Preparation and evaluation of bioactive cellulose acetate films from Musa acuminata
Received
4th December 2023
, Accepted 21st June 2024
First published on 27th June 2024
Abstract
Natural biodegradable polymers can be utilized in place of synthetic materials used in food packaging, as they are more effective from an economic and environmental standpoint. Cellulose acetate, derived from the esterification of cellulose with acetic anhydride, possesses inherent properties that can be enhanced by incorporating essential oils, thereby strengthening its antioxidant and antimicrobial properties. In this study, cellulose acetate films were fabricated using extracts from Musa acuminata (banana) leaves, with varying concentrations (25%, 50%, and 75%) of tea tree oil. Efficient cellulose extraction from Musa acuminata leaves was achieved after a 30 min incubation period, with optimal cellulose acetate production obtained following a 30 min delignification process and subsequent 15 min bleaching treatment. Among the cellulose acetate films produced, designated as CAT1 (25% tea tree oil), CAT2 (50% tea tree oil), and CAT3 (75% tea tree oil), CAT1 exhibited reduced opacity while CAT3 displayed the lowest water uptake capacity. Notably, CAT3 demonstrated pronounced antibacterial and antifungal activities, along with substantial phenolic content and antioxidant potential, surpassing CAT2 and CAT1. The environmental sustainability of the cellulose acetate films was demonstrated by their notable biodegradability and significant swelling in water and chloroform solvents. Moreover, the films exhibited varying solubility in organic solvents. Remarkably, the developed films effectively extended the shelf life of fruits, resulting in significantly reduced weight loss and deterioration compared to both unpacked fruits and those wrapped in polyethylene packaging. These findings underscore the potential of cellulose acetate-based films as sustainable alternatives for food packaging, offering enhanced functionality while minimizing environmental impact.
Sustainability spotlight
The research emphasizes the sustainability potential of cellulose acetate-based films for food packaging. Through the utilization of natural, biodegradable polymers and integrating tea tree oil, we have developed films with improved antioxidant, antimicrobial, and biodegradable characteristics. The efficient extraction of cellulose from Musa acuminata leaves and the environmentally friendly fabrication process further contribute to the sustainability of our approach. These films significantly prolong the shelf life of fruits while minimizing environmental impact. These findings advocate for the use of cellulose acetate-based films as sustainable solutions for food packaging, ensuring functionality while prioritizing environmental responsibility.
|
1. Introduction
The ubiquity of petroleum-based plastics in everyday life, spanning consumer goods, industrial applications, and electronics, underscores their unparalleled utility. Despite their commendable attributes such as excellent processability, cost-effectiveness, and lightweight nature, the persistent challenge of non-biodegradability remains a pressing concern.1 Driven by the imperative to achieve carbon neutrality and curb the detrimental effects of plastic pollution, there is growing momentum behind initiatives aimed at advancing sustainable alternatives.2 The allure of renewable and biodegradable materials derived from biomass lies in their potential to serve as viable substitutes for non-biodegradable petrochemical plastics, embodying a shift towards eco-friendly and sustainable material choices.3 As the most abundant renewable and biodegradable biopolymer, cellulose is seen to be one of the most promising substitutes for plastic.4 While cellulose holds promise as a renewable and biodegradable alternative to fossil-based plastics, its thermo-processing performance is hindered by the presence of robust intermolecular hydrogen bond interactions. These interactions limit chain mobility, thereby posing challenges to the efficient conversion of cellulose into plastic materials.3 Cellulose acetate is produced through homogeneous or heterogeneous acetylation of the biopolymer cellulose. Diverse biomass sources, including cotton wastes,5 recycled paper,6 agricultural waste,7 and woods,8 are used in the generation of cellulose acetate. The cellulose acetate structure is significantly less crystalline and hence more soluble in common organic solvents than cellulose.9,10 Additionally, cellulose acetate is more easily processed than cellulose, and typical processing techniques include electrospinning, melt mixing, and solvent casting.11 The unique characteristics of cellulose acetate include extensive transparency, tensile strength, heat resistance, low water absorption, and ease of biodegradation. Thus, these attributes of cellulose acetate find profound utility in multiple sectors, including coating, films, fiber textiles, plastics, LCDs, photo films,12 packaging, membranes and cigarette manufacturing.13 The amount of acetyl and the degree of substitution have a significant impact on the quality of cellulose acetate as they may affect the final product and the solubility of cellulose acetate in solvent. Their acetyl content is influenced by several factors such as acetylation time, interaction between treatment factors and cellulose to anhydride acetic ratio.14 The cellulose acetate generation necessitates varying acetylation time depending on the cellulose source to obtain substantial acetyl concentration. The synthesis of cellulose acetate from nata de soya takes around 5–10 h, while from the pineapple waste requires about 2 h and straw needs 2–3 h.15
The potential of biopolymers derived from renewable resources to preserve and then release the incorporated active compounds is particularly a significant property that finds application in the packaging sector.16 The development of antimicrobial active packaging films using these biopolymers can extend the shelf-life of packaged products, meanwhile simultaneously encouraging the use of sustainable resources. The cellulose acetate polymer has undergone extensive research in preparation for application as an active packaging material.16 Active compounds like antioxidants and antimicrobials are introduced into the polymeric matrix of cellulose acetate, which could facilitate the shelf life through controlled release of the compounds during the storage.17 Due to their constant contact with the surface of packed materials, they also significantly contribute to minimizing food deterioration.18 Additionally, there is a rising trend in the food packaging sector to switch out synthetic additives for natural ones, including tocopherol, catechols, essential oils, and plant extracts, in both petrochemical-based and bio-based biodegradable polymers.19,20
Essential oils are substances obtained from plants that have a unique fragrance with even potential antimicrobial properties.21 They are volatile liquids with a high concentration of phenolic compounds that are produced by plants as secondary metabolites.22,23 Additionally, they are approved for contact with food-grade materials because they are regarded as “Generally Recognized as Safe” (GRAS) by the Food and Drug Administration (FDA).24 Different essential oils comprise distinctive compounds and their combination could give rise to potential synergistic effect that can facilitate an increased antimicrobial activity.25,26 Their antimicrobial strategy encompasses disruption of the enzyme system, interaction with membrane phospholipids and interference with the genetic material.27 Therefore, their use in food preservation is on the rise and they are swiftly replacing the synthetic alternatives. However, due to their potent flavoring ability, their direct use as food preservatives is limited. The incorporation of these substances into an appropriate polymer matrix, such as cellulose acetate, could reduce the sensory impact and facilitate a slow, sustained release of the active components.
Recognizing the imperative to mitigate the environmental impact of packaging materials, the current investigation endeavors to pioneer a natural polymeric film endowed with antimicrobial properties for food packaging applications. To accomplish this, cellulose extracted from Musa acuminata leaf samples underwent transformation into cellulose acetate serving as a sustainable foundation for the ensuing film formulation. Further, through incorporation of varying concentrations of tea tree oil, films are expected to offer robust protection against microbial contamination while extending the shelf life of packaged food products. Therefore, the study tries to harness the inherent properties of natural polymers and essential oil in order to develop films that could ultimately result in shift towards environmentally responsible packaging solutions with enhanced functionality and reduced environmental footprint.
