A green process for the specific decomposition of chicken feather keratin into polythiol building blocks†
Received
4th August 2023
, Accepted 23rd November 2023
First published on 7th December 2023
Abstract
Defined peptides with exclusive molecular functionalities from biomass streams provide an untapped treasure for innovative biogenic specialty chemicals and materials. In this context, feather keratin, a natural structural protein with high L-cysteine content, enables access to polythiol-containing peptides, which can be used as matrix compounds for new materials per se and be specifically modified via their amino and acid moieties. This study describes an innovative two-step approach for tailored feather keratin fragmentation involving selective enzymatic hydrolysis followed by optional chemical reduction. Several proteases were investigated to serve as a benchmark for the decomposition of chicken feather keratin, and we succeeded in the controlled decomposition of chicken feathers using trypsin and other specific proteases, producing polythiol-containing peptide fragments. We were able to implement a green hydrolysis process without the need for any denaturants or reducing agents and achieved yields of soluble protein up to 81% (w/w) and thiol concentrations up to 21 mmol L−1. The obtained hydrolysates were used to produce peptide films, and the scalability of the newly developed hydrolysis process has been demonstrated in 25 L batch reactions.
Sustainability spotlight
The transition of the chemical industry from fossil-based to sustainable feedstocks is one of the key challenges today. Especially biopolymers provide unique access to exclusive building blocks that can be functionally integrated into specialty chemicals. A widely untapped source for this approach are structural proteins obtained from food processing side streams. We propose a new method for the controlled hydrolysis of feathers into keratin-based polythiol-peptides, which can be used for various applications. Keratin depolymerisation is achieved at moderate temperatures without harmful substances and can be carried out using bio-based chemicals. Our work aligns with the UN sustainable development goals 9 (industry, innovation, and infrastructure) and 12 (responsible consumption and production).
|
1 Introduction
The transition from fossil-based raw materials to renewable resources for use in the chemical industry, especially for materials sciences, is a crucial technological task for developing a sustainable future.1 Such approaches offer many opportunities since different raw materials also extend the availability of synthesis space, which can lead to new functional properties of chemical products (bio-enhanced properties) and are in alignment with strategic sustainability goals. Among renewable resources, keratins are essential as bio-based and biodegradable macromolecules with unique molecular features. Still, they have yet to be explored as a platform for molecular building blocks. As natural polyamides, they harbour exclusive peptide fragments that can be isolated via mild hydrolysis and specifically used for chemical synthesis. Chicken feathers represent a homogeneous and highly enriched protein source comprising about 90% (w/w) keratin.2–4 The selective, mild, and atom economic hydrolysis of feather keratin yielding defined keratin fragments is challenging because of the keratin composition and its compact structure.
Keratin is a protein that assembles in mechanically robust and chemically resistant structures with hydrophobic properties. Almost all keratogenic materials, such as feathers, hair, bristles, hooves, nails, or wool, consist of mixtures of α-keratin and β-keratin.5 The latter predominantly comprises β-folded sheets, while α-keratins form mainly α-helical structures.6 Feathers consist of 41–67% α-keratin, 33–38% β-keratin, and amorphous keratin components.5 Feather keratins have a molecular weight of about 10 kDa.7 About 7% of the amino acids contained are L-cysteine.4
Generally, avian and reptilian hard keratins are composite materials with a filament matrix texture.4,8 Crosslinking is accomplished through chemical bonding by disulfide crosslinks between fibre and matrix.8 The amino acid sequences of hard keratins contain three distinct domains: An N-terminal 23-residue long L-cysteine-rich domain, a central domain consisting of 34 amino acids and rich in β-favouring residues, which is highly conserved in a wide variety of avian and reptilian keratin molecules, and a C-terminal domain of variable length and composition.9
Keratins thus represent an outstanding raw material source for preparing bio-originated polythiols, which may benefit many areas and improve otherwise sustainable chemistries. And the demand is huge: the global market for polythiols is estimated at US$ 243.30 million in 2023, with an estimated increase to US$ 360.20 million by 2033, representing a compound annual growth rate (CAGR) of 4%.11 In the adhesives sector, among others, many new developments would benefit from suitable bio-based polythiols. In a study of mussel-inspired adhesives, Krüger et al. built thiol-catechol polymers which were fully biobased except for the thiol component.12 Horsch et al. described a method for creating adhesive protein analogues through enzyme-induced thiol-quinone-polymerization of peptides utilising free thiols of cysteine-residues, resulting in artificial proteins with solid adhesion properties.13 Another biopolymer that can yield promising materials through thiol–ene crosslinking chemistry is lignin.14,15
In 2018, the global poultry industry was estimated to produce 12 million tons of feather biomass.2 The countries of the European Union account for more than 3 million tons of feather biomass per year.16 Chicken feathers can only be poorly thermally recycled due to the high sulfur content of the incorporated cysteine moieties17 and the environmental and health considerations associated with the incineration of poultry-derived wastes due to the formation of NOx, SOx and H2S.18,19 Instead, waste feathers are mainly processed into low-value animal feed ingredients, landfilled, composted3,16 or on a smaller scale, processed into fertilizer20 or biodegradable surfactants.17
Notably, this animal biomass must be considered within ethical contexts and the respective regulations for further industrial use, in particular, the EU Regulation (EC) No 1774/2002.21
Recently, a variety of new applications for chicken feather keratin (CFK) have been developed that involve their use for electrical and electronic applications, bio-fertilizers, films, absorbents, composite materials, flame retardants, production of keratinases, nanoparticles, thermoplastics, textile yarn sizing agents, and regenerated protein fibres.22 One of the most recent advancements is the treatment of feather keratin in natural deep eutectic solvents (DES), yielding high and low molecular weight fractions that can each be used for film formation.23 In this context, feather keratin represents an emerging biogenic protein source with a unique molecular pattern that can serve as a starting point for synthesising specialty peptides that possess polythiol moieties and enable the synthesis of target products with new functional properties.
Despite the proven examples, no defined feather keratin-based peptides are available as platform intermediates that can be used for synthetic diversification. This is mainly caused by a lack of mild hydrolysis protocols that agree with the principles of sustainable and green chemical processing.24
In this work, we aimed to develop a mild, selective, and modular hydrolysis protocol for chicken feathers, generating thiol-rich keratin hydrolysis peptides (KHPs) that potentially serve as building blocks for the development of innovative polymers,25 enzyme-responsive materials,26 and as hardeners for established polymer materials such as thiol–ene- 27–29 and epoxy-systems,30,31 polyurethanes, or for orthogonal grafting strategies in the development of novel peptide-based materials, among other applications.
Hence, producing well-defined keratin hydrolysis peptides as platform chemicals that can be functionally integrated into polymers would represent a hallmark in the valorisation of keratin biomass and for developing novel functional materials.
1.1 Feather hydrolysis – state of the art
The hydrolysis of chicken feathers is extensively described for diverse approaches and under various conditions. Representative hydrolysis strategies are listed in Table 1. These protocols predominantly use unspecific peptide bond cleavage and reduction of disulfide bonds, mainly leading to undefined keratin fragments of low molecular weights. Additionally, most of these conversions include denaturing agents, harmful reductants, unspecific enzymes, high enzyme loading, high salt concentrations, the formation of adducts such as Bunte-salts (S-alkyl- and S-arylthiosulfates),32,33 and harsh reaction conditions at elevated temperatures.
Table 1 Representative hydrolysis protocols of keratins of different origins
Approach |
Keratin treatment |
References |
Year |
Trypsin catalysed hydrolysis |
Non-reductive hydrolysis without protein denaturing agents, selective hydrolysis, 2-step process with optional reduction of disulfides |
This study |
2023 |
Chemical reduction |
Reductive cleavage of keratin disulfide bridges |
Wang et al.34 |
2016 |
Xu et al.4 |
2014 |
Poole et al.7 |
2011 |
Maclaren et al.35 |
1981 |
O'donnell and Thompson36 |
1964 |
Goddard et al.37 |
1935 |
Incubation in ionic liquids (IL) and deep eutectic solvents (DES) |
Keratin is dissolved in IL or DES, which are environmentally acceptable and capable of keratin disintegration |
Nuutinen et al.2 |
2019 |
Jiang et al.38 |
2018 |
Idris et al.39 |
2014 |
Physical explosion |
Keratin is treated with steam under high pressure and temperature with water vapour, which is then released over a brief period, yielding denatured keratin with cleaved disulfide bridges |
Yu et al.40 |
2012 |
Zhao et al.41 |
2012 |
Microwave treatment |
Microwave irradiation can have a similar effect on keratin as a physical explosion |
Zoccola et al.42 |
2012 |
Combined microbial and enzymatic hydrolysis |
Keratinolytic organisms or respective hydrolytic enzymes and mixtures of keratin hydrolysates are available |
Burtt et al.43 |
1999 |
Lin et al.44 |
1999 |
Ramnani and Gupta45 |
2007 |
Lange et al.5 |
2016 |
Chemical oxidation |
Use of peracids for the decomposition of keratin |
Earland et al.46 |
1955 |
Alkaline hydrolysis |
Hot alkaline solutions are used to dissolve wool keratin |
Blackburn et al.47 |
1956 |
1.2 Development of a mild and selective hydrolysis protocol for feather keratin
Herein, we describe a novel two-step process for mild hydrolysis of native CFK under mild conditions, involving a site-specific protease within aqueous buffer systems and subsequent reduction to obtain protein hydrolysates containing polythiol peptides with an average functionalisation of two or more free thiol moieties per fragment (Fig. 1).
