DOI:
10.1039/D3SU00295K
(Paper)
RSC Sustain., 2024,
2, 710-720
Fulvic acid modification with phenolic precursors towards controllable solubility performance†
Received
23rd August 2023
, Accepted 29th January 2024
First published on 5th February 2024
Abstract
Fulvic acids possess important properties for soil improvement and take part in processes such as complexation, chelation, buffering, transport of vitamins and minerals, as well as pH effects. However, their high solubility in water results in rapid runoff through soil especially when applied on sandy soils, allowing fulvic acids only a short-term amendment activity. To address this problem, commercial fulvic acid, which has high-water solubility, was modified to tune solubility performance through acid-catalyzed condensation with phloroglucinol and glyoxylic acid. Phloroglucinol acts as a source of aromaticity and as a reactive aromatic compound while glyoxylic acid possesses an aldehyde function, which plays the role of formaldehyde in classical phenol-formaldehyde condensation, and a carboxylic acid functional group, which provides necessary acidity for the self-catalyzed condensation process. The formation mechanism of such oligomers was investigated through NMR, GPC and CPMAS. By modifying commercial fulvic acid using sustainable methods, its solubility can be tuned, enabling controlled and prolonged delivery of beneficial properties to soils, thereby enhancing soil improvement while minimizing rapid runoff and optimizing resource utilization.
Sustainability spotlight
Humic substances are soil biopolymers regarded as a massive sink of the carbon with potential to store the excessive amounts of carbon dioxide in form of organic matter and help to balance the green gas effects on the climate. Humic substances are essential for soil quality, prevent soil erosion and desertification. They are a fundament of soil fertility and have positive effects on plants. Fulvic acids are water soluble components of humic substances. They are commercially available as soil fertilizers obtained as a byproduct of urban water purification. To prolong their effects in soil, we tailored their solubility by co-polymerizing with environmentally friendly glyoxylic acid and phloroglucinol as a model aromatic linkage. Obtained products didn't compromise plant growth. UN's Sustainable Development Goals: G2 Zero hunger; G11 Sustainable cities and communities; G13 Climate action; G15 Life on Land.
|
Introduction
Humic substances are omnipresent natural polymers with rich functionality originating from the biological and chemical degradation of animal and plant residues.1 These species represents 95% of all natural polymers within the biosphere.2 Humic substances of soil are often distinguished into three major components: humin,3 humic acids (HAs)4 and fulvic acids (FAs).5 These constituents represents up to 80% of the total soil organic matter (SOM).6,7
Accumulation of the SOM starts from photosynthetic carbon dioxide fixation by plants in the form of complex chemical compounds and natural polymers. Annually photosynthesis results in the fixation of upwards of 60 billion tons of carbon through terrestrial plants,8 and nearly as much inside the world's oceans.9 Depending on the type of biomass and climate conditions, only a limited amount of this fixed carbon undergoes humification reactions and thereby preserves the carbon in form of humic substances. Climate change and destructive agricultural practices prohibit soil natural recovery and cause carbon to leak back into the atmosphere. Soil fertility is also an issue for the increasing demand for agricultural lands. Targeted introduction of humic substances can simultaneously increase the amount of fixed carbon within soil, help to maintain healthy soil and increase crop yields preventing the constantly increasing overuse of agricultural lands.10
Fulvic acids are one of the most underrated contributors to our health and to the agricultural industry. They are strong antioxidants, include complex organic phenols and polyelectrolytes that enable the beneficial ingestion of nutrients in our food and support plants growth.11–13 The complex and heterogeneous FA structure contains molecules with many different functional groups among which the carboxylic and phenolic groups stand out as the most prominent in terms of both quantity and biological activity.13 These groups, characterized by their unique chemical structures and reactivity, play crucial roles in numerous biological processes.14 The carboxylic group imparts acidity and participates in diverse biochemical reactions, such as enzyme catalysis and cellular signaling.15 Phenolic groups endow compounds with antioxidant,16 anti-inflammatory17 and antimicrobial properties.18 There are several main natural sources of FAs from which they are extracted as side products of more widely used HAs, such as: shilajit,19,20 soil,21,22 peat,23,24 coal,14 latosols25 and lake sediments26etc. Fulvic acids perform several important tasks for soil improvement such as complexation, chelation, buffering, transportation of vitamins and minerals, as well as pH adjustment. Despite all of the advantages of using FAs to improve soil fertility, they have a common drawback that drastically hinders their agricultural application when compared to HAs: their high solubility in water. This property results in the rapid runoff of FAs through soil, especially when applied on sandy soils, allowing the FAs only short-term amendment activity.27
In order to tune and improve certain characteristics, including solubility and binding affinity to mineral compounds, a modification of FAs could be performed. Hua et al. proposed FA modification with potassium persulfate as initiator and acrylic acid as the graft monomer in order to synthesize a modified mineralized FA that was investigated as a solubilizing agent for scale and for its dispersing properties of calcium phosphate. The modified FA product chelates calcium ions, which significantly increased the dispersibility of calcium phosphate in solution and effectively inhibited its precipitation during irrigation with a water-soluble fertilizer through the pipeline.28 Zhang et al. employed p-phenylenediamine and poly(lactic acid) to enhance the impact strength, crystallinity, and nucleation rate of mineral crystals by FA.29 Liu et al. used FA-modified walnut shell biochar to enhance the adsorption efficiency of isopropyl chloride in water. The maximum adsorption capacity achieved was 99.01 mg g−1, almost a two-fold increase compared to the original biochar (51.28 mg g−1).30 However, for practical soil applications, FAs modifications are still to be investigated. Surely, these modifications should be environmentally safe, accessible and fitting within the paradigms of circular economy. Hydrothermal carbonization represents a sustainable solution for biomass valorization for soil application, but produces considerable amounts of phenols as side products.31 While toxic in direct application, phenols could be used for FAs modification following nature inspired transformations of FAs to less water soluble HAs.