2. Experimental
2.1 Materials
The Musa acuminate plant leaves samples were collected from the foothills of Western Ghats and transported to the laboratory in sterile bags for further processing. Acetic acid, acetic anhydride, concentrated sulphuric acid, hydrochloric acid, sodium hydroxide, 2′-azino-bis 3-ethylbenzothiazoline-6-sulfonic acid (ABTS, Cat. No. RM9270), 2,2-diphenyl-1-picrylhydrazyl (DPPH, Cat. No. MB263) solution and all the other analytical-grade reagents were supplied by HiMedia chemicals and used as received without further purification. Tea tree oil (Cat. No. W390208) was purchased from Sigma-Aldrich and gelatin (Cat. No. GRM019) was procured from HiMedia chemicals.
2.2 Extraction of cellulose
The extraction of cellulose from Musa acuminata samples was commenced through fine shredding of the leaves and thereafter subjecting to shade drying for 72 h at room temperature. Then, 10 g of shade dried leaves were added to 200 mL of 17.5% sodium hydroxide solution and boiled at 90 °C for varying durations (30 min, 60 min, 90 min, 120 min and 150 min). Following the alkaline treatment, the material underwent rigorous washing with deionized water until achieving neutral pH, ensuring the removal of residual alkali and other impurities. Subsequent treatment with a 10% hydrogen peroxide solution, under a controlled temperature of 80 °C, further facilitated the purification process. The varying durations of treatment (15, 30, 45, 60, and 75 min) allowed for optimization of the extraction process. The resulting cellulose was then carefully filtered to separate it from the solution, followed by thorough washing with deionized water to remove any residual chemicals. Finally, the purified cellulose was dried in an oven at 105 °C for 6 hours to achieve the desired low moisture content, ensuring its suitability for subsequent processing and applications.24
2.3 Production of cellulose acetate
The synthesis of cellulose acetate commenced by combining glacial acetic acid and cellulose at a ratio of 1
:
20 (w/v), initiating a controlled activation process of the cellulose substrate. Subsequently, the activated cellulose was subjected to reaction with acetic anhydride, with the addition of the sulfuric acid (H2SO4) catalyst to facilitate the acetylation reaction. Following the addition of acetic anhydride, the ratio of cellulose to acetic anhydride was adjusted to 1
:
10 ratio. The resulting solution underwent stirring for varying durations (30, 45, 60, 75, and 90 min) to initiate and control the acetylation process. Upon completion of the acetylation reaction, the addition of deionized water in a ratio of 5
:
1 (water
:
glacial acetic acid) served to terminate the acetylation process and initiate hydrolysis. The solution was stirred for an additional 30 min to ensure the cessation of acetylation. Subsequent to the termination of the reaction, the cellulose acetate product was carefully filtered to separate it from the solution and washed thoroughly with deionized water. Finally, the purified cellulose acetate was dried in an oven at 105 °C for 6 hours to remove residual moisture and ensure product stability.28
2.4 Preparation of cellulose acetate films
A cellulose acetate (CA) based membrane was meticulously prepared using the conventional casting evaporation technique, employing gelatin and glycerol as critical facilitators. Initially, a 0.6% (w/v) gelatin solution was hydrated in 10 mL of distilled water for 1 hour, followed by heating at 70 °C for 10 min to ensure complete dissolution. Subsequently, 4 mL of glycerol was carefully added to the solution and stirred slowly for 10 min to prevent the formation of bubbles. Following the preparation of the gelatin–glycerol solution, 1 g of cellulose acetate was dispersed in 25 mL of acetone and mixed with the gelatin–glycerol solution to form the filmogenic solution. Tea tree oils were then incorporated into the filmogenic solutions at final concentrations of 25%, 50%, and 75% (v/v), resulting in the development of four distinct films: cellulose acetate tea tree oil 1 (CAT 1, 25%), cellulose acetate tea tree oil 2 (CAT 2, 50%), cellulose acetate tea tree oil 3 (CAT 3, 75%), and cellulose acetate without tea tree oil (CA). Subsequently, each acetate film-forming solution was cast onto Petri dishes and allowed to dry at room temperature for 72 h. Following drying, the membranes were gently separated and rinsed with 20 mL of distilled water to remove any residual solvents or impurities. Finally, the membranes were affixed to clean glass supports using clamps and left to dry at room temperature for an additional 24 h, ensuring the formation of stable and uniform membranes suitable for subsequent characterization and application.
2.5 Characterization of cellulose acetate films
2.5.1 SEM analysis.
The surface morphology of the CA films and tea tree oil incorporated CA films was observed under a scanning electron microscope (FEI, model no.: Quanta 200) operated at a voltage of 5 kV. The samples were subsequently subjected to gold sputter coating in order to avoid sample charging and the emergence of contrasting structures.
2.5.2 Water uptake.
The film samples were placed in distilled water and incubated for 150 min in order to facilitate the equilibrium swelling condition. Then the membrane was filtered off, carefully anchored onto a filter paper and weighed to determine the amount of water uptake (%). The following equation was employed to obtain the water uptake percentage,
where M is the weight swelled sample weight and Mo is the weight of the dried sample.29
2.5.3 Opacity.
The films were trimmed into rectangles measuring 400 mm long and 100 mm wide, and the pieces were then affixed to the quartz cuvette of the spectrophotometer. The visible light absorption pattern for each sample was measured between 400 nm and 700 nm. The spectrum was calibrated employing a cuvette without any affixed sample as 100% transmittance. The area under the curve divided by the thickness of the film was used to determine the film's opacity and the same was expressed as absorbance units/nanometers (A nm−1).30
2.6
In vitro antimicrobial activity of the developed films
2.6.1 Antibacterial activity.
The antibacterial activities of CA films and tea tree oil incorporated CA films were determined according to previous reports. In brief, Staphylococcus aureus, Escherichia coli, Pseudomonas aeruginosa and Klebsiella pneumoniae were cultured overnight in Luria Bertani at 37 °C. Thereafter, 0.1 mL of the overnight cultures was added onto 10 mL of peptone medium and 50 mg of the films were added to the culture medium. The samples were incubated for 24 h at 37 °C and their optical density values were recorded at 620 nm. The inhibition percentage of the bacterial samples by the developed films was calculated with the following equation
where ODb and ODs correspond to the optical density of the bacterial cultures with and without the test films, respectively.31
2.6.2 Anti-fungal activity.
The yeast cells were cultured on Sabouraud Dextrose Agar (SDA) for 24–48 h at 35 °C and thereafter, the grown cells were suspended in 2 mL of sterile distilled water (SDW). This suspension density was set to McFarland standard tube 1, and it was serially diluted in SDW to a concentration of 2 × 103 CFU mL−1. Thereafter, 0.1 mL of the inoculum was plated onto SDA and the CA films were placed at the center, followed by incubation at 35 °C. The diameter of the fungal growth inhibition halo surrounding the film placed on the surface of the agar was measured to assess the culture growth. The inhibition percentage was calculated with the following equation
where ø total corresponds to the diameter of the Petri dish.32
2.7 Estimation of phenolic compounds in CA films
The total phenolic content of the developed CA films was determined by the method of Navajas et al., 2013.33 5 mL ethanol was added to the CA films weighing 50 mg to facilitate the phenolic compound extraction. Subsequently, 2 mL of 2.0 mL Folin–Ciocalteu reagent (10%, v/v) and 2 mL of sodium bicarbonate solution (7.5%; w/v) were added to 0.5 mL of the extract. The samples were incubated at 50 °C for 5 min and optical density values were measured at 700 nm. The standard curve was developed with gallic acid solution (10–100 μg) and the estimated phenolic content was expressed as microgram gallic acid equivalent per gram film (μg GAE per g film).
