of perovskite solar cells. |
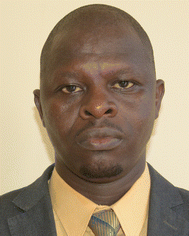
Koech K. Richard
| Dr Richard Koech holds a PhD degree in material science and engineering from African University of Science and Technology (AUST)-Abuja, Nigeria, and an MSc degree in Physics (Renewable energy) from Moi University, Kenya. He was a visiting researcher at Higgin's laboratory in Worcester Polytechnic Institute (WPI) (2019–2021). He is a lecturer in the department of mathematics, physics and computing of Moi University, Kenya (2014-date). His research interest is on materials for applications in solar cells, light emitting diodes and energy (LEDs) storage devices. Dr Koech's current focus is on the role of additives in the optoelectronic properties and performance of perovskite solar cells and LEDs. |
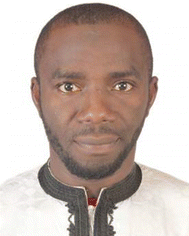
Dahiru Sanni
| Dr Dahiru Sanni holds a PhD and MSc degree in Theoretical and Applied Physics from the African University of Science and Technology (AUST) Abuja, Nigeria. He also holds a Bachelor of technology degree in Physics/Electronics from the Federal University of Technology Minna (FUTMINNA) Niger State, Nigeria. After his bachelor's degree, he worked as an Assistant Lecturer in the Department of Physics Federal College of Education (FCE) Yola, Nigeria (2009–2010) and Physics Teacher at Great Heights Academy Abuja, Nigeria (2011). Dr Sanni Joined the Federal University Dutsinma (FUDMA) as a Lecturer in the Department of Physics in 2014 and Lecturer with the Nile University of Nigeria (NUN) 2018. He was a visiting scholar at Macro Technology Works in the Arizona State University (ASU) Tempe, AZ, USA (2017) and also Fellow of The French National Centre for Scientific Research (Centre national de la recherche scientifique, CNRS, Orleans, France) (2019–2020). Dahiru was a participant of the Solar Decthlon Africa, Morocco 2019. His research interests are in the areas of Materials Physics, Solar cells and Energy storage. |
1. Introduction
Global energy statistics continue to report a consistent increase in energy demand, usually driven by factors such as rapid industrialization, increasing human population, rising standards of living, and advancement in information and communication technology.1 In order to meet the rising energy demand in a sustainable manner, a shift from conventional fossil-fuel-based energy resources to abundant renewable energy resources has been emphasized.2–6 The utilization of renewable and low-carbon energy resources is expected to reduce dependence on fossil fuels, mitigate climate change, ensure energy security, cushion nations against possible price fluctuations caused by disruptions of energy supplies, and guarantee sustainability.7
Solar energy is one of the most abundant renewable energy resources that can be harnessed to provide much-needed electrical energy through photovoltaic (PV) technology. PV technology is a promising alternative to fossil fuels owing to its rapid pace of development and reduction in manufacturing costs.8 Recent advancements in material science and nanotechnology, as well as numerous research initiatives aimed at enhancing solar energy conversion efficiencies and lowering production costs, have all contributed to the remarkable progress in PV technology.9 In line with these initiatives, different types of materials have been used as active layers (ALs) in solar cells. These include crystalline and amorphous silicon, cadmium telluride, copper indium diselenide, gallium arsenide, organic dyes, and perovskite materials.9–12 Emerging PV technologies such as dye-sensitized solar cells (DSSCs), organic photovoltaics (OPVs), and perovskite solar cells (PSCs), which are relatively cheap, are being developed and are likely to be in the forefront in addressing the global energy challenges.13
2. Perovskite solar cells
Perovskite solar cell (PSC) is a technology that has gained preference among the emerging PV technologies due to the remarkable increase in their power conversion efficiency (PCE) within a short period of time (3.8% in 2009 to the current achievable efficiency of 25.8%).14 They utilize a material with a perovskite crystal structure (ABX3) as their AL. In the structure, A is a large monovalent cation usually methyl ammonium (MA) CH3NH3+, formamidinium (FA), NH2CH
NH2+, and cesium (Cs+), B is a divalent cation (Pb2+ or Sn2+), and X is a halide anion (I−, Br−, Cl−).14–16 The ionic radii of the various materials control the Goldschmidt's tolerance factor (t) and the octahedral factor (μ), which determine whether a particular mixture of materials can form a perovskite crystal structure or not.16 The ABX3 crystal structure of a hybrid organic–inorganic perovskite is shown in Fig. 1. Perovskite materials, made of organic and inorganic compounds, are good light absorbers and transform sunlight effectively into electrical energy.17,18 They exhibit excellent properties such as controllable band gaps, small Urbach energies, low defect densities, long charge carrier diffusion lengths, high dielectric constants, and low exciton binding energies.19,20
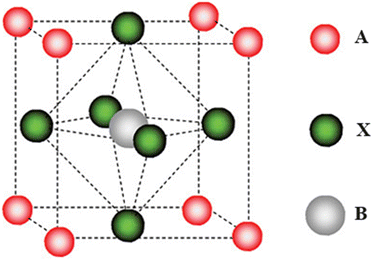 |
| Fig. 1 Crystal structure of a perovskite; reproduced from ref. 15 with permission from American Chemical Society, Copyright [2009]. | |
2.1 Structure, properties, and working principle of PSCs
A perovskite layer, acting as the AL in PSCs, is usually sandwiched between two charge transport layers (CTLs) with electrodes on either side.18 In this arrangement, the AL absorbs the incident sunlight and generates charge carriers, which are separated and transported via the CTLs to their corresponding electrodes.18 This PV technology has become a game changer in PV research on account of several factors. First, their effectiveness in converting sunlight into electricity is quite remarkable and is expected to outperform the crystalline silicon-based technology, which has long been in the PV market. Also, because their optoelectronic properties can be changed, they can be made to absorb a wider range of solar radiation. This makes them the best candidates for next-generation photovoltaics.21 Furthermore, unlike the energy-intensive crystalline silicon-based PV technologies, PSCs can be fabricated easily using low-temperature solution-based processes that can easily be scaled up.22 This intrinsic cost efficiency might make solar energy more inexpensive and available to more consumers. PSCs’ ability to be fabricated on flexible substrates is another notable feature that makes them very useful for applications in flexible, semi-transparent solar panels, integrated construction materials, and lightweight portable gadgets.23 PSCs can be designed with different device architectures, namely the mesoscopic (mesoporous) structure and planar structures. In the planar configuration, the intrinsic absorber layer is sandwiched between a compact electron transport material (ETM) and the hole transport material (HTM). In the mesoscopic structure, a mesoporous material is deposited on the ETM before the deposition of the AL. These structures can further be categorized into an n–i–p (regular) structure, where light is incident from the ETM side, or an p–i–n (inverted) structure, where light is incident from the HTM side.24,25 The different PSC device architectures are illustrated in Fig. 2.
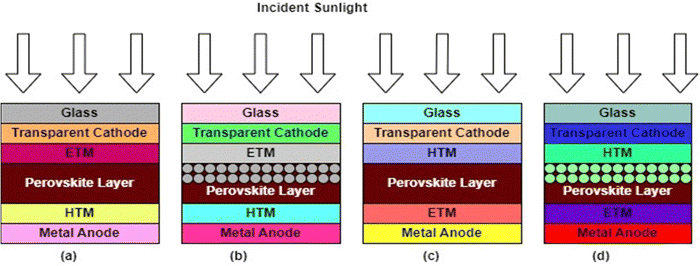 |
| Fig. 2 PSC device architectures: (a) n–i–p planar, (b) n–i–p mesoscopic, (c) p–i–n planar, and (d) p–i–n mesoscopic. | |
Therefore, a PSC is a device with a multi-layered structure, made via a step-by-step process that starts with substrate and precursor preparation, and ends with the deposition of the last electrode, as summarized in Fig. 3. The structure and material properties of the various layers that make up the device have a significant impact on the charge carrier dynamics, which influences the performance of PSCs.26–30 They control the values of the series resistance (Rs), the shunt resistance (Rsh) and the interfacial band offsets, which are linked to the non-radiative power losses that greatly affect the values of the saturation current density (Jsc), open circuit voltage (Voc), fill factor (FF), and power conversion efficiency (PCE) of the PSC device.31,32Rs, Rsh, and the interfacial band offsets are related to the bulk and surface properties of the thin film, which are determined by the film homogeneity, surface coverage, surface roughness, the presence of defects, and the surface work function.33,34 Improving the film quality of the different layers and reducing the interfacial energy mismatch is therefore the best strategy to achieve higher PCEs in PSCs.
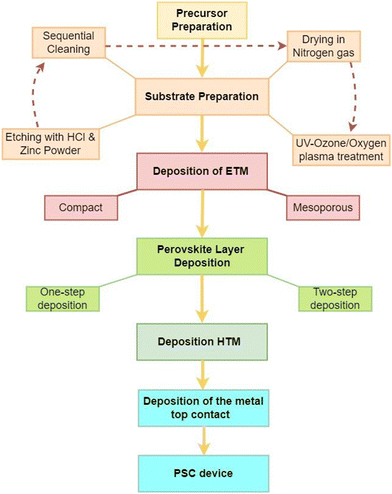 |
| Fig. 3 A simplified procedure for the fabrication of n–i–p planar PSCs. | |
Various strategies, which include compositional engineering, solvent engineering, architectural control, controlling the deposition conditions, doping, use of additives, and interface engineering, have been explored to improve the crystal quality and morphology of the films of the different components of PSCs.27,35–39 With all these strategies, good progress has been achieved with regards to the PCE of PSCs, though the defect-mediated recombination losses limit it below what is theoretically achievable.40,41 Despite this remarkable progress, stability remains the main bottleneck to the commercialization of PSC technology. The performance of such devices decreases with time under exposure to environmental and measuring conditions.42,43 The decrease in performance is caused by degradation in PSC, which has been attributed to intrinsic and extrinsic factors like lattice shrinkage, phase segregation, and morphological deformation in perovskite films due to hydration, oxidation, elemental diffusion, ion migration, exposure to UV, mechanical stress, and heat.44–47 The hysteresis phenomenon is another common problem in PSCs where there is a mismatch in the current density–voltage (J–V) curves under different bias conditions, scan direction, and scan rates.48,49 It is mainly attributed to ion migration, ferroelectricity, unbalanced charge transport, and defects induced through the electronic traps.50,51
Most of these challenges in PSCs can be controlled through the proper design of the different device components to reduce the defects in the bulk and the interfaces between them.52,53 The design of a perovskite AL with good bulk and surface properties is very important in improving the overall photophysical properties and structural integrity of PSCs. It plays a critical role in improving the quality of the interface with the CTLs and is therefore important in reducing the non-radiative recombination power losses and J–V hysteresis, and improving the stability of PSCs. Research efforts geared towards decelerating the crystallization dynamics in the perovskite AL with a view to fabricating quality films with increased grain sizes, reduced grain boundaries, and improved surface coverage have been carried out.50 These include compositional engineering, antisolvent treatment, solvent engineering, post-treatment, and incorporation of additives to the perovskite precursors.54–56 In recent years, more research has been done on adding additives to the photoactive perovskite layer because they play a bigger role in improving the quality of the film and the movement of charge carriers, even for large-area PSC devices. This strategy is therefore very important as efforts to scale up the fabrication process of PSCs intensify.
2.2 Role of additives in PSCs
Additives are chemicals that are often mixed into perovskite precursor solutions to help the film crystallize well. They do not always help make the perovskite crystal structure but play a significant role in the film formation process. The incorporation of additives in perovskite film serves the following purposes: first, the additives slow down the crystallization rate while the perovskite film is being grown. This improves the quality and shape of the crystals, which in turn improves their optoelectronic properties and durability.35,55,57,58 They can also improve the structural characteristics of perovskite films through strong chemical interactions and coordination among different cations and anions in the film, thus enhancing the PCE, stability, and reproducibility of PSCs.59,60 Secondly, it can improve the phase stability of the perovskite material by the suppressing phase transition at room temperature, which is one of the major causes of instability in PSCs.61,62 Thirdly, additives help to passivate the defects on the surface and bulk of the perovskite film through proper coordination and bonding with uncoordinated ionic species (e.g. Pb2+), which not only reduces the defect densities but also reduces ion migration. The functional groups present in the additives help in the formation of Lewis adducts with the perovskite and act as molecular cross-linkers at grain boundaries, leading to the formation of perovskite films with reduced defect densities and longer charge carrier lifetimes.63–65 They can also inhibit the formation of some impurities, such as metallic lead (Pb0), that are detrimental to the performance of PSCs during crystallization of the perovskite.66,67 Fourthly, additives can act as dopants, thereby modulating the interfacial energetics of PSCs by altering the work function of the perovskite film.68 This helps in reducing the energy mismatch at the interface with the CTLs, thus improving charge carrier extraction. Finally, some additives are hydrophobic and may have antioxidant properties. This will significantly increase the perovskite film's resistance to oxidation, heat, and water damage.69 Additionally, the steric effects of additives can make the film resistant to degradation by water and oxygen and also stop phase redistribution as the film ages.70,71
Some important substances that have been used as additives to change the properties of perovskite film are metal salts, nanoparticles, polymers, fullerenes and their derivatives, organic halides, inorganic salts, metal organic frameworks, and some solvents.59,63,72,73 The additives can be introduced into the perovskite films by mixing with the precursor solutions as anti-solvents or via interdiffusion. These additives have different roles in modulating the material properties of the specific component of the PSC depending on their chemical structures, the functional groups they contain, and their chemical interactions with the precursors.74 When they are incorporated into the perovskite film in the right proportions, they can improve the optoelectronic properties by altering the defect state and defect density, surface chemistry, charge carrier mobility, and light absorption capacity of the film in a positive way.75–77Fig. 4(a) and (b) show the different categories of additives, how they are usually incorporated into the perovskite film, and the material properties of the film that they can modify.
