DOI:
10.1039/D4SD00017J
(Critical Review)
Sens. Diagn., 2024,
3, 718-744
Emergence of integrated biosensing-enabled digital healthcare devices
Received
16th January 2024
, Accepted 26th March 2024
First published on 26th April 2024
Abstract
Digital biosensors facilitate real-time, remote, precise disease detection and biochemical analysis. Recent trends in biosensing methods have focused on miniaturization, automation, and multiplexing. The miniaturization of biosensors has led to the development of portable, flexible, and wearable devices that can be used for point-of-care diagnostics and continuous health monitoring. Furthermore, digital automation has enabled the high-throughput screening of samples, reducing the time and cost of analysis, while integrated multiplexing allows for the simultaneous detection of multiple analytes, increasing the efficiency and accuracy of analysis. This article examines recent scientific advances in developing miniaturized biosensing procedures for digital healthcare. Advancements in digital devices have also contributed to the development of integrated biosensing. The use of smartphones, smartwatches, and other digital devices as readout platforms for biosensors has made biosensing more accessible and user-friendly. The development of artificial intelligence and machine learning algorithms has allowed for the interpretation and analysis of complex biosensor data. This review compares biosensing with current state-of-the-art diagnostic technology. After incorporating biosensors with artificial intelligence in an internet of things platform, they will have enormous potential and market value in the future for personalized healthcare. Based on various device performances and impacts, sensing methods, designs, compatibilities, functionalities, technology integrations, and developments are systematically discussed in this article. The primary objective of this review was to present a comprehensive discussion from the point of view of both technological advancements and translational wisdom. It is essential to have intelligent point-of-care devices with digital technologies for real-time healthcare management. The vision of the future healthcare industry encompasses a range of biosensing methods that offer a glimpse into new possibilities for the market.
1. Introduction
Intelligent point-of-care (PoC) devices are crucial for managing healthcare and making decisions in real-time.1,2 The internet of things (IoT) and associated health applications are increasing the commercial value of sensors. Biosensor nanotechnology is used to design wearable electronic devices with advanced materials.3–5 The vision of the future that encompasses a range of sensing devices offers a glimpse into new possibilities whose impact is expected to have a positive effect on the healthcare industry.6,7 Intelligent sensor devices promote the transformation of healthcare from a conventional hospital-based to a patient-based approach by means of monitoring an individual's biochemical signs, such as perspiration, blood, interstitial fluids, tears, and wound fluids, for diagnosis and treatment in a smart home care setting.6,8–10 An innovative, worthwhile, and cost-effective solution could be provided for every healthcare application by a biosensor and an electronic system with computing support. This review emphasizes the efficacy of point-of-care (PoC) devices in field diagnostics with low resource settings; for example, where cost-effectiveness is essential or there is a low power supply or input, making such systems suitable for low-income countries for the quantification of certain bioanalyses, such as nucleic acids or proteins, to aid clinical decisions.11–14 Devices configured for prognosis integrate sensor products that include processors, memory devices, and computing support to offer a comprehensive product that is simple to operate.
The internet of medical things (IoMT) has inspired scientists to create new ‘smart’ healthcare systems that focus on early diagnosis, controlling disease spread, improving knowledge, and effective treatment and that support the use of technologies with cybersecurity-attentive strategies, thoughtful planning, and clear policies within healthcare establishments.15,16 Several studies have reviewed the various services and applications of IoT in wearable devices and smartphones for diagnostic to control-based healthcare.15–17 Additionally, display devices may be coupled to a visual display system to facilitate real-time monitoring. Therefore, there is a significant demand in the market for miniaturized biosensors that can overcome the difficulties presented by conventional techniques. Furthermore, analytical devices for climate neutral biosensing would be an important step toward sustainable society.18 Healthy healthcare management models require the achievement of net zero carbon emissions in health systems.18,19 According to existing requirements, the path to zero emissions is inextricably linked with the development of more resilient health systems and the achievement of global health goals. The objective of this article is to emphasize the climate neutral directions for utilizing digital and sustainable healthcare systems. In this paper, we present a review of the sensing methods, designs, compatibilities, functionalities, technology integrations, and developments based on various device performances and their impact. This article defines various sensor technologies and their usability in healthcare devices. Furthermore, new scenarios, their importance, challenges, innovative methods, and prospects are discussed in terms of next-generation technologies for sustainable healthcare management.
2. Sensing methods, designs, and technology for disease prognosis
It is considered that a more effective public health model could be achieved through the early management of control measures. When developing the most efficient logistic support for training, administering, and developing research items for control activities, it is necessary to evaluate all possible solutions and research avenues considering the risk. Fig. 1 shows some forward-thinking approaches to the design of sensors, technological advancements, and methodical research and development in the field of healthcare diagnosis and treatment.