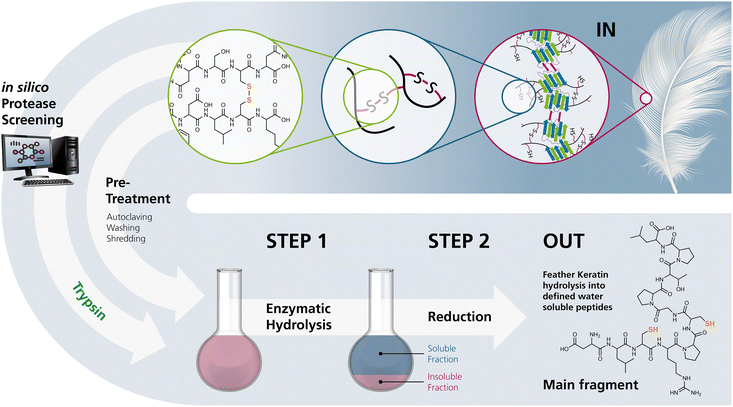 |
| Fig. 1 The process developed in this study for the selective hydrolysis of pretreated chicken feathers yielding polythiol-containing oligomers. Selective hydrolysis yields products that can either directly be processed or reduced on demand prior to processing for further use. (The keratin structure in the upper right was taken from Jabbari, 2019.10) | |
To our knowledge, such biocatalytic hydrolysis of feathers with high selectivity before a separate reduction step yielding defined higher molecular weight oligomers that bear access to a specified number of free thiol moieties has not been reported.
Extensive chemical and enzymatic hydrolysis approaches had shown very good degradability of chicken feathers after pretreatment by autoclaving at elevated temperature and pressure. Still, these strategies led unselectively to small keratin fragments and single amino acids. To identify appropriate keratinolytic enzymes for controlled hydrolysis, an in silico analysis of digestion products of feather keratin was performed using the Expasy Peptide Cutter tool48 based on selected specific protease recognition sites. The peptide pattern obtained by in silico analysis identified trypsin as an enzyme candidate yielding the most promising polythiol-containing peptides (Fig. S2†). Then, hydrolysis studies were carried out on an experimental basis, following a matrix approach in different buffers and under non-denaturing conditions at moderately elevated temperatures. After removing insoluble residues and freeze-drying, the KHPs were applied in film formation experiments without further purification.
2 Experimental
2.1 Materials
Chicken feathers were provided by a local poultry meat producer and stored at −20 °C until further treatment after sterilisation. All required chemicals and enzymes were purchased from Carl Roth GmbH & Co. KG, Karlsruhe (Germany), or Merck KGaA, Darmstadt (Germany). Proteases A-01 and S-02 were purchased from ASA Spezialenzyme GmbH, Wolfenbüttel (Germany). Details on the used enzymes are summarised in the ESI.† An unstained protein molecular weight standard from New England Biolabs, Ipswich, USA (order no. P7717) was used for SDS-PAGE. The main chemicals for the process were selected so that they were either derived from renewable resources or could be reused or recycled. Trypsin, purchased from Merck KGaA, Darmstadt, was derived from porcine pancreas.
2.2 Pretreatment of chicken feathers
Pretreatment included sterilisation, cleaning, drying, and shredding. Chicken feathers were sterilised by autoclaving at 121 °C at 2 bar in a steam-saturated atmosphere for 20 min. After autoclaving, feathers were washed using a protease-free basic detergent and dried in a standard washer-dryer under mild conditions at a washing temperature of 30 °C. Dried feathers were shredded using a blender and stored in a sealed plastic bag at room temperature. We confirmed sterility by incubating autoclaved feathers at 21 °C, 37 °C, and 50 °C for 24 h with gentle shaking in lysogeny broth-medium. No turbidity of the cultures was observed, which would indicate microbial growth.
2.3 Development of a new hydrolysis protocol for chicken feather keratin
To develop a new protocol for controlled chicken feather decomposition, existing protocols were tested as benchmarks and served as a basis for materials handling and defining the new protocol. This approach also offered the possibility to benchmark the hydrolysis experiments, which accounts for standardisation that needs to be improved in the development of biomass conversion.
2.3.1 Chemical hydrolysis of chicken feathers.
Following a method described by Xu and Yang,4 a process for the reductive decomposition of chicken feathers was implemented. Therefore, 0.5 g of pretreated chicken feathers were mixed in 50 mL centrifuge tubes with 8.5 mL of a reaction solution consisting of 8 mol L−1 urea and 50 mmol L−1 reducing agent. The reaction batch was incubated under agitation (150 rpm) for up to 19 h at 70 °C. Subsequently, the supernatant was centrifuged at 4142×g at room temperature for 20 min to remove insoluble particles and stored at 4 °C for further processing. The insoluble fraction was washed with desalted water, dried at 40 °C in vacuo, and weighed.
2.3.2 Hydrothermal hydrolysis.
A pressure reaction vessel of 45 mL size was filled with 2.5 mL desalted water and 0.1 g pretreated chicken feathers. The vessel was sealed and placed in a drying oven at 180 °C. After 3 h, the oven was turned off, and the vessel remained in the oven until cooled to room temperature. Then, the hydrolysate was poured into a beaker and vacuum-filtered through a paper filter. Insoluble feather residues were rinsed from the reaction vessel with desalted water and filtered. The filter residues were dried in vacuo at 45 °C and weighed.
2.3.3 Alkaline hydrolysis.
5 g of pretreated chicken feathers were mixed in a 0.5 L round bottom flask with 85 mL of a 5% (w/v) sodium hydroxide (NaOH) solution containing 0.1 mol L−1 sodium bisulfite and stirred on a sand bath at 40 °C and 300 rpm for 2 h. The solutions were centrifuged for 20 min at 4000×g at room temperature, and the resulting pellets were washed with desalted water, dried in vacuo at 45 °C, and weighed.
2.3.4 Enzymatic hydrolysis of chicken feathers.
The enzymes alcalase, protease A-01, savinase, esperase, trypsin, chymotrypsin, papain, and pepsin were tested for enzymatic hydrolysis of chicken feathers. The activities of the tested enzymes are listed in ESI† Section 2.3. As the specific activities towards feather keratin are not known and our first selection of the proteases was based on the expected fragmentation pattern, we applied identical substrate/enzyme ratios (w/w) without considering activities at this point.
0.5 g of pretreated chicken feathers were added into 8.5 mL of an appropriate aqueous buffer (Table S1†) containing 10 mg of solid freeze-dried enzyme or 100 μL enzyme solution. The reaction batch was incubated under agitation (150 rpm) for up to 48 h at the enzyme temperature optimum (Table S1†). The supernatant was centrifuged at 4142×g at room temperature for 20 min to remove insoluble particles. Insoluble fractions were washed with desalted water, dried at 40 °C in vacuo, and weighed. The supernatants were freeze-dried, weighed, and analysed. Negative controls were performed without the addition of enzymes. The workflow is summarised in Fig. S3.†
Combined hydrolysis approaches were carried out by performing chemical hydrolysis as described in Section 2.3.1, followed by preparing keratin-hydrolysis-peptides (KHPs) as described in this section.
2.4 Reduction of KHP disulfides
The KHP-disulfides were reduced with sodium borohydride to detect free thiols, as described in Section 2.5.2.
For Raman measurements, KHPs were extracted with methanol before reduction to remove salts. Therefore, KHPs were suspended at a ratio of 15 mL methanol per gram of peptide mixture. The suspension was stirred for 12 h at room temperature and filtered. The filtrate was dried in vacuo. For disulfide reduction, water (10 mL) was bubbled with nitrogen for 15 min in a round bottom flask. Extracted KHPs (1155 mg) and tris-(2-carboxyethyl)-phosphine (TCEP, 100 mg, 0.35 mmol) were added to the nitrogen counterflow and dissolved. The mixture was then stirred for 30 min at room temperature under a nitrogen atmosphere. The solution was then transferred to a dialysis tube, dialysed against desalted water (molecular weight cut-off: 100–500 Da, 3 × 2 h, 1
:
150), and freeze-dried, yielding 782 mg of freeze-dried substance.