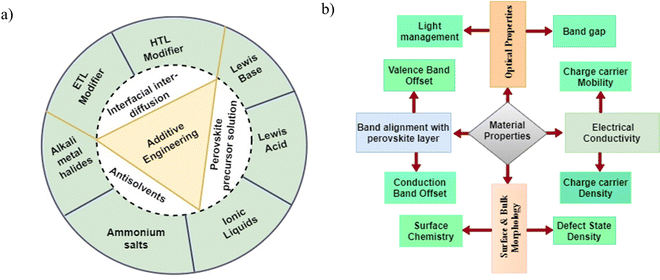 |
| Fig. 4 (a) Categories of additives,59,63 and (b) targeted material properties of the perovskite film. | |
In order to achieve a good performance, the resulting material properties of the constituent layers should ensure efficient incident light absorption, enhanced charge carrier dynamics, and improved resistance to degradation.26,78,79 This can be achieved through enhancement in film morphology and crystallinity, better interfacial band alignment with the CTLs, and reduction in interfacial defect density, as well as increased water repellency and increased resistance to oxidation, heat, and UV-induced degradation.80 As a consequence, the PV parameters of the resulting PSC device will be improved, and their values will be maintained for a longer period. Fig. 5 shows the comparison of the J–V and internal power conversion efficiency (IPCE) curves for PSCs with and without poly(9,9′-bis(3′-N,N-dimethylamino)-propyl)-2,7-fluorene-alt-2,7-9,9-dioctylfluorene (PFN-P1), where it is seen that the PSC with the PFN-P1 additive displays better charge carrier collection efficiency and better performance in terms of higher open-circuit voltage, Voc and short circuit current density (Jsc) relative to their counterparts without the additive.59
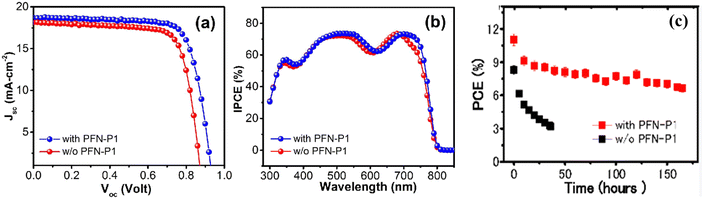 |
| Fig. 5 (a) J–V curves and (b) IPCE curves and (c) variation in PCE under continuous illumination for PSCs with and without PFN-P1 additive reproduced from ref. 81 with permission from American Chemical Society, Copyright (2016). | |
Different additives can modulate the properties of perovskite films and the J–V characteristics of PSCs to different degrees, even if they are incorporated in equal proportions. For instance, Wang et al. used methylammonium thiocyanate (MASCN), methylammonium acetate (MAAc), and methylammonium chloride (MACl), which are all volatile methyl ammonium salts, to change how the perovskite film crystallized.50 They observed that the different methylamine salt additives modulated the crystal growth in perovskite films by different degrees, with perovskite films modified with MACl additives yielding quality films and PSC devices with better PCE, improved stability, and reduced hysteresis. Wang et al.77 also made a PSC that worked better by adding a small molecular additive called hydrobromide additive (α-amino-γ-butyrolactone, ABL) to the perovskite film. The functional groups (–NH2 and C
O) and hydrophobic nature of the ABL additive respectively terminated the Pb2+ dangling bond and reduced moisture ingress in the film, leading to a reduction in non-radiative recombination power losses and moisture-induced degradation in the resulting PSC device.74,82 As such, additive engineering has therefore played a central role in the efforts to synergistically improve the PCE, stability, and reproducibility of PSCs.83 A comprehensive review on additive engineering and its important role in PSCs has been given by Li et al.,59 Zhang & Zhu,84 and Mahapatra et al.,85 and recently updated by Pereyra et al.74
Recent trends in the use of additives in PSCs have shifted towards the use of natural products owing to their eco-friendliness, biocompatibility, availability, and low cost when compared to their synthetic counterparts.86 These products have multiple functional groups that can coordinate with uncoordinated ions in perovskite films, form strong bonding interactions with atoms in the perovskite material, suppress crystal imperfections and passivate defects, and help in scavenging free radicals in the perovskite, thus improving its optoelectronic properties and degradation resistance.64,87 They therefore play a great role in morphological control, acting as cross-linkers at grain boundaries and modulating interfacial band alignment, thus improving charge carrier transport and collection in PSCs.
3. Natural products as additives in PSCs
Natural products have unique and diverse structural, chemical, and biological properties that are of great interest in the biomedical, industrial, and energy fields.88–90 In the field of energy, some natural products have found applications in photonics and photovoltaics.91,92 They include amino acids, fatty acids, vitamins, carbohydrates, keratin, cellulose, and phenolic compounds, among others.93–96 They can be added directly to precursor solutions to make thin films for optoelectronic devices, or they can be used indirectly as raw materials to make specific nanostructured materials.97
Amino acids are natural products that form the basic building blocks of proteins. Carboxyl (–COOH) and amino (–NH2) groups are the main functional groups in these compounds. They can strongly interact with the non-coordinated ions in the perovskite film thus eliminating possible defect sources. Though different amino acid classes can have the same functional groups, they can produce different impacts on the properties of their PSCs due to differences in their steric effects that influence their coordination capabilities.98 Hu et al.99 carried out a comparative study to understand the effects of four amino acid molecules as additives (proline, glutamic acid, arginine, and glycine) on the properties and performance of blade-coated PSCs.98,99 They found that the arginine additive coordinated strongly with Pb2+ through its guanidine group, producing better passivation effects on the perovskite film, suppression of non-radiative recombination power losses, improvement in the PCE, and enhanced moisture stability in the modified PSC device. The chemical structures of the different groups of amino acids and the morphology of the different perovskite films are shown in Fig. 6. These structures have an impact on the shape and crystallinity of the perovskite films as well as the performance of the whole PSC device. Amino acids derived from plants, such as L-Theanine (Thea), have also been shown to be effective in improving the PCE and stability of PSCs through the promotion of nucleation and crystallization of the perovskite film, inhibition of metallic lead (Pb0) and passivation of Pb2+ ions. The functional (–C
O and –NH2) groups present in Thea effectively reduced the non-radiative recombination of charge carriers with a consequent improvement in PSC device PCE from 22.29% to 24.58%.66
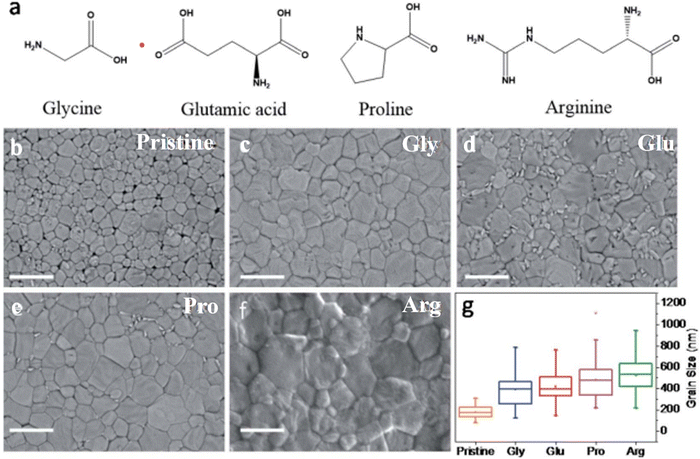 |
| Fig. 6 (a) Chemical structures of the different amino acids and SEM images of (b) pristine and (c) glycine-, (d) glutamine-, (e) proline- and (f) argentine-modified perovskite films; (g) grain sizes of the pristine and modified perovskite films. Reproduced from ref. 99 with permission from Royal Society of Chemistry, Copyright (2021). | |
Vitamins represent another class of natural products, with some of their members attracting applications in PSCs due to their interesting properties. For example, ascorbic acid is a water-soluble vitamin with good antioxidant properties, which is mainly found in fruits and vegetables.100 Apart from their significant role in human life, they are also seen to enhance the performance and stability of PSCs. It has been shown that adding ascorbic acids to the perovskite films reduces oxidation, thus improving their stability.101 Uric acid, a natural antioxidant, has also been used as an additive in tin (Sn)-based PSCs, yielding useful results in terms of improving the PCE and stability.102 A summary of the use of natural biomaterials and natural molecules as additives in perovskites, their interaction mechanisms with the perovskite material, and their effects on the performance of PSCs has been done by Chen and his team.103 The area of natural products is broad, as it encompasses animal-derived and plant-derived products (phytochemicals). This review narrows the focus to the application of phytochemical-based natural products as additives in PSCs. By limiting the scope, a proper and in-depth analysis will be performed on the use of phytochemicals, making it easier for the reader to understand the roles of these compounds and to observe the trends in their application in PSCs.
3.1 Phytochemicals as additives in PSCs
Phytochemical-based additives are becoming more popular over the synthetic ones because they are more sustainable, environmentally friendly, and readily available. The existing literature reports, which are in the form of reviews, focus mainly on the use of synthetic additives in engineering the properties of PSCs.59 However, literature reviews on the use of phytochemicals to guide further research in the fabrication of PSCs are yet to be reported. Therefore, a summary of the recent research articles on the use of phytochemicals as additives in PSCs is the focus of this review. Phytochemicals are compounds obtained from plant extracts as natural products.104 They are classified into several broad categories or classes, which include flavonoids, carotenoids, phenols or polyphenols, glycosides, tannins, saponins, lipids, fatty acids, alkaloids, and terpenoids; based on their basic structural features, biosynthetic pathways, or biological functions.105 A summary of the major classes of phytochemicals and their percentage composition in plant extracts is given in Fig. 7.106
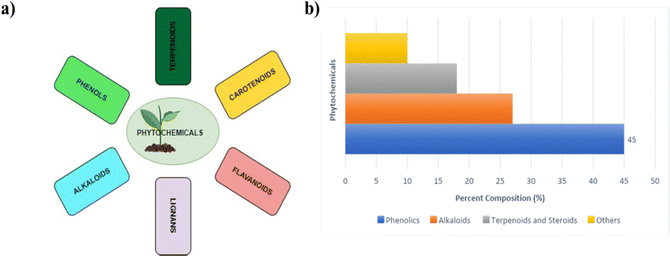 |
| Fig. 7 (a) Major classes of phytochemicals; (b) histogram with estimates of the percent composition of the major classes of phytochemicals. | |
Phytochemicals have widely been studied for their biological properties for biomedical applications. They are endowed with different functional groups, and some of them exhibit scavenging properties on free radical species such as reactive oxygen species (ROS) and reactive nitrogen species (RNS).107 Quercetin, for instance, is well-known as an effective antioxidant against ROS and can have excellent stability under ultraviolet (UV) light exposure.107 These properties can improve the stability in PSCs under exposure to oxygen and UV radiation. In addition, some of these phytochemicals have displayed interesting photophysical properties upon interaction with light photons, an indication that they can be useful in the field of energy.108 Suwa et al.109 used a small quantity of two commercial antioxidant phenolic compounds, 2,6-di-tert-butyl-4-cresol (BHT) and pentaerythritol tetrakis [3-(3,5-di-tert-butyl-4-hydroxyphenyl)propionate] (PTP), to make a mixed-cation lead halide PSC for photovoltaic applications. They observed that the presence of these compounds in the perovskite film reduced degradation by virtue of their ROS-scavenging capabilities. Furthermore, the interaction between O atoms in the phenolic compounds and Pb2+ in lead halide perovskite films helps in the formation of films with large grains, fewer grain boundaries, and a low density of defect states.110
Phytochemicals such as chlorophyll, anthocyanins, and their combinations have found application as light sensitizers in DSSCs due to their good photophysical properties and ability to improve light absorption in the visible region of the solar spectrum.111–115 This led to appreciable improvement in PCE and stability of these devices. Their applications as additives in the fabrication of PSCs is a recent development that has been found to boost the PCE and stability in PSCs.109,116,117 This review explores the different categories of phytochemicals that have been utilized as additives in the fabrication of PSCs. Emphasis will be placed on the chemical structures of the phytochemicals, their interaction with the ionic or atomic species in perovskite materials and how they impact on the optoelectronic properties, degradation resistance and overall performance of the fabricated PSCs.