The general aspects of phloroglucinol (PG) and glyoxylic acid (GA) condensation were firstly developed by Ghimbeu and co-workers aiming for environmentally friendly precursors for mesoporous carbon formation in 2014.32 Phloroglucinol acts in this process as a source of aromaticity and as a reactive aromatic compound. This phenol derivative is the monomeric unit of phlorotannins and can be isolated from the bark of fruit trees,33 from brown algae34 or through diverse biosynthetic pathways.35 Phloroglucinol is produced commercially as an intermediate for pharmaceutical industry and as a reprographic chemical, though the production of phloroglucinol at the moment remains concerning,36 and thus used in this work as a model aromatic compound due to the well defined interaction with GA. Another component of this modification process is GA. It has been selected for several reasons: GA possesses aldehyde functional group which can take the role of formaldehyde in classical phenol-formaldehyde condensation and a carboxylic acid functionality which provides the necessary acidity for the self-catalyzed condensation process. Investigation of this treatment could potentially solve a few common issues within the realm of agriculture. For instance, the reaction aims removal and valorization of phenol derivatives released during the hydrothermal synthesis of biochars, as phenols can be directly condensed with FAs. Moreover, modification processes can provide a tool over transition from FAs towards humic-like acids as it is known that the main attribute of FAs is a lower molecular weight (ranging from approximately 1000 to 10
000 Da) characterized by the absence of (–CH3) groups, and a lower concentration of aromatic groups compared to humic acids obtained from the same sources.37 Finally, the control over molecular weight should affect solubility properties and run-off behavior when modified FAs are applied.
The goal of this study is to modify a commercially available, water-soluble, bioactive growth promoting product based on natural aquatic FAs with a triphenol, PG, by a condensation reaction with GA, obtaining compounds with lower solubility and therefore less likely to run off in sandy soils and preserving a long term fertility. Precursors for the modification can be derived from natural sources, laying background for sustainability of the process. Decreased solubility of the FAs should also provide prolonged effects due to the longer retention time in topsoil layer. The assessment of the effects of the biostimulant is beyond the scope of this paper, however, a germination assay on Lepidium sativum was performed with modified FAs in order to assess possible phytotoxic effects.
Experimental section
Materials
Phloroglucinol (PG) (Sigma Aldrich, ≥99.0%), glyoxylic acid (GA) (Sigma Aldrich, monohydrate, 98.0%), FA (Basic Fulvagra, WSG 90, for leaf and ground application pH = 7–8, water soluble granules), deuterium oxide (ThermoScientific, 100 atom %), syringe filters (Whatman, Puradisc FP, PTFE, 30 mm, 0.20 μm, sterile), Lepidium sativum seeds, sandy soil collected nearby Max-Planck Institute of Colloids and Interfaces, Department of Colloid Chemistry, squared Petri dishes.
FA modification
For the modification of FA, 2 solutions were prepared. To prepare a solution of phenolic precursors, solid GA (0.760 g) and PG (1.025 g) were dissolved in 25 ml of water. To ensure complete dissolution of these substances, the solution was left on a magnetic stirrer for 1 hour at a stirring speed of 300 rpm. It is important to utilize a freshly prepared solution since the condensation process of phenolic precursors after dissolution is inevitable. The FA solution was prepared by dissolving 1 g of FA (Basic Fulvagra WSG 90, aquatic FA) in 25 ml of water. Each sample was denoted as VTxy, where x represents the volume of the FA solution (in ml) and y represents the volume of the phenolic precursor's solution used (in ml). For example, VT21 indicates that 2 ml of FA and 1 ml of phenolic precursors solutions were utilized.
Once both solutions were prepared, they were combined in 25 ml vials by employing various ratios of the initial reagents. To ensure complete filling, each vial was topped up with water. The resulting mixture was then heated at 60 °C for a duration of 24 hours. Two different modification protocols were employed: the open-vial protocol (OVP) and the closed-vial protocol (CVP).
In the OVP, all water evaporated from the vial during the modification process. For the CVP, freeze-drying was employed to obtain the modified product.
NMR spectroscopy
1H NMR spectra were recorded on Agilent 400 MHz. NMR of FA was performed as received in d-D2O at 1 wt%. FA after both OVP and CVP modification with phenolic precursors was dried in vacuum oven during 1 day at 60 °C to reduce moisture content. The samples were dissolved in d-D2O at 1 wt%.
CPMAS spectroscopy
13C–1H cross polarization magic angle spinning (CPMAS) measurements were carried out using a Bruker range Avance 400 MHz Solid State spectrometer operating at 100.6 MHz and a Bruker 4 mm double resonance probe-head operating at a spinning rate of 8 kHz.
GPC
Molar weights of modified FA were determined by SEC in 0.067 mol L−1 Na2HPO4 in Bi-distilled H2O at 25 °C. Samples with precise concentrations in the range 1–1.5 mg ml−1 were filtered through 0.45 μm filter before the injection. The flow rate was 1.0 ml min−1. The following Agilent 1260 Infinity series setup was used: a PSS-SECurity-1260 isocratic pump; a G1322A degasser; a G1329B auto-sampler; a G1316A thermostated column compartment equipped with a set of sulfonated styrene-divinylbenzene copolymer network (guard column (50 × 8 mm) and main column (30 × 8 mm); a G1314B variable wavelength detector; a G7800A multidetector suite equipped with MDS refractive index detector and MDS viscometer detector. Universal calibration was performed using a set of polystyrene sulfonic acid-sodium salt (PSS). Agilent GPC/SEC software and multi-detector upgrade were used to determine molar weight values and distributions.