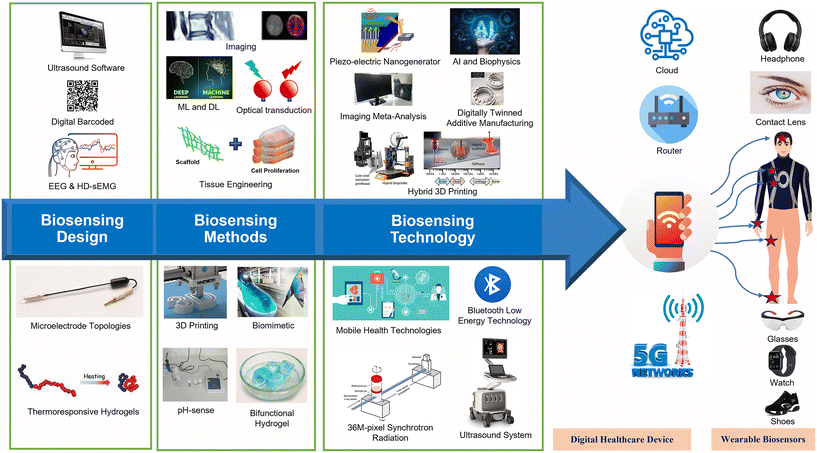 |
| Fig. 1 Diagnostic and therapeutic innovations enabled by novel sensor design, technology, and development methods. | |
Table 1 lists some innovative designs and methods reported for the development of sensor technologies.20–37 Understanding various mechanisms through methodogical investigation is important. Here, 3D printed CNT electrodes, distinct interaction, Janus 2D materials, biomimetic receptor, pH-sensitive drug sensing, hematopoietic stem cell differentiations are some of the unique processes involved in developing methods for sensing purposes.32–37
Table 1 Recently reported designs, methods, and sensing technologies developed using innovative design and procedures for sensing in relation to analysis, comparison, evaluation, integration, and implementation
Category |
Detail |
Ref. |
Design |
Medical software based on sensor design |
20
|
Digital barcoded particles and impedance spectroscopy |
21
|
Integrated EEG and HD-sEMG acquisitions |
22
|
Elliptical-shaped planar Hall sensor |
23
|
Microelectrode topologies for electrochemical oxygen sensing |
24
|
Thermoresponsive polyamine cross-linked perfluoropolyether hydrogels |
25
|
Methods |
Photolithography and light-cured inkjet printing methods |
26
|
Imaging methods in mechanosensing |
27
|
Multi-dimensional cardio-mechanical signals |
28
|
Human posture detection based on wearable devices |
29
|
Self-powered tetherless sensing and opto-spintronic RF-to-optical transduction |
30
|
Silicate-based electroconductive inks for electronics and tissue engineering |
31
|
3D printed CNT electrodes to the virulence factor pyocyanin |
32
|
Distinct interaction of carbohydrates with lectins |
33
|
Janus 2D materials |
34
|
Selective glucose sensing using a biomimetic receptor |
35
|
Nano-carriers based on pH-sensitive drug release |
36
|
Nanostructured bifunctional hydrogels for hematopoietic stem cell differentiation |
37
|
Biosensing technology |
Tunable seesaw-like 3D capacitive sensor |
38
|
All-fiber pyro- and piezoelectric nanogenerator for healthcare monitoring |
39
|
Medical biophysics using artificial intelligence and mathematical modeling |
40
|
The role of computer remote monitoring technology |
41
|
Digitally twinned additive manufacturing |
42
|
Molecular imaging meta-analysis |
43
|
Integrating digital light processing |
44
|
3D-printable carbon nanotubes-based composite |
45
|
Mobile health technologies for health record |
46
|
Development of a real-time technology to facilitate clinical applications |
47
|
Biomedical REAl-Time Health Evaluation (BREATHE) |
48
|
Motion and muscle artifact removal validation |
49
|
36M-pixel synchrotron radiation micro-CT |
50
|
An ultrasound-based biomedical system |
51
|
Vitamin D-conjugated gold nanoparticles |
52
|
Healthcare has advanced with biological digital sensor systems, which enable more sustainable medical monitoring. These systems use cutting-edge technology to non-invasively track vital signs, biochemical markers, and physiological states in real time, providing important data for early disease detection, management, and individualized treatment regimens. They contribute to sustainable medical monitoring through numerous environmental and operational efficiency factors. Biological digital sensor systems have a lower environmental impact than traditional medical monitoring equipment, demonstrating their better sustainability. These sensors reduce healthcare's carbon footprint by using less material and energy. Their non-invasive nature and remote patient monitoring reduce hospital visits and throwaway medical supplies, further supporting sustainability goals.2–4
These technologies have been applied to innovate and develop low-power sensor modes that can enable devices to function for long periods without compromising their performance. These modes typically use innovative algorithms and energy-efficient components to substantially minimize sensor power consumption, while increasing the battery life and therefore decreasing the battery replacement requirements. This extends the device's lifespan, reduces electronic waste, and thus maintain the flow of health data. Low-power sensor modes further boost patient compliance and thus the effectiveness of remote health monitoring. Patients are more likely to follow monitoring regimens if devices require less maintenance and last longer. For chronic disease management, continuous data gathering is essential for treatment adjustments. Low-power modes allow biological digital sensors to be used in distant or underserved locations with limited healthcare access. By making sensors work for long periods without sophisticated infrastructure or frequent maintenance, these technologies can improve healthcare accessibility for hard-to-reach people by enabling high-quality and remote monitoring.6–9
2.1. Biosensing materials, designs, and procedures
Biosensor designs are based on integrating diverse elements, including electronics, structural, and computational aspects, as well as spectroscopic methods, electrodes, and thermoresponsive sensing analytes, often each with a unique mechanism.20–25 Diverse methods of detection, such as photolithography, imaging, cardio-mechanical signal detection, posture detection based on wearable devices, self-powered tetherless detection, and silicate-based electroconductive mechanisms, are used in different studies.26–31 They offer a solution to issues related to the life-threatening potential by the delayed treatment of diseases and complications due to the fact that not all segments of human society have the financial means to pay for such specialized diagnoses. Physiological, aural, gustatory, olfactory, and haptic stimuli, as well as touch, taste, and smell experiences, and other sensory experiences in the environment or on the human body, can be useful in today's world and can be exploited in sensing applications.