2.8 Antioxidant assay of the CA films
2.8.1 ABTS assay.
The free radical scavenging activities of the CA film and tea tree incorporated CA films were estimated by the ABTS radical cation decolonization assay. Concisely, ABTS (7 mM) was dissolved in deionized water to which potassium persulfate (2.45 mM) was added to facilitate the ABTS free radical production. The solution was kept in the dark for 12–16 h before use. The ABTS solution was diluted to an absorbance value of 0.7 OD at 700 nm. The CA films were extracted as given in Section 2.7, and 3 mL of the ABTS solution was added to 100 μL of the extract. The solution was incubated at 30 °C for 10 min and the absorbance values were recorded at 700 nm. The percentage of inhibition of ABTS radicals was calculated with the following equation
where Ab and Ae correspond to the absorbance values of the blank sample and film extract sample, respectively.34
2.8.2 DPPH assay.
The DPPH free radical scavenging activity of the films was also determined. 3 mL of DPPH solution was added to 100 μL of the CA film extracts and the mixture was incubated at 37 °C in a water bath for 20 min. The blank comprised 100 μL methanol and 3 mL of DPPH solution. The samples were then proceeded for absorbance measurement at 515 nm.35,36 The percentage inhibition of DPPH radicals was calculated with the equation given in Section 2.8.1.
2.9 Environmental compatibility assessment of the CA films
2.9.1 Biodegradability assessment of the CA films.
The generated CA films were cut into 100 × 150 mm in size in order to perform the biodegradation test. For all four samples (CA, CAT1, CAT2, and CAT3), the initial weight of the film was recorded. 10 cm of garden soil was added to four 500 mL beakers. Following their clamping between two nylon meshes, the film samples were buried at a depth of 5 cm within the corresponding beakers. Weekly samples were collected, and the attached debris was gently removed with deionized water. In order to assess the level of degradation, the weight loss of each sample was calculated by measuring consistent weight loss every 2 days for a period of 10 days.37
2.9.2 Swelling index.
The swelling studies were conducted employing three different solvent systems water, methanol and chloroform, separately. The samples (CA, CAT1, CAT1 and CAT3) were sliced into uniform pieces (2 × 2 cm2) and their initial weight was recorded. The pre-weighed samples were submerged into the solvent medium for about 2 h. The samples were then assessed for the weight gain at an interval of 20 min and the percentage gain was determined through pre weighed observation.38
where Fw and Iw are the weight of the swollen film and initial dry weight of the sample (g) respectively.
2.9.3 Solubility test.
The samples (CA, CAT1, CAT1 and CAT3) were cut into small pieces and placed in a test tube containing different solvents for a duration of 2 h. The solubility of these samples was then assessed wherein their soluble, partially soluble or insoluble nature was determined. The solvents employed include acetic acid, acetone, ammonia, chloroform, methanol, orthophosphoric acid, sulphuric acid, ethanol and water.39
2.10 Application of the CA films
A packaging bag (about 10 by 10 cm in size) was developed by adhering two sheets of cellulose film together. The same-sized plastic packaging bags were also made utilizing polyethylene wrap that was obtained commercially. The mango fruits were bought at a nearby fruit market, then washed under tap water for a duration of 1 min and allowed to air dry. Thereafter, the fruits were individually packed into the developed cellulose acetate films (CA, CA1, CA2 and CA3) as well as the polyethylene film bags and incubated at room temperature or in an incubator set at 11 °C. Unpacked fruits samples served as the control and each group consisted of 5 fruit samples. The color index to determine the process of fruit peel degreening was assessed using the equation
wherein the following scoring system was adopted: 0 point for all green; 1 point for a yellow fruit pedicel; 2 points for localized parts turning yellow; 3 points for most parts turning yellow; 4 points for all yellow.
The disease index was determined through assessing the total decayed area using the following equation
where the following scoring system was adopted: 0 point for no visible decay; 1 point for less than 1% decay spots; 2 points for less than 20% decay spots; 3 points for more than 20%, but less than 50% decay spots; 4 points for more than 50% decay spots.
The proportion of fruits that have reduced their total weight is known as the weight loss ratio. The total percentage of fruits that obtain a score of 1–4 is referred to as the “diseased fruit rate”.40
3. Results and discussion
3.1 Extraction of cellulose
The cellulose extraction was processed from the leaves of Musa acuminate plant through delignification followed by a bleaching process with variation in processing time. Delignification represents a critical step in the purification of cellulose, enabling the removal of undesirable components such as hemicellulose and lignin. The utilization of a sodium hydroxide concentration of 17.5% optimally facilitates the dissolution of these impurities, ensuring the retention of high cellulose content and purity. The delignification is followed by bleaching with hydrogen peroxide, wherein the remaining impurities are dissolved and removed. The obtained result substantiates that the yield is maximum within 30 min treatment for delignification and 15 min for bleaching, beyond which the yield was observed to remain constant as shown in Fig. 1a and B. The extracted cellulose samples are displayed in Fig. 1d.