2.5 Analytical methods
2.5.1 Analysis of peptide concentration.
A biuret assay was used to determine the peptide concentration in CFK hydrolysates. Although cross-reactions with amino acids, dipeptides, and other organic substances may occur, biuret assays are widely used, for example, in clinical chemistry to determine protein and peptide concentrations in urine samples.49 We decided to use the biuret method after we could show that substances such as urea, amino acids, and reducing agents did not affect our measurements in the relevant concentration ranges (Table S2†). The preparation of the Biuret solution used and the determination of peptide concentrations are described in the ESI† in Section 3.2.1.
2.5.2 Determination of free thiols and disulfide moieties.
Based on the publications by Hansen et al.50 and Kurz et al.,51 a modified HPLC method for detecting thiols and disulfides was established. This enabled us to determine the number of free thiols and the number of disulfide bonds in aqueous peptide mixtures. Please refer to ESI† Section 3.2.3 for method details, calibration, and evaluation.
2.5.3 Sequence analysis of chicken feather keratins.
Chicken feathers were pretreated and hydrolysed as described above with the following conditions: 5 g chicken feathers, 85 mL desalted water, 8 mol L−1 urea, 50 mmol L−1L-cysteine, pH 10.5 for 16 h at 70 °C while shaking at 300 rpm. A 500 μL sample was taken, and SDS-PAGE was performed as described in ESI† Section 3.2.4. Stained protein bands of interest were cut out of the gel, destained, and after a tryptic in-gel digest, a nanoLC-ESI-MS/MS was performed to identify the original 10 kDa proteins. (Proteome Factory, Berlin, Germany, performed tryptic in-gel digest and all following analytical steps).
2.5.4 Peptide screening after enzymatic hydrolysis of chicken feathers.
A lyophilised chicken feather keratin hydrolysate was dissolved in desalted water and reduced with TCEP, followed by alkylation of free thiols with iodoacetamide. The peptide mixture was separated, and peptide masses and sequences were identified using nanoLC-ESI-MS/MS. Peak areas from nanoLC were used to estimate the abundances of identified peptides (all steps were performed by Proteome Factory Berlin, Germany).
2.5.5 SDS-PAGE.
Sodium dodecyl sulfate-polyacrylamide gel electrophoresis (SDS-PAGE) was performed according to Laemmli (1970)52 and as described in ESI† Section 3.2.4.
2.5.6 Raman spectroscopy.
Raman-spectra were collected with a DXR3 xi Raman Microscope from Fisher Scientific (Thermo Fisher Scientific GmbH, Dreieich, Germany). A 532 nm diode-pumped solid-state (DPSS) laser with a maximum power of 10 mW was used. A 4000–300 cm−1 spectral range was covered, and a spectral resolution of 2 cm−1 was applied. Measurement settings were optimised for each sample individually. Samples were subjected to the laser for at least 45 min before measurements to reduce fluorescence effects. All analysed samples were solids and were placed on glass slides for analysis.
2.5.7 ATR-FT-IR-spectroscopy.
Infrared (IR) spectra were collected with a Bruker Tensor 27 spectrometer (Bruker Optik GmbH, Ettlingen, Germany) equipped with an ATR crystal. A spectral range of 4000–600 cm−1 and a spectral resolution of 4 cm−1 were applied, respectively. 16 scans were recorded per measurement. Before each measurement, the ATR window and the stamp were cleaned with 95% (v/v) ethanol. All analysed samples were solids.
2.5.8 SEM-imaging.
Chicken feathers were subjected to enzymatic hydrolysis, as described in Section 2.3.4, without prior shredding. Trypsin was used in a potassium phosphate buffer at pH 7.8 for up to 48 h. The treated feathers were then carefully washed with desalted water and dried at room temperature.
To prepare for scanning electron microscopy (SEM), the chicken feather samples were first sputtered with silver using a sputter coater (Cressington 108auto) and a silver target and then examined in secondary electron mode correlated with surface topography using a SEM type FEI Nova NanoSem 450.
2.6 Crosslinking of KHPs and casting of KHP films
Following the method described by Nuutinen et al. (2021), KHPs were cross-linked and cast into films.23 For this purpose, 1.67 g KHPs were dissolved in 25 mL desalted water and centrifuged for 20 min at 20
000×g and 4 °C. The clear supernatant was decanted and vacuum-filtered through a 2.5 μm paper filter. The pH of the resulting KHP solution was pH 9.5. 0.16 g 1,4-butanediol diglycidyl ether (BDE) was added, and the solution was stirred at 60 °C for 15 min. Subsequently, the pH was adjusted to pH 12 with 50% NaOH, 0.17 g glycerol was added, and the suspension was mixed. The mixture was cast into a Petri dish lined with PTFE film and dried for 100 h at 23 °C and 50% relative humidity. Negative controls were made without the addition of glycerol or BDE. Fig. S4† summarises the workflow for chicken feather hydrolysis and KHP film preparation.
2.7 Differential scanning calorimetry (DSC)
DSCs of KHP films were performed on a DSC1 device from Mettler Toledo (USA) in a nitrogen atmosphere with a heating rate of 10 K min−1 from −30.0 °C to 200.0 °C. Heating was performed twice, and the second curve was evaluated using Mettler Toledo's STARe software.
2.8 Statistics
All measurements were performed as duplicate or triplicate determinations. The results given are mean values. Error bars or ±-ranges are the corresponding standard deviations.
3 Results and discussion
3.1 Development of a mild and selective chicken feather hydrolysis protocol – matrix approach
To selectively produce peptides from feather keratin by a biocatalytic process, the recalcitrant and hydrophobic chicken feathers had to be solubilised in a suitable solvent combined with a suitable functional enzyme at specific conditions. Besides that, we applied several solubilisation protocols to benchmark the newly developed enzymatic hydrolysis method.
A water-soluble keratin fraction with a yield of 91% (w/w) was obtained via thermal hydrolysis. Alkaline hydrolysis yielded 51% (w/w) of soluble keratin. Protein concentrations in the obtained hydrolysates were 38.7 g L−1 and 20.2 g L−1, respectively.
Thermal and chemical hydrolysis of keratins under various conditions is extensively explored and experimentally verified.3,41,53 As a limitation of such an approach in the context of the objectives of our study, the use of superheated water results in the complete loss of the cystine and cysteine residues and is accompanied by a distinct formation of odorous sulfur compounds.54 Although the solubility of keratin can generally be improved by breaking peptide bonds and disulfides, this effect is accompanied by a loss of certain functional amino acids such as L-cysteine.55 Since, for our study, it was mandatory to preserve the L-cysteine residues contained in native keratin to be available for thiol-functionalization or crosslinking strategies, a milder hydrolysis regimen had to be established.
3.1.1 Effect of reducing agents.
Based on a feather decomposition protocol described by Xu et al.,4 using 8 mol L−1 urea, 10% (w/w) reducing agent (based on the weight of chicken feathers) at 70 °C and pH 10.5 for 12 h, we screened several combinations of urea and reducing agents and could confirm the excellent performance of L-cysteine as a reducing agent for the decomposition of chicken feathers. Moreover, we could further extend this approach using dithiothreitol (DTT), β-mercaptoethanol (ME), sodium sulfite, reduced glutathione (GSH), and dithiobutylamine (DTBA) as highly effective reducing agents for chicken feather keratin degradation under these conditions. The obtained yields of dissolved keratin ranged between 68.7% (w/w) and 86.5% (w/w) (Table 2). This shows that effective decomposition of chicken feathers is possible under denaturing conditions at 8 mol L−1 urea with a reducing agent.
