DOI:
10.1039/D3SU00411B
(Communication)
RSC Sustain., 2024,
2, 621-625
Biomass composting with gaseous carbon dioxide capture†
Received
7th November 2023
, Accepted 18th January 2024
First published on 24th January 2024
Abstract
Biomass carbon removal and storage (BiCRS) technologies must scale rapidly to mitigate climate change via the removal of carbon dioxide (CO2) from the atmosphere. BiCRS technologies passively concentrate atmospheric CO2 and thus greatly reduce energy demands for atmospheric carbon removal, relative to direct air capture (DAC) technologies. Composting with gaseous CO2 capture is an overlooked BiCRS technology with significant potential for atmospheric carbon removal. For the first time, we demonstrate the capture of high purity gaseous CO2 from biomass composting. Biomass is composted in simple, closed reactors with automated cycling of air or oxy-fuel to generate gaseous streams with CO2 concentrations varying between 18 and 95%, which are significantly higher than the CO2 concentration of air (∼0.04%); the minimum thermodynamic energy needed for CO2 capture from composting is 72–98% lower than that for the capture of CO2 directly from the air. Genomic data indicate microbial diversity decreases with the use of oxy-fuel relative to air. Globally, the composting of food waste could capture 0.3–1.0 billion tonnes of biogenic CO2 per year, and the inclusion of other biomass feedstocks could increase the total capture rate to more than 3.5 billion tonnes per year.
Sustainability spotlight
Atmospheric carbon removal technologies that scale quickly and are of low technical, economic, environmental, and social risk are needed urgently. Biomass carbon removal and storage (BiCRS) technologies use biomass to remove CO2 from the atmosphere and store that CO2 underground or in long-lived products. Many BiCRS technologies have potential negative side effects that hinder the overall sustainability, including nutrient robbery, indirect land use change, and soil degradation. Composting with gaseous CO2 capture has the potential to avoid such negative side effects by providing substantial ecosystem benefits in addition to carbon removal. This work addresses the following UN Sustainable Development Goals: climate action, responsible consumption and production, sustainable cities and communities, clean water and sanitation, and industry, innovation, and infrastructure.
|
Introduction
By 2050, billions of tonnes of CO2 must be removed from the atmosphere annually to keep global warming below 2 °C and avoid the worst effects of climate change, according to the Intergovernmental Panel on Climate Change (IPCC).1 Biomass carbon removal and storage (BiCRS) entails a set of carbon dioxide removal (CDR) technologies that rely on the passive process of photosynthesis to remove carbon from the atmosphere with subsequent stabilization and sequestration of the biomass carbon.2 Specifically, BiCRS technologies must (1) use biomass to remove CO2 from the atmosphere, (2) store that CO2 underground or in long-lived products, and (3) do no damage to – and ideally promote – food security, rural livelihoods, biodiversity conservation, and other important values.2 Relative to direct air capture technologies, BiCRS technologies require significantly less energy in the forms of electricity and heat, which enables the potential for significantly lower costs of CO2 capture and stabilization.3 The leading BiCRS technologies include gasification, pyrolysis, combustion, anaerobic digestion, fermentation and biomass burial.4 Given the urgency of the changing climate, society needs BiCRS solutions that scale quickly with minimal risk. Herein, we demonstrate for the first time composting with CO2 capture and sequestration as a new BiCRS technology pathway with potential to deliver near-term carbon removal with substantial co-benefits. Composting, like all BiCRS technologies, begins with CO2 fixation in living biomass via photosynthesis followed by temporary carbon storage in various forms of biomass products, as shown in Fig. 1. Composting utilizes naturally occurring microorganisms to passively convert part of the carbon stored in biomass to CO2via aerobic respiration.5 Essentially, composting controls and accelerates the natural decay of organic carbon. Significant quantities of CO2 are released during the composting process, thereby offering an opportunity for atmospheric carbon removal if the CO2 is captured and sequestered permanently. Globally, the composting of food waste could capture 0.3–1.0 billion tonnes of biogenic CO2 per year, and the inclusion of other biomass feedstocks could increase the total capture rate to more than 3.5 billion tonnes of biogenic CO2 per year, thereby representing a significant opportunity for atmospheric carbon removal.6,7