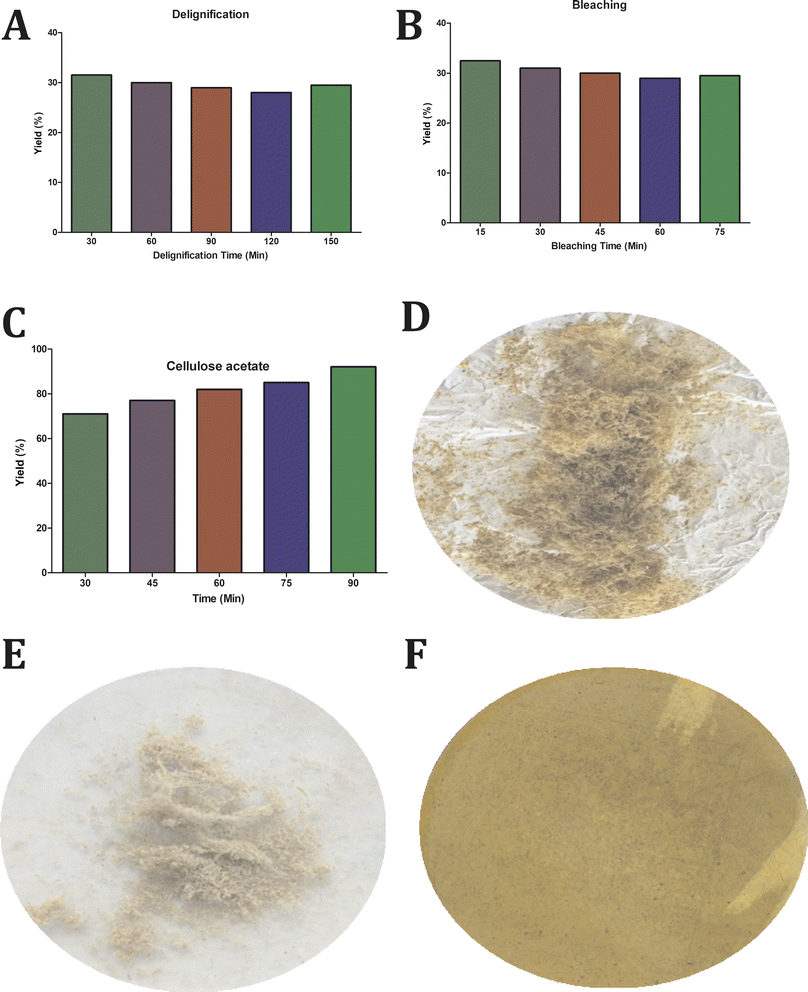 |
| Fig. 1 Cellulose Acetate Film preparation. (A) Incubation duration of Musa acuminata leaf extract for delignification, (B) incubation duration of the delignified sample for bleaching, (C) incubation duration for acetylation of the extracted cellulose, (D) extracted cellulose sample from Musa acuminata, (E) acetylated cellulose sample and (F) cellulose acetate film. | |
3.2 Preparation of cellulose acetate
Cellulose acetate was thereafter prepared from the purified cellulose obtained from Musa acuminata plant leaves through acetylation with glacial acetic acid. Glacial acetic acid activates cellulose, inflating its structure to increase the surface area while reducing intermolecular hydrogen bonds. Consequentially, the cellulose reacted rapidly and established a bond with the acetyl groups. Acetyl sulfate, produced by the catalytic reaction between acetic anhydride and sulfuric acid, combines with cellulose to produce cellulose acetate. The color of the solution shifts from clear brown to turbid white during the hydrolysis process, which is directly associated with the formation of cellulose acetate. The processing time of 90 min produced the highest yield of cellulose acetate at 92% as established in Fig. 1c and the acetylated sample is shown in Fig. 1e. The prepared cellulose acetate film is exhibited in Fig. 1f.
3.3 Characterization of cellulose acetate films
3.3.1 SEM analysis.
The morphology of cellulose acetate films and the corresponding oil incorporated films were investigated under scanning electron microscopy (Fig. 2). The cellulose acetate film exhibited highly fused spherical structures uniformly dispersed through the surface (Fig. 2a), whereas the oil incorporated films revealed considerably more fine structures distributed uniformly throughout the surface (Fig. 2b).
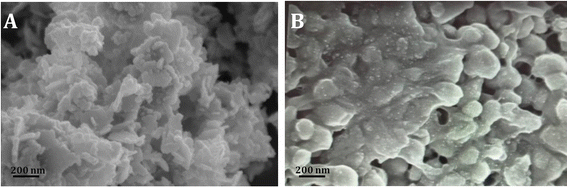 |
| Fig. 2 Scanning electron microscopy of cellulose acetate films. (A) Cellulose acetate film and (B) tea tree oil incorporated cellulose acetate film. | |
3.3.2 Water uptake.
The water content uptake of the films was found to decrease upon incorporation of the tea tree oil. The results depicted in Fig. 3a reveal that as the percentage of tea tree oil increases, the water uptake capacity begins to decline. This is substantiated by the water uptake percentage of 6% for CAT3, whereas those of CAT2 and CAT1 are 19% and 25%, respectively. The cellulose acetate film without tea tree oil showed water uptake capability of about 29%. This could be ascertained to the fact that the oil incorporation leads to an increased hydrophobicity of the films, thereby decreasing their inherent capacity to take up water.
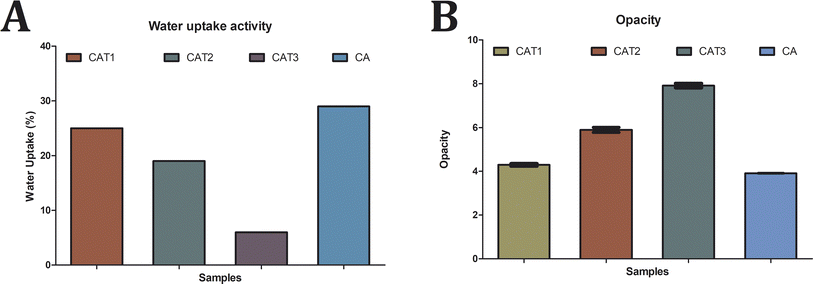 |
| Fig. 3 Characterization of cellulose acetate films. (A) Water uptake activity of the cellulose acetate films and (B) opacity analysis of the cellulose acetate films. | |
In addition, water molecules should disperse mostly through the uninterrupted polymeric phase, but the existence of EO droplets acts as discontinuities that cause an efficient, increase in the tortuosity parameter for water transfer in the matrix and so hydrophobicity increases. This effect relies on various factors, such as the polymer kind, the amount and composition of the oil added and the interactions between the oil and the matrix.29
3.3.3 Opacity.
The opacity values for the cellulose acetate films and the oil encompassing films are shown in Fig. 3b. The CAT1 tea tree oil incorporated samples exhibited opacity values closer to the control films with corresponding values of 4.29 and 3.91, respectively. However, the CAT2 and CAT3 tea tree oil incorporated films revealed considerable opacity of 5.89 and 7.91, respectively. Opacity contributes significantly to the films that are considered for food packaging. The physical appearance of packaged food products can be influenced by their transparency, which also impacts the extent to which they get accepted by consumers. The transparency of the films is significantly influenced by the presence of additional substances that are incorporated. This could be ascertained to the differences in refractive indices between concentration, size and phases of the dispersed particles.41 As an alternative, this could possibly have been affected by the interaction of the molecules of cellulose acetate with tea tree oil, which potentially reduced the amount of light that can transit through the film.42,43
3.4
In vitro antimicrobial activity of the developed films
3.4.1 Antibacterial activity.
The antibacterial activity of the developed films was assessed to evaluate their efficacy in inhibiting pathogenic organisms. Our results demonstrated that the tea tree oil-incorporated films exhibited significantly higher activity against Escherichia coli, Pseudomonas aeruginosa, and Klebsiella pneumoniae compared to Staphylococcus aureus. Furthermore, the study revealed a dose-dependent increase in antibacterial activity with increasing concentrations of tea tree oil (Fig. 4a). Conversely, the cellulose acetate film without tea tree oil showed no significant antibacterial activity. The enhanced antibacterial activity of the tea tree oil-incorporated films can be attributed to the presence of phenolic compounds in the oil. These bioactive compounds have been shown to interact with bacterial cell membranes, ultimately inhibiting bacterial growth.36 The antibacterial activity observed in our study aligns with previous findings, confirming the potent activity of tea tree oil against different bacterial pathogens.44–46
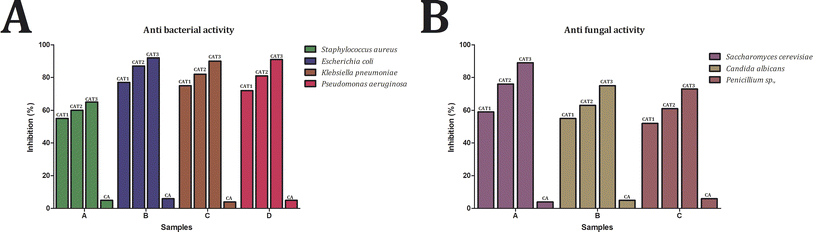 |
| Fig. 4 Antimicrobial activity of cellulose acetate films. (A) Antibacterial activity of the cellulose acetate films and (B) antifungal activity of the cellulose acetate films. | |