Table 2 Common synthetic and bio-based reducing agents for the hydrolysis of chicken feathers. Yields represent the percentage of solubilised feather biomass relative to the total feather biomass used. Hydrolysis was performed according to Xu et al.4 with an extended screening of reducing agents
Origin |
Reducing agent |
Yield [% (w/w)] |
Common synthetic |
β-Mercaptoethanol |
74.3 ± 1.2 |
Dithiothreitol |
78.2 ± 1.7 |
Sodium sulfite |
78.6 ± 8.3 |
Bio-based |
L-Cysteine |
68.7 ± 4.9 |
Glutathione (reduced) |
72.6 ± 0.7 |
Dithiobutylamine |
86.5 ± 1.4 |
The use of ME, DTT, and sodium sulfite for the cleavage of disulfides in feather keratins and an associated decomposition of the material was already described.3,56 But, to our knowledge, this is the first report describing the successful application of GSH and DTBA as reducing agents for keratin extraction from chicken feathers. Since DTBA can be obtained from aspartic acid,57 together with GSH and L-cysteine, three bio-based reducing agents are available, showing high performance in feather keratin decomposition. Even more, DTBA was revealed as the best-performing reducing agent in this study since a significantly higher yield of 86.5 ± 1.4% (w/w) was achieved by using DTBA compared to L-cysteine (68.7 ± 4.9% (w/w)) or GSH (72.6 ± 0.7% (w/w)).
Fig. 2 shows the insoluble residues obtained in the various decomposition experiments by applying urea, sodium sulfite or different combinations of urea and reducing agents. The concentrations were 8 mol L−1 for urea and 50 mmol L−1 for the respective reducing agent. Lower urea concentrations were also tested, but no significant decomposition of the feather biomass occurred (data not shown). Thus, from this matrix approach, it can be concluded that the substantial decomposition of chicken feathers via a chemo-reductive method requires large amounts of chemicals, making it challenging to develop an efficient process that follows the principles of sustainable chemistry. Although it is possible to recycle the urea or to use it (to a limited extent) as fertiliser,58,59 we aimed to develop a process that could be run from scratch without a high chemical input, primarily also to facilitate later upscaling. Therefore, we focused our further work on the enzymatic hydrolysis of feather keratin. However, since a high proportion of the chicken feather biomass was solubilised, we used a chemical hydrolysate to characterise the solubilised keratins.
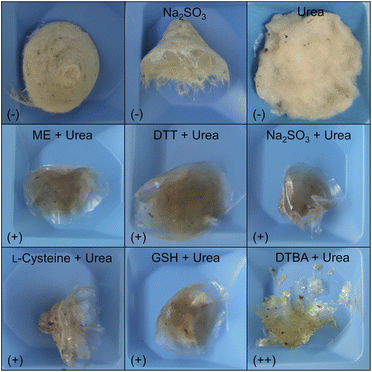 |
| Fig. 2 Insoluble residues after chemical hydrolysis of chicken feathers with urea, and the respective reducing agent (Na2SO3, ME, DTT, L-cysteine, GSH, or DTBA) and as described in Section 2.3.1. The upper left example is a control experiment without addition of urea or a reducing agent. Since we aimed for the highest possible proportion of soluble keratin, very low proportions of insoluble keratin were rated very good (++), and higher proportions of insoluble material were rated as good (+), or below average (−). | |
3.1.2 Identification of solubilised feather keratins.
After chemical decomposition, protein analysis via SDS-PAGE under denaturing and reducing conditions and subsequent Coomassie staining led to a clearly visible and strong band around the expected molecular weight of 10 kDa (Fig. 3A).
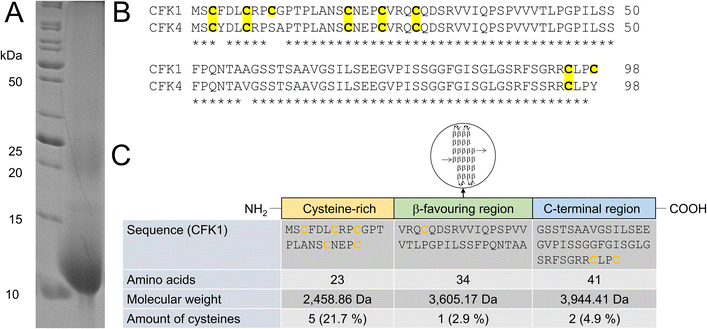 |
| Fig. 3 Identification of solubilized chicken feather keratin. (A) SDS-PAGE, performed under denaturing and reducing conditions, after hydrolysis of chicken feathers with 8 mol L−1 urea and 50 mmol L−1L-cysteine, shows a strong band around 10 kDa, which allowed MS-based identification of chicken feather keratin 1 (CFK1) and chicken feather keratin 4 (CFK4). (B) Sequences of CFK1 and CFK4, showing high sequence homology. (C) Domains and cysteine content of CFK1 (modified from Fraser et al.,9 molecular weights and L-cysteine amounts calculated with Expasy ProtParam47). | |
In the SDS-PAGE shown, in addition to the prominent 10 kDa band, a weak band at approx. 25 kDa can be seen. This is also reported by other authors and was attributed to the removal of the reducing agent β-mercaptoethanol, used for keratin extraction by dialysis and the resulting re-crosslinking of the keratin molecules by new formation of cysteinyl-disulfides.60 Several 10 kDa bands were excised from a gel. After a tryptic in-gel digest followed by nano-HPLC separation of peptide fragments and subsequent ESI-MS, it was possible to identify the underlying proteins as chicken feather keratin 1 (CFK1, Uniprot AN P02450) and chicken feather keratin 4 (CFK4, Uniprot AN P20308). The molecular weights of both keratins are 9.972 kDa (CFK1) and 10.104 kDa (CFK4), respectively.
Thus, we concluded that both keratins were present as intact molecules in the hydrolysates, and no hydrolysis of the peptide backbone had occurred. It has to be noted that there is a significant homology regarding the amino acid sequence between the two identified keratins (Fig. 3B).
3.2 Enzymatic hydrolysis of chicken feather keratin
The amino acid sequences of hard keratins, which the feather keratins belong to, can be divided into three domains. Interestingly, the L-cysteine residues in the sequence are not evenly distributed throughout the complete sequence and over these three domains (Fig. 3C). As shown exemplarily for feather keratin 1, five of eight L-cysteine residues are found in a cysteine-rich region within the first 23 amino acids. Thus, the L-cysteine content of this domain is 21.7%. Only one L-cysteine residue is found in the β-sheet favouring region; two additional L-cysteine residues are located at the C-terminal end of the sequence. In order to isolate L-cysteine-rich peptides from the 10 kDa feather keratin, the N-terminal keratin domain had to be enzymatically excised and separated as completely as possible, leaving the L-cysteine residues intact. Thus, highly specific hydrolysis had to be performed under the mildest possible conditions and, if possible, with low use of additional chemicals. Specific enzymatic decomposition of feathers to produce defined feather keratin fragments (keratin-based peptide oligomers) and usage of such fragments in further materials development is widely unexplored. Despite several proteases for animal protein hydrolysis being commercially available at a technical scale and the use of microbial enzymes to degrade feather keratin is described, most protocols aim for unspecific hydrolysis of the feathers into small peptide fragments and free amino acids.
3.2.1 Unspecific proteases.
We screened selected specific proteases based on their fragmentation pattern and unspecific proteases to identify suitable conditions for chicken feather decomposition. Namely, we tested the unspecific subtilisin-like proteases alcalase, protease A-01, savinase, and esperase, and the acidic protease S-02 for their capability to hydrolyse chicken feathers. Only the respective enzyme preparation was applied, and no denaturing or reduction agents were added. The results are summarised in Fig. 4, wherein the different enzymes are compared to a negative control (without enzyme). A maximum percentage yield of soluble keratin of up to 74.3% (w/w) was achieved with esperase, whereby 47.0% could be classified as soluble protein via protein determination; 27.3% could not be assigned. In the negative control, 92.4% (w/w) of the chicken feathers remained undissolved, 2.9% could be classified as soluble protein, and the missing 4.7% were attributed to losses during processing (washing of the insoluble residues, poor centrifugability of the non-digested intact feathers in the negative controls). Alcalase, savinase, protease A-01, and esperase showed high keratinolytic activity reflected by the respective %-yields of soluble peptides in ranges between 43.9% (w/w) (savinase) and 47.0% (w/w) (esperase). The overall process led in every case to a high amount of unclassified material (24.2–27.5% (w/w)) produced by these proteases, indicating a remarkable degree of unspecific hydrolysis, generating, for example, single amino acids or dipeptides, not detectable with peptide determination via the Biuret method. In the case of protease S2, only a minimal decomposition of the feathers used was observed, and 84.3% (w/w) remained undissolved, so there was hardly any keratinolytic activity.