2.2. Nanobiosensing technology
Nanobiosensors, which use nanoparticles, are revolutionizing medical diagnosis. These sensors detect biomarkers at low concentrations with high sensitivity and specificity, making them essential for early illness detection. Graphene-based nanobiosensors can detect cancer biomarkers with unprecedented sensitivity, allowing earlier diagnosis and better patient outcomes.5
Nanobiosensors' fast response time contrasts with the typical lengthy processes for laboratory testing. In emergencies, like suspected myocardial infarction, rapid cardiac biomarker testing can greatly impact treatment decisions and outcomes. Nanobiosensors also enable non-invasive and minimally invasive health monitoring. For diabetic patients, wearable nanobiosensors can continually measure glucose levels without the need for blood sampling, thereby improving compliance and the patients' quality of life.6
Nanobiosensors combined with digital health technology enable remote patient monitoring and continuous health assessment outside of the typical healthcare settings. In chronic disease management, this capability makes healthcare delivery more convenient and efficient. Magnetic nanobiosensors use nanoparticles' magnetic characteristics and can often detect tumor-related proteins earlier than standard imaging methods. Early disease identification by such sensors could improve patient prognosis and survival by optimizing therapies.10
For the sake of being more specific, the focus is on the development of intelligent wearable nanobiosensing devices. These devices should be capable of being handled by laypeople and should assist in the continuous monitoring of vital parameters. As a result, the patient would be able to receive timely treatment and avoid major catastrophes. Real-time monitoring and reporting systems are essential for efficient healthcare management. Recent public health concerns, such as emerging, zoonotic, and lifestyle diseases, and antimicrobial resistance, require device-supported systems to understand and control diseases. Guidance and surveillance systems based on evidence have the potential to strengthen control by preventing transmission, eliminating risks, and controlling the progression of a disease. Biosensing technologies based on tunable seesaw-like 3D fiber pyro- and piezo-electric nanogenerators, AI-biophysics, and computational and digitally twinned additive manufacturing have been used extensively for monitoring and data collection.38–42 Furthermore, meta-analysis, integrated digital light processing, 3D printing, mobile health, real-time processing, motion understanding, synchrotron radiation utilization, and ultrasound and nanomaterial-based technologies facilitate quality and quantitative data results.43–52Table 2 lists some biosensing materials, including conductive polymers, nanoparticles, and biocompatible materials.
Table 2 Overview of biosensing materials, design considerations, and integration procedures
Material category |
Specific materials |
Properties |
Selection criteria |
Application in sensor design |
Integration procedures |
Conductive polymers |
Polypyrrole, PEDOT |
High conductivity, biocompatibility |
Conductivity, environmental stability |
Transducers, electrodes |
Chemical vapor deposition, electrochemical polymerization |
Nanoparticles |
Gold nanoparticles, quantum dots |
High surface area, enhanced catalytic properties |
Size, surface area, reactivity |
Signal amplification, sensitivity enhancement |
Nanoparticle synthesis, functionalization, and immobilization |
Bio-compatible materials |
Silicones, hydrogels |
Non-toxicity, flexibility, permeability to bio-analytes |
Biocompatibility, mechanical properties |
Wearable sensors, implantable devices |
Molding, curing, 3D printing |
Transducers and electrodes use conductive polymers like polypyrrole and PEDOT due to their excellent conductivity and biocompatibility. Based on conductivity and environmental stability, these materials have been combined in sensors through chemical vapor deposition and electrochemical polymerization techniques. Gold nanoparticles and quantum dots have high surface areas and catalytic capabilities. They can improve biosensor signal amplification and sensitivity through their size, surface area, and reactivity effects. Nanoparticle integration requires their synthesis, functionalization, and immobilization. Finally, silicones and hydrogels are biocompatible, flexible, and bio-analyte-permeable, making them ideal for wearable and implanted devices. For sensor integration, biocompatible and mechanically sound materials are molded, cured, or 3D printed. Table 2 shows the importance of material selection, their quality, and the painstaking processes required to develop and build dependable biosensing devices.