3.1.1 Carotenes and carotenoids.
These are phytochemicals that exhibit antioxidant properties and are responsible for the yellow, orange, red, or purple coloration of plants. They possess various functional groups such as hydrocarbons (C–H), carbonyls (–C
O), and hydroxyls (–OH) among others, which can play important roles in engineering the properties of PSCs.118 These compounds include lycopene, bixin, crocin, cucurmin, zeaxanthin, and fucoxanthin among others.119 They have mainly been used as light sensitizers in DSSCs.119 As of now, it is only lycopene which has found recent application as an additive in the fabrication of PSCs. The C
C bonds form the main functional groups in lycopene that interact with the components of perovskite to yield useful results. The incorporation of lycopene into the perovskite film was found to help in crystal formation, reduction in trap density state, and passivation of defects at the surface, hence improving the PCE and durability of the PSCs.117
3.1.2 Phenolic compounds.
Another group of phytochemicals is the phenolic compounds, which have one or more phenyl rings and –OH that serves as a functional group.120–122 Depending on their chemical structures, these compounds can be subdivided into several classes, which are summarized in Fig. 8.
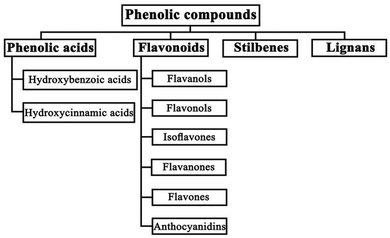 |
| Fig. 8 Classification of phenolic compounds. Reproduced from ref. 120 with permission from Ebrahimi & Lante, Copyright (2021). | |
Phenolic acids exist in the form of hydroxybenzoic acid (HBA) or hydroxycinnamic acid (HCA). They include gallic acid, cinnamic acid, ferulic acid, vanillic acid, caffeic acid, and sinapic acid among others.121,123 Flavonoids, stilbenes, and lignans, such as catechin, anthocyanidin, hydroquinone, resveratrol, piceatannol, and lignins, are the other types of phenolic compounds.121,123 The presence of an –OH functional group in their structure gives phenolic compounds the ability to scavenge free radicals and block UV light, making them good for reducing degradation and defects in PSCs.123
The application of phenolic compounds as additives in the fabrication of PSCs has been reported to improve the crystal quality as well as the antioxidant and water repellent properties of the perovskite film, with an overall improvement in PCE and stability.109,110 Wang et al. reported an improvement in the PCE and stability of tin-based PSCs by using gallic acid as a co-additive during its fabrication.124 Usually, the addition of Sn2+ to the thermodynamically stable Sn4+ leads to stability issues in Sn-based PSCs. This causes additional trap states and Sn2+ vacancies in the perovskite film, which makes the PSC work less well. Through FTIR spectroscopy and XRD analysis, the carboxyl (–COOH) group of the gallic acid was shown to coordinate with the uncoordinated Sn2+ ions in the perovskite film, thereby improving its crystal quality and reducing the defect densities. The presence of gallic acid also reduced oxidation-induced degradation due to its oxygen scavenging capability. As a result, there were fewer charge carrier trapping states, and the PCE of the PSC that contained gallic acid remained stable for a longer time. Fig. 9(a) and (b) illustrates the respective free radical scavenging mechanisms and the coordinative effects of gallic acid on Sn2+ in the film, which led to the operational stability of the PSC devices. Liu et al.125 added caffeic acid to the perovskite precursor solution, which improved the shape of the perovskite film by blocking defects at the grain boundaries and the surface of the film in a manner similar to that of gallic acid (Fig. 9(c) and (d)). This was supported by the observed reduction in the root mean square roughness (ΔRMS) of the film by about 6.3 nm and a reduction in defect density as seen in Fig. 9(e) and (f). This significant improvement in defect passivation was associated with the hydrogen bonding and the coordination interactions between the –COOH group and the uncoordinated Pb2+, Sn2+ ions or Sn4+ vacancies in the perovskite film. The overall improvement in the stability of the caffeic acid-modified PSC (ΔPCE = 1.59%) was attributed to the caffeic acid's ability to scavenge oxygen radical species through its phenolic group.
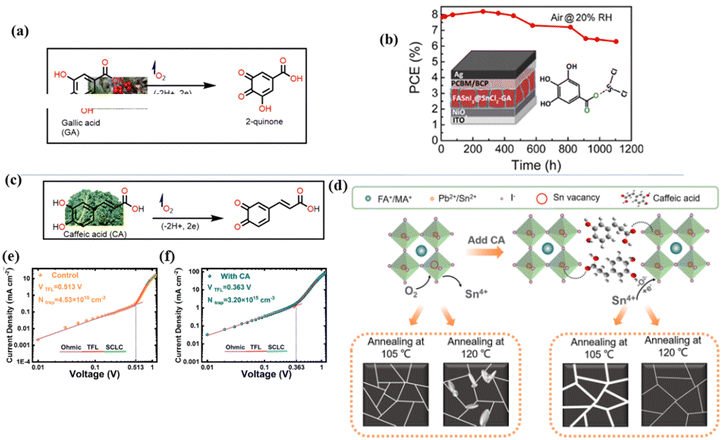 |
| Fig. 9 (a) Structure of gallic acid and its mode of oxygen radical scavenging; (b) the coordinative interaction between gallate and SnCl2 reprinted with permission from ref. 124, Copyright©2020, American Chemical Society, (c) structure of caffeic acid and its mode of oxygen radical scavenging; (d) coordination of caffeic acid with Sn4+ vacancies in the perovskite film; (e) dark I–V curve of the control device; and (f) dark I–V for the caffeic acid-modified perovskite film; (c)–(f), reproduced from ref. 125 with permission American Chemical Society, Copyright (2021). | |
Ferulic acid has also been shown to be effective in suppressing light-induced halide segregation in perovskite films because of its ability to bring about strain relaxation. Liu and co-workers126 incorporated trans-ferulic acid into a wide band gap PSC and reported a great improvement in performance. They attributed the improvement to trans-ferulic acid's ability to increase the perovskite crystal sizes and to stabilize the perovskite lattice structure. In one of the most recent studies, Zhang et al.127 utilized flavonoids (quercetin, morin, kaempferol, and myricetin) to stabilize Sn-based PSCs. They reported remarkable improvement in the performance of the PSC in the order of myricetin (ΔPCE = 0.84% w.r.t. the reference pristine) > quercetin (ΔPCE = 0.65%) > morin (ΔPCE = 0.50%). However, the kaempferol additive decreased the PCE of PSC (ΔPCE = −1.02%). The multifunctional groups in myricetin favored interactions with the perovskite film, and myricetin was found to anchor on the grain boundaries and the surface of the perovskite film. Hydrogen bonding interactions with the –NH groups of the perovskite film promoted crystallization kinetics, which led to improved crystal quality. Moreover, its multiple phenolic groups boosted the free radical-scavenging properties of myricetin, which hindered Sn2+ ion oxidation. Under the same experimental conditions, the other flavonoids quercetin, morin, and kaempferol performed less well than myricetin and, in order, correlated to the number of –OH groups in the phenyl ring C attached to the pyran structure. The myricetin-modified PSCs exhibited negligible hysteresis index (HI) relative to the pristine device. The use of myricetin also led to improved hole mobility (3.77 × 10−3 cm−2 V−1 s−1versus 1.19 × 10−3 cm−2 V−1 s−1 for the pristine device) and a reduction in defect density (0.396 × 1016 cm−2versus 3.16 × 1016 cm−2 for the pristine device), which indicates its excellent surface passivation properties. Taken together, these factors led to the improved performance and enhanced stability of the myricetin-modified PSC. Even though these flavonoids have fascinating properties (Table 1), their application was limited to Sn-based PSCs and therefore might present an interesting development when considered in future research on Pb-based PSCs.
Table 1 A summary of the photovoltaic parameters for the phytochemical-modified perovskite solar cells relative to the control pristine (values in brackets)
Phytochemical |
FG |
Interaction with perovskite |
J
sc (mA cm−2) |
V
oc (V) |
FF (%) |
PCE (%) |
Ref. |
|
–OH & –C C |
H-bond with N–H group & I− ions, favorable interactions for crystal formation |
23.0 (23.1) |
1.059 (1.051) |
72.1 (66.2) |
17.5 (16.1) |
130
|
|
–C C |
C C π coordination with Pb2+, favorable interactions for crystal formation |
23.86 (22.78) |
1.20 (1.14) |
79.43 (74.92) |
22.72 (19.48) |
117
|
|
–NH–CO–, –OH, C C |
H-bond with N–H group |
23.01 (22.30) |
1.142 (1.092) |
79.39 (75.66) |
20.85 (18.43) |
134
|
Favorable interactions for crystal formation: reduced trap density & improved charge carrier transport |
|
–NH, –C O, C C |
H-bond with N–H group of the film; C C π & –C O coordination with Pb2+ |
24.85 (23.92) |
1.150 (1.06) |
79 (78) |
22.80 (19.45) |
142
|
Favorable interactions for crystal formation and defect passivation. |
|
–C C–, –COOH |
H-bond with N–H group of the film; C C π & –C O coordination with Pb2+ |
23.5 (24.4) |
0.93 (0.86) |
41.7 (36) |
9.11 (7.62) |
146
|
Favorable interactions for crystal formation and defect passivation |
|
–C( O)–OH |
H-bond with N–H group of perovskite film; C C π & –C O coordination with Pb2+ |
21.40 (20.21) |
1.18 (1.10) |
79 (73) |
21.36 (18.08) |
144
|
Favorable interactions for crystal formation and defect passivation |
|
–OH, –COOH, –C C |
H-bond with N–H group of perovskite film; C C π & –C O coordination with Pb2+, free oxygen radical scavenging through phenol; antioxidant activity |
19.73 (19.07) |
0.61 (0.28) |
69.4 (53.9) |
8.32 (2.82) |
124
|
Favorable interactions for crystal formation and defect passivation: improved stability of the PSC. |
|
–COOH |
H-bond with N–H group of the film; –C O coordination with Pb2+; favorable interactions for crystal formation and defect passivation |
23.83 (20.64) |
0.95 (0.92) |
75 (73) |
17.12 (14.06) |
147
|
Improved thermal stability of the PSC. |
|
–OH, COOH, –C C |
H-bond with N–H group of perovskite material; C C π & –C O coordination with Pb2+; free oxygen radical scavenging through phenol; antioxidant activity; improved phase purity (eliminates PbI2 & SnI2 impurities) |
28.99 (28.28) |
0.839 (0.785) |
76.51 (76.65) |
18.60 (17.01) |
125
|
|
–COOR, –C ≡ N, –C C– |
H-bond with N–H group of perovskite film; C C π & –C O coordination with Sn2+ |
22.91 (22.06) |
1.115 (1.08) |
80.41 (83.50) |
20.54 (19.89) |
150
|
Favorable interactions for crystal formation and defect passivation |
Improved stability of the Sn-PSC. |
|
–COOH |
H-bond with N–H group of perovskite film; –C O coordination; favorable interactions for crystal formation and defect passivation |
21.37 (21.46) |
0.95 (0.94) |
80 (78) |
16.43 (16.01) |
145
|
|
–COOH, phenol |
H-bond with N–H group of perovskite film; C C π & –C O σ coordination; free |
18.08 (17.94) |
1.30 (1.256) |
82.17 (76.88) |
19.31 (17.33) |
126
|
Oxygen radical scavenging through phenol; antioxidant activity; favorable interactions for crystal formation and defect passivation |
Improved stability of the PSC. |
|
–NR, –C O |
H-bond with N–H group of perovskite film; –C C π & –C O coordination; favorable interactions for crystal formation and defect passivation |
25.04 (24.78) |
1.178 (1.164) |
75.76 (72.88) |
22.32 (20.36) |
138
|
|
–NR, –C O |
H-bond with N–H group of perovskite film; –C C π & –C O coordination; favorable interactions for crystal formation and defect passivation |
25.24 (24.78) |
1.191 (1.164) |
78.11 (72.88) |
22.64 (20.36) |
138
|
|
–NR, –C O |
H-bond with N–H group of the film; –C C π & –C O coordination; favorable interactions for crystal formation and defect passivation |
24.27 (24.78) |
1.163 (1.164) |
71.58 (72.88) |
20.24 (20.36) |
138
|
|
Improved stability of the PSC. |
|
–OH & –C C |
H-bond with N–H group of the film; –OH & –C C |
23.43 (22.59) |
1.106 (1.078) |
81.57 (78.62) |
21.15 (19.14) |
131
|
|
Favorable interactions for crystal formation and defect passivation |
Catechin, a flavanol that occurs naturally and is a great antioxidant, was added to a Sn-based perovskite film to reduce the oxidation problem.128 By using catechin, the researchers were able to simultaneously improve the stability and PCE of Sn-based PSCs. With catechin as a dopant, the PCE improved from an average value of 4.29% to an average value of 5.45% (Champion = 6.69%) for catechin-doped PSC devices.