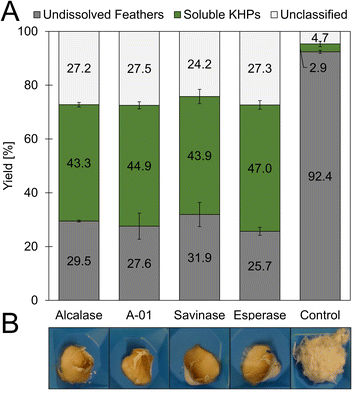 |
| Fig. 4 Unspecific enzymatic hydrolysis of chicken feathers. (A) The percentages of soluble proteins or amino acids and insoluble residues are given in relation to the feather biomass used. Results are mean values ± SD, n = 2. (B) Insoluble residues of chicken feather hydrolysis approaches with alcalase, protease A-01, savinase, esperase, compared to a negative control without addition of an enzyme. The residues were washed with desalted water and dried at 40 °C in vacuo. | |
3.2.2 Specific proteases.
The hydrolysis of feathers by conventional serine- and cysteine-proteases, including subtilisin, papain, chymotrypsin, pepsin, and trypsin, was already investigated by Ramnani et al.45 They could show that subtilisin, chymotrypsin, and papain degrade feathers in the presence of a suitable reducing agent (ME, L-cysteine, DTT, GSH, sodium sulfite, thioglycolate). Trypsin and pepsin failed to degrade chicken feathers in this experimental setup. If chicken feathers were pretreated with another protease (subtilisin/chymotrypsin) for 2 h, trypsin and pepsin degraded the feather biomass, but this was not achieved without this additional pretreatment step.45
In our study, we could demonstrate the hydrolysis of chicken feathers by trypsin, chymotrypsin, and papain with yields of soluble keratin fragments of up to 47% (w/w) without the addition of reducing or denaturing agents after 16 h hydrolysis time (Fig. S9†). The addition of reducing agents and urea was nevertheless also investigated and led to an increased yield of soluble keratin of 89% (w/w) (Fig. S10†). Pepsin showed no keratinolytic activity in the respective experiments. Thus, we could show that standard technical-grade proteases can decompose feather keratin.
3.2.3 Trypsin.
Among the investigated enzymes, trypsin was identified as the most promising protease for selective hydrolysis of chicken feathers by performing a detailed in silico analysis with the Expasy peptide cutter.48 In contrast to the other proteases, hydrolysis with trypsin leads to an N-terminal 2714 Da fragment after cleavage at Arg25 with the sequence Met-Ser-Cys-Phe-Asp-Leu-Cys-Arg-Pro-Cys-Gly-Pro-Thr-Pro-Leu-Ala-Asn-Ser-Cys-Asn-Glu-Pro-Cys-Val-Arg. This predicted fragment contains five L-cysteine moieties and thus fully meets the objectives of our study. Following this in silico approach, we explored the keratinolytic capabilities of trypsin by varying the hydrolysis time and measuring the release of thiols into the hydrolysates (Fig. 5).
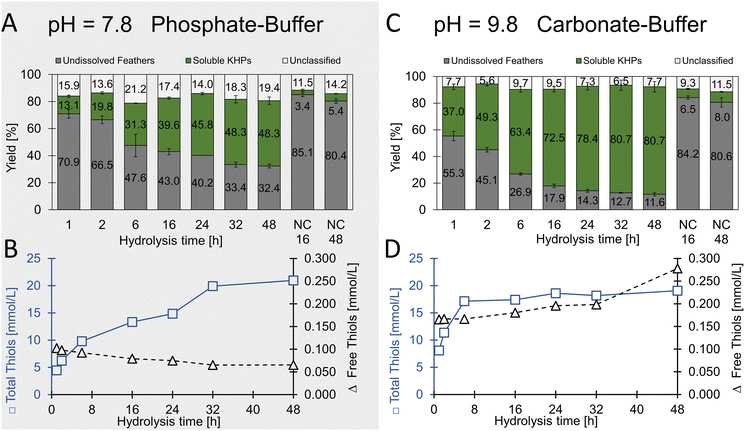 |
| Fig. 5 Specific enzymatic hydrolysis of chicken feathers using trypsin. (A and B) were performed in phosphate buffer at pH 7.8. (C and D) were performed in carbonate buffer at pH 9.8. | |
In extended hydrolysis reactions (total reaction time 48 h), up to 67.6% (w/w) of the feather biomass was dissolved, and peptide concentrations of up to 28.4 g L−1 were obtained. 48.3% (w/w) of the solubilised material could be assigned to soluble proteins. The content of unclassified material after 48 h was determined to be 19.4% (w/w) and thus significantly lower than in the case of the non-specific proteases investigated as described above, in which mass fractions significantly above 25% (w/w) were determined after 19 h. This result indicates the higher specificity of trypsin towards keratin biomass under the chosen conditions and aligns very well with the criteria of the desired process.
With regard to the water-soluble keratin-based peptides, a total thiol concentration of 21.0 ± 0.8 mmol L−1 was determined after 48 h hydrolysis time with less than 0.1 mmol L−1 free thiols. Since hydrolysis was carried out under relatively mild conditions, we concluded that the disulfide bridges in the feathers were retained. When assuming trypsin as pure enzyme, a maximal theoretical amount of 1.4% thiol moieties present is introduced to the hydrolysates through trypsin (see Table S4†). Future studies may involve enzyme immobilisation or enzyme recovery-systems after the process if necessary.
It remains unclear whether all thiols form disulfide bridges or are otherwise oxidised and whether the disulfide bridges are formed as intramolecular or intermolecular bonds.
In an additional experiment, we added the reducing agent sodium sulfite and found a significantly increased yield of soluble protein from 37 to 46% (w/w) after 19 h hydrolysis with trypsin (Fig. S11†).
These findings advance earlier studies where no keratinolytic activity for trypsin, even in the presence of reducing agents, was observed.45
3.2.4 Optimization and upscaling.
The hydrolysis experiments described so far were carried out at the optimum pH and temperature of the enzymes used. For porcine trypsin, the optimum conditions are between pH 7 and pH 9 and temperatures of 50–55 °C.61–64 We could show that more alkaline conditions (pH 9.8) increased the yield of dissolved feather biomass significantly from 67.6% (w/w) to 88.4% (w/w) with a soluble protein yield of 80.7% (w/w) after 48 h without adjustment of the enzyme–substrate ratio.
Remarkably, the thiol concentration in relation to the protein concentration increased steadily over time in the phosphate buffer (pH 7.8) compared to the carbonate buffer (pH 9.8). In contrast, although much higher protein concentrations were achieved in the carbonate buffer, the thiol concentration remained essentially constant in relation to the protein concentration. From this, we concluded that initially, the more accessible amorphous and cysteine-rich regions of feather keratin were targeted by the enzyme, and later, increasingly cysteine-free peptides were solubilised from the crystalline central CFK domain.
A direct comparison of the tryptic chicken feather hydrolysis with the subtilisin-like savinase showed a lower yield of soluble material in the hydrolysate for trypsin, indicating a higher specificity and lower number of cleavage sites (Fig. S12†). Applying a combined chemical and enzymatic decomposition approach, the efficient hydrolysis of feather keratins by trypsin could be shown via SDS-PAGE: the chemical hydrolysis resulted in a clearly visible 10 kDa band, and after the addition of trypsin to the hydrolysate, the 10 kDa band disappeared. This indicated the hydrolysis of the respective protein. Already 0.5 h after addition, total hydrolysis of the keratin was accomplished. The 10 kDa band faded and disappeared completely after 19 h incubation and subsequent SDS-PAGE analysis (Fig. S13†).
The optimal compromise of hydrolysis time, protein, and thiol concentration was found at a hydrolysis time of 16–19 h when using the more basic carbonate buffer system. The newly developed hydrolysis process was scaled up from laboratory to technical scale (Fig. S4†).
Trypsin is susceptible to autolysis,65 and corresponding studies are often carried out without adding another substrate.65,66 In particular, no data are available on trypsin autolysis in the presence of feather keratins. As we could not achieve full decomposition of the feather biomass, we added trypsin again after a reaction time of 24 h, but this resulted in only minor further feather decomposition. Thus, the incomplete degradation was most probably not due to autolysis effects but to a lack of accessible substrate (Table S5†). When optimising the trypsin/feather keratin ratio, we found an optimum mass ratio of 1
:
50 (Fig. S14†) and investigated the trypsin activity over reaction time. Here, we found a significant activity drop within the first hour, while the residual activities remained higher if chicken feathers were present as substrate (Fig. S15†).