3. Prognostic complications and technological requirements
The diagnosis of many diseases has gotten more difficult in the modern world due to the arrival of emerging pathogens and new environments. Nonetheless, quick developments in the field of biomedical instruments are balancing the additional difficulties.
3.1. Disease markers and sensing process
Conventional blood sample analysis (blood testing) is quite common in hospitals and clinics for the evaluation of specific metabolites, which are potential carriers of disease. For example, diagnosis of the SARS-CoV-2 virus is mostly performed by reverse transcript polymerase chain reaction (RT-PCR).53 This technique uses DNA or RNA receptors for the identification of SARS-CoV-2 RNA strands. Furthermore, because of the rapid advancements that have been made in the field of biomedical instrumentation, doctors are now more likely to accurately diagnose a variety of diseases and the complications associated with them. Conventional blood sampling, also known as blood testing, is performed quite frequently in hospitals and clinics for the purpose of determining the presence of metabolites that may be potential carriers of disease through a variety of techniques and instrumentation. For example, the reverse transcript polymerase chain reaction (RT-PCR) is commonly utilized for making a diagnosis of the SARS-CoV-2 virus.53
Meanwhile, infections and diseases caused by bacteria are spreading rapidly throughout the world. The consumption of raw, undercooked, or contaminated food has been reported to be the root cause of a variety of foodborne diseases caused by bacteria. These diseases include cholera, diarrhea, meningitis, and gastritis, to name just a few. Further, it is well established that the latter contributes to the development of stomach ulcers and colon cancer. Typical causative bacterial species are Salmonella, E. coli, H. pylori, and L. monocytogenes.54,55 The recognition of SARS-CoV-2 RNA strands can be achieved using DNA or RNA receptors with this method. Furthermore, RT-PCR and the enzyme-linked immunosorbent assay (ELISA) are frequently used in serological tests for the detection of chikungunya virus, dengue virus, and bacterial species, such as salmonella and H. pylori.5,8–10 ELISA is also used for the detection of cholesterol, glycated hemoglobin (HbA1C) and cardiac troponin-I, among other things. In addition to these methods, specialized equipment, such as electrocardiogram (ECG), electroencephalogram (EEG), and electromyogram (EMG) machines, are utilized to monitor the rate of the heartbeat, cognitive conditions, and muscular activities, respectively. Most of the time, imaging methods, such as computed tomography (CT) scanning and magnetic resonance imaging (MRI), are utilized to visualize the precise location(s) of cancerous and tumor cells. The use of thermal imaging techniques is also common to analyze the temperatures of the plantar feet of diabetic patients when they have foot ulcers (DFU).56
Moreover, diabetes can cause severe comorbid situations and is therefore referred to as a “silent killer”. Diabetes can cause a number of serious health complications, including kidney disease, foot ulceration (which, in chronic cases, may require amputation), retinopathy, high blood pressure, and cerebral stroke. This is because diabetes and its complications and comorbidities often reinforce the formation of cancer or tumor cells, which can ultimately lead to death.57 Despite significant technological advances in healthcare diagnostics, conventional methodologies still suffer from serious drawbacks.
3.2. Emerging risks and technological limitations
Global public health risks should be fought with innovative solutions that should strengthen identification, diagnosis, treatment, and control. The development of such connected health platforms can be made possible through the combination of artificial intelligence (AI) capabilities and miniaturized sensors. Understanding and refining measurement technology, its development, cost-effectiveness, adaptability, and the supply chain for products are real challenges today that research and development businesses need to focus on. Today, a multitude of experts are required to solve the most demanding sensing measurement issues and their integration with appropriate technology. Serious and continuous efforts are required for overcoming the regular challenges. In addition to pathogenic species, complications caused by the excessive consumption of unhealthy foods or junk food are also common throughout the entire world. This kind of food is a source of high cholesterol and fat content, which in turn leads to a high risk of arteries becoming blocked, preventing blood from flowing through them. The latter is extremely fatal, as it can result in complications, such as hypertension and acute myocardial infarction (heart attack). Consuming junk food can also lead to diabetes, a condition characterized by a pancreas that is unable to function properly.