UV-vis spectroscopy
Kinetics of the VT11 run condensation was monitored using a T70+ UV/VIS Spectrometer (PG Instruments Ltd) equipped with a thermostat at 60 °C during the first 40 minutes. The measurements were performed in 1 mm cuvette in the wavelength range from 350 to 700 nm.
FTIR spectroscopy
FTIR spectra were measured on the Nicolet iS5 with an iD5 ATR crystal, Thermo Fisher Scientific Inc. (Waltham, Massachusetts, USA), with a resolution of 0.5 cm−1.
Solubility study
To assess changes in solubility, 50 mg of FA modified via either OVP or CVP procedures were mixed in 5 ml of Millipore water to obtain a 1 wt% of solid. The resulting mixture was then subjected to stirring at 300 rpm for 2 hours. It is worth noting that although a highest concentration of 0.01 wt% was employed in the germination experiments, a concentration of 1% was chosen for the dissolution test. This concentration was the minimum required for the selected method to accurately determine the percentage of soluble modified FA that passed through the 200 nm filter.
Subsequently, the solution was filtered through a 200 nm filter using a syringe, and the solvent was evaporated in a vacuum oven at 80 °C for 24 hours. The quantity of modified FA that passed through the filter was calculated as a percentage relative to the initially used 50 mg of modified FA. This approach allowed for the evaluation of the solubility changes and the determination of the soluble fraction of the modified FA.
Elemental analysis
Elemental analysis was performed with a vario-MICRO cube CHNOS Elemental Analyzer (Elementar Analysensysteme GmbH, Langenselbold) The elements have been detected with a thermal conductivity detector (TCD) for C, H and N.
Germination study
The germination tests were carried out on cress seeds (Lepidium sativum L.) using sandy soil collected nearby Max-Planck Institute of Colloids and Interfaces. The soil was placed into an oven to completely dry (48 h at 60 °C) and then passed through a 2 mm sieve. Around 95 g of dry soil were placed into squared Petri dishes and the moisture content was adjusted by adding distilled water to the soil until the moisture content of 16.8% was reached. Next, 10 seeds of Lepidium sativum L. were sowed on the top of the Petri dishes and 10 μL of either distilled water, control FA, or modified FAs were added on the zone immediately below each seed. The tests were conducted using three different concentrations (0.01 wt%, 0.005 wt%, and 0.001 wt%) of the substrates (FA, VT11, and VT15, respectively). To provide a specific example, a concentration of 0.01 wt% corresponds to applying 0.0001 g of the substrate per 1 g of soil. The Petri dishes were closed and vertically incubated in dark at 25 °C for 72 h. Each germination test was performed in triplicate for the three concentrations, for a total of 30. Then the Petri dishes were opened, and the root and shoot length were analyzed with the software ImageJ (https://imagej.nih.gov/ij/index.html), while germination percentage (GP) was calculated defining as germinated the seedlings that had both, shoot and root, longer than 1 cm.
Results and discussion
Mechanism of FA modification with phenolic precursors
Natural FAs are rich in oxygen containing functional groups suitable for modifications with phenolic precursors and aldehyde-acids. The presence of carboxylic and phenolic functional groups on initial FA used in this study for modification is evidenced by FTIR spectroscopy (see ESI: Fig. S1†). Namely, prominent infrared adsorption bands include the C
O stretching band of carboxylic functional groups (∼1740 cm−1), the asymmetric absorption band of carboxylate (COO−) groups (∼1546 cm−1), and the vibrational mode of phenolic hydroxyl groups (∼1367 cm−1). The presence of phenol and carbonyl groups makes the FAs available to react with PG and GA. Due to the involvement of the phenolic carboxylic groups of FAs, the general mechanisms of the reaction between phenols and aldehyde-acids can be adopted for this modification, though unlike the classical case of the PP and GA interaction presence of the phenylacetate groups in FAs adds variability to the modification steps.
The mechanisms of two possible pathways of the FAs modification with PP and GA are depicted on Scheme 1. In the first pathway, the reaction starts with the aromatic electrophilic substitution of the phenolic ring of FAs by GA hydrate resulting in phenylacetic acid formation (red arrow) that proceeds the same way as interaction of GA with PG. The next step of the modification is electrophilic aromatic substitution of the phenylacectic acid with phenol depicted with the green arrow on the left hand of the Scheme 1. A phenylacetic group required for this step is also present in the FAs and will undergo the substitution reaction with phenols as well. This alternative is shown with the green arrow on the right. Phenylacetic acid in this step can interact either with monophenolic molecules or with condensed oligomers that can be present in solution. Further water elimination leads to the lactone formation (blue arrow). The second modification pathway is a direct condensation of FAs carboxylic groups with a hydroxyl group of PG and formation of esters (purple arrow). This pathway will further progress through nucleophilic substitution with GA (red arrow) and result in phenylacetate functionality available for the modification according to the first pathway. Multiple reaction combinations thus are possible on the phenol ring depending on the reaction time, precursors local concentration etc., as well as functional diversity of FA and phenolic precursors.