3.1.3 Terpenes and terpenoids.
These groups of phytochemicals are identified by the isoprene structural units that are present in their structures. They are classified into monoterpenes (two isoprene), sesquiterpenes (three isoprene), diterpenes (four isoprene), and triterpenes (six isoprene units) depending on the number of isoprene units in their structures. Terpenoids are functionalized terpenes, with the oxidized terpenes forming the largest percentage of terpenoids. Alpha (α)-terpineol belongs to the terpenoids group and can be extracted from oil plants.129 It has recently been used as a solvent additive to boost the performance of PSCs. The film was more uniform and had better crystallinity after α-terpineol was added to the perovskite precursors. So, the PSC that was made with the α-terpineol additive had better PCE, stability, and repeatability compared to the control device.130Fig. 10 shows a comparison of the properties of the perovskite film and the behavior of PSCs with and without the α-terpineol additive. It is seen that the presence of the α-terpineol additive improved the water repellency of the perovskite film and the PCE and stability of the PSC (Fig. 10(e)).
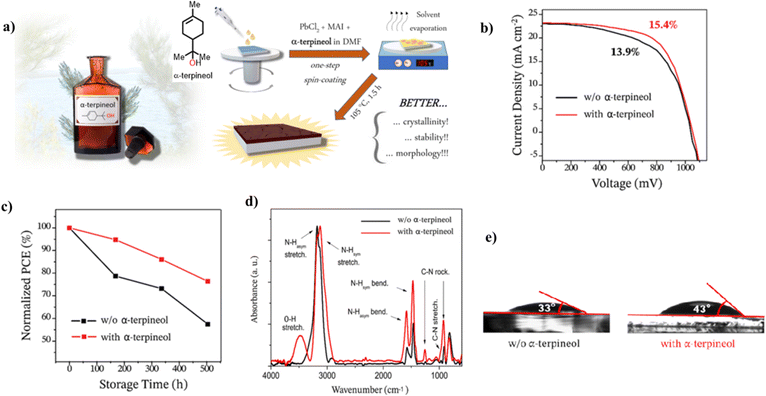 |
| Fig. 10 (a) Chemical structure of α-terpineol and the steps involved in the fabrication of the perovskite film, (b) J–V curves for the PSC fabricated with and without α-terpineol, (c) normalized PCE for the PSC with and without α-terpineol, (d) FTIR spectra of the perovskite film with and without α-terpineol, and (e) water contact angle for the perovskite film with and without α-terpineol. Reproduced from ref. 130 with permission from America Chemical Society, Copyright (2021). | |
Xiong et al.131 used betulin as an additive to control the crystallization of the MAPbI3-based perovskite film. Fig. 11 shows how the presence of betulin in the perovskite precursors improved the morphology of the film, thereby reducing its surface roughness. The improvement in the PCE and stability stemmed from betulin's ability to passivate uncoordinated Pb2+via Lewis acid-based reactions, as revealed by the XPS analysis. The strong hydrogen bonding interaction between the H atom from –NH3+ in the perovskite and the O atom from the –OH group of betulin stabilized the perovskite structure and suppressed ion migration and surface charge trapping. Furthermore, the hydrophobicity of betulin increased the water contact angle from 36.6° to 71.4°, improving the water repellency of the perovskite film, which improved its stability against moisture exposure. With the incorporation of betulin additive in the perovskite film, the PCE of PSC improved from 19.14% to 21.15%, and the hysteresis index decreased from 0.054 to 0.029.
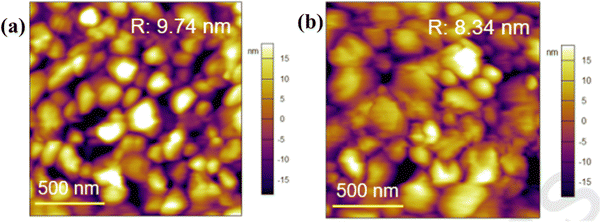 |
| Fig. 11 Comparison of the surface roughness of the perovskite film (a) without and (b) with betulin; reproduced from ref. 131 with permission from Elsevier Inc., Copyright (2019). | |
3.1.4 Alkaloids.
Alkaloids are nitrogen-containing phytochemicals commonly referred to as organic bases. They include nicotine, quinoline, capsaicinoids, acridone, theophylline, caffeine, and theobromine, among others.132 Capsaicin, a common alkaloid from the capsaicinoids class majorly obtained from chili pepper, has been used in the fabrication of PSCs.133 Xiong and his team added capsaicin to a perovskite film as an antisolvent. They observed that it improved the shape of the perovskite film, made charge carriers move around more easily, reduced defect-mediated recombination, and modified the interface with the CTL, which led to improved charge carrier extraction and PCE in the PSC.134 This improvement was attributed to the favorable interactions, mainly hydrogen bonding, between the –NH2 and –C
O groups of capsaicin with the MA+ and the uncoordinated Pb2+ ions of the MAPbI3-based perovskite film, which reduced the density of defects in the perovskite film leading to an enhancement in the charge carrier collection efficiency in the capsaicin-modified PSC device. The HI was also reduced by about 1.8% for the capsaicin-based PSC (HI = 1.4%) relative to the control device (HI = 3.4%).137 Li and co-workers used quinoline as an additive in perovskite precursor solutions and reported that the presence of quinoline in the right amounts improved the perovskite film morphology and crystallinity, reducing the density of defects and consequently the non-radiative recombination power losses, which led to the observed improvement in the PCE and stability of the PSC.135 The presence of quinoline also led to a reduction in hysteresis in the PSC as indicated by the reduction in HI from 0.049 to 0.012.
Caffeine has also been incorporated into PSCs as an additive during their fabrication.136 The presence of the –C
O group interacted with Pb2+ ions in the perovskite, modulating its crystallization, inducing preferred crystal orientation, and improving the film morphology, thereby reducing the defect density and improving the charge transport in the resulting PSC device. Fig. 12 shows a comparison of the charge carrier dynamics, J–V curves, and EQE of the control and caffeine-containing PSC devices. The latest study on the use of caffeine as an additive in PSCs was carried out by Dhanabal et al.,137 who incorporated caffeine into a MAPI-based perovskite film in different proportions. In their study, they also observed an improvement in the performance of PSC devices when modified with caffeine. They found that 5 wt% of caffeine was the optimum proportion that yielded the highest PCE and stability.
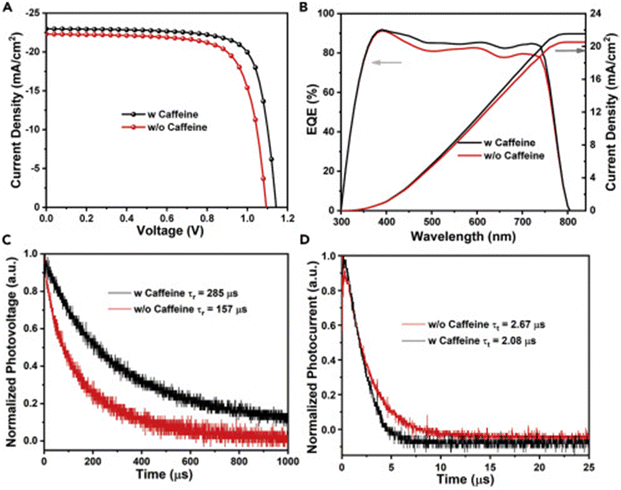 |
| Fig. 12 Comparison of (A) J–V curves; (B) EQE curves; (C) normalized transient photovoltage decay; and (D) normalized transient photocurrent decay for pristine and caffeine-modified PSC devices. Reproduced from ref. 136 with permission from Elsevier Inc. (2019). | |
Wang and his research team138 used three purine alkaloids (caffeine, theophylline, and theobromine) as molecular additives in the fabrication of PSCs. Their study focused on the interaction between the defects and the functional groups (–C
O and –NH2) in these molecular additives and how this could influence the device PCE and stability. Their results revealed that the presence of both –C
O and –NH groups played a pivotal role in surface defect passivation in the perovskite film where the hydrogen-bond formation between –NH2 and iodine aided –C
O binding with Pb antisite defects. The theophylline and caffeine additives effectively passivated the surface defects in the perovskite films, leading to improvements in the PCE and stability of the PSC. The PSC device with theophylline additive had a higher PCE and better operational stability compared to the control and the devices with caffeine. However, the theobromine-containing PSC device showed a decline in the PCE relative to the control device. The difference in the observed performance is due to the differences in the way these molecular additives interact with the defects in the perovskite film. Fig. 13 shows the top view of the surface defects, the interaction of the different additives with the defects, and the J–V curves of the PSC devices.
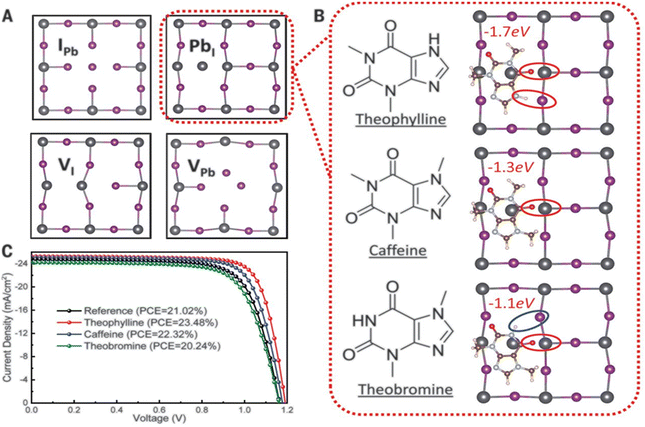 |
| Fig. 13 (A) Top view of the surface defects in the perovskite films. (B) Interaction of the defects with additives; and (C) comparison of the J–V curves of theophylline-, caffeine-, and theobromine-based PSCs and the control device, reproduced from ref. 138 with permission from American Association for the Advancement of Science (2019). | |
Liu et al.139 used coumarin 343 (C343) dye as a defect passivator in FA-based PSCs. The presence of the –C
O group in this compound helped in passivating defects on the surface of the perovskite film by coordinating with the uncoordinated Pb2+, thereby prolonging the charge carrier lifetime and improving interfacial charge carrier extraction. This led to an improvement in device PCE and operational stability. In addition to defect passivation, coumarin improved the hydrophobicity of the perovskite film, thus improving the moisture stability of the PSCs.139 Coumarin is also said to be a good residual stress reliever, a phase segregation inhibitor, and deformable. This means that it can be used to make flexible PSCs that are stable mechanically and have good performance.140
3.1.5 Indigoids.
These are classes of natural dyes obtained from the Indigofera genus plant.141 They are insoluble in water and have functional groups that make them suitable for improving moisture-tolerance and passivating defects in perovskite films. Indigo molecules were used by Guo and his team142 to make a perovskite film that had good morphological properties and better crystal quality. The –C
O and –NH2 groups present in indigo acted as chemical defect passivators, improving the PCE and stability of PSC. Fig. 14 shows the effects of indigo molecules on the properties of the perovskite film and the effects on the PV parameters and degradation properties of the PSC. It is seen that the presence of indigo in the film improves the Jsc and Voc, and the indigo-modified PSC device can retain over 80% of its initial PCE for a longer time compared to the control device.
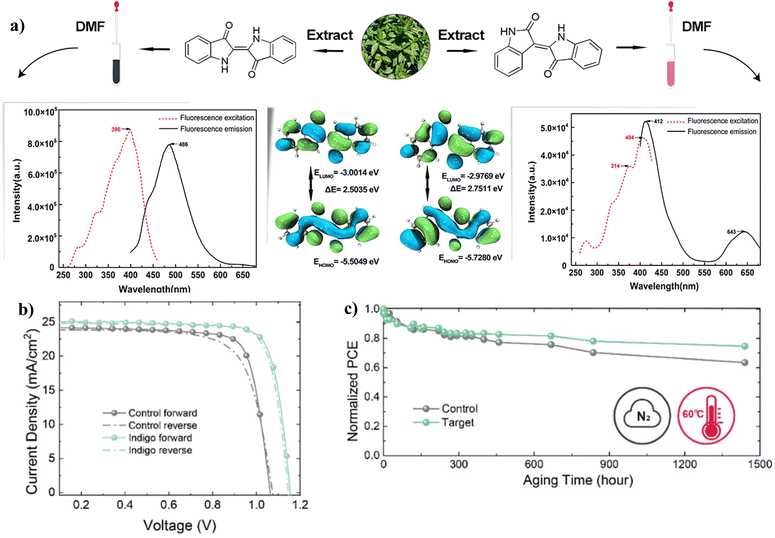 |
| Fig. 14 (a) Structures of indigotin (left) and its oxidized derivative indirubin (right),141 (b) J–V curves for PSCs with and without indigo. (c) Normalized PCE for PSCs with and without indigo; reproduced from ref. 142 with permission from Wiley-VCH GmbH (2022). | |
3.1.6 Carboxylic acids.
Carboxylic acids are phytochemicals characterized by the presence of the –COOH functional group in their structures. Recently, carboxylic acids have attracted the attention of researchers in the solar cell industry, mainly in the development of PSCs.143 This has been attributed to the attractive properties of the –COOH functional group in coordination with the uncoordinated divalent cations of the perovskite films and their hydrogen bonding interactions, which are known to influence the crystallization quality of the perovskite film.144 By using lauric acid as an interfacial modifier in the perovskite film, Yang's team was able to improve the performance and stability of PSCs significantly (ΔPCE = 3.28%).144 The –COOH of lauric acid passivated in the surface defects leading to a reduction in non-radiative power losses whereas its alkyl chain improved the moisture resistance of the perovskite film, and hence the stability in the PSC. Fig. 15 compares the J–V curves and variation in PCE over time for the control and lauric acid-modified PSC, showing the importance of lauric acid in improving the PV parameters and operational stability of the PSC. Similarly, the use of stearic acid as an additive enhanced the moisture stability of the PSC and its morphology through defect passivation.145
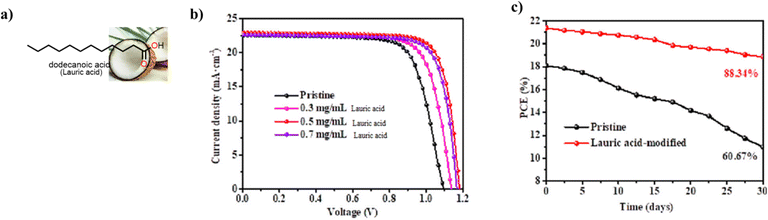 |
| Fig. 15 (a) Structure of lauric acid, (b) J–V curves for PSC with and without lauric acid additive and (c) variation of PCE with time for PSC with and without lauric acid additive, reproduced from ref. 144 with permission from American Chemical Society (2022). | |
In a recent study, Abdelmageed et al.146 reported improved PSC stability by using oleic acid (OA) as an interfacial modifier under a highly humid environment (relative humidity, RH ∼76.7%). The –COOH group of OA passivated the defects and led to the formation of a thin hydrophobic layer over the perovskite film surface that prevented water ingress. This is what brought the observed improvement in PCE and stability of the OA-modified PSC device.