3.4.2 Anti-fungal activity.
The cellulose acetate films incorporated with different concentrations of tea tree oil (CAT1, CAT2, and CAT3) demonstrated significant antifungal activity, as evidenced by the considerable inhibition percentages observed. In contrast, cellulose acetate films without tea tree oil showed negligible inhibition, as illustrated in Fig. 4b. The efficacy of the films varied depending on the fungal species tested, with Saccharomyces cerevisiae exhibiting the highest sensitivity, followed by Candida albicans and Penicillium sp. The incorporation of tea tree oil into the films resulted in a notable enhancement of the inhibition percentages, effectively delaying the onset of fungal growth.
After seven days of incubation, cellulose acetate tea tree oil 3 (CAT3) demonstrated remarkable antifungal activity, with inhibition percentages of 89% against Saccharomyces cerevisiae, 75% against Candida albicans, and 73% against Penicillium sp. Similarly, cellulose acetate tea tree oil 2 (CAT2) exhibited substantial inhibition, with percentages of 76%, 63%, and 61% against Saccharomyces cerevisiae, Candida albicans, and Penicillium sp., respectively. In comparison, cellulose acetate tea tree oil 1 (CAT1) showed slightly lower inhibition percentages, with values of 59% against Saccharomyces cerevisiae, 55% against Candida albicans, and 52% against Penicillium sp. These findings underscore the dose-dependent antifungal efficacy of tea tree oil-incorporated cellulose acetate films, with higher concentrations resulting in greater inhibition of fungal growth. The antifungal activity demonstrated by our tea tree oil-incorporated films is consistent with previous research, highlighting the effectiveness of tea tree oil against a range of fungal pathogens.47–49
3.5 Estimation of phenolic compounds in CA films
The CA film extracts demonstrated an increased total phenolic content with higher tea oil concentration determined through the Folin–Ciocalteu method as shown in Fig. 5a. This reveals that soaking of the generated films in ethanol for the phenolic compound extraction resulted in expansion of their structural integrity and liberation of the oil content. The CAT1 extracts showed a phenolic content of 2485.52 μg GAE per g of the sample, while those of CAT2 and CAT3 were 4340.31 μg GAE per g and 6827.51 μg GAE per g, respectively. The CA film without oil incorporation exhibited the total phenolic content of 138.65 μg GAE per g, which is substantially very low in comparison with the tea tree oil-incorporated films. Our results indicate that the incorporation of tea tree oil into cellulose acetate films significantly enhances their phenolic content. This observation is consistent with prior research and highlights the potential of tea tree oil-incorporated cellulose acetate films as an effective carrier of phenolic compounds.50 These findings underscore the versatility of tea tree oil-incorporated cellulose acetate films, which offers a promising platform for the controlled delivery of phenolic compounds. The presence of phenolic groups contributes towards their antioxidant potential.51,52 This could be attributed to the characteristic structure of the phenolic compounds that facilitates them to act as antioxidants and scavenge free radicals.53 The presence of monoterpenoid ketones and oxygenated monoterpenes in the oil has significant antioxidant potential,54,55 having significant implications for various applications in food packaging and other industries.
 |
| Fig. 5 Estimation of Phenolic content and antioxidant potential. (A) Total phenolic content determination of the cellulose acetate films, (B) antioxidant potential of cellulose acetate films by the ABTS assay and (C) antioxidant potential of cellulose acetate films by the DPPH assay. | |
3.6 Antioxidant assay of the CA films
3.6.1 ABTS assay.
The ABTS cation scavenging activity assay that primarily assesses water soluble antioxidants with the single electron transfer mechanism was measured for the tea tree oil comprising CA films and is displayed in Fig. 5b. The CAT 1 films exhibited 44.93% inhibition, while CAT2 and CAT3 exhibited 67.9% and 84.28% inhibition, respectively. We observed a proportional increase in the percentage of inhibition with higher concentrations of tea tree oil, with cellulose acetate tea tree oil 3 (CAT3) exhibiting the highest antioxidant activity, followed by CAT2 and CAT1. The CA films without tea tree oil produced 13.77% inhibition, which could be due to the presence of polysaccharide hydroxy groups. These results corroborate previous studies, further substantiating the role of tea tree oil concentration as a key determinant of the antioxidant activity exhibited by cellulose acetate films.56
3.6.2 DPPH assay.
The DPPH assay of the tea tree oil incorporated CA films exhibited an activity of 40.55% for CAT1, 61.08% for CAT2 and 80.61% for CAT3 as shown in Fig. 5c. The CA films without oil incorporation produced 10.58% inhibition. The color of the diphenyl-picrylhydrazine radical changed from violet to yellow upon acceptance of free radicals from the antioxidants released from the CA films.57 The observed correlation between tea tree oil concentration and antioxidant activity aligns with the existing literature56,58 and further emphasizes the importance of oil concentration for development of films with enhanced properties.
3.7 Environmental compatibility assessment of the CA films
3.7.1 Biodegradability assessment of the CA films.
The changes have been discernible since the first day of the experiment, suggesting that the biodegradation of CA films is happening concurrently. During the initial stages of degradation, there was minimal mass loss of cellulose acetate films; however, as the degradation progressed, the mass loss rate increased. Over the course of the period, the compactness of CA films has decreased, resulting in an overall loss of thickness and form that has driven the films to shrink in size. The parameters thus assessed to signify these changes were the loss of weight as well as size of the films that were allowed to decompose. The results provided in Table 1 depict the successive loss of weight and size of these films over a period of 10 days. The data obtained demonstrate that substantial degradation was recorded on the fourth and sixth day of incubation, with all samples exhibiting a loss ranging from 0.110 g to 0.160 g. Simultaneously, a 60 mm decline in size had been observed in each sample during the same time period.
Table 1 Weight and size reduction analysis of the CA films
S. No. |
Sample |
0th day |
2nd day |
4th day |
6th day |
8th day |
10th day |
(g) |
(mm) |
(g) |
(mm) |
(g) |
(mm) |
(g) |
(mm) |
(g) |
(mm) |
(g) |
(mm) |
1 |
CA |
0.331 |
200 |
0.291 |
150 |
0.189 |
106 |
0.081 |
41 |
0.021 |
21 |
0.003 |
1 |
2 |
CAT1 |
0.359 |
200 |
0.312 |
159 |
0.213 |
111 |
0.091 |
49 |
0.032 |
26 |
0.005 |
1 |
3 |
CAT2 |
0.374 |
200 |
0.339 |
161 |
0.221 |
115 |
0.093 |
51 |
0.039 |
31 |
0.006 |
1 |
4 |
CAT3 |
0.388 |
200 |
0.341 |
169 |
0.235 |
118 |
0.098 |
58 |
0.042 |
34 |
0.009 |
2 |
It is an established fact that even under ideal laboratory exposure conditions, barely 0.1% of the carbon in the PE polymer is transformed into CO2 annually by biodegradation. The conditions in the natural environment are not ideal for the breakdown of polymers, and thus the degradation processes occur even more slowly.59 As technology advances, it becomes apparent that the most effective packaging materials must have both durability to keep the contents intact and biodegradability, which allows the components to break down or decompose after utilization. In the interim, it shouldn't contaminate or pollute the air, water, or soil. It is interesting to note that the present attempts to develop an ideal packing material have demonstrated good ecological sustainability.