 |
| Scheme 1 Schematic representation of possible reactions of FA modification with PG and GA. | |
While the abundance of FA functionality allows for the possibility of reaction with phenols, this alone is not enough to ensure successful modification. The main feature of successful FA modification relies on the ability to extend the FA molecule. In this regard, the concentration of phenolic precursors can affect the accessibility of active sites (available –COOH and phenolic –OH groups) on the FA molecular chain. A too high concentration of phenolic precursors leads to formation of massive condensation products that could block addition to active sites of FA molecule. In this scenario, the formation of separate condensation products derived from PG and GA condensation is possible. This alternative polymerization of the mono-molecular precursors when appearing in parallel with the FA modification is expected to result in a broad or even bimodal molecular weight distribution seen in the SEC elugrams. However, the SEC traces illustrate relatively narrow size distributions typical for polycondensations with one maximum and the absence of any significant residual shoulders for all modified samples (Fig. 1a), disregarding the phenolic precursor concentrations. The gradual increase of molecular weights alongside a stable polydispersity index remaining within the range of 1.2–1.4 (see Fig. 1b) suggests a progressive extension of FA molecules without formation of separated oligomeric or polymeric condensed phenolic matter in reaction media.
 |
| Fig. 1 Modification of commercially available FA with PG and GA: (a) SEC chromatograms (RI detector) in 0.067 mol l−1 Na2HPO4 in bidestilled-H2O; (b) number-average molecular weight (Mn), weight-average molecular weight (Mw), size-average molecular weight (Mw) and dispersity index (PDI = Mw/Mn). | |
Following the FA modification with UV-vis spectroscopy
An important feature of the proposed modification process is the possibility to perform the reaction at either 60 °C during 24 hours leading to the rapid condensation or at room temperature resulting in slightly lingering condensation. Both reaction conditions are mild and thus endow green chemistry attributes to this process.
The reaction kinetics of VT11 run at 60 °C was studied with UV-VIS spectroscopy (see Fig. 2a). An increase of the peak at ∼450 nm was observed. This illustrates the change of the solution color from slightly yellow, mainly attributed to FA absorption, to orange (see Fig. 2a inset). A consistent and distinct pattern of color change is observed across all experiments (VT11–VT35). The initially transparent solutions gradually transform into shades of dark orange and brown hues, which serves as a clear indication of the progression of the condensation reaction (see Fig. 2b). This color evolution is attributed to the formation of a new phenolic-resin-based chromophore, resulting from the condensation of the phenolic precursors. The emerging chromophore exhibits absorption in the visible region, with the maximum absorbance observed at approximately 450 nm.38 This phenomenon provides valuable evidence of the chemical transformation occurring during the condensation process, highlighting the successful formation of the desired phenolic resin structure. The consistent color changes observed across the experimental variations further reinforce the reliability and reproducibility of the observed phenomenon. The gradual shift towards longer wavelengths, specifically to 462 nm after 40 minutes, represents a notable bathochromic shift observed in the absorption spectra. This shift can be attributed to the charge transfer, as FAs are oxidized and electron poor, while phenols are reduced and electron rich. A phenomenon that has not been previously reported in the literature when PG and GA were condensed independently.38 This observation also provides further validation and support to the data obtained from GPC.
 |
| Fig. 2 (a) Kinetics of the VT11 condensation monitored by UV-VIS spectroscopy at 60 °C during the first 40 minutes. (b) The visual appearance of the reaction mixtures before the reaction and after 1 hour of condensation; in each group of three samples FAs ratio increases according to the first number; the PP ratio in each series is increased according to the second number. | |
Solubility tuning via FA modification
The FA after extension with phenolic precursors was analyzed by 1H NMR (see Fig. 3). The FA modified using the open-vial protocol (OVP) exhibited already visual reduction in solubility compared to the initial FA. An especially drastic difference was observed for the samples prepared with excess of phenolic precursors in VT15 and VT25 runs (see Fig. 4a). This decreased solubility is due to the formation of more cross-linked matter from PG and GA when higher ratios are employed (90 wt% and 81 wt% of phenolic precursors for VT15 and VT25, respectively). Solvent evaporation promotes gradual increase of PG and GA local concentrations. The increased GA concentration during evaporation of solvent causes a decrease of pH of the reaction mixtures, and as a consequence, more cross-linked matter forms.
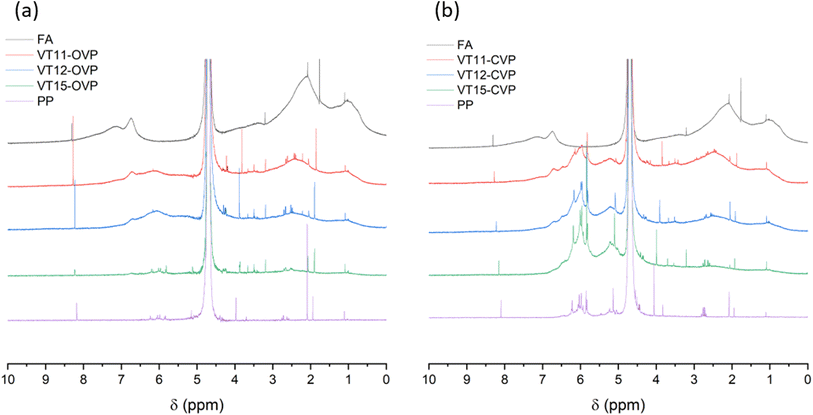 |
| Fig. 3 Series of 1H NMR spectra of final products: FA, VT11, VT12, VT15 and PG + GA (phenolic precursors alone – PP). (a) Open-vial protocol (VT11-OVP, VT12-OVP and VT15-OVP). (b) Closed-vial protocol (VT11-CVP, VT12-CVP and VT15-CVP). | |
 |
| Fig. 4 (a) Visual demonstration of the limited solubility of FA modified with phenolic precursors in the open-vial protocol (OVP) for runs VT15 and VT25. (b) Visual appearance of the final modified products obtained through the closed-vial protocol (CVP) and OVP for the VT15 run. (c) Quantified solubility test of OVP runs, presenting the percentage of dissolved modified FA after filtration of the 1 wt% solution through a 200 nm filter. | |
To verify this suggestion, modification of FA has been performed in a CVP (VT11-CVP, VT12-CVP and VT15-CVP) where the change of PG and GA concentrations is avoided. The solubility test revealed similar solubility of FA modified in the CVP in comparison with initial commercial aquatic FA. (see Fig. 4b – absence of residual particles unlike OVP samples). In contrast, solubility test confirmed change in solubilities of the OVP modified FA samples (see Fig. 4c). The drastic decrease of solubility has been observed for VT15 and VT25 runs reaching 16.4% and 37.8% compared to the referent solution, respectively. These runs result in modified products with 10 and 19 wt% of FA content, respectively. All other runs result in the products with 23 wt% (VT12) or higher FA content possessing no more than 10% solubility decrease. It means that it is possible to tailor the solubility in the application relevant range by changing the FA content in the final product from 10 to 23 wt% of FA. Such modification could endow fertilizing material with tunable runoff ability providing controllable time of FA fertilizing activity in the direct proximity of the plant root system that is hardly achievable with conventional FA.