Other non-fatty acids, such as oxalic acid, citric acid, malonic acid, and malic acid, have also been used as additives in the fabrication of PSCs.147–149 Guo et al.148 observed that the introduction of citric acid into the perovskite film modulated the crystallization kinetics of the film, leading to an improvement in its crystal quality. Whereas the pristine device gave a polycrystalline mixture of cuboid and elongated dodecahedral crystals on crystallization, the addition of citric acid to the precursor solution led to the formation of closely packed, cuboidal crystals, which resulted in a perovskite film with better morphology. As a result, the citric acid-modified PSC device showed a better PCE relative to the pristine device (ΔPCE = 3.51%). A fascinating observation in this system is that the PCE of the citric acid-based PSC displayed light illumination-dependent performance in the order of 28.1% (under white light LED 100Ix) > 20.4% 0.087 suns (equivalent to a rainy day) > 16.75% under 1 sun (100 mW cm−2). This implies that the citric acid-based PSCs are suitable for applications under low light illumination, which include indoor applications. These notable properties of citric acid in crystal engineering are associated with stronger hydrogen bonding of the –COOH of citric acid (compared to that of malonic acid used in the same study)148 to the –NH of the film and the coordination ability of the multiple –COOH groups to the uncoordinated divalent cations of the perovskite film. By adding malonic acid (Fig. 16) to the precursor solution, Mohammed et al.149 made a better MAPI-based PSC without a hole transport material, which had a PCE of 14.14% (relative to 11.88% for the reference device). The better performance (ΔPCE = 2.26%) of this malonic acid-modified PSC was achieved on account of suppressed charge carrier recombination due to improved film morphology. The malonic acid-based PSC device also showed improved stability as it could retain over 80% of the initial PCE for over 800 h.
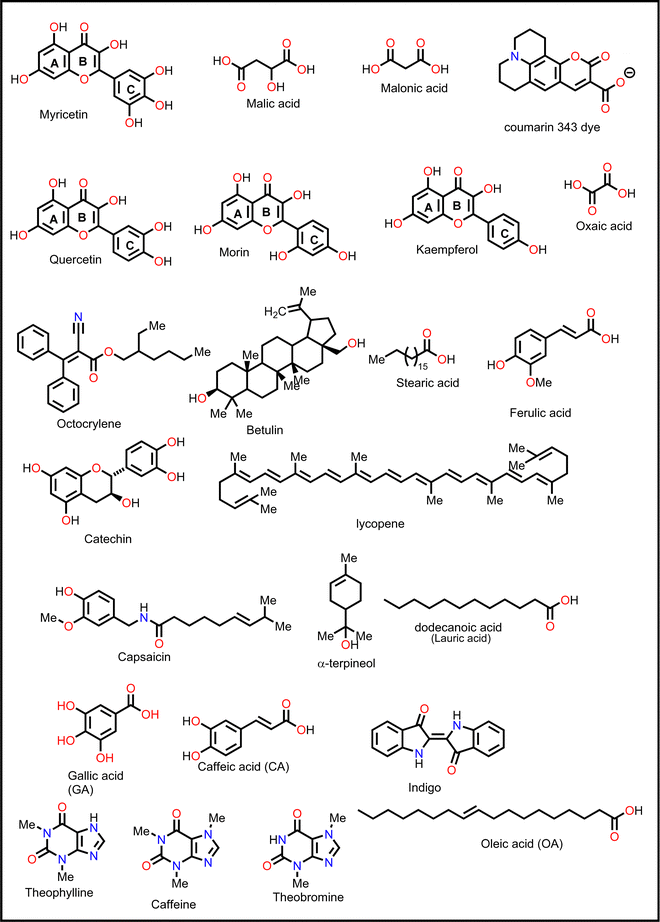 |
| Fig. 16 Chemical structures of some of the phytochemicals that have been used in PSCs. | |
Adil Afroz et al.147 used oxalic acid as an additive in the precursor solution to fabricate a MAPI-based PSC, which further demonstrates the performance versatility of the carboxylic acids. In their study, they obtained an oxalic acid-modified PSC with a better PCE (ΔPCE = 3.06%) and enhanced stability (retained over 90% of the initial PCE at 100 °C versus 14% of the reference pristine after 9 h). This was attributed to the strong hydrogen bonding from the bifacial –COOH group, which modulated the perovskite film crystallization and led to excellent crystal quality, increased grain sizes and improved surface passivation. Phytochemical-based esters, also known as carboxylic acid derivatives, have also been applied as additives in the fabrication of PSCs. For example, Huang et al.150 reported an improved performance of a Sn-based PSC (ΔPCE = 0.65% relative to the reference device) and a reduction in hysteresis index on using octocrylene as an additive. Octocrylene (Fig. 16) is a phytochemical-based lipophilic ester (log
kow = 6.88) which is resistant to sunlight degradation and hence a common UV filter. The improved moisture stability of the octocrylene-modified PSC was attributed to the lipophilic nature of octocrylene and defect passivation through –C
O coordination. Fig. 16 shows the chemical structures of some of the phytochemical-based additives that have been used in PSCs.
Overall, most of the phytochemical-based additives that have been applied in the literature to fabricate PSCs have shown good performances, as indicated by the reported improvement in the PCE (i.e., positive values of ΔPCE) and improvement in stability under exposure to varied environmental conditions. The improvement in performance has mainly been attributed to the presence of various functional groups in their chemical structures, which interacted with different ionic or atomic species in the perovskite films, thus modulating their crystal quality, morphology, charge carrier mobilities, defect chemistry, work function, and resistance to degradation. All these lead to a reduction in defect-mediated recombination power losses as well as reduction in the oxygen-, moisture-, heat- and light-induced degradation, which improves the PCE and stability of PSCs. A summary of the relative improvement in the PCE in PSCs with different classes of phytochemical-based additives relative to the pristine devices used in the empirical studies is provided in Fig. 17.
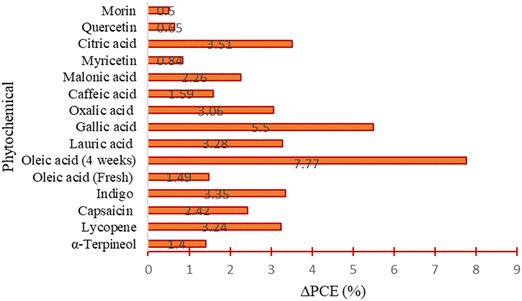 |
| Fig. 17 Comparison of ΔPCE values for PSCs modified with different phytochemical-based additives. | |
It is worth noting that several phytochemical-based compounds exist, and their effects on the overall performance of PSC are dependent on the strength of the chemical bonding interaction and coordination between their functional groups (FGs) and the ionic or atomic species in the perovskite material. This interaction determines their defect passivation efficacy and longevity as well as perovskite lattice stabilization, residual stress relief, phase segregation, ion migration and degradation inhibition capabilities. FGs with a terminal O atom and those with lone pairs of electrons on their N or S functionalities are effective in controlling perovskite crystal growth and defect passivation by virtue of their ability to bond and coordinate well with atoms in the perovskite material, form Lewis acid–base adducts and anchor well to the perovskite surface. Other FGs are effective in stabilizing perovskite precursors and the perovskite structure as well as improving the interfacial energetics in PSCs for improved charge transport. Whenever a number of FGs co-exist, their synergistic effects may lead to the formation of perovskite films with excellent optoelectronic properties and PSCs with good performance. However, other factors such as the molecular configuration of the additive, nature of the perovskite precursors and solvents used, steric hindrance effects, design of PSC, and post-treatment techniques also play a role in the performance of PSCs. A summary of the PV parameters of PSCs with and without phytochemical-based additives is given in Table 1. It is seen that different phytochemicals can improve the PCE of PSCs to different degrees and no generalization can be made about the best class of phytochemicals to use. With regards to the stability of PSCs, the ability of the phytochemical-based additive to passivate defects and suppress oxygen-, moisture-, UV-light- and thermal-induced degradation depends on their ability to modulate the perovskite film morphology and crystal quality as well as their free radical scavenging, residual stress relief, perovskite surface anchoring, hydrophobic surface layer formation and perovskite phase stabilization capabilities. This is usually controlled by the interaction and coordination effects between their FGs and the atoms or ions in the perovskite material. It is therefore important to explore the use of more phytochemical-based additives in the preparation of PSCs, as this can help in establishing the criteria to use when selecting the best phytochemical to use as additives and can bring a breakthrough in addressing the challenges hindering the commercialization of PSC technology.
4. Conclusion and future prospects for the development of PSCs
In summary, this review focused on the recent empirical results on the use of phytochemicals as additives in PSCs. A detailed assessment of the effects of each category of phytochemicals on the properties of perovskite films and performance of PSCs was performed, as a means to unravel the key factors responsible for them. It is clear from the comparison that the functional groups present in the phytochemical-based additives play important roles during perovskite film formation ranging from modulating the nucleation and crystallization dynamics, to inhibiting precipitation of defects, passivating defects at grain boundaries and engineering the work function. All these help in the formation of perovskite films with good morphology, improved crystal quality and improved resistance to degradation. For carboxylic acid-based phytochemicals, the hydrogen bonding interactions with the –NH groups and the uncoordinated Pb2+ or Sn2+ ions were found to influence the perovskite crystallization kinetics leading to perovskite films with improved crystal quality and reduced defect density. The multifunctional groups present in phenolic compounds were also found to play a key role as degradation inhibitors in perovskite films by virtue of their free radical scavenging capability, precursor stabilization and ability to passivate defects in the bulk and on the surface of perovskite films.
In addition to the functional group-rich chemical structures, the high antioxidant activities and hydrophobic nature of some phytochemicals endows them with excellent qualities for addressing the challenges of non-radiative recombination power loss and stability in PSCs. Future research on PSCs should consider using phytochemical-based additives in perovskite precursors to improve their performance. Special attention should be given to phytochemicals derived from invasive wild plants, commonly known in the agricultural sector as weeds, which in the context of agricultural production, present an economic burden to farmers and reduce crop yields. Furthermore, phytochemicals can also be extracted from agricultural plant wastes such as stalks, fruit peels and other parts that are usually thrown away as waste. The use of phytochemicals derived from such weed plants or plant wastes in additive engineering of PSC technology will render them beneficial and of great economic importance to farmers. More research work in the future should therefore focus on investigating the effects of specific classes of phytochemicals derived from weed plants or agricultural plant wastes and their synergistic effects when applied in combined forms or as mixtures.
Data availability
No data was collected in this study. The data used in the review has been included in the main text of the document.
Author contributions
NC: writing original draft; GMM: writing original draft; MMG: writing original draft and editing; KKR: review and editing; GKY: review and editing; SCC: review and editing; RKC: review and editing; DS: review and editing; BA: review and editing; EN: review and editing.
Conflicts of interest
The authors declare no competing interest.
Acknowledgements
The authors acknowledge the financial support of the PASET-RSIF through the JIRA research grant (RSIF/JIRA/003) and MIRET project. N. C. and G. M. M. acknowledge the African Centre of Excellence (ACE II)–Phytochemicals, Textile & Renewable Energy (PTRE), Moi University, for the Scholarships.