4. Methodologies for wearable biosensor devices and associated digital technology
Digital representation by a device based on a biosensor can provide prompt information, allowing patients to get the care they need as quickly as possible. Technological advancements in the field of nanoscience and nanotechnology have allowed the development of novel nanobiosensors for PoC diagnostics. Incorporating appropriate sensor solutions developed by researchers based on data from experiments into technological products is of critical importance. The goal of the current field of sensor science and technology is to develop methods by which any measurable value or state can be converted into a digital representation by a device based on reliable performance. In addition to their usefulness as PoC devices, biosensors can also reduce the amount of time patients have to wait for the results of conventional tests. Recent advances in wearable biomedical sensors for products have proven this to be a challenging task. As a result, most of the research and development efforts have been directed toward developing novel approaches and methods to solve the sensing depth, as well as their incorporation into appropriate technologies. Fig. 2 shows some sensing methodologies and technological avenues for the development of healthcare devices and methods.
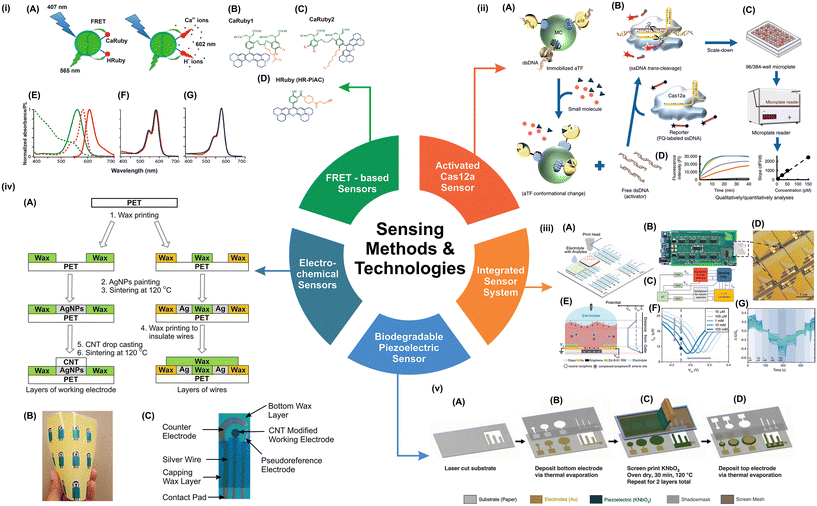 |
| Fig. 2 Sensing methodologies and technological potential for the development of healthcare devices and methods. (i) (A) Principle of FRET-based red-emitting ion sensors. When a green-emitting quantum dot (QD: here CANdot®565) donor is coupled to a red-emitting rhodamine-based ion sensor, an analyte-dependent FRET signal is produced upon donor excitation at 405 nm. The custom red-emitting ion sensors used here are Ca2+ (or H+) indicators, whose emission is suppressed by PET in the absence of their ligand. The binding of an analyte causes a strong fluorescence peak at 602 nm; (B–D). All sensors are based on a rhodamine moiety that has been extended (blue). For the lower and higher affinity families, CaRuby1 (M-mM range) and CaRuby2 (sub-M range), respectively, as the two Ca2+ sensor families incorporate a BAPTA moiety (green), without (B) and with (C) an oxygen introduced on one of the aromatic ring of the BAPTA. The pH sensor family (HRubies), (D) is distinguished by the addition of a phenol rather than a BAPTA. Through a piperazine carbamate link, HR-PiAC bears an alkyne moiety at the ortho position of the phenol. (E–G) Spectral properties of the retained donor/acceptor pairs' (E) normalized absorbance and emission spectra (dashed and plain lines, respectively) of QD565 (green) and CaRu-Me (red). CaRu-Me and QDCaRu-Me KDs were similar, as expected, because there was only a slight Ca2+ sensitivity in CaRu-Me absorbance when switching from an EGTA- to 2 mM Ca2+-containing solution. CaRu2-F and HR-PiAC should have similar properties because their absorbance is Ca2+ and pH insensitive, as shown in panels (F) and (G), where blue and red traces represent the absence and presence of the analyte, respectively.58 (i) Illustrations adapted from ref. 58, CC BY 4.0 (http://creativecommons.org/licenses/by/4.0/), (ii) schematic diagram of the CaT-SMelor (sensing platform of CRISPR-Cas12a and allosteric transcription factors, aTF) functionality (A), on microcrystalline cellulose, aTF fused to a cellulose-binding domain (CBD-aTF), was immobilized. The functional dsDNA contained both the PAM sequence and the binding motif of the corresponding aTF, and it specifically binds the aTF's DNA-binding domain. aTF's conformation was changed in the presence of the target small molecule, resulting in the dissociation of dsDNA from the aTF-binding domain.59 (B) Cleaving of a fluorophore quencher (FQ)-labeled ssDNA probe is initiated by activated Cas12a. (C and D) Target small molecules can be analyzed