The formation of more cross-linked aromatic matter is also traceable within NMR data (Fig. 3). The peak at 8.29 ppm corresponds to protons in aromatic systems with few electron-withdrawing substituents such as –COOH, –Ph etc. When introducing 1 and 2 eq. of phenolic precursor the formation of a new aromatic network occurs, and an increased aromatic proton signal (8.29 ppm) derived from condensed PG is observed (see Fig. 3a runs VT11 and VT12). In VT15 run the concentration of GA is higher, and also the lower pH promotes more efficiently the condensation process, resulting in formation of more cross-linked aromatic matter (see Scheme 2a). For comparison, the CVP reveals constant intensity of the peak at 8.29 ppm for runs with different concentration of phenolic precursors (see Fig. 3b), that is only a constant amount of phenol reacts with FA.
 |
| Scheme 2 Proposed pathways of FA modification with PP through OVP and CVP. (a) The formation of more cross-linked matter with decreased solubility promoted by solvent evaporation through OVP. (b) The formation of more branched structure with no change of solubility through CVP modification of FA. | |
Considering other spectral regions, the level of condensation can also be evaluated using the peak around 4 ppm. This signal corresponds to the methylene bridge derived from mono-reacted GA. The decrease of this signal in OVP in VT15 run means that more methylene units were converted to tertiary carbon through electrophilic aromatic substitution giving rise to carboxylic acid bridges between the benzene rings compared to runs VT11 and VT12 (see Scheme 1), i.e. polycondensation is more efficient. The modification of FA through CVP results in comparable signal intensities at 4 ppm, indicating that the predicted reactions are run in similar way regardless FA to PP ratio, but forming more soluble final products. The aliphatic and oxygenated aliphatic region have similar general pattern meaning that well-soluble parts represented by oxygenated and not-oxygenated alkyl chains do not take part in the phenolic polycondensation processes.
Solid-state characterization via13C-CPMAS-NMR
The 13C CP-MAS NMR spectra of OVP modified (VT11 and VT15) FA and the initial FA are presented in Fig. 5. The spectrum of FA without modification exhibits the common pattern for this class of compounds with broad signals in the O-alkyl (45–90 ppm) and alkyl (0–45 ppm) regions.39 Within this region, the peak at 82 ppm corresponds to the overlapping resonances of carbon atoms in the pyranoside unit and is commonly observed in holocellulose-like structures. Broad peaks in 90–165 ppm region indicate that aromatics are also present in high quantities. In case of FA these aromatics are mostly derived from lignin and after modification of VT11 and VT15 runs from PG. Signals at 170 ppm correspond to carboxylic groups derived from initial FA and at 190 ppm region from GA after the modification process.40 These 4 distinct spectral regions can be divided into 2 groups to access solubility properties. Alkyl (0–45 ppm) and aromatic (90–165 ppm) regions are mostly responsible for hydrophobicity, while O-alkyl (45–90 ppm) and carboxylic carbon (170–190 ppm) for hydrophilicity. Relative integrals are presented in Table 1. It is possible to estimate the hydrophobic/hydrophilic ratio (HHR) of initial FA and modified ones.41 The gradual HHR increase from initial FA to modified through VT11 and VT15 runs from 1.33 to 3.99 and 6.07, respectively. This is in a good agreement with the abovementioned assumption that thermal condensation of phenolic derivatives with FA can tune not only solubility but also changes HHR towards more hydrophobic behavior. Increased hydrophobicity is also traceable within a van Krevelen diagram (see ESI: Fig. S2†) based on elemental analysis. It illustrates that the condensation process with PG works through dehydration. This results in the formation of less oxygenated materials. This dehydration reaction path is a common feature when humic acids and FAs from the same source are investigated.42 Thereby, FA modification with PP provides a model tool to analyze the structural maturation of FA towards structures similar to humic acids.
 |
| Fig. 5
13C-CPMAS-NMR spectra of modified (VT11 and VT15) FA through OVP and initial FA. | |
Table 1 Distribution of C relative intensity in different regions (ppm) of 13C-CPMAS-NMR spectra of FA, VT11 and VT15 runsa
Sample |
13C chemical shift (ppm) |
0–45 |
45–90 |
90–165 |
170–190 |
HHB |
HHB = hydrophobic/hydrophilic ratio. .