References
- International Energy Agency, Global energy data: Energy Statistics Data Browser, 2023. Accessed: Oct. 27, 2023. [Online]. Available: https://www.iea.org/data-and-statistics/data-tools/energy-statistics-data-browser?country=WORLD&fuel=Energy%20supply&indicator=TESbySource.
-
M. Hafner and S. Tagliapietra, The Global Energy Transition: A Review of the Existing Literature, in The Geopolitics of the Global Energy Transition Lecture Notes in Energy, ed. M. Hafner and S. Tagliapietra, Springer International Publishing, Cham, 2020, vol. 73, pp. 1–24 DOI:10.1007/978-3-030-39066-2_1.
- S. Harichandan, S. K. Kar, R. Bansal, S. K. Mishra, M. S. Balathanigaimani and M. Dash, Energy transition research: A bibliometric mapping of current findings and direction for future research, Cleaner Prod. Lett., 2022, 3, 100026, DOI:10.1016/j.clpl.2022.100026.
- G. Mutezo and J. Mulopo, A review of Africa's transition from fossil fuels to renewable energy using circular economy principles, Renewable Sustainable Energy Rev., 2021, 137, 110609, DOI:10.1016/j.rser.2020.110609.
- T. S. Genc and S. Kosempel, Energy Transition and the Economy: A Review Article, Energies, 2023, 16(7), 2965, DOI:10.3390/en16072965.
- I. R. E. A. IRENA, World Energy Transitions Outlook 2023: 1.5 °C Pathway. [Online]. Available: https://www.irena.org/Publications/2023/Jun/World-Energy-Transitions-Outlook-2023.
- SRREN, Renewable Energy Sources and Climate Change Mitigation: Special Report of the Intergovernmental Panel on Climate Change. Accessed: Nov. 09, 2023. [Online]. Available: https://www.ipcc.ch/site/assets/uploads/2018/03/SRREN_Full_Report-1.pdf.
- G. Kavlak, J. McNerney and J. E. Trancik, Evaluating the causes of cost reduction in photovoltaic modules, Energy Policy, 2018, 123, 700–710, DOI:10.1016/j.enpol.2018.08.015.
- V. V. Tyagi, N. A. A. Rahim, N. A. Rahim and J. A. L. Selvaraj, Progress in solar PV technology: Research and achievement, Renewable Sustainable Energy Rev., 2013, 20, 443–461, DOI:10.1016/j.rser.2012.09.028.
- J. Kettle,
et al., Review of technology specific degradation in crystalline silicon, cadmium telluride, copper indium gallium selenide, dye sensitised, organic and perovskite solar cells in photovoltaic modules: Understanding how reliability improvements in mature technologies can enhance emerging technologies, Prog. Photovolt. Res. Appl., 2022, 30(12), 1365–1392, DOI:10.1002/pip.3577.
- A. Furasova,
et al., Resonant Silicon Nanoparticles for Enhanced Light Harvesting in Halide Perovskite Solar Cells, Adv. Opt. Mater., 2018, 6(21), 1800576, DOI:10.1002/adom.201800576.
- J. Pastuszak and P. Węgierek, Photovoltaic Cell Generations and Current Research Directions for Their Development, Materials, 2022, 15(16), 5542, DOI:10.3390/ma15165542.
- M. Giannouli, Current Status of Emerging PV Technologies: A Comparative Study of Dye-Sensitized, Organic, and Perovskite Solar Cells, Int. J. Photoenergy, 2021, 2021, 1–19, DOI:10.1155/2021/6692858.
- M. A. Green,
et al., Solar cell efficiency tables (Version 61), Prog. Photovolt. Res. Appl., 2023, 31(1), 3–16, DOI:10.1002/pip.3646.
- A. Kojima, K. Teshima, Y. Shirai and T. Miyasaka, Organometal Halide Perovskites as Visible-Light Sensitizers for Photovoltaic Cells, J. Am. Chem. Soc., 2009, 131(17), 6050–6051, DOI:10.1021/ja809598r.
- M. A. Green, A. Ho-Baillie and H. J. Snaith, The emergence of perovskite solar cells, Nat. Photonics, 2014, 8(7), 506–514, DOI:10.1038/nphoton.2014.134.
- N. Suresh Kumar and K. Chandra Babu Naidu, A review on perovskite solar cells (PSCs), materials and applications, J. Materiomics, 2021, 7(5), 940–956, DOI:10.1016/j.jmat.2021.04.002.
- H. J. Snaith, Perovskites: The Emergence of a New Era for Low-Cost, High-Efficiency Solar Cells, J. Phys. Chem. Lett., 2013, 4(21), 3623–3630, DOI:10.1021/jz4020162.
- M. Hasan and K. A. Khan, Dynamic model of Bryophyllum pinnatum leaf fueled BPL cell: a possible alternate source of electricity at the off-grid region in Bangladesh, Microsyst. Technol., 2019, 25(6), 6, DOI:10.1007/s00542-018-4149-y.
- Z. Yang,
et al., Unraveling the Exciton Binding Energy and the Dielectric Constant in Single-Crystal Methylammonium Lead Triiodide Perovskite, J. Phys. Chem. Lett., 2017, 8(8), 1851–1855, DOI:10.1021/acs.jpclett.7b00524.
- M. B. Johnston and L. M. Herz, Hybrid Perovskites for Photovoltaics: Charge-Carrier Recombination, Diffusion, and Radiative Efficiencies, Acc. Chem. Res., 2016, 49(1), 146–154, DOI:10.1021/acs.accounts.5b00411.
- L. A. Zafoschnig, S. Nold and J. C. Goldschmidt, The Race for Lowest Costs of Electricity Production: Techno-Economic Analysis of Silicon, Perovskite and Tandem Solar Cells, IEEE J. Photovolt., 2020, 10(6), 1632–1641, DOI:10.1109/JPHOTOV.2020.3024739.
- H. S. Jung, G. S. Han, N.-G. Park and M. J. Ko, Flexible Perovskite Solar Cells, Joule, 2019, 3(8), 1850–1880, DOI:10.1016/j.joule.2019.07.023.
- Z. Song, S. C. Watthage, A. B. Phillips and M. J. Heben, Pathways toward high-performance perovskite solar cells: review of recent advances in organo-metal halide perovskites for photovoltaic applications, J. Photonics Energy, 2016, 6(2), 022001, DOI:10.1117/1.JPE.6.022001.
- S. A. Deepika, A. Singh, U. K. Verma and A. Tonk, Device Structures of Perovskite Solar Cells: A Critical Review, Phys. Status Solidi A, 2023, 220(9), 2200736, DOI:10.1002/pssa.202200736.
- J. Shi,
et al., From Ultrafast to Ultraslow: Charge-Carrier Dynamics of Perovskite Solar Cells, Joule, 2018, 2(5), 879–901, DOI:10.1016/j.joule.2018.04.010.
- W. Cha, W.-Y. Cha, I. Noh, S. Seki, H. Ohkita and D. Kim, Controlling the charge carrier dynamics by modulating the orientation diversity of perovskites, Mater. Chem. Front., 2022, 6(8), 1026–1032, 10.1039/D1QM01586A.
- T. Zhao, W. Shi, J. Xi, D. Wang and Z. Shuai, Intrinsic and Extrinsic Charge Transport in CH3NH3PbI3 Perovskites Predicted from First-Principles, Sci. Rep., 2016, 6(1), 19968, DOI:10.1038/srep19968.
- A. Solanki, P. Yadav, S.-H. Turren-Cruz, S. S. Lim, M. Saliba and T. C. Sum, Cation influence on carrier dynamics in perovskite solar cells, Nano Energy, 2019, 58, 604–611, DOI:10.1016/j.nanoen.2019.01.060.
- J. Peng, Y. Chen, K. Zheng, T. Pullerits and Z. Liang, Insights into charge carrier dynamics in organo-metal halide perovskites: from neat films to solar cells, Chem. Soc. Rev., 2017, 46(19), 5714–5729, 10.1039/C6CS00942E.
- S. Wang,
et al., Impact of loss mechanisms on performances of perovskite solar cells, Phys. B, 2022, 647, 414363, DOI:10.1016/j.physb.2022.414363.
- C. M. Wolff, P. Caprioglio, M. Stolterfoht and D. Neher, Nonradiative Recombination in Perovskite Solar Cells: The Role of Interfaces, Adv. Mater., 2019, 31(52), 1902762, DOI:10.1002/adma.201902762.
- C. Ding,
et al., Effect of the conduction band offset on interfacial recombination behavior of the planar perovskite solar cells, Nano Energy, 2018, 53, 17–26, DOI:10.1016/j.nanoen.2018.08.031.
- S. Ma, S. Sansoni, T. Gatti, P. Fino, G. Liu and F. Lamberti, Research Progress on Homogeneous Fabrication of Large-Area Perovskite Films by Spray Coating, Crystals, 2023, 13(2), 216, DOI:10.3390/cryst13020216.
- Y. Wang,
et al., Charge Carrier Dynamics in Electron-Transport-Layer-Free Perovskite Solar Cells, ACS Appl. Electron. Mater., 2019, 1(11), 2334–2341, DOI:10.1021/acsaelm.9b00528.
- C. Zhou,
et al., Unveiling Charge Carrier Recombination, Extraction, and Hot-Carrier Dynamics in Indium Incorporated Highly Efficient and Stable Perovskite Solar Cells, Adv. Sci., 2022, 9(11), 2103491, DOI:10.1002/advs.202103491.
- G. Yang,
et al., Study on carrier dynamics of perovskite solar cells via transient absorption, J. Alloys Compd., 2023, 952, 170051, DOI:10.1016/j.jallcom.2023.170051.
- Y. Yin, M. Wang, V. Malgras and Y. Yamauchi, Stable and Efficient Tin-Based Perovskite Solar Cell via Semiconducting–Insulating Structure, ACS Appl. Energy Mater., 2020, 3(11), 10447–10452, DOI:10.1021/acsaem.0c01422.
- Y. Yin,
et al., Efficient and Stable Ideal Bandgap Perovskite Solar Cell Achieved by a Small Amount of Tin Substituted Methylammonium Lead Iodide, Electron. Mater. Lett., 2020, 16(3), 224–230, DOI:10.1007/s13391-020-00206-3.
- T. Wu,
et al., The Main Progress of Perovskite Solar Cells in 2020–2021, Nano-Micro Lett., 2021, 13(1), 152, DOI:10.1007/s40820-021-00672-w.
- S. Liu, V. P. Biju, Y. Qi, W. Chen and Z. Liu, Recent progress in the development of high-efficiency inverted perovskite solar cells, NPG Asia Mater., 2023, 15(1), 1, DOI:10.1038/s41427-023-00474-z.
- N. Elumalai, M. Mahmud, D. Wang and A. Uddin, Perovskite Solar Cells: Progress and Advancements, Energies, 2016, 9(11), 861, DOI:10.3390/en9110861.
- S. A. Olaleru, J. K. Kirui, D. Wamwangi, K. T. Roro and B. Mwakikunga, Perovskite solar cells: The new epoch in photovoltaics, Sol. Energy, 2020, 196, 295–309, DOI:10.1016/j.solener.2019.12.025.
- D. Zhang, D. Li, Y. Hu, A. Mei and H. Han, Degradation pathways in perovskite solar cells and how to meet international standards, Commun. Mater., 2022, 3(1), 58, DOI:10.1038/s43246-022-00281-z.
- H.-Q. Wang,
et al., Understanding degradation mechanisms of perovskite solar cells due to electrochemical metallization effect, Sol. Energy Mater. Sol. Cells, 2021, 230, 111278, DOI:10.1016/j.solmat.2021.111278.
- S. Mazumdar, Y. Zhao and X. Zhang, Stability of Perovskite Solar Cells: Degradation Mechanisms and Remedies, Front. Electron., 2021, 2, 712785, DOI:10.3389/felec.2021.712785.
- S. Kundu and T. L. Kelly,
In situ studies of the degradation mechanisms of perovskite solar cells, EcoMat, 2020, 2(2), e12025, DOI:10.1002/eom2.12025.
- C. Li,
et al., Low-bandgap mixed tin–lead iodide perovskites with reduced methylammonium for simultaneous enhancement of solar cell efficiency and stability, Nat. Energy, 2020, 5(10), 768–776, DOI:10.1038/s41560-020-00692-7.
- I. M. Dharmadasa, Y. Rahaq and A. E. Alam, Perovskite solar cells: short lifetime and hysteresis behaviour of current–voltage characteristics, J. Mater. Sci. Mater. Electron., 2019, 30(14), 12851–12859, DOI:10.1007/s10854-019-01759-2.
- Z. Wang,
et al., Modulated crystal growth enables efficient and stable perovskite solar cells in humid air, Chem. Eng. J., 2022, 442, 136267, DOI:10.1016/j.cej.2022.136267.
- M. Minbashi and E. Yazdani, Comprehensive study of anomalous hysteresis behavior in perovskite-based solar cells, Sci. Rep., 2022, 12(1), 14916, DOI:10.1038/s41598-022-19194-5.
- M. Cheng,
et al., Charge-transport layer engineering in perovskite solar cells, Sci. Bull., 2020, 65(15), 1237–1241, DOI:10.1016/j.scib.2020.04.021.