qualitatively or quantitatively by measuring the shift in the fluorescence signal.59 (ii) Illustrations adapted from ref. 59, CC BY 4.0 (http://creativecommons.org/licenses/by/4.0/). (iii) (A) Schematic of a sensing chip with NN sensor units (N = 16 in this paper). Multiplexed measurements are possible by printing different surface functionalization membranes onto different regions of the sensor array. (B) Photograph of the measurement system and sensor array taken with an optical camera. (C) Color-coded block diagram of the measurement system with dashed boxes in B. (D) Graphene sensing arrays on a glass wafer as seen through a microscope. 1 cm is the scale bar. (E) Individual sensing unit schematic with the ion-sensitive surface functionalization membrane. On the right, the electrostatic potential as a function of distance from the graphene surface is depicted. ISM: ion-sensitive membrane; VM: membrane potential; VGS: gate to source voltage; VDS: drain to source voltage; VS: potential at source. (F) I–V curves were shifted to the left in a typical device from a Na+ ISM functionalized sensing chip with an increased sodium ion concentration and VDS = 300 mV. The black dashed line represents the current level at VGS = 0.1 V, and the left-shift of the I–V curves causes a decrease in the current. (G) Normalized conductance transient responses of 215 working sensing units to changing concentrations of ionized sodium at VDS = 300 mV and VGS = 0.1 V.60 (iii) Illustrations adapted from ref. 60, CC BY 4.0 (http://creativecommons.org/licenses/by/4.0/). (iv) A. Fabrication and layering of hand-painted CNT/AgNPs electrochemical sensors. The fabrication steps 1–6 were completed in order. In step 4, a wax layer was selectively printed to insulate wires, and in steps 5 and 6, CNTs were drop-cast on the working electrode before sintering. (B) Flexibility of 12 hand-painted CNT/AgNPs electrochemical sensors on a wax-on-plastic platform. (C) Hand-painted CNT/AgNPs electrochemical sensor image.61 (iv) Illustrations adapted from ref. 61, CC BY 4.0 (http://creativecommons.org/licenses/by/4.0/). (v) (A) Laser cutting the substrate for cantilever devices, (B) thermal evaporation deposition of the Au bottom electrode, (C) screen printing of the KNbO3 ink, which was repeated once, with a 30 min oven drying step at 120 °C between layers, and (D) thermal evaporation of the Au top electrode.62 (v) Illustrations adapted from ref. 62, CC BY 4.0 (http://creativecommons.org/licenses/by/4.0/). | |
4.1. Wearable biosensor functionality
The functionality of diverse and unique materials offers unique sensing methodologies and technological avenues that may be useful for the development of healthcare devices. The principle of FRET-based red-emitting ion sensors and Cas12a activation offers opportunities for unique sensing methods and further functionality for development in the biomedical field.58,59 Studies on these have shown that the chemical structure of such sensors can be based on a color indication against variable molecular compositions. Further, it was also shown that the free dsDNA then binds to the Cas12a-crRNA complex, triggering Cas12a's nonspecific ssDNA trans cleavage activity (activated Cas12a). Cas12a is thus activated and begins cleaving a fluorophore quencher (FQ)-labeled ssDNA probe. Highly integrated sensing systems can be developed through multiplexed measurements by using sensor arrays based on electronic and electrochemical methods.60 It was shown that hand-fabricated CNT/AgNPs electrodes using a wax-on-plastic platforms could be developed for electro-immunosensing applications.61 In another study, printing paper was designed for use with printed electronics because of its low surface roughness (2–3 m) and robust thermal properties (stable up to 200 °C)62 in comparison to the surface roughness and thermal stability of other biodegradable substrates.