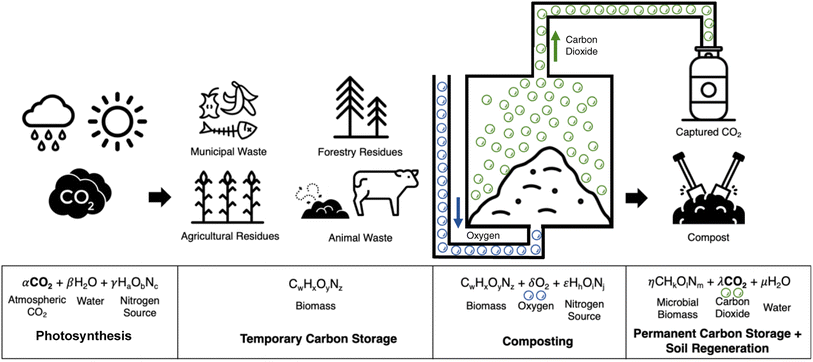 |
| Fig. 1 Process for atmospheric carbon removal via composting of biomass waste with gaseous CO2 capture. | |
Composting is an autothermal process wherein the operating temperature is naturally maintained at 40–65 °C due to exothermic biochemical reactions, thereby reducing the need for energy input to control temperature and potentially offering the opportunity to recover heat for other uses. The elevated temperature of composting deactivates pathogens and weed seeds in the biomass waste materials, ensuring the solid compost product will not promote plant disease or weed growth when applied to soils. According to EPA regulations, static aerated composters must treat organic materials at 55 °C or higher for 3 days and windrow composters must treat organic materials at 55 °C or higher for 15 days or longer with at least 5 turning events to inactivate pathogens.8 Industrial composting operators typically allow their compost to degrade in open piles, and use aeration and/or mixing to control the temperature of their organic material, and temperatures exceeding 55 °C are common.9 Unlike most other BiCRS technologies, composting returns organic carbon and nutrients (NPK) to the soil for fertilization and regeneration. In addition, composting is capable of handling inconsistent, diverse, and low quality biomass feedstocks due to the robust and abundant communities of wild-type microorganisms that thrive on decaying biomass of varying compositions. The conditions in landfills and lagoons promote anaerobic decomposition of biomass which releases significant quantities of heat trapping methane.10 The use of aerobic microorganisms prevents methane generation during composting. However, traditional composting methods have been shown to produce methane when not operated in an optimal fashion with sufficient oxygen supply, and thus new composting methods that eliminate methane generation are needed.11 Nitrous oxide has also been observed from composting operations with excessive nitrogen loading, warranting careful mixing of feedstocks and frequent gas monitoring to minimize nitrous oxide emissions.11 Engineered anaerobic digestion (AD) is similar in approach to that of composting given its reliance on wild-type communities of microorganisms. However, AD operations are difficult to reliably control, require costly inputs, have relatively slow kinetics, and are limited to fewer biomass feedstocks.4 Notably, the AD of lignocellulosic biomass typically requires exogenous energy input to maintain temperatures of 50–60 °C for optimal enzyme performance, whereas aerobic composting achieves these temperatures passively.12–14 Like composting, AD has the potential to recycle nutrients to the soil through application of digestate, but there are some potential barriers including the presence of pathogens and excess ammonium.15 Finally, composting generates significant quantities of relatively pure water via biochemical reactions, as shown in Fig. 1. Currently, much of the water generated from composting is passively evaporated from open compost piles to the atmosphere. In closed systems, the opportunity exists to capture the water for other uses, such as irrigation of crops.
Capturing CO2 from composting operations can be accomplished via pre- or post-composting, analogous to pre- and post-combustion CO2 capture.16 In pre-composting CO2 capture, pure O2 or oxy-fuel (a mix of O2 and CO2) is fed to the composting reaction with subsequent collection of high purity CO2 (∼95 vol%); the term pre-composting is used because gas separation takes place prior to composting via separation of O2 from N2 in air. In post-composting CO2 capture gas separation takes place after composting via CO2 separation from N2. For the first time, we generate high purity biogenic CO2 from composting and demonstrate the potential of pre- and post-composting CO2 capture. In addition, we quantify pertinent composting metrics including carbon to nitrogen ratio, moisture content, gas concentrations, and the carbon conversion efficiency. We also explore the variation in microbial diversity and abundance between different gas atmospheres.