- A. Muradov, D. Frolushkina, V. Samusenkov, G. Zhamanbayeva and S. Kot, Methods of Stability Control of Perovskite Solar Cells for High Efficiency, Energies, 2021, 14(10), 2918, DOI:10.3390/en14102918.
- J. Kumar, P. Srivastava and M. Bag, Advanced Strategies to Tailor the Nucleation and Crystal Growth in Hybrid Halide Perovskite Thin Films, Front. Chem., 2022, 10, 842924, DOI:10.3389/fchem.2022.842924.
- Q. Liang, J. Liu and Y. Han, Optimizing film morphology and crystal orientation of perovskite for efficient planar-heterojunction solar cells by slowing crystallization process, Org. Electron., 2018, 62, 26–34, DOI:10.1016/j.orgel.2018.07.009.
- X. Meng,
et al., Crystallization Kinetics Modulation of FASnI 3 Films with Pre-nucleation Clusters for Efficient Lead-Free Perovskite Solar Cells, Angew. Chem., Int. Ed., 2021, 60(7), 3693–3698, DOI:10.1002/anie.202012280.
- S. Liu,
et al., A Review on Additives for Halide Perovskite Solar Cells, Adv. Energy Mater., 2020, 10(13), 1902492, DOI:10.1002/aenm.201902492.
- R. Zhuang,
et al., Effect of molecular configuration of additives on perovskite crystallization and hot carriers behavior in perovskite solar cells, Chem. Eng. J., 2023, 463, 142449, DOI:10.1016/j.cej.2023.142449.
- T. Li, Y. Pan, Z. Wang, Y. Xia, Y. Chen and W. Huang, Additive engineering for highly efficient organic–inorganic halide perovskite solar cells: recent advances and perspectives, J. Mater. Chem. A, 2017, 5(25), 12602–12652, 10.1039/C7TA01798G.
- C.-Y. Chang,
et al., Tuning Perovskite Morphology by Polymer Additive for High Efficiency Solar Cell, ACS Appl. Mater. Interfaces, 2015, 7(8), 4955–4961, DOI:10.1021/acsami.5b00052.
- G.-H. Kim and D. S. Kim, An intermediate phase stability for high performance of perovskite solar cells, Matter, 2021, 4(11), 3377–3378, DOI:10.1016/j.matt.2021.10.014.
- Z. Li, M. Yang, J.-S. Park, S.-H. Wei, J. J. Berry and K. Zhu, Stabilizing Perovskite Structures by Tuning Tolerance Factor: Formation of Formamidinium and Cesium Lead Iodide Solid-State Alloys, Chem. Mater., 2016, 28(1), 1, DOI:10.1021/acs.chemmater.5b04107.
- S. Abicho, B. Hailegnaw, G. A. Workneh and T. Yohannes, Role of additives and surface passivation on the performance of perovskite solar cells, Mater. Renew. Sustain. Energy, 2022, 11(1), 47–70, DOI:10.1007/s40243-021-00206-9.
- M.-J. Choi,
et al., Functional additives for high-performance inverted planar perovskite solar cells with exceeding 20% efficiency: Selective complexation of organic cations in precursors, Nano Energy, 2020, 71, 104639, DOI:10.1016/j.nanoen.2020.104639.
- J.-W. Lee, H.-S. Kim and N.-G. Park, Lewis Acid–Base Adduct Approach for High Efficiency Perovskite Solar Cells, Acc. Chem. Res., 2016, 49(2), 311–319, DOI:10.1021/acs.accounts.5b00440.
- Y. Li,
et al., Plant-Derived l-Theanine for Ultraviolet/Ozone Resistant Perovskite Photovoltaics, Adv. Energy Mater., 2023, 13(3), 2203190, DOI:10.1002/aenm.202203190.
- J. Liang,
et al., Origins and influences of metallic lead in perovskite solar cells, Joule, 2022, 6(4), 816–833, DOI:10.1016/j.joule.2022.03.005.
- D. Bai,
et al., Surface modulation for highly efficient and stable perovskite solar cells, RSC Adv., 2023, 13(40), 28097–28103, 10.1039/D3RA00809F.
- H. Zheng, G. Liu, W. Wu, H. Xu and X. Pan, Highly efficient and stable perovskite solar cells with strong hydrophobic barrier via introducing poly(vinylidene fluoride) additive, J. Energy Chem., 2021, 57, 593–600, DOI:10.1016/j.jechem.2020.09.026.
- Y. Miao, Y. Chen, H. Chen, X. Wang and Y. Zhao, Using steric hindrance to manipulate and stabilize metal halide perovskites for optoelectronics, Chem. Sci., 2021, 12(21), 7231–7247, 10.1039/D1SC01171E.
- J. Zhang,
et al., Exploring the Steric Hindrance of Alkylammonium Cations in the Structural Reconfiguration of Quasi-2D Perovskite Materials Using a High-throughput Experimental Platform, Adv. Funct. Mater., 2022, 32(43), 2207101, DOI:10.1002/adfm.202207101.
- Y. Ye, Y. Yin, Y. Chen, S. Li, L. Li and Y. Yamauchi, Metal–Organic Framework Materials in Perovskite Solar Cells: Recent Advancements and Perspectives, Small, 2023, 19(25), 2208119, DOI:10.1002/smll.202208119.
- R. K. Koech,
et al., Effects of polyethylene oxide particles on the photo-physical properties and stability of FA-rich perovskite solar cells, Sci. Rep., 2022, 12(1), 12860, DOI:10.1038/s41598-022-15923-y.
- C. Pereyra, H. Xie and M. Lira-Cantu, Additive engineering for stable halide perovskite solar cells, J. Energy Chem., 2021, 60, 599–634, DOI:10.1016/j.jechem.2021.01.037.
- Y. Sun, J. Zhang, H. Yu, C. Huang and J. Huang, Several Triazine-Based Small Molecules Assisted in the Preparation of High-Performance and Stable Perovskite Solar Cells by Trap Passivation and Heterojunction Engineering, ACS Appl. Mater. Interfaces, 2022, 14(5), 6625–6637, DOI:10.1021/acsami.1c21081.
- W. Zhao,
et al., A Special Additive Enables All Cations and Anions Passivation for Stable Perovskite Solar Cells with Efficiency over 23%, Nano-Micro Lett., 2021, 13(1), 169, DOI:10.1007/s40820-021-00688-2.
- J. Yang,
et al., A Review on Improving the Quality of Perovskite Films in Perovskite
Solar Cells via the Weak Forces Induced by Additives, Appl. Sci., 2019, 9(20), 4393, DOI:10.3390/app9204393.
- D. Sirbu, F. H. Balogun, R. L. Milot and P. Docampo, Layered Perovskites in Solar Cells: Structure, Optoelectronic Properties, and Device Design, Adv. Energy Mater., 2021, 11(24), 2003877, DOI:10.1002/aenm.202003877.
- W. Xiang, S. (Frank) Liu and W. Tress, Interfaces and Interfacial Layers in Inorganic Perovskite Solar Cells, Angew. Chem., Int. Ed., 2021, 60(51), 26440–26453, DOI:10.1002/anie.202108800.
- Y. Guo, H. Liu, W. Li, L. Zhu and H. Chen, Additive Engineering Toward High-Performance CsPbI3 Perovskite Solar Cells, Sol. RRL, 2020, 4(12), 2000380, DOI:10.1002/solr.202000380.
- N. Tripathi, Y. Shirai, M. Yanagida, A. Karen and K. Miyano, Novel Surface Passivation Technique for Low-Temperature Solution-Processed Perovskite PV Cells, ACS Appl. Mater. Interfaces, 2016, 8(7), 4644–4650, DOI:10.1021/acsami.5b11286.
- F. Wang,
et al., Modulating Crystallization and Defect Passivation by Butyrolactone Molecule for Perovskite Solar Cells, Molecules, 2023, 28(14), 5542, DOI:10.3390/molecules28145542.
- Y. Ahmed, B. Khan, M. Bilal Faheem, K. Huang, Y. Gao and J. Yang, Organic additives in all-inorganic perovskite solar cells and modules: from moisture endurance to enhanced efficiency and operational stability, J. Energy Chem., 2022, 67, 361–390, DOI:10.1016/j.jechem.2021.09.047.
- F. Zhang and K. Zhu, Additive Engineering for Efficient and Stable Perovskite Solar Cells, Adv. Energy Mater., 2020, 10(13), 13, DOI:10.1002/aenm.201902579.
- A. Mahapatra, D. Prochowicz, M. M. Tavakoli, S. Trivedi, P. Kumar and P. Yadav, A review of aspects of additive engineering in perovskite solar cells, J. Mater. Chem. A, 2020, 8(1), 1, 10.1039/C9TA07657C.
- A. Boufridi and R. J. Quinn, Harnessing the Properties of Natural Products, Annu. Rev. Pharmacol. Toxicol., 2018, 58(1), 451–470, DOI:10.1146/annurev-pharmtox-010716-105029.
- J. Xie,
et al., Identifying the functional groups effect on passivating perovskite solar cells, Sci. Bull., 2020, 65(20), 1726–1734, DOI:10.1016/j.scib.2020.05.031.
- K. Joyce, G. T. Fabra, Y. Bozkurt and A. Pandit, Bioactive potential of natural biomaterials: identification, retention and assessment of biological properties, Signal Transduction Targeted Ther., 2021, 6(1), 122, DOI:10.1038/s41392-021-00512-8.
- H. Zhu,
et al., Wood-Derived Materials for Green Electronics, Biological Devices, and Energy Applications, Chem. Rev., 2016, 116(16), 9305–9374, DOI:10.1021/acs.chemrev.6b00225.
- X. W. Sun, Y. X. Zhang and D. Losic, Diatom silica, an emerging biomaterial for energy conversion and storage, J. Mater. Chem. A, 2017, 5(19), 8847–8859, 10.1039/C7TA02045G.
-
Y. Ulusu, N. Eczacioglu and I. Gokce, Sustainable biomaterials for solar energy technologies, Sustainable Material Solutions for Solar Energy Technologies, Elsevier, 2021, pp. 557–592 DOI:10.1016/B978-0-12-821592-0.00019-4.
- Y. Zhang, Z. Wang and Y.-C. Chen, Biological tunable photonics: Emerging optoelectronic applications manipulated by living biomaterials, Prog. Quantum Electron., 2021, 80, 100361, DOI:10.1016/j.pquantelec.2021.100361.
- C. Zhang,
et al., Boosting the performance of MA-free inverted perovskite solar cells via multifunctional amino acid additives, J. Mater. Chem. C, 2023, 11(33), 11157–11166, 10.1039/D3TC01259J.
- B. Liu,
et al., Vitamin Natural Molecule Enabled Highly Efficient and Stable Planar n–p Homojunction Perovskite Solar Cells with Efficiency Exceeding 24.2%, Adv. Energy Mater., 2023, 13(2), 2203352, DOI:10.1002/aenm.202203352.
- L. Zhang,
et al., Crystallization control and multisite passivation of perovskites with amino acid to boost the efficiency and stability of perovskite solar cells, J. Mater. Chem. C, 2020, 8(48), 17482–17490, 10.1039/D0TC04186F.
- S. Dalwadi, A. Goel, C. Kapetanakis, D. Salas-de La Cruz and X. Hu, The Integration of Biopolymer-Based Materials for Energy Storage Applications: A Review, Int. J. Mol. Sci., 2023, 24(4), 3975, DOI:10.3390/ijms24043975.
- S. Bhandari, D. Mondal, S. K. Nataraj and R. G. Balakrishna, Biomolecule-derived quantum dots for sustainable optoelectronics, Nanoscale Adv., 2019, 1(3), 913–936, 10.1039/C8NA00332G.
- H. Yuan,
et al., Steric effect of amino-acids as additives for perovskite solar cells, J. Alloys Compd., 2021, 876, 160140, DOI:10.1016/j.jallcom.2021.160140.
- J. Hu,
et al., Overcoming photovoltage deficit via natural amino acid passivation for efficient perovskite solar cells and modules, J. Mater. Chem. A, 2021, 9(9), 5857–5865, 10.1039/D0TA12342K.
-
M. Abdullah, R. T. Jamil and F. N. Attia, Vitamin C (Ascorbic Acid), StatPearls, StatPearls Publishing, Treasure Island (FL), 2023. Accessed: Oct. 10, 2023. [Online]. Available: https://www.ncbi.nlm.nih.gov/books/NBK499877/ Search PubMed.
- X. Xu,
et al., Ascorbic acid as an effective antioxidant additive to enhance the efficiency and stability of Pb/Sn-based binary perovskite solar cells, Nano Energy, 2017, 34, 392–398, DOI:10.1016/j.nanoen.2017.02.040.
- H. Mohammadian-Sarcheshmeh, M. Mazloum-Ardakani, M. Rameez, S. Shahbazi and E. W.-G. Diau, Application of a natural antioxidant as an efficient strategy to decrease the oxidation in Sn-based perovskites, J. Alloys Compd., 2020, 846, 156351, DOI:10.1016/j.jallcom.2020.156351.