4.2. Wearable biosensor technology
The integration of appropriate sensor solutions into technology products based on experimental data is very important. Among the key aims in the current field of sensor science and technology is to take advantage of appropriate measurable values or states that can be converted into a digital representation by a device based on reliable performance. In this way, biosensors will be able to provide accurate and prompt information, allowing patients to get the care they need as quickly as possible. The incorporation of nanomaterials into suitable substrates has opened up a multitude of possibilities for anchoring bioreceptors, such as enzymes, DNA, and antibodies, along with provisions for further functionalization, resulting in rapid and highly sensitive biosensing platforms with very low detection limits. The miniaturization of biosensing devices has been greatly facilitated by the development of advances in capabilities for designing, fabricating, and analyzing in the micro/nano regime. A glucose oxidase (GOx)-modified electrode strip glucometer that can display blood glucose levels in a pocket-friendly module is a good illustration of a typical example of this. These glucometers are commercially available and are used by many people. The development of wearable nanobiosensing devices, which offer provisions for the constant and real-time monitoring of vital parameters, is essential to the focus on establishing affordable healthcare; however, this development is heavily dependent on the development of wearable nanobiosensing devices. Wearable biosensor technology is a rapidly developing area of research in the discipline of modern healthcare and biomedical instrumentation. It is estimated that the global market value of wearable sensors will increase annually by 24.1% from 2017 to 2023, eventually reaching the target of $99 billion.64 The global market value of wearable sensors was reported to have reached the staggering mark of $22 billion in 2016. The need for wearable biosensing devices has emerged in response to rising dangers in the physical and mental health conditions of human beings, as well as the universal demand for real-time patient monitoring that requires as little intervention from outside sources as possible, and the increased likelihood that favorable outcomes will be achieved. It is possible to create highly sensitive, efficient, and cost-effective smart wound dressings for real-time health monitoring by combining the benefits offered by nanomodified electrodes with their integration with miniaturized electronics.65,66
4.3. AI-based biosensor technology
The benefit of artificial intelligence derives from the implementation of IoT-based technology, and, as a result, relies on the machine learning feature to make recommendations regarding suitable doctors or medications, or even to automatically call an ambulance in the event of an emergency. These recommendations are made based on the patient's previous medical records, which can be accessed from dedicated cloud servers. Furthermore, AI relies on this feature to make recommendations about suitable doctors or medications. To make smart decisions, the following factors are considered: (1) data, where devices contribute to a common data model; (2) parallel data processing, from acquisition to storage; (3) big data analytics and the use of AI for real-time decision; (4) privacy-oriented data processing with encryption and security.67
Due to recent developments in IoT, AI, and big data analytics, a continuous flow of data along with a patient's medical history can efficiently support personalized healthcare and can automate many tasks, such as archiving medical data and evaluating the effectiveness of medical interventions. Therefore, the implementation of highly reliable, accurate, miniaturized, sensitive, and efficient nanobiosensing devices possesses huge market potential to revolutionize and establish next-generation connected health platforms.67,68 In the following section we highlight and evaluate the latest advancements in the field of wearable biosensing devices that are integrated with miniaturized electronics and AI, as well as devices that have the potential to be translated into IoT and AI platforms. In addition, this section focuses on devices that have the potential to be integrated into these fields. Smart sensor modules typically consist of a cost-effective flexible sensing strip, preferably modified with a suitable bio-nano hybrid, along with a portable electronic microcontroller integrated with a touch screen display (i.e., smartphone or personal digital assistant (PDAs)) and Bluetooth. The latter is an important part of the overall module because it can enable real-time monitoring and wireless data transmission to nearby hospitals and clinics. This will allow for prompt interventions, which will ultimately lead to an effective connected health platform. In addition, the incorporation of Bluetooth feature would make it possible to manage local databases using cloud computing, with the electronic microcontroller serving as the central hub. For more effective patient monitoring and diagnostics, dedicated cloud servers may also be set up in nearby centralized clinics or hospitals at specific locations.
4.4. Fabrication, electrochemical, and other sensing performances
A recent study by Lim et al. focused on the fabrication of an all-in-one flexible wireless sodium (Na+) sensor (Na+).69 The latter was developed using a combination of microfabrication, screen printing, and electrodeposition techniques, whereby the working/sensing electrode area consisted of Au/CNT/Au nanocomposites. That recent study presented a schematic of the flexible wireless Na+ sensor along with its various components used during its fabrication. Here, a wireless, modular sodium sensor system was outlined, where electrochemical deposition was used to create a multilayered integrated device and sodium sensor on Au pads.69 The initial Au working area was defined as a 2 mm diameter region using conventional photolithography. Then, pristine SWCNTs were continuously dropped on the circular Au surface, followed by the electrodeposition of Au nanoparticles. To ensure the selectivity of the flexible sensor toward Na+, a 3 μL droplet of a synthesized ion-selective membrane solution was further coated on the nanomodified electrodes.