It was already reported that the contamination of raw feathers and the absence of cleaning and disinfection processes in the poultry industry limit the value-added processing of chicken feathers.16 Although our feathers were contaminated with blood, skin residues and dust, our new decomposition process reliably produced comparable results regardless of the feather- and trypsin-batches used. Tests with other keratins (goose feathers, human hair, horn shavings) showed successful decomposition of goose feathers and horn shavings but with lower yields of soluble peptides compared to chicken feathers (data not shown). Since a high microbial load of the waste feathers had to be assumed,67 processing of non-autoclaved chicken feathers was not applicable. Tests with sterilised goose feathers showed that autoclaving significantly influences the enzymatic decomposition process (Fig. S8†). This result should be transferable to chicken feathers. The autoclaving conditions are close to hydrothermal treatment, which has been described for keratin decomposition.68–70
3.3 Characterisation of tryptic feather keratin hydrolysates
3.3.1 Confirmation of disulfides via Raman spectroscopy.
We recorded Raman spectra of a chicken feather, KHPs, and KHPs after reduction with TCEP to verify the presence of thiols and disulfides. Raman spectra contain information about amino acid side chains and the protein-typical amide bands. In particular, the S–S stretching vibrations of disulfide bridges being in a gauche–gauche–gauche arrangement can be detected in a narrow range between 508 and 512 cm−1.71
We detected a signal at 509.95 cm−1, indicating the presence of disulfides in the analysed chicken feather hydrolysate and the original chicken feather (Fig. 6). The Raman spectrum of the reduced KHPs no longer showed a signal in the 509 cm−1 region but a signal at approximately 2573 cm−1, characteristic of free thiols.
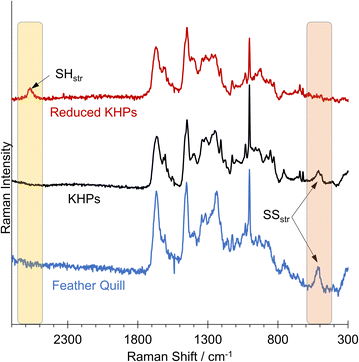 |
| Fig. 6 Raman spectra of a chicken feather quill, KHPs and reduced KHPs. | |
This allows the conclusion that all thiols were initially present in their oxidised form as disulfides in untreated chicken feathers and hydrolysis did not influence this. Subsequently, a reduction can be carried out in a second step, after which no more disulfides were found in the Raman spectrum. Instead, a new band was formed, indicating the presence of thiols. We assume that the thiol-containing reducing agents tested in the initial benchmarking experiments also would allow a corresponding release of thiols.
3.3.2 Peptide pattern after tryptic hydrolysis.
A peptide screening was performed with the freeze-dried feather keratin hydrolysate to identify the peptides present in it. A total of up to 103 peptides were found that could be assigned to chicken feather keratins 1 and 4 (CFK1, CFK4, Table S6†). Of these, 20 peptides contained two or more L-cysteine residues. The complete feather keratin sequences, except for the last eight C-terminal amino acids, are covered. The L-cysteine-containing peptides from the N-terminal region could be assigned to CFK1, while some peptides from the middle part originate from CFK4. An abundance estimate was made based on the ionic currents recorded in MS. The most abundant peptide, according to the signal intensity, is an 11mer with the sequence Asp-Leu-Cys-Arg-Pro-Cys-Gly-Pro-Thr-Pro-Leu (Fig. 1 and S16†).
3.3.3 SEM imaging.
In our study, no total hydrolysis of chicken feathers was achieved. In a model experiment, we investigated which areas of a feather were attacked during enzymatic decomposition.
Instead of shredded chicken feathers, entire feathers were used, which only had been sterilised and cleaned. Trypsin was used for hydrolysis, and the process conditions were selected analogously to the hydrolysis experiments described above. After 16 or 48 hours, the feathers were carefully taken from the hydrolysis solution and washed to remove the protease and buffer salts. SEM images were taken to show changes in the feather structures over time (Fig. 7).
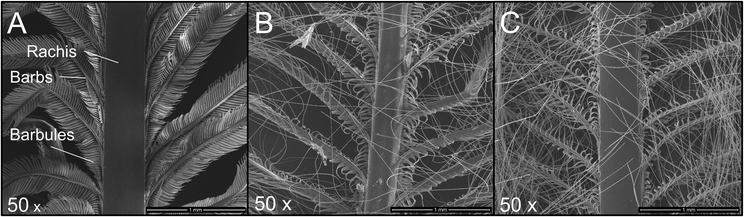 |
| Fig. 7 SEM of chicken feathers treated with trypsin. (A) Control without trypsin, (B) trypsin hydrolysis for 16 h, (C) trypsin hydrolysis for 48 h. The magnification is 50×. | |
Fig. 7A shows an untreated feather. Starting from the rachis, the branched structures of the barbs and barbules are visible. The following images were taken after the feathers were exposed to enzymatic hydrolysis for 16 h (Fig. 7B) and 48 h (Fig. 7C), respectively.
It can be seen that, primarily, the finer feather structures were disentangled over time. The solid rachis remains intact, and the finer barbs and barbules are increasingly decomposed.
3.4 Cross-linking of KHPs
In addition to providing polycysteine-containing KHPs, the present study also aimed to identify potential applications for the KHPs. Crosslinking and subsequent film formation are one way to highlight the unique properties of KHPs.
3.4.1 Film-casting with KHPs.
A widely used cross-linker for proteins is glutaraldehyde (GA). However, the reaction mechanism comprises primarily the ε-amino groups of lysine reacting with the aldehyde moieties of GA.23 Since no lysine is present in the main KHP fragment, we decided not to use GA for cross-linking experiments. Instead, we worked with 1,4-butanediol diglycidyl ether (BDE), which is less toxic and has already been used for crosslinking gelatin72 and feather keratin23 films.
For the initially tested unreduced KHPs, we assume the epoxy groups of BDE and the amino groups of KHPs participate in the crosslinking reaction. The reduction of cystine residues buried in the KHPs to free thiols should allow so-called thiol-epoxy “click” reactions under basic conditions,73 and enable tuning the properties of the resulting films.
Casting films from KHP solutions without adding BDE and the plasticiser glycerol resulted in a brittle material that did not form a continuous film. The addition of BDE brought an improvement, but only after adding BDE and glycerol was a film obtained that was stable enough to be removed from the Petri dish. The high transparency of the 100 μm thick film was remarkable (Fig. 8).
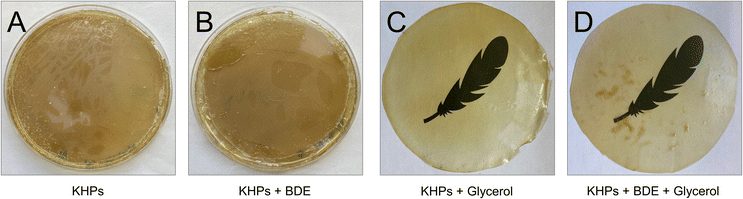 |
| Fig. 8 Keratin films cast from aqueous KHP solutions and with addition of the crosslinker BDE and the plasticizer glycerol. All films were cast in Petri dishes coated with PTFE film and dried for 100 h. (A) KHP solution, the very brittle material could not be removed from the Petri dish. (B) KHP solution with BDE. (C) KHP solution with glycerol. The soft material could be removed from the Petri dish. (D) KHP solution with BDE and glycerol, the resulting films could be removed from the PTFE-coated dish. The films in (C) and (D) were placed on a paper with a printed black chicken feather motif to illustrate the high transparency of the film. | |
Differential Scanning Calorimetry was carried out with all 4 films (Table S7†), and a pronounced glass transition temperature Tg at 172 °C was measured with the pure KHP film (A). The addition of glycerol (film C) had an apparent plasticising effect and was accompanied by a reduction of Tg to 107 °C. The addition of BDE (film B) also had an impact on the chain mobility (Tg = 152 °C) (but at the current time without explicit knowledge of the mechanism), and the addition of glycerol to the KHP-BDE-mixture (film D) lowered Tg significantly to 104 °C due to softening effects.