Results and discussion
Carbon conversion efficiency
An important metric for the efficiency of composting is the percentage of initial carbon converted to CO2, which is referred to as carbon conversion efficiency, or CCE (eqn (1)). |  | (1) |
The CCE values for traditional composting range from 10 to 75%, with the composition of the feedstock and composting conditions playing major roles.17–19 Food and animal waste feedstocks typically have CCE values exceeding 30%, whereas lignocellulosic materials have CCE values less than 30%.17–19 CCE values in excess of 30% are achieved in both air and oxy-fuel composting reactions in this study, as shown in Table 1. The composting reaction under air conditions exhibits higher CCE than oxy-fuel, due in part to the increased kinetics at the beginning of the reaction. A lag phase is observed under oxy-fuel conditions, which is likely due to the microbes adapting to and selecting for the high CO2 environment. At the end of 15 days, all reactors still exhibit CO2 production, indicating that the composting reactions are not complete, which is deemed acceptable since the primary objective of this work is to demonstrate the ability to generate high purity biogenic CO2 from composting. The increase in moisture content shown in Table 1 is due to the synthesis of water from the biomass waste; see Fig. 1 for the biochemical reaction stoichiometry.
Table 1 Initial and final carbon to nitrogen ratios and moisture contents, and carbon conversion efficiencies (CCEs) for biomass waste composted under air and oxy-fuel conditions
Oxidant |
C : N ratio |
Moisture content |
Carbon conversion efficiency (CCE) |
Initial |
Final |
Initial |
Final |
Air |
21.3 |
14.5 ± 0.4 |
61.9% |
67.6% ± 0.2% |
39.3% ± 0.3% |
Oxy-fuel |
21.3 |
15.2 ± 0.1 |
61.9% |
65.1% ± 0.2% |
30.6% ± 1.7% |
High purity CO2 capture
The generation of high purity CO2 is achieved using oxy-fuel (70 vol% CO2 and 30 vol% O2) and air, as shown in Fig. 2. As the O2 concentration decreases the CO2 concentration increases due to the biochemical reactions involved in microbial respiration, as shown in Fig. 1. The oxy-fuel reactors achieve CO2 concentrations exceeding 95 vol%, similar to the concentrations achieved during ethanol fermentation of starch.20 Oxy-fuel is used over pure O2 due to the safety concerns of using pure O2 in the presence of biomass for extended periods of time; proof of concept using pure O2 is demonstrated for several hours of operation and the resultant data can be found in Fig. S1.† The air-fed reactors achieve CO2 concentrations exceeding 18 vol%, similar to the concentrations achieved from lime calcination.21 Notably, gas chromatography showed no methane present in the headspace of both air and oxy-fuel reactors; the gas samples were taken from the reactors when oxygen levels were near-zero. Regarding N2O emissions, 16S rRNA sequencing data were used to conduct a Phylogenetic Investigation of Communities by Reconstruction of Unobserved States (PICRUSt2) analysis, which showed a lack of genes necessary for N2O synthesis; see ESI† for more information. As shown in Fig. 2, the reactors that operate under pressure (3 psig), demonstrate less frequent gas cycling due to the availability of more O2 to the microbes, relative to the ambient pressure reactors. The reactors are pressurized to ensure the high purity CO2 gas can be ejected downstream for capture before injecting fresh O2 and to increase the CO2 productivity per cycle. As shown in Table S1,† the minimum thermodynamic energies required for capturing CO2 from the air-fed and oxy-fuel fed composting reactors are 144 and 10 kJ per kg CO2, respectively, which are 72% and 98% lower than that for direct air capture (500 kJ per kg CO2). Thus, composting with CO2 capture has the potential to be considerably more energy efficient than direct air capture. We recognize a transition from traditional open composting system to engineered composting systems with CO2 capture would require a substantial investment in infrastructure due to the increase in process intensity and complexity. However, with CO2 purities ranging from 18 to 95%, this investment would likely be less intensive than other carbon capture pathways, such as direct air capture. In the current composting industry, a relatively large facility generates 20
000 wet tonnes of compost per year.22 If CO2 capture is implemented at such a facility, approximately 10
000 tonnes of CO2 would be captured per year. Thus, the scale of CO2 capture per site is relatively small compared with other biomass facilities such as bioenergy with carbon capture and sequestration, which can capture more than 500
000 tonnes of CO2 per year per site.4 However, there is sufficient compost feedstock available to capture relatively large quantities of biogenic CO2 at new, larger industrial composting facilities (Fig. 3).