3.7.2 Swelling test.
The swelling percentage study revealed that samples treated with water and chloroform have shown a highest percentage of 51% and 53%, respectively for CAT3 and the lowest of 48% and 50% respectively for CA. Moreover, the swelling percentages were found to have reached a saturation point from the 80 min incubation duration. This was observed to be the same case with the swelling index of samples treated with methanol, however, an increased value was observed for CA (38%), followed by CAT1 (35%), CAT2 (34%) and CAT3 (33%). The results are shown in Fig. 6. The film's swelling activity indicates the extent to which it can absorb liquids, such as water. This is important because it establishes the film's capacity to hold moisture from the food item or its surroundings. Films with high water absorption capacity can help maintain the moisture content of perishable foods, thereby extending their shelf life. While films used for food preservation should have the ability to absorb water to some extent, they should also possess adequate moisture barrier properties to prevent excessive moisture ingress.
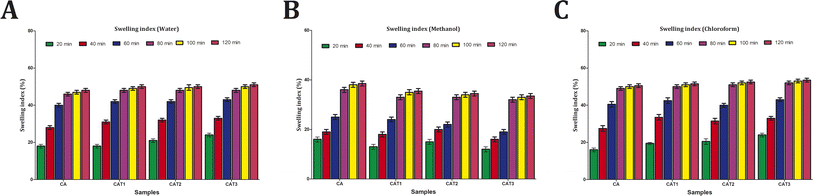 |
| Fig. 6 Swelling index profile of cellulose acetate films. (A) Swelling index of films in water, (B) swelling index of films in methanol and (C) swelling index of films in chloroform. | |
3.7.3 Solubility test.
Another crucial factor to take into account when applying films for food preservation is their ability to dissolve in various solvents. The film samples CA, CAT1, CAT2 and CAT3 were found to be partially soluble in water and ethanol. Acetic acid, chloroform, and orthophosphoric acid displayed partial solubility for CAT2 and CAT3, whereas complete solubility for CAT1 and CA. Alternatively, the solvents acetone and methanol exhibited partial solubility for CAT1, CAT2 and CAT3 and complete solubility for CA. The solvents ammonia and sulphuric acid showed partial solubility only for CAT3, whereas complete solubility for CA, CAT1 and CAT2. The data for the solubility test are provided in Table 2. Food preservation films should not be very soluble in fatty or watery food matrices since this could cause the components of the film to diffuse into the food product. Soluble film components may impart off-flavors, odors, or undesirable characteristics to the food, compromising its quality and safety.
Table 2 Solubility analysis of the CA filmsa
Solvent |
Sample |
Insoluble |
Partially soluble |
Completely soluble |
− Negative, + positive.
|
Acetic acid |
CA |
− |
− |
+ |
CA1 |
− |
− |
+ |
CA2 |
− |
+ |
− |
CA3 |
− |
+ |
− |
Acetone |
CA |
− |
− |
+ |
CA1 |
− |
+ |
− |
CA2 |
− |
+ |
− |
CA3 |
− |
+ |
− |
Ammonia |
CA |
− |
− |
+ |
CA1 |
− |
− |
+ |
CA2 |
− |
− |
+ |
CA3 |
− |
+ |
− |
Chloroform |
CA |
− |
− |
+ |
CA1 |
− |
− |
+ |
CA2 |
− |
+ |
− |
CA3 |
− |
+ |
− |
Methanol |
CA |
− |
− |
+ |
CA1 |
− |
+ |
− |
CA2 |
− |
+ |
− |
CA3 |
− |
+ |
− |
Orthophosphoric acid |
CA |
− |
− |
+ |
CA1 |
− |
− |
+ |
CA2 |
− |
+ |
− |
CA3 |
− |
+ |
− |
Sulfuric acid |
CA |
− |
− |
+ |
CA1 |
− |
− |
+ |
CA2 |
− |
− |
+ |
CA3 |
− |
+ |
− |
Ethanol |
CA |
− |
+ |
− |
CA1 |
− |
+ |
− |
CA2 |
− |
+ |
− |
CA3 |
− |
+ |
− |
Water |
CA |
− |
+ |
− |
CA1 |
− |
+ |
− |
CA2 |
− |
+ |
− |
CA3 |
− |
+ |
− |
3.8 Application of the CA films
The investigation of mango fruit preservation demonstrated that cellulose acetate films were far superior to polyethylene ones both at room temperature as well as during refrigeration (Fig. 7). Among the samples held in cold conditions, polyethylene and no packaging showed nearly comparable values in the disease index analysis, however the samples without packaging displayed the highest value at room temperature. The cellulose acetate films have been found to exhibit relatively low disease index values, with CAT3 possessing the lowest value and CAT2, CAT1, and CA following in order. In comparison to the samples kept in cold storage, it was determined that the samples kept at room temperature had elevated disease index values.
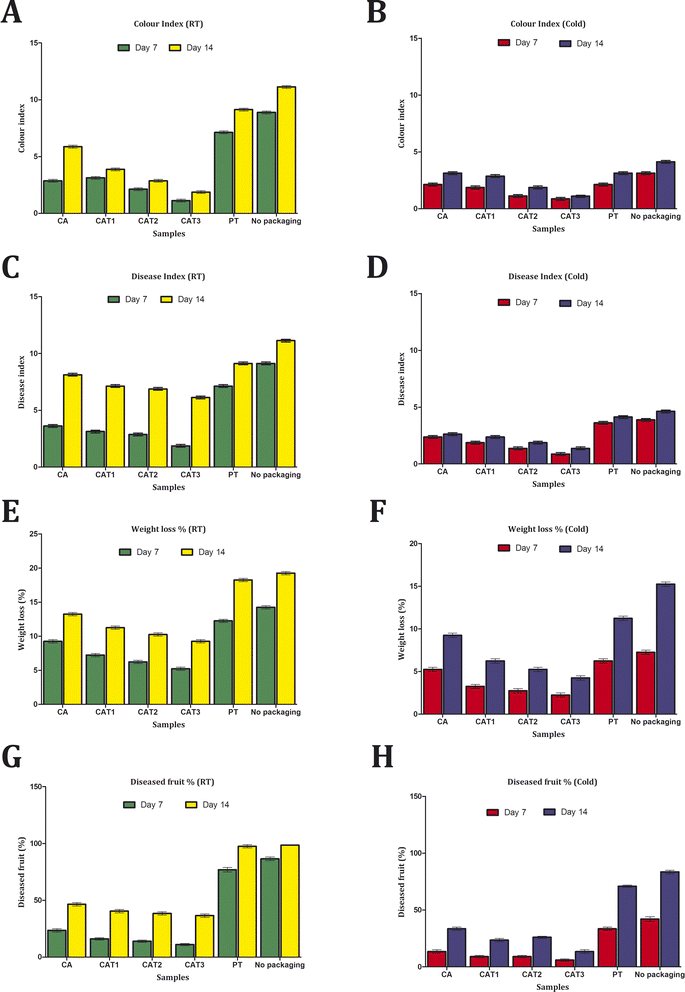 |
| Fig. 7 Application of cellulose acetate films in fruit preservation. (A) Color index of the films at room temperature, (B) color index of the films under refrigeration, (C) disease index of the films at room temperature, (D) disease index of the films under refrigeration, (E) weight loss percentage of the films at room temperature, (F) weight loss percentage of the films under refrigeration, (G) diseased fruit percentage at room temperature and (H) diseased fruit percentage under refrigeration. | |
The fruits packed in cellulose acetate films had a reduced rate of yellowing, according to the color index study. Despite not meeting the higher color index values displayed by the unpacked samples, the fruits packed in polyethylene demonstrated a notably elevated rate of yellowing. According to the disease fruit investigation, fruits that were unpacked and wrapped in polyethylene exhibited 100% deterioration, while fruits packed in cellulose acetate films showed less than 50% loss at room temperature. In comparison to the above instance, the cold storage samples wrapped in cellulose acetate films exhibited an even lower percentage of diseased fruit level. Concurrently, the weight loss percentage analysis showed that the cellulose acetate films continued to have substantially reduced levels while the unpacked and polyethylene-wrapped samples had higher measurements. It can be concluded, then, that preventing the intensity of respiration by lowering the temperature can postpone the onset of disease and the ripening of fruit. Nevertheless, cellulose acetate film wrapping can help to increase the fruit shelf life rate and lengthen the preservation term under room temperature storage circumstances, when cold storage technology is scarce.