|
FA |
1.03 |
1.01 |
1.64 |
1 |
1.33 |
VT11 |
0.96 |
0.38 |
4.54 |
1 |
3.99 |
VT15 |
1.14 |
0.19 |
6.08 |
1 |
6.07 |
Germination assay
To exclude the phytotoxicity of the modified FAs, synthesized through OVP, a germination assay on Lepidium sativum seeds was carried on. Four different set of experiments were performed on Petri dishes filled with sandy soil: distilled water, initial FA, VT11 and VT15. Three different concentrations were tested for the FA, VT11 and VT15 samples (low, 0.001 wt%; mid, 0.0055 wt% and high, 0.01 wt%). Preliminarily, all the samples were applied to the soil and the diffusion was visually assessed (see Fig. 6. The applied solutions of both modified and initial FA can be observed on the white tray, see ESI: Fig. S3†). The modified compounds VT11 and VT15 could be easily detected visually as the condensed phenolic matter increased their chromophore properties. For instance, VT11 and VT15 were clearly distinguishable as dark brown halos on the soil surface as increasing concentrations were applied. The solubility of VT15, as low as 16.4%, is manifested in the darker and bigger spots left on the soil compared to those of VT11 samples (solubility of 88.5%) at the respective concentrations, and is observed to penetrate just few millimeters in depth of soil layers, up to 1–2 cm for the highest concentration. Conversely, initial FA is completely soluble, but its diffusion can just be assumed to be quite similar to VT11, since it was not visually detectable. To better visualize the distribution of the modified FA in the sandy soil, a tenfold increased concentration of modified FAs was applied (see ESI: Fig. S4†). The excess of the FAs showed no negative effect on the seeds but visually confirmed slower run off trough the soil in accordance with the changed solubility and the higher mineral adsorption.
 |
| Fig. 6 Visual assessment of the solubility of the compounds. For each sample, a 10 μL drop was positioned 0.5 cm below the dashed white line. The photograph was captured 5 minutes after the samples were applied to the soil. The control sample was represented by distilled water (Ctr). | |
The germination assay highlighted little differences in root length (Fig. 7a), shoot length (Fig. 7b) or germination (Fig. 7c) among treatments, but showed similar values compared to the control in all cases but one. For instance, the shoot length of seedlings grown with the highest concentration of VT15 are 15% shorter than the control, although the root length is not significantly influenced. All the other modes of applications of the compounds do not affect seedling growth directly when compared to the control; moreover, there were not evident patterns suggesting dose responses for FA and VT11 while VT15 treated plants resulted in slightly longer roots and shoots when lower concentrations were applied. The germination, however, was not significantly affected among the applied VT15 doses, nor it is in the broader comparison among treatments. We can therefore conclude that the compounds VT11, at all concentrations, and VT15, for the low and intermedium concentration, do not show any phytotoxic effect, while the highest concentration of VT15 moderately decreases root length.
 |
| Fig. 7 Cress germination assay with application of FA, VT11 and VT15. Root length (a), shoot length (b) and germination percentage (c) of cress seedlings grown for 72 hours in soil treated with water (Ctr), a commercial FA precursor, or its modified version (VTT11 and VTT15) applied at three increasing concentrations (low – 0.001%; med – 0.005%; high – 0.01%). Treatments were supplied adding 10 μL of the samples directly underneath the seed. The graphs present merged data from three independent replicates: mean values per plant are reported in (a) and (b) (n ≥ 74); mean values of the three separate Petri dishes are reported in (c) (n = 3). Bars represent the standard error of the mean (SEM). A one-way ANOVA with Tukey's post hoc test was performed, p < 0.05; significant differences are indicated by different letters. | |
It is well known and multiply described in scientific literature that FA play an essential role in soil fertility and plant nutrition, and improve plant growth.43 Fulvic acid positively affects a large number of plant physiological processes, such as the uptake and leaf allocation of nitrate and other important ions,44–46 the chelation of micronutrients47 and the rhizosphere acidification.48 Fulvic acid also improve soil structure, water retention,49 and microbial activity,50 thus contributing to soil fertility. These features possibly all can be enhanced and tuned by modifications of the functional groups of FA, or by the addition of new ones.51,52 Our results suggest that modifying a FA with phenolic compounds can increase the hydrophobicity of the aquatic FAs, resulting in a decreased solubility that could prevent run-off without compromising plant growth when supplied at low doses.
Conclusions
A simple and efficient approach for the modification of natural aquatic fulvic acid (FA) is demonstrated in this work to tune solubility performance with model phenolic precursor and environmentally friendly glyoxylic acid. The formation mechanism of such structures was studied by GPC, NMR and CPMAS. Different FA to phloroglucinol and glyoxylic acid ratios for the modification process have been investigated through open-vial protocol (OVP). A significant reduction in solubility was observed in runs with 10- and 5-fold decreased FA content, demonstrating a 6- and 3-fold decrease in solubility, respectively, compared to unmodified FA. All other runs in OVP result in the products with 23 wt% or higher of FA content possessing ∼10% solubility decrease. To summarize, the change of solubility performance in water of the synthesized materials through OVP can be controlled by tuning the experimental conditions (FA content). The samples were then applied to a model soil. Initial aquatic FA without modification possesses complete solubility, and it leaks rapidly to the bottom layers of soil. In case of barely soluble FA (VT15, content of FA = 16.4 wt%) retention at the top soil layers was observed. VT11 (content of FA = 37.8 wt%) possesses slightly lower solubility (88.5% of the initial aquatic FA solubility) and illustrates the excellent balance of retention/leakage features. The germination assay suggests that these compounds are not phytotoxic and encourages new investigations on their fertilizing effects. In summary, the demonstrated method offers a sustainable route to modify natural aquatic fulvic acid, enhancing its solubility properties for soil improvement, without the need for catalysts, and through a straightforward, efficient process that holds promise for industrial scalability and environmentally friendly applications.