3.4.2 Secondary structure of keratin samples obtained after trypsin catalysed hydrolysis from different process stages.
IR measurements were performed with KHPs, insoluble hydrolysis residues, and cross-linked KHP films (Fig. 9). For all samples, the typical protein vibrations were found, namely the N–H stretching vibration at ∼3300 cm−1 (amide A), the C
O stretching vibration at ∼1650 cm−1 (amide I), the amide II vibration at ∼1550 cm−1, which is mainly an out-of-phase combination of NH in-plane bend and the CN stretching vibration, and the amide III vibration at 1400–1200 cm−1, which is the in-phase combination of the latter two modes. The amide I mode is highly sensitive to the secondary structure of peptides and proteins.74
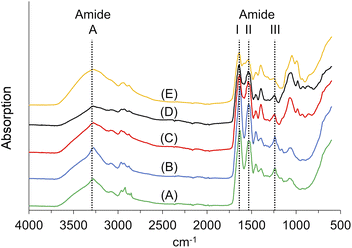 |
| Fig. 9 ATR-FTIR-Spectra of keratin samples of different process stages. (A) Insoluble residue after chicken feather hydrolysis (negative control without addition of trypsin), (B) insoluble residue after chicken feather hydrolysis with trypsin, (C) KHPs from laboratory hydrolysis test, (D) KHPs from pilot hydrolysis test, (E) KHP film, cross-linked with BDE. Samples (C) and (D) were analysed as lyophilized powder. | |
We identified the amide A vibrations in all samples at 3272 cm−1 or 3273 cm−1, respectively. The amide II vibration appeared at wavenumbers between 1538 cm−1 and 1531 cm−1. The amide III vibrations were found between 1235 cm−1 and 1244 cm−1. The amide I vibration of KHPs appeared at 1635 cm−1; for the insoluble residues, it was located at 1629 cm−1. Both wave numbers are in accordance with β-sheet secondary structure motifs, which can be found at ∼1630 cm−1.74 After crosslinking, we found the amide vibration at 1639 cm−1, which is still consistent with β-folds. The typical amide I vibrations for helical structures would occur at ∼1655 cm−1 but are down-shifted to wave numbers at 1640–1650 cm−1 if the helix is exposed to water.75
Interestingly, the FT-IR spectra of the KHPs indicate the presence of β-sheet structures. Accordingly, we assume that parts of the crystalline portion of the feather keratin can also be solubilised (Fig. 4). Corresponding cleavage sites for the enzyme used are available (Arg25, Arg31).
4 Conclusions
A new biocatalytic method for the mild hydrolysis of chicken feathers was developed. A simple pretreatment is followed by an enzymatic decomposition using the specific protease trypsin in technical quality, in which peptide mixtures suitable for preparing protein films were obtained.
All previously known approaches for the hydrolysis of chicken feathers required harsh conditions, high temperatures and/or pressures, a high input of chemicals, or multi-step enzymatic processes. With our method, we could demonstrate for the first time the decomposition of chicken feathers under mild conditions and without the addition of denaturing substances, detergents, and reducing agents, which would have made upscaling very difficult due to the separation from the product.
The novel method was developed on a laboratory scale and scaled up to 25 L and is currently being investigated at an 800 L scale. The resulting peptide mixtures contain a significant number of thiols in their oxidised form. Chemical reduction makes it possible to provide peptides containing free thiols.
In particular, the amino acid sequence of feather keratin proves to be advantageous in terms that the L-cysteine residues of feather keratin are located almost exclusively within a cysteine-rich domain within the first 25 N-terminal amino acids, which can be cleaved with the help of trypsin, resulting in short-chain peptides with a molecular weight of about 1200 Da, which carry two or more L-cysteine residues, providing bio-originated polythiols suitable for a vast range of applications.
These peptide mixtures allowed the production of protein films without further purification or reduction of cystine disulfides. Even though they required the addition of a cross-linker, stable films with high transparency were obtained. Moreover, this work paves the way for using keratin-based peptides as platform chemicals for various applications. Specifically, the liberated thiol moieties may enable selective orthogonal modification and crosslinking strategies.
In future studies, enrichment of the polythiol-containing peptides from the keratin hydrolysates at a larger scale may significantly lead to advances in developing respective peptide-based functional materials. Similarly, investigating the properties of such KHP films in dependence on various additives (cross-linkers, plasticisers) is expected to yield functional materials with different properties depending on the type and amount of additive. In addition, the preparation of protein hydrolysates from alternative keratin and protein sources according to the approach described will allow a diversification of the toolbox of available KHPs.
Author contributions
Andreas Schieder: conceptualization, investigation, methodology, validation, visualisation, writing – original draft, writing – review and editing. Julia Diener: investigation, validation, methodology, writing – review and editing. Martin Diekmann: investigation, writing – review and editing. Christian Bartsch: investigation, methodology. Florian Dietrich: investigation, methodology Claudia Falcke: investigation, methodology. Iva Anic: investigation, writing – review and editing. Steffen Roth: conceptualization, supervision, writing – review and editing. Volker Sieber: supervision, writing – review and editing. Andreas Taden: conceptualization, supervision, writing – review and editing. Michael Richter: conceptualization, supervision, writing – review and editing.
Conflicts of interest
There are no conflicts to declare.
Acknowledgements
The authors are grateful for funding of this work by the German Federal Ministry of Food and Agriculture (BMEL) coordinated through Fachagentur Nachwachsende Rohstoffe e.V. within the project “KERAbond – Specialty chemicals from tailored polythiol keratin proteins” (grant 22014218).
References
- G. Centi and S. Perathoner, Green Chem., 2022, 24, 7305–7331 RSC.
- E.-M. Nuutinen, P. Willberg-Keyriläinen, T. Virtanen, A. Mija, L. Kuutti, R. Lantto and A.-S. Jääskeläinen, RSC Adv., 2019, 9, 19720–19728 RSC.
- I. Sinkiewicz, A. Śliwińska, H. Staroszczyk and I. Kołodziejska, Waste Biomass Valorization, 2017, 8, 1043–1048 CrossRef CAS.
- H. Xu and Y. Yang, ACS Sustain. Chem. Eng., 2014, 2, 1404–1410 CrossRef CAS.
- L. Lange, Y. Huang and P. K. Busk, Appl. Microbiol. Biotechnol., 2016, 100, 2083–2096 CrossRef CAS.
- M. A. Meyers, P.-Y. Chen, A. Y.-M. Lin and Y. Seki, Prog. Mater. Sci., 2008, 53, 1–206 CrossRef CAS.
- A. J. Poole, R. E. Lyons and J. S. Church, J. Polym. Environ., 2011, 19, 995–1004 CrossRef CAS.
- J. McKittrick, P.-Y. Chen, S. Bodde, W. Yang, E. Novitskaya and M. Meyers, J. Miner. Met. Mater. Soc., 2012, 64, 449–468 CrossRef.
- R. D. B. Fraser and D. A. D. Parry, J. Struct. Biol., 2011, 173, 391–405 CrossRef CAS PubMed.
- E. Jabbari, Gels, 2019, 5, 5 CrossRef PubMed.
- Fact.MR, Polythiols Market Outlook (2023 to 2033), https://www.factmr.com/report/1256/polythiols-market, accessed 26 October, 2023.
- J. M. Krüger, C.-Y. Choi, F. Lossada, P. Wang, O. Löschke, D. Auhl and H. G. Börner, Macromolecules, 2022, 55, 989–1002 CrossRef.
- J. Horsch, P. Wilke, M. Pretzler, M. Seuss, I. Melnyk, D. Remmler, A. Fery, A. Rompel and H. G. Börner, Angew. Chem., Int. Ed., 2018, 57, 15728–15732 CrossRef CAS PubMed.
- S. Chandna, C. A. Olivares M, E. Baranovskii, G. Engelmann, A. Böker, C. C. Tzschucke and R. Haag, Angew. Chem., Int. Ed., 2023, e202313945 Search PubMed.
- M. Jawerth, M. Johansson, S. Lundmark, C. Gioia and M. Lawoko, ACS Sustain. Chem. Eng., 2017, 5, 10918–10925 CrossRef CAS.
- E. Dieckmann, R. Onsiong, B. Nagy, L. Sheldrick and C. Cheeseman, Waste Biomass Valorization, 2021, 12, 1119–1131 CrossRef CAS.
- A. Shavandi, T. H. Silva, A. A. Bekhit and A. E.-D. A. Bekhit, Biomater. Sci., 2017, 5, 1699–1735 RSC.
- N. Florin, A. Maddocks, S. Wood and A. Harris, Waste Manage., 2009, 29, 1399–1408 CrossRef CAS PubMed.
- P. Billen, J. Costa, L. Van der Aa, J. Van Caneghem and C. Vandecasteele, J. Clean. Prod., 2015, 96, 467–475 CrossRef CAS.
- R. Bhari, M. Kaur and R. Sarup Singh, Curr. Microbiol., 2021, 78, 2212–2230 CrossRef CAS PubMed.
-
E. Parliament, Regulation (EC) No. 1774/2002.
- N. Reddy, Waste Manage., 2015, 45, 91–107 CrossRef CAS PubMed.
- E.-M. Nuutinen, T. Virtanen, R. Lantto, M. Vähä-Nissi and A.-S. Jääskeläinen, RSC Adv., 2021, 11, 27512–27522 RSC.
-
P. Anastas and J. Warner, Green Chemistry: Theory and Practice, Oxford University Press, New York, 1998 Search PubMed.
- A. C. Pauly and F. di Lena, Polymer, 2015, 72, 378–381 CrossRef CAS.
- M. Zelzer, S. J. Todd, A. R. Hirst, T. O. McDonald and R. V. Ulijn, Biomater. Sci., 2013, 1, 11–39 RSC.