4. Conclusion
The successful production and characterization of Musa acuminata derived cellulose acetate films have been accomplished. The presence of phenolic compounds and exhibition of substantial antimicrobial and antioxidant activity by the generated films suggest towards their potential application in the food packaging sector. Besides boosting food safety and maintaining its quality, active packaging based on cellulose acetate incorporated with essential oil reduces the environmental impact of traditional packaging since it is made from renewable resources. As a result, synthetic chemical preservatives directly incorporated into foods can be replaced with such antimicrobial active packaging.
Author contributions
Achuth Jayakrishnan & Shirin Shanana designed the work. Achuth Jayakrishnan drafted the original manuscript. Reshma Ayswaria and Achuth Jayakrishnan proof read the manuscript.
Conflicts of interest
The authors declare no conflict of interest.
Acknowledgements
The authors would like to express their sincerest gratitude to the Institutions, Hindusthan College of Arts & Science, Coimbatore and Mercy College, Palakkad for providing all the resources to carry out the work. The authors are thankful to the technicians from Hindusthan College of Arts & Science, Coimbatore and Mercy College, Palakkad for their consistent support in execution of the experiments.
References
- D.-H. Li, Z. Han, H. Qian, K. Yang, W. Sun, H.-C. Liu, Y. Zhao, Z.-X. Liu, C.-N.-Y. Zong, H. Yang, Q. Guan and S. Yu, Adv. Mater., 2023, 35(1), 2208098 CrossRef CAS PubMed.
- A. Barhoum, J. Jeevanandam, A. Rastogi, P. Samyn, Y. Boluk, A. Dufresne, M. K. Danquah and M. Bechelany, Nanoscale, 2020, 12, 22845–22890 RSC.
- Q. Xia, C. Chen, Y. Yao, J. Li, S. He, Y. Zhou, T. Li, X. Pan, Y. Yao and L. Hu, Nat. Sustain., 2021, 4, 627–635 CrossRef.
- Z. Su, Y. Liu, L. Cui, G. Zhou, X. Zhang, X. Qiu, C. Chen and X. Wang, ACS Nano, 2023, 17, 21420–21431 CrossRef PubMed.
- H. N. Cheng, M. K. Dowd, G. W. Selling and A. Biswas, Carbohydr. Polym., 2010, 80, 449–452 CrossRef CAS.
- G. R. Filho, D. S. Monteiro, C. Da Silva Meireles, R. M. N. De Assunção, D. A. Cerqueira, H. S. Barud, S. J. L. Ribeiro and Y. Messadeq, Carbohydr. Polym., 2008, 73, 74–82 CrossRef.
- A. U. Israel, I. B. Obot, S. A. Umoren, V. Mkpenie and J. E. Asuquo, E-J. Chem., 2008, 5, 81–85 CrossRef.
- H. Sato, Y. Uraki, T. Kishimoto and Y. Sano, Cellulose, 2003, 10, 397–404 CrossRef CAS.
- S. Fischer, K. Thümmler, B. Volkert, K. Hettrich, I. Schmidt and K. Fischer, Macromol. Symp., 2008, 262, 89–96 CrossRef CAS.
- J. A. Á. Ramírez, C. J. Suriano, P. Cerrutti and M. L. Foresti, Carbohydr. Polym., 2014, 114, 416–423 CrossRef PubMed.
- F. Rodríguez, M. J. Galotto, A. Guarda and J. E. Bruna, Sustainable Dev. Biodiversity, 2016, 219–234 Search PubMed.
- J. Puls, S. A. Wilson and D. Hölter, J. Polym. Environ., 2010, 19, 152–165 CrossRef.
- B. Hinterstoisser, M. Åkerholm and L. Salmén, Biomacromolecules, 2003, 4, 1232–1237 CrossRef CAS PubMed.
-
Susanti, Effect of anhydrous Acetate Ratio and Time Acetylation against Characteristics of Cellulose Acetate on the Production Process of Cellulose Acetate Membranes, MSc thesis, Department of Chemistry, Bogor Agricultural University, 2003.
-
Safriani, Production of Cellulose Acetate Biopolymer from Nata De Soya, MSc thesis, Graduate School of Bogor Agricultural University, 2000.
- D. Su and M. S. Chinnan, Crit. Rev. Food Sci. Nutr., 2004, 44, 223–237 CrossRef PubMed.
- C. L. De Dicastillo, R. Navarro, A. Guarda and M. J. Galotto, Antioxidants, 2015, 4, 533–547 CrossRef CAS PubMed.
- J. Gómez-Estaca, C. López-De-Dicastillo, P. Hernández-Muñoz, R. Catalá and R. Gavara, Trends Food Sci. Technol., 2014, 35, 42–51 CrossRef.
- V. Del-Valle, P. Hernández-Muñoz, A. Guarda and M. J. Galotto, Food Chem., 2005, 91, 751–756 CrossRef CAS.
- M. P. Arrieta, V. Sessini and L. Peponi, Eur. Polym. J., 2017, 94, 111–124 CrossRef CAS.
- L. Peponi, V. Sessini, M. P. Arrieta, I. Navarro-Baena, Á. Sonseca, F. Dominici, E. Giménez, L. Torre, A. Tercjak, D. López and J. M. Kenny, Polym. Degrad. Stab., 2018, 151, 36–51 CrossRef CAS.