Author contributions
Vitalii Tkachenko: conceptualization, data curation, formal analysis, investigation, methodology, visualization, writing – original draft. Stefano Ambrosini: data curation, formal analysis, investigation, visualization, writing – original draft. Nader Marzban: formal analysis, data curation. Ashish Pandey: conceptualization, writing – review & editing. Sarah Vogl: data curation, formal analysis. Markus Antonietti: project administration, resources, supervision, validation, writing – review & editing. Svitlana Filonenko: conceptualization, project administration, resources, supervision, validation, writing – original draft, writing – review & editing.
Conflicts of interest
There are no conflicts to declare.
Acknowledgements
This research did not receive any specific grant from funding agencies in the public, commercial, or not-for-profit sectors. VT, SA, NM, MA and SF gratefully acknowledge the Max Planck Society for financial support. SV is grateful for the funding by the Deutsche Forschungsgemeinschaft (DFG, German Research Foundation) under Germany's Excellence Strategy – EXC 2008 – 390540038 – UniSysCat.
References
- A. Nebbioso and A. Piccolo, Anal. Chim. Acta, 2012, 720, 77–90 CrossRef CAS PubMed.
- F. Yang and M. Antonietti, Prog. Polym. Sci., 2020, 100, 101182 CrossRef CAS.
-
M. Hayes, R. Swift, C. Byrne, G. Song and A. Simpson, 2010.
-
F. J. Stevenson, Humus Chemistry: Genesis, Composition, Reactions, John Wiley & Sons, 1994 Search PubMed.
- A. Zavarzina, N. Danchenko, V. Demin, Z. Artemyeva and B. Kogut, Eurasian Soil Sci., 2021, 54, 1826–1854 CrossRef CAS.
- J. Shan, A. Brune and R. Ji, Soil Biol. Biochem., 2010, 42, 1455–1462 CrossRef CAS.
- M. H. Hayes, R. Mylotte and R. S. Swift, Adv. Agron., 2017, 143, 47–138 Search PubMed.
- C. Beer, M. Reichstein, E. Tomelleri, P. Ciais, M. Jung, N. Carvalhais, C. Rödenbeck, M. A. Arain, D. Baldocchi and G. B. Bonan, Science, 2010, 329, 834–838 CrossRef CAS PubMed.
- C. B. Field, M. J. Behrenfeld, J. T. Randerson and P. Falkowski, science, 1998, 281, 237–240 CrossRef CAS PubMed.
- R. Yao, J. Yang, W. Zhu, H. Li, C. Yin, Y. Jing, X. Wang, W. Xie and X. Zhang, Plant Soil, 2021, 464, 539–558 CrossRef CAS.
- S. Wei, Z. Li, Y. Sun, J. Zhang, Y. Ge and Z. Li, Renewable Sustainable Energy Rev., 2022, 170, 112984 CrossRef CAS.
- N. Taherkhani, A. Hekmat, H. Piri and K. Haghbeen, J. Food Biochem., 2022, 46, e14279 CrossRef CAS PubMed.
- G. Huang, L. Huang, C. Geng, T. Lan, X. Huang, S. Xu, Y. Shen and H. Bian, Int. J. Biol. Macromol., 2022, 219, 767–778 CrossRef CAS PubMed.
- G.-Q. Gong, Y.-F. Zhao, Y.-J. Zhang, B. Deng, W.-X. Liu, M. Wang, X. Yuan and L.-W. Xu, Fuel, 2020, 267, 117210 CrossRef CAS.
- F. Gao, Z. Li, Y. Du, J. Duan, T. Zhang, Z. Wei, L. Guo, W. Gong, Z. Liu and M. Zhang, Agronomy, 2022, 12, 1400 CrossRef CAS.
- S. Li, K. Zhang, J. Tian, K. Chang, S. Yuan, Y. Zhou, H. Zhao and F. Zhong, Environ. Sci. Pollut. Res., 2023, 30, 28780–28790 CrossRef CAS PubMed.
- T. Lieke, C. E. Steinberg, T. Meinelt, K. Knopf and W. Kloas, Sci. Rep., 2022, 12, 5886 CrossRef CAS PubMed.
- M. Takó, E. B. Kerekes, C. Zambrano, A. Kotogán, T. Papp, J. Krisch and C. Vágvölgyi, Antioxidants, 2020, 9, 165 CrossRef PubMed.
- U. Pingali and C. Nutalapati, Phytomedicine, 2022, 105, 154334 CrossRef CAS PubMed.
- M. Anwer, S. P. Agarwal, A. Ali and Y. Sultana, J. Inclusion Phenom. Macrocyclic Chem., 2010, 67, 209–215 CrossRef CAS.
- M. Wu, M. Song, M. Liu, C. Jiang and Z. Li, Sci. Rep., 2016, 6, 1–10 CrossRef PubMed.
- H. V.-M. Nguyen, H.-S. Lee, S.-Y. Lee, J. Hur and H.-S. Shin, Sci. Total Environ., 2021, 790, 148142 CrossRef CAS PubMed.