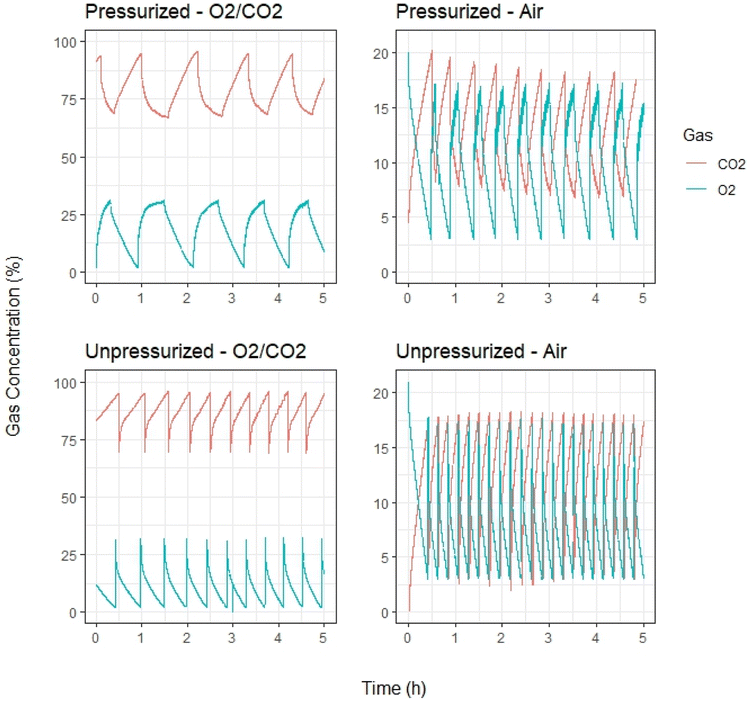 |
| Fig. 2 Experimental bioreactor gas composition data demonstrating the process of achieving high purity biogenic CO2 using oxy-fuel (O2/CO2) and air under ambient and pressurized conditions. Data collected over a 5 hour period once reactors are at steady state. | |
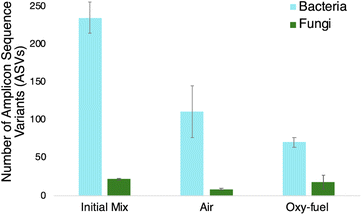 |
| Fig. 3 The microbial diversity of the initial mix before composting and final compost materials after 15 days of reaction under air and oxy-fuel conditions. | |
Microbial diversity
16S and internal transcribed spacer (ITS) rRNA sequencing are performed to understand the microbial diversity of bacteria and fungi, respectively, present in the biomass materials under different composting conditions. Notably, the use of oxy-fuel causes a decrease in microbial diversity, relative to air, which can be explained by the very high CO2 environment (∼95 vol%). The high CO2 environment can lead to relatively high levels of carbonic acid production, which may contribute to the decrease in microbial diversity. Notably, the number of amplicon sequence variants (ASVs) indicates relative microbial diversity, but does not indicate relative abundance; see ESI† for information pertaining to relative abundance. The air-fed reactors also exhibit a reduced diversity relative to the initial mix, which indicates that moderate CO2 concentrations (∼18 vol%) also select for certain microbes. Although the CO2 concentration in the air-fed reaction is lower than the oxy-fuel reaction, it is still much higher than the ambient CO2 concentration.
Conclusions
For the first time, we demonstrate the capture of high purity gaseous CO2 from biomass composting using simple, closed bioreactors with automated gas cycling. CO2 concentrations ranging from 18 to 95 vol% are obtained using air and oxy-fuel as oxidants, requiring 72–98% less energy for final CO2 capture and purification relative to direct air capture. Microbial biodiversity in the composting process is reduced in such systems likely due to the elevated CO2 concentrations. The potential for atmospheric carbon removal exists via composting with CO2 capture, and further work should include complete bioprocess optimization with multiple feedstocks and life cycle assessment to quantify the net carbon removal potential. In addition, further metagenomic analyses are needed to understand and improve microbial metabolics and kinetics. Finally, the solid compost products resultant from systems with CO2 capture must be assessed for quality and compared to state-of-the-art compost products.
Author contributions
E. Woods led experimental work and contributed to literature review and writing. V. R. Berrio, Y. Qiu, and P. Berlin contributed to literature review and experimental work. N. Clauser contributed to literature review and writing. W. J. Sagues led literature review and writing.
Conflicts of interest
The authors declare the following competing financial interest(s): William J. Sagues has equity in Flip Biosystems, Inc.
Acknowledgements
This research was financially supported by funding internal to North Carolina State University via a Goodnight Early Career Award. The authors acknowledge “The Noun Project” (https://thenounproject.com/) for the icons used in the graphical abstract.
References
-
M. Allen, H. Coninck, O. Pauline, O. Hoegh-Guldberg, D. Jacob, K. Jiang, A. Revi, J. Rogelj, J. Roy, D. Shindell, W. Solecki, M. Taylor, P. Tschakert and H. Waisman, IPCC, 2018 Search PubMed.