- C. E. Hoyle and C. N. Bowman, Angew. Chem., Int. Ed., 2010, 49, 1540–1573 CrossRef CAS PubMed.
- X. Ye, J. Yuan, Z. Jiang, S. Wang, P. Wang, Q. Wang and L. Cui, Eng. Life Sci., 2020, 20, 17–25 CrossRef CAS PubMed.
- A. B. Lowe, Polym. Chem., 2014, 5, 4820–4870 RSC.
- S.-M. Hong and S.-H. Hwang, ACS Omega, 2022, 7, 21987–21993 CrossRef CAS PubMed.
- A. O. Konuray, X. Fernandez-Francos and X. Ramis, Polymer, 2017, 116, 191–203 CrossRef CAS.
- H. Distler, Angew. Chem., Int. Ed., 1967, 6, 544–553 CrossRef CAS.
- F. Sączewski, M. Gdaniec and K. Data, Heterocycl. Commun., 2017, 23, 359–363 CrossRef.
- K. Wang, R. Li, J. H. Ma, Y. K. Jian and J. N. Che, Green Chem., 2016, 18, 476–481 RSC.
-
J. A. Maclaren and B. Milligan, Wool Science. The Chemical Reactivity of the Wool Fibre, Science Press, 1981 Search PubMed.
- I. O'donnell and E. Thompson, Aust. J. Biol. Sci., 1964, 17, 973–978 CrossRef.
- D. R. Goddard and L. Michaelis, J. Biol. Chem., 1935, 112, 361–371 CrossRef CAS.
- Z. Jiang, J. Yuan, P. Wang, X. Fan, J. Xu, Q. Wang and L. Zhang, Int. J. Biol. Macromol., 2018, 119, 423–430 CrossRef CAS PubMed.
- A. Idris, R. Vijayaraghavan, A. F. Patti and D. R. MacFarlane, ACS Sustainable Chem. Eng., 2014, 2(7), 1888–1894 CrossRef CAS.
- Z. Yu, B. Zhang, F. Yu, G. Xu and A. Song, Bioresour. Technol., 2012, 121, 335–341 CrossRef CAS PubMed.
- W. Zhao, R. Yang, Y. Zhang and L. Wu, Green Chem., 2012, 14, 3352–3360 RSC.
- M. Zoccola, A. Aluigi, A. Patrucco, C. Vineis, F. Forlini, P. Locatelli, M. C. Sacchi and C. Tonin, Text. Res. J., 2012, 82, 2006–2018 CrossRef.
- E. H. Burtt Jr and J. M. Ichida, Auk, 1999, 116, 364–372 CrossRef.
- X. Lin, G. Inglis, L. Yanke and K. Cheng, J. Ind. Microbiol. Biotechnol., 1999, 23, 149–153 CrossRef CAS PubMed.
- P. Ramnani and R. Gupta, World J. Microbiol. Biotechnol., 2007, 23, 1537–1540 CrossRef CAS.
- C. Earland and C. Knight, Biochim. Biophys. Acta, 1955, 17, 457–461 CrossRef CAS PubMed.
- S. Blackburn and G. Lee, Biochim. Biophys. Acta, 1956, 19, 505–512 CrossRef CAS PubMed.
-
E. Gasteiger, C. Hoogland, A. Gattiker, S. e. Duvaud, M. R. Wilkins, R. D. Appel and A. Bairoch, in The Proteomics Protocols Handbook, ed. J. M. Walker, Humana Press, Totowa, NJ, 2005, DOI: DOI:10.1385/1-59259-890-0:571, pp. 571–607.
- G. L. Hortin and B. Meilinger, Clin. Chem., 2005, 51, 1411–1419 CrossRef CAS PubMed.
- R. E. Hansen, H. Østergaard, P. Nørgaard and J. R. Winther, Anal. Biochem., 2007, 363, 77–82 CrossRef CAS PubMed.
- F. Kurz, C. Hengst and U. Kulozik, MethodsX, 2020, 7, 101112 CrossRef CAS PubMed.
- U. K. Laemmli, Nature, 1970, 227, 680–685 CrossRef CAS PubMed.
- H. Di Domenico Ziero, L. C. Ampese, W. G. Sganzerla, P. C. Torres-Mayanga, M. T. Timko, S. I. Mussatto and T. Forster-Carneiro, J. Supercrit. Fluids, 2022, 181, 105492 CrossRef CAS.
- J. Yin, S. Rastogi, A. E. Terry and C. Popescu, Biomacromolecules, 2007, 8, 800–806 CrossRef CAS PubMed.
- Y. S. Lee, L.-Y. Phang, S. A. Ahmad and P. T. Ooi, Waste Biomass Valorization, 2016, 7, 1147–1157 CrossRef CAS.
-
S. Sharma, A. Gupta, S. Chik, K. Gek, P. Podde, J. Thraisingam and M. Subramaniam, Extraction and characterization of keratin from chicken feather waste biomass: a study, Proceedings of the national conference for postgraduate research (NCON-PGR 2016), Universiti Malaysia Pahang (UMP), Pekan, 2016 Search PubMed.
- J. C. Lukesh, M. J. Palte and R. T. Raines, J. Am. Chem. Soc., 2012, 134, 4057–4059 CrossRef CAS PubMed.
-
J. M. Bremner, in Nitrogen Economy in Tropical Soils: Proceedings of the International Symposium on Nitrogen Economy in Tropical Soils, Held in Trinidad, W.I., January 9–14, 1994, ed. N. Ahmad, Springer Netherlands, Dordrecht, 1996, DOI:10.1007/978-94-009-1706-4_30, pp. 321–329.
- B. Beig, M. B. K. Niazi, Z. Jahan, A. Hussain, M. H. Zia and M. T. Mehran, J. Plant Nutr., 2020, 43, 1510–1533 CrossRef CAS.
- S. Alahyaribeik and A. Ullah, Int. J. Biol. Macromol., 2020, 148, 449–456 CrossRef CAS PubMed.
- F. F. Buck, A. J. Vithayathil, M. Bier and F. F. Nord, Arch. Biochem. Biophys., 1962, 97, 417–424 CrossRef CAS PubMed.
- W. G. Crewther, Aust. J. Biol. Sci., 1953, 6, 597–616 CrossRef CAS PubMed.
- M. Kunitz and J. H. Northrop, J. Gen. Physiol., 1934, 17, 591–615 CrossRef CAS PubMed.
- T. Sipos and J. R. Merkel, Biochemistry, 1970, 9, 2766–2775 CrossRef CAS PubMed.
- M. M. Vestling, C. M. Murphy and C. Fenselau, Anal. Chem., 1990, 62, 2391–2394 CrossRef CAS PubMed.
- P. Sriram, N. Kalogerakis and L. A. Behie, Biotechnol. Tech., 1996, 10, 601–606 CrossRef CAS.
-
R. Fries, V. Bergmann and K. Fehlhaber, Praxis der Geflügelfleischuntersuchung, Schlütersche, 2001 Search PubMed.
- K. Chojnacka, H. Górecka, I. Michalak and H. Górecki, Waste Biomass Valorization, 2011, 2, 317–321 CrossRef.
- M. Škerget, M. Čolnik, L. F. Zemljič, L. Gradišnik, T. Ž. Semren, B. T. Lovaković and U. Maver, Polymers, 2023, 15, 2658 CrossRef PubMed.
- T. Kaewsalud, K. Yakul, C. Insomphun, K. Jantanasakulwong, P. Rachtanapun, W. Tapingkae, S. Chuetor, M. Watanabe and T. Chaiyaso, J. Chem. Technol. Biotechnol., 2023, 98, 1203–1214 CrossRef CAS.
- A. Rygula, K. Majzner, K. M. Marzec, A. Kaczor, M. Pilarczyk and M. Baranska, J. Raman Spectrosc., 2013, 44, 1061–1076 CrossRef CAS.
- J. F. Martucci, J. P. Espinosa and R. A. Ruseckaite, Food Bioprocess Technol., 2015, 8, 1645–1656 CrossRef CAS.
- M. C. Stuparu and A. Khan, J. Polym. Sci., Part A: Polym. Chem., 2016, 54, 3057–3070 CrossRef CAS.
- A. Barth, Biochim. Biophys. Acta, Bioenerg., 2007, 1767, 1073–1101 CrossRef CAS PubMed.
- S. Y. Venyaminov and N. N. Kalnin, Biopolymers, 1990, 30, 1259–1271 CrossRef CAS PubMed.
|
This journal is © The Royal Society of Chemistry 2024 |
Click here to see how this site uses Cookies. View our privacy policy here.