- V. Pallier, G. Feuillade-Cathalifaud, B. Serpaud and J.-C. Bollinger, J. Colloid Interface Sci., 2010, 342, 26–32 CrossRef CAS PubMed.
- M. Makunina, I. Pozdnyakov, V. Grivin and V. Plyusnin, High Energy Chem., 2014, 48, 197–201 CrossRef CAS.
- S. Jayaganesh and V. Senthurpandian, Asian J. Earth Sci., 2010, 3, 130–135 CrossRef CAS.
- Y. V. Zhernov, A. I. Konstantinov, A. Zherebker, E. Nikolaev, A. Orlov, M. I. Savinykh, G. V. Kornilaeva, E. V. Karamov and I. V. Perminova, Environ. Res., 2021, 193, 110312 CrossRef CAS PubMed.
- F. Yang, S. Zhang, Q. Fu and M. Antonietti, Land Degrad. Dev., 2020, 31, 884–893 CrossRef.
- Q. Hua, Z. Nie, Y. Luo, X. Feng, B. Wang, J. Tang and Y. Liu, Chem. Pap., 2022, 76, 203–211 CrossRef CAS.
- H. Zhang and W. Zhen, Int. J. Biol. Macromol., 2019, 139, 181–190 CrossRef CAS PubMed.
- L. Liu, W. Fang, M. Yuan, X. Li, X. Wang and Y. Dai, J. Environ. Chem. Eng., 2021, 9, 106238 CrossRef CAS.
- V. Tkachenko, N. Marzban, S. Vogl, S. Filonenko and M. Antonietti, Sustainable Energy Fuels, 2023, 7, 769–777 RSC.
- C. M. Ghimbeu, L. Vidal, L. Delmotte, J.-M. Le Meins and C. Vix-Guterl, Green Chem., 2014, 16, 3079–3088 RSC.
- J. Sidana, W. J. Foley and I. P. Singh, Phytochem. Anal., 2012, 23, 483–491 CrossRef CAS PubMed.
- K. W. Glombitza, S. Hauperich and M. Keusgen, Nat. Toxins, 1997, 5, 58–63 CrossRef CAS PubMed.
- F. Yang and Y. Cao, Appl. Microbiol. Biotechnol., 2012, 93, 487–495 CrossRef CAS PubMed.
- R. A. Sheldon, ACS Sustain. Chem. Eng., 2018, 6, 32–48 CrossRef CAS.
- S. Bagherifam, T. C. Brown, S. Bagherifam and A. Baglieri, Environ. Pollut., 2023, 327, 121610 CrossRef CAS PubMed.
- M. Sopronyi, F. Sima, C. Vaulot, L. Delmotte, A. Bahouka and C. Matei Ghimbeu, Sci. Rep., 2016, 6, 1–13 CrossRef PubMed.
- R. Spaccini and A. Piccolo, Soil Biol. Biochem., 2009, 41, 1164–1172 CrossRef CAS.
- R. Baigorri, M. Fuentes, G. Gonzalez-Gaitano, J. M. Garcia-Mina, G. Almendros and F. J. Gonzalez-Vila, J. Agric. Food Chem., 2009, 57, 3266–3272 CrossRef CAS PubMed.
- N. O. Aguiar, F. L. Olivares, E. H. Novotny, L. B. Dobbss, D. M. Balmori, L. G. Santos-Júnior, J. G. Chagas, A. R. Façanha and L. P. Canellas, Plant Soil, 2013, 362, 161–174 CrossRef CAS.
- J. A. Rice and P. MacCarthy, Org. Geochem., 1991, 17, 635–648 CrossRef CAS.
- B. Rauthan and M. Schnitzer, Plant Soil, 1981, 63, 491–495 CrossRef CAS.
- N. Tomasi, T. Mimmo, R. Terzano, M. Alfeld, K. Janssens, L. Zanin, R. Pinton, Z. Varanini and S. Cesco, Biol. Fertil. Soils, 2014, 50, 973–982 CrossRef CAS.
- M. Nikolic, S. Cesco, R. Monte, N. Tomasi, S. Gottardi, A. Zamboni, R. Pinton and Z. Varanini, BMC Plant Biol., 2012, 12, 1–12 CrossRef PubMed.
- L. Zanin, N. Tomasi, S. Cesco, Z. Varanini and R. Pinton, Front. Plant Sci., 2019, 10, 675 CrossRef PubMed.
- A. Zamboni, L. Zanin, N. Tomasi, L. Avesani, R. Pinton, Z. Varanini and S. Cesco, BMC genomics, 2016, 17, 1–17 CrossRef PubMed.
- R. Pinton, S. Cesco, S. Santi and Z. Varanini, J. Plant Nutr., 1997, 20, 857–869 CrossRef CAS.
- H. Khaled and H. A. Fawy, Soil Water Res., 2011, 6, 21–29 CrossRef CAS.
- Q. Ning, S. Hättenschwiler, X. Lü, P. Kardol, Y. Zhang, C. Wei, C. Xu, J. Huang, A. Li and J. Yang, Global Change Biol., 2021, 27, 5976–5988 CrossRef CAS PubMed.
- A. B. Volikov, V. A. Kholodov, N. A. Kulikova, O. I. Philippova, S. A. Ponomarenko, E. V. Lasareva, A. M. Parfyonova, K. Hatfield and I. V. Perminova, Catena, 2016, 137, 229–236 CrossRef CAS.
- O. I. Klein, N. A. Kulikova, A. I. Konstantinov, M. V. Zykova and I. V. Perminova, Polymers, 2021, 13, 3262 CrossRef CAS PubMed.
|
This journal is © The Royal Society of Chemistry 2024 |