- P. N. Kaul, A. K. Bhattacharya, B. R. S. Rao, K. V. Syamasundar and S. Ramesh, J. Sci. Food Agric., 2002, 83, 53–55 CrossRef.
- A. Rodríguez, R. Batlle and C. Nerín, Prog. Org. Coat., 2007, 60, 33–38 CrossRef.
- S. A. Burt, Int. J. Food Microbiol., 2004, 94, 223–253 CrossRef CAS PubMed.
- C. S. Romano, K. Abadi, V. Repetto, A. A. Vojnov and S. Moreno, Food Chem., 2009, 115, 456–461 CrossRef CAS.
- A. Y. Gibriel, H. M. A. Al-Sayed, A. H. Rady and M. A. Abdelaleem, J. Food Saf., 2013, 33, 222–228 CrossRef.
- S. A. Burt, R. Van Der Zee, A. P. Koets, A. M. De Graaff, F. Van Knapen, W. Gaastra, H. P. Haagsman and E. J. A. Veldhuizen, Appl. Environ. Microbiol., 2007, 73, 4484–4490 CrossRef CAS PubMed.
- D. Tristantini and C. Sandra, E3S Web Conf., 2018, 67, 04035 CrossRef CAS.
- G. F. E. Fawal, A. M. Omer and T. M. Tamer, J. Food Sci. Technol., 2019, 56, 1510–1518 CrossRef PubMed.
- N. Gontard, S. Guilbert and J.-L. Cuq, J. Food Sci., 1992, 57, 190–195 CrossRef CAS.
- M. J. Moreno-Vásquez, E. L. Valenzuela-Buitimea, M. Plascencia-Jatomea, J. C. Encinas, F. Rodríguez-Félix, S. Sánchez-Valdés, E. C. Rosas-Burgos, V. M. Ocaño-Higuera and A. Z. Graciano-Verdugo, Carbohydr. Polym., 2017, 155, 117–127 CrossRef PubMed.
- M. P. Balaguer, P. Fajardo, H. Gartner, J. Gómez-Estaca, R. Gavara, E. Almenar and P. Hernández-Muñoz, Int. J. Food Microbiol., 2014, 173, 62–71 CrossRef CAS PubMed.
- Y. Ruiz-Navajas, M. Viuda-Martos, E. Sendra and J. Fernández-López, Food Control, 2013, 30, 386–392 CrossRef CAS.
- R. Re, N. Pellegrini, A. R. Proteggente, A. S. Pannala, M. Yang and C. Rice-Evans, Free Radicals Biol. Med., 1999, 26, 1231–1237 CrossRef CAS PubMed.
- W. Brand-Williams, M.-E. Cuvelier and C. Berset, LWT-Food Sci. Technol., 1995, 28, 25–30 CrossRef CAS.
- G. Miliauskas, P. R. Venskutonis and T. A. Van Beek, Food Chem., 2004, 85, 231–237 CrossRef CAS.
- K. A. Zahan, N. M. Azizul, M. Mustapha, W. Y. Tong, M. S. A. Rahman and I. S. Sahuri, Mater. Today: Proc., 2020, 31, 83–88 CAS.
- T. Gasti, V. D. Hiremani, S. Sataraddi, V. N. Vanjeri, N. Goudar, S. P. Masti, R. B. Chougale and R. B. Malabadi, Chem. Data Collect., 2021, 33, 100684 CrossRef CAS.
- J. S. Yaradoddi, N. R. Banapurmath, S. V. Ganachari, M. E. M. Soudagar, N. M. Mubarak, S. A. Hallad, S. Hugar and H. Fayaz, Sci. Rep., 2020, 10(1), 21960 CrossRef CAS PubMed.
- B. Ai, L. Zheng, W. Li, X. Zheng, Y. Yang, D. Xiao, J. Shi and Z. Sheng, Front. Plant Sci., 2021, 12, 625878 CrossRef PubMed.
- F. M. Monedero, M. J. Fabra, P. Talens and A. Chiralt, J. Food Eng., 2009, 91, 509–515 CrossRef CAS.
- C.-H. Chen, W. S. Kuo and L.-S. Lai, Food Hydrocolloids, 2009, 23, 714–721 CrossRef CAS.
- M. G. Carneiro-Da-Cunha, M. A. Cerqueira, B. W. S. Souza, M. P. De Souza, J. A. Teixeira and A. A. Vicente, J. Food Eng., 2009, 95, 379–385 CrossRef CAS.
- X. Li, D. Shen, Q. Zang, Y. Qiu and X. Yang, Nat. Prod. Commun., 2021, 16, 1934578X2110383 CrossRef.
- L. B. Proença, C. A. P. Pena, G. V. Da Silva, I. L. B. Da Cunha Camargo and M. C. Branciforti, Macromol. Symp., 2020, 394(1), 2000073 CrossRef.
- Y. Yuan, X. Geng, W. Hua, R. Kumar, J. Wang, J. Xiao and H. Tian, J. Food Process. Preserv., 2022, 46(7), e16585 CAS.
- Z. Tomičić, R. Tomičić, S. Kocić-Tanackov and P. Raspor, Food Feed Res., 2022, 49, 107–115 CrossRef.
- F. Laffleur, M. Ataii and M. Nagler, Eur. J. Pharm. Sci., 2021, 167, 105989 CrossRef CAS PubMed.
- F. Chidi, A. Bouhoudan and M. Khaddor, J. King Saud Univ., Sci., 2020, 32, 2041–2045 CrossRef.
- A. Kadam, S. Singh and K. K. Gaikwad, Food Control, 2021, 124, 107877 CrossRef CAS.
- A. B. Arfa, S. Combes, L. Preziosi-Belloy, N. Gontard and P. Chalier, Lett. Appl. Microbiol., 2006, 43, 149–154 CrossRef PubMed.
- V. Katalinić, M. Miloš, T. Kulišić and M. Jukić, Food Chem., 2006, 94, 550–557 CrossRef.
- C.-C. Wong, H.-B. Li, K. Cheng and F. Chen, Food Chem., 2006, 97, 705–711 CrossRef CAS.
- M. C. López, F. M. Martínez, C. Valle, M. Ferrit and R. Luque, Talanta, 2003, 60, 609–616 CrossRef PubMed.
- M. Viuda-Martos, Y. R. Navajas, E. S. Zapata, J. Fernandez-Lapez and J. A. Parez-Alvarez, Flavours Fragrances, 2009, 25, 13–19 CrossRef.
- P. Cazón, A. Antoniewska, J. Rutkowska and M. Vázquez, Int. J. Biol. Macromol., 2021, 186, 365–376 CrossRef PubMed.
- A. A. Dehpour, M. A. Ebrahimzadeh, N. S. Fazel and N. S. Mohammad, Grasas Aceites, 2009, 60, 405–412 CrossRef CAS.
- A. Rašković, I. Milanović, N. Pavlović, T. Ćebović, S. Vukmirović and M. Mikov, BMC Complementary Altern. Med., 2014, 14, 1–9 CrossRef PubMed.
- B. Gewert, M. Plassmann and M. MacLeod, Environ. Sci.: Processes Impacts, 2015, 17, 1513–1521 RSC.
|
This journal is © The Royal Society of Chemistry 2024 |
Click here to see how this site uses Cookies. View our privacy policy here.