-
D. Sandalow, J. Friedmann and C. Mccormick, ICEF, 2021 Search PubMed.
- J. K. Soeherman, A. J. Jones and P. J. Dauenhauer, ACS Eng. Au, 2023, 3(2), 114–127 CrossRef CAS.
- J. P. Dees, W. J. Sagues, E. D. Woods, H. Goldstein, A. J. Simon and D. Sanchez, Green Chem., 2023, 25, 2930–2957 RSC.
-
R. Rynk, L. Cooperband, C. Oshins, H. Wescott, J. Bonhotal, M. Schwarz, R. Sherman and S. Brown, Why Compost?, Elsevier Inc., 2022 Search PubMed.
- N. S. Bentsen, C. Felby and B. J. Thorsen, Prog. Energy Combust. Sci., 2014, 40, 59–73 CrossRef.
-
Food and Agriculture Organization of the United Nations, Food Wastage Footprint, 2013 Search PubMed.
-
EPA Composting Regulations, https://www3.epa.gov/npdes/pubs/503pe_5.pdf Search PubMed.
-
F. Michel, T. O'Neill, R. Rynk, J. Gilbert, S. Wisbaum and T. Halbach, Passively Aerated Composting Methods, Including Turned Windrows, Elsevier Inc., 2022 Search PubMed.
-
C. Oshins, F. Michel, P. Louis, T. L. Richard and R. Rynk, The Composting Process, Elsevier Inc., 2022 Search PubMed.
- S. L. Nordahl, C. V Preble, T. W. Kirchstetter and C. D. Scown, Environ. Sci. Technol., 2023, 57, 2235–2247 CrossRef CAS PubMed.
- L. Kabaivanova, P. Petrova, V. Hubenov and I. Simeonov, Life, 2022, 12, 702 CrossRef CAS PubMed.
- F. R. Amin, H. Khalid, H. Zhang, S. Rahman, R. Zhang, G. Liu and C. Chen, AMB Express, 2017, 7, 72 CrossRef PubMed.
- C. Sawatdeenarunat, K. C. Surendra, D. Takara, H. Oechsner and S. K. Khanal, Bioresour. Technol., 2015, 178, 178–186 CrossRef CAS PubMed.
- P. Swiatczak and A. Cydzik-Kwiatkowska, Water, Air, Soil Pollut., 2018, 229, 247 CrossRef PubMed.
- M. Bui, C. S. Adjiman, A. Bardow, E. J. Anthony, A. Boston, S. Brown, P. S. Fennell, S. Fuss, A. Galindo, L. A. Hackett, J. P. Hallett, H. J. Herzog, G. Jackson, J. Kemper, S. Krevor, G. C. Maitland, M. Matuszewski, I. S. Metcalfe, C. Petit, G. Puxty, J. Reimer, D. M. Reiner, E. S. Rubin, S. A. Scott, N. Shah, B. Smit, J. P. M. Trusler, P. Webley, J. Wilcox and N. Mac Dowell, Energy Environ. Sci., 2018, 11, 1062–1176 RSC.
- G. Breitenbeck and D. Schellinger, Compost Sci. Util., 2004, 12, 365–371 CrossRef.
- X. Hao, C. Chang and F. Larney, J. Environ. Qual., 2004, 33, 37–44 CrossRef CAS PubMed.
- J. K. Andersen, A. Boldrin, T. H. Christensen and C. Scheutz, Waste Manage., 2011, 31, 1934–1942 CrossRef CAS PubMed.
- D. Sanchez, N. Johnson and S. McCoy, Proc. Natl. Acad. Sci. U. S. A., 2018, 115, 4875–4880 CrossRef CAS PubMed.
- W. J. Sagues, H. Jameel, D. L. Sanchez and S. Park, Energy Environ. Sci., 2020, 13, 2243–2261 RSC.
-
R. Rynk, N. Koerting, J. Ziegenbein, J. Hardin, C. Oshins, N. J. Brown, N. J. Lampen and D. Lilkas-Rain, Facility Management, Elsevier Inc., 2021 Search PubMed.
Footnote |
† Electronic supplementary information (ESI) available: Materials and methods.15–20 Additional bioreactor gas composition data in Fig. S1.† Thermodynamic minimum work requirements for CO2 separation from various gas streams in Table S1.† Predicted pathways heatmap with PICRUSt2 (Phylogenetic Investigation of Communities by Reconstruction of Unobserved States) from 16S rRNA. Functional genes involved in denitrification to form nitrous oxide. Relative abundance of bacteria at taxonomic level for compost samples. See DOI: https://doi.org/10.1039/d3su00411b |
|
This journal is © The Royal Society of Chemistry 2024 |