- Y. Chen,
et al., Application of Natural Molecules in Efficient and Stable Perovskite Solar Cells, Materials, 2023, 16(6), 2163, DOI:10.3390/ma16062163.
- R. J. Molyneux, S. T. Lee, D. R. Gardner, K. E. Panter and L. F. James, Phytochemicals: The good, the bad and the ugly?, Phytochemistry, 2007, 68(22–24), 2973–2985, DOI:10.1016/j.phytochem.2007.09.004.
- F. Rabizadeh, M. S. Mirian, R. Doosti, R. Kiani-Anbouhi and E. Eftekhari, Phytochemical Classification of Medicinal Plants Used in the Treatment of Kidney Disease Based on Traditional Persian Medicine, J. Evidence-Based Complementary Altern. Med., 2022, 2022, 1–13, DOI:10.1155/2022/8022599.
- A. Thakur, Health Promoting Phytochemicals in Vegetables: A Mini Review, Int. J. Food Ferment. Technol., 2018, 8(2), 2, DOI:10.30954/2277-9396.02.2018.1.
- A. Abdal Dayem,
et al., The Role of Reactive Oxygen Species (ROS) in the Biological Activities of Metallic Nanoparticles, Int. J. Mol. Sci., 2017, 18(1), 1, DOI:10.3390/ijms18010120.
- L. S. Berlim, A. G. Bezerra, W. M. Pazin, T. S. Ramin, W. H. Schreiner and A. S. Ito, Photophysical properties of flavonoids extracted from Syngonanthus nitens, the golden grass, J. Lumin., 2018, 194, 394–400, DOI:10.1016/j.jlumin.2017.10.040.
- K. Suwa, T. Suga, K. Oyaizu, H. Segawa and H. Nishide, Phenolic antioxidant-incorporated durable perovskite layers and their application for a solar cell, MRS Commun., 2020, 10(2), 312–316, DOI:10.1557/mrc.2020.25.
- J. Fu,
et al., Efficiency improvement of planar perovskite solar cells using a phenol additive, J. Mater. Chem. C, 2018, 6(43), 11519–11524, 10.1039/C8TC04525A.
- M. Hosseinnezhad,
et al., Dye-sensitized solar cells based on natural photosensitizers: A green view from Iran, J. Alloys Compd., 2020, 828, 154329, DOI:10.1016/j.jallcom.2020.154329.
- N. Y. Amogne, D. W. Ayele and Y. A. Tsigie, Recent advances in anthocyanin dyes extracted from plants for dye sensitized solar cell, Mater. Renew. Sustain. Energy, 2020, 9(4), 4, DOI:10.1007/s40243-020-00183-5.
- S. C. Ezike, C. N. Hyelnasinyi, M. A. Salawu, J. F. Wansah, A. N. Ossai and N. N. Agu, Synergestic effect of chlorophyll and anthocyanin Co-sensitizers in TiO2-based dye-sensitized solar cells, Surf. Interfaces, 2021, 22, 100882, DOI:10.1016/j.surfin.2020.100882.
- T. Rajaramanan,
et al., Natural
sensitizer extracted from Mussaenda erythrophylla for dye-sensitized solar cell, Sci. Rep., 2023, 13(1), 13844, DOI:10.1038/s41598-023-40437-6.
- Y. Kusumawati, A. S. Hutama, D. V. Wellia and R. Subagyo, Natural resources for dye-sensitized solar cells, Heliyon, 2021, 7(12), e08436, DOI:10.1016/j.heliyon.2021.e08436.
- E. González-Juárez,
et al., Enhancing the stability and efficiency of MAPbI3 perovskite solar cells by theophylline-BF4 − alkaloid derivatives, a theoretical-experimental approach, RSC Adv., 2023, 13(8), 5070–5080, 10.1039/D2RA07580F.
- X. Zhuang,
et al., Learning From Plants: Lycopene Additive Passivation toward Efficient and ‘Fresh’ Perovskite Solar Cells with Oxygen and Ultraviolet Resistance, Adv. Energy Mater., 2022, 12(25), 2200614, DOI:10.1002/aenm.202200614.
- C. O. Perera and G. M. Yen, Functional Properties of Carotenoids in Human Health, Int. J. Food Prop., 2007, 10(2), 201–230, DOI:10.1080/10942910601045271.
- A. Singh and T. Mukherjee, Application of carotenoids in sustainable energy and green electronics, Mater. Adv., 2022, 3(3), 1341–1358, 10.1039/D1MA01070K.
- P. Ebrahimi and A. Lante, Polyphenols: A Comprehensive Review of their Nutritional Properties, Open Biotechnol. J., 2021, 15(1), 164–172, DOI:10.2174/1874070702115010164.
- Y. Zhang, P. Cai, G. Cheng and Y. Zhang, A Brief Review of Phenolic Compounds Identified from Plants: Their Extraction, Analysis, and Biological Activity, Nat. Prod. Commun., 2022, 17(1), 1934578X2110697, DOI:10.1177/1934578X211069721.
-
D. Tsimogiannis and V. Oreopoulou, Classification of Phenolic Compounds in Plants, Polyphenols in Plants, Elsevier, 2019, pp. 263–284 DOI:10.1016/B978-0-12-813768-0.00026-8.
-
H. Al Mamari, Phenolic Compounds: Classification, Chemistry, and Updated Techniques of Analysis and Synthesis, in Biochemistry, ed. F. A. Badria, IntechOpen, 2022, vol. 26 DOI:10.5772/intechopen.98958.
- T. Wang,
et al., Highly Air-Stable Tin-Based Perovskite Solar Cells through Grain-Surface Protection by Gallic Acid, ACS Energy Lett., 2020, 5(6), 1741–1749, DOI:10.1021/acsenergylett.0c00526.
- H. Liu,
et al., Modulated Crystallization and Reduced VOC Deficit of Mixed Lead–Tin Perovskite Solar Cells with Antioxidant Caffeic Acid, ACS Energy Lett., 2021, 6(8), 2907–2916, DOI:10.1021/acsenergylett.1c01217.
- H. Liu, J. Dong, P. Wang, B. Shi, Y. Zhao and X. Zhang, Suppressing the Photoinduced Halide Segregation in Wide-Bandgap Perovskite Solar Cells by Strain Relaxation, Adv. Funct. Mater., 2023, 33(41), 2303673, DOI:10.1002/adfm.202303673.
- Y. Zhang, B. Zhao, L. Liu and N. Wang, Interfacial Molecular Lock Enables Highly Efficient Tin Perovskite Solar Cells, ACS Appl. Mater. Interfaces, 2023, acsami.3c10146, DOI:10.1021/acsami.3c10146.
- W.-F. Yang,
et al., Suppressed oxidation of tin perovskite by Catechin for eco-friendly indoor photovoltaics, Appl. Phys. Lett., 2021, 118(2), 023501, DOI:10.1063/5.0032951.
- C. Khaleel, N. Tabanca and G. Buchbauer, α-Terpineol, a natural monoterpene: A review of its biological properties, Open Chem., 2018, 16(1), 349–361, DOI:10.1515/chem-2018-0040.
- G. Giuliano, A. Bonasera, M. Scopelliti, D. Chillura Martino, T. Fiore and B. Pignataro, Boosting the Performance of One-Step Solution-Processed Perovskite Solar Cells Using a Natural Monoterpene Alcohol as a Green Solvent Additive, ACS Appl. Electron. Mater., 2021, 3(4), 4, DOI:10.1021/acsaelm.1c00084.
- S. Xiong,
et al., Defect passivation by nontoxic biomaterial yields 21% efficiency perovskite solar cells, J. Energy Chem., 2021, 55, 265–271, DOI:10.1016/j.jechem.2020.06.061.
-
P. Dey, et al., Analysis of alkaloids (indole alkaloids, isoquinoline alkaloids, tropane alkaloids), Recent Advances in Natural Products Analysis, Elsevier, 2020, pp. 505–567 DOI:10.1016/B978-0-12-816455-6.00015-9.
- H. E. Cortés-Ferré, D. Guajardo-Flores, G. Romero-De La Vega and J. A. Gutierrez-Uribe, Recovery of Capsaicinoids and Other Phytochemicals Involved With TRPV-1 Receptor to Re-valorize Chili Pepper Waste and Produce Nutraceuticals, Front. Sustain. Food Syst., 2021, 4, 588534, DOI:10.3389/fsufs.2020.588534.
- H.-W. Zhang,
et al., Improving Efficiency and Stability of p–i–n Perovskite Solar Cells by Capsaicin-Based Antisolvent Additive Engineering, Sol. RRL, 2023, 7(21), 2300511, DOI:10.1002/solr.202300511.
- G. Li,
et al., Excellent quinoline additive in perovskite toward to efficient and stable perovskite solar cells, J. Power Sources, 2021, 481, 228857, DOI:10.1016/j.jpowsour.2020.228857.
- R. Wang,
et al., Caffeine Improves the Performance and Thermal Stability of Perovskite Solar Cells, Joule, 2019, 3(6), 1464–1477, DOI:10.1016/j.joule.2019.04.005.
- R. Dhanabal, D. Kasinathan, A. Mahalingam, K. Madhuri, A. C. Bose and S. R. Dey, Caffeine additive based nanoarchitectonics of methylammonium lead iodide (MAPbI3) perovskite solar cell device: investigations on charge carrier properties using AC impedance spectroscopy, J. Mater. Sci. Mater. Electron., 2023, 34(33), 2205, DOI:10.1007/s10854-023-11569-2.
- R. Wang,
et al., Constructive molecular configurations for surface-defect passivation of perovskite photovoltaics, Science, 2019, 366(6472), 1509–1513, DOI:10.1126/science.aay9698.
- S. Liu,
et al., Boost the efficiency of nickel oxide-based formamidinium-cesium perovskite solar cells to 21% by using coumarin 343 dye as defect passivator, Nano Energy, 2022, 94, 106935, DOI:10.1016/j.nanoen.2022.106935.
- L. Xie,
et al., A Deformable Additive on Defects Passivation and Phase Segregation Inhibition Enables the Efficiency of Inverted Perovskite Solar Cells over 24%, Adv. Mater., 2023, 35(38), 2302752, DOI:10.1002/adma.202302752.
- Z. Ju, J. Sun and Y. Liu, Molecular Structures and Spectral Properties of Natural Indigo and Indirubin: Experimental and DFT Studies, Molecules, 2019, 24(21), 3831, DOI:10.3390/molecules24213831.
- J. Guo,
et al., Indigo: A Natural Molecular Passivator for Efficient Perovskite Solar Cells, Adv. Energy Mater., 2022, 12(22), 2200537, DOI:10.1002/aenm.202200537.
- Y. Deng, X. Li and R. Wang, Carboxyl functional group-assisted defects passivation strategy for efficient air-processed perovskite solar cells with excellent ambient stability, Sol. Energy Mater. Sol. Cells, 2021, 230, 111242, DOI:10.1016/j.solmat.2021.111242.
- F. Cai,
et al., Performance Improvement of Planar Perovskite Solar Cells Using Lauric Acid as Interfacial Modifier, ACS Appl. Energy Mater., 2022, 5(7), 8501–8509, DOI:10.1021/acsaem.2c01035.
- P. M. Hangoma,
et al., Improved Moisture Stability of Perovskite Solar Cells with a Surface-Treated PCBM Layer, Sol. RRL, 2019, 3(2), 1800289, DOI:10.1002/solr.201800289.
- G. Abdelmageed, H. R. Sully, S. Bonabi Naghadeh, A. El-Hag Ali, S. A. Carter and J. Z. Zhang, Improved Stability of Organometal Halide Perovskite Films and Solar Cells toward Humidity via Surface Passivation with Oleic Acid, ACS Appl. Energy Mater., 2018, 1(2), 387–392, DOI:10.1021/acsaem.7b00069.
- M. Adil Afroz,
et al., Thermal Stability and Performance Enhancement of Perovskite Solar Cells Through Oxalic Acid-Induced Perovskite Formation, ACS Appl. Energy Mater., 2020, 3(3), 2432–2439, DOI:10.1021/acsaem.9b02111.
- Y. Guo,
et al., Citric Acid Modulated Growth of Oriented Lead Perovskite Crystals for Efficient Solar Cells, J. Am. Chem. Soc., 2017, 139(28), 9598–9604, DOI:10.1021/jacs.7b03856.
- M. K. A. Mohammed,
et al., Stable Hole-Transporting Material-Free Perovskite Solar Cells with Efficiency Exceeding 14% via the Introduction of a Malonic Acid Additive for a Perovskite Precursor, Energy Fuels, 2022, 36(21), 13187–13194, DOI:10.1021/acs.energyfuels.2c02878.
- Y. Huang, S. Li, C. Wu, S. Wang, C. Wang and R. Ma, Interfacial modification by multifunctional octocrylene for high efficiency and stable planar perovskite solar cells, Chem. Commun., 2020, 56(49), 6731–6734, 10.1039/C9CC09075D.
|
This journal is © The Royal Society of Chemistry 2024 |