The development of the sensor through fabrication based on electrochemical, and other sensing performances is shown in Fig. 3. One study reported paper made from CF/GO/cellulose, whereby to enable the assembly of GO sheets onto the surface of the cellulose fibers, cationic polyacrylamide (CPAM) was used to control the interfacial interactions.70 In same study, high-performance, long-lasting paper-based sensors for portable electrochemical detection were demonstrated.70 In another study, a lens-free optofluidic plasmonic sensor was used for the real-time and label-free monitoring of molecular binding events over a wide field-of-view.71 Also, Roy et al. developed screen-printed microfluidic technology to monitor diabetic foot ulcers using a portable potentiostat (DFU).72
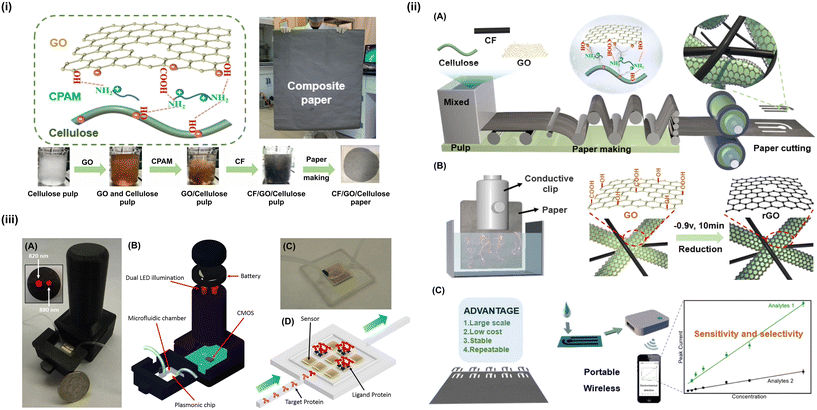 |
| Fig. 3 Development of sensors through various fabrication processes based on electrochemical and other sensing performances. (i) Preparation and characterization of CF/GO/cellulose paper.70 (i) Illustrations adapted from ref. 70, CC BY 4.0 (http://creativecommons.org/licenses/by/4.0/). (ii) Large-scale preparation of high-performance paper-based electrochemical sensors. (A) Papermaking on a large scale. By controlling interfacial interactions, the composite paper was prepared and composed of cellulose, carbon fibers (CFs, confers conductivity) and graphene oxide (GO, confers electrocatalytic activities). (B) GO reduction via electrochemistry. Electrochemistry is a simple and scalable method for GO reduction. (C) Paper-based electrochemical sensor concept. The goal was to develop high-performance, long-lasting paper-based sensors for portable electrochemical detection.70 (ii) Illustrations adapted from ref. 70, CC BY 4.0 (http://creativecommons.org/licenses/by/4.0/). (iii) (A) Photo of an on-chip sensing device with a plasmonic substrate at the bottom of a microfluidic channel. Inset: the lensless device's head, which contains two spectrally distinct LEDs (with peak wavelengths of 820 nm and 880 nm) for simultaneous illumination of the microfluidic chip. (B) On-chip biosensing device composed of a battery, two LEDs, a plasmonic chip, a microfluidic chamber, and a CMOS imager chip comprise. (C) Illustration of the microfluidic system's inlet and outlet ports. (D) Schematic of the flow-over scheme for delivering target samples to the plasmonic substrate.71 (iii) Illustrations adapted from ref. 71, CC BY 4.0 (http://creativecommons.org/licenses/by/4.0/). | |
The fabrication of a nanomodified microfluidic smart wound dressing accepted in that analysis was demonstrated in several studies. The microcontroller was then sealed on top of the tape at the rear of a band-aid. In such circumstances, Kapton tape served as a heat sink that could draw the microcontroller heat and dissipate it through the edges of the tape.73 Only the working electrode could be quantified electrochemically, which reduces the proof-of-concept costs. As supported by voltammetric and chronoamperometric analysis, they observed a linear performance between the sensor current and different concentrations of tyrosine, within 5 nM–500 μM, with detection limit and sensitivity considered at 0.71 nM and 0.67 μA nM−1 mm2, with reproducible results in both simulated and human serum samples noted in actual time.72 In contrast to the earlier all-in-one wireless Na+ sensor, Roy et al.'s bandage was extremely cost-effective, because it eliminates the need for tedious microfabrication of the bandage/electrode and due to the ease of integrating miniaturized electronics on screen-printed nano-enabled bandages for real-time data acquisition. Utilizing such intelligent wound dressings could enable the real-time monitoring of the ongoing condition of diabetic foot ulcers, resulting in more expedient treatment while mitigating the risks of comorbidities and, in the worst-case scenario, amputation.
Another study on the development of smart bandages was conducted by Kassal et al. for monitoring uric acid concentrations, as an indicator of wound status.74 Bandages were manufactured by screen printing Prussian blue carbon ink onto the surface and then printing as Ag/AgCl pseudo-reference electrodes. The overall design of the bandage was based on a standard two-electrode configuration, which is advantageous for device miniaturization and microcontroller integration. For the selective detection of uric acid, the Prussian blue carbon working electrode was modified with uricase enzyme via glutaraldehyde cross-linking. The bandage was then incorporated with a portable potentiostat the size of a credit card equipped with an RFID/NFC interface for data transmission to a smartphone or PDA. Real-time chronoamperometric analysis at −0.3 V versus Ag/AgCl indicated a linear response to uric acid concentrations between 100 and 800 μM. The sensitivity coefficient of the developed smart bandage in uric acid detection was reported to be ∼2.4 nA μM−1. Furthermore, repeated calibrations of the smart bandage-based sensor yielded a consistent sensitivity coefficient of ∼2.39 ± 0.04 nA μM−1. The wearable sensor exhibited a selective response solely toward uric acid, thus significantly negating interference due to common electroactive wound metabolites, such as ascorbic acid, creatinine, lactate, and glucose. The UA biosensor exhibited excellent linearity over the full physiological concentration range independent of the instrument, with R2 = 0.9987 for the CHI 440 electrochemical analyzer, R2 = 0.9985 for the wearable potentiostat, and with excellent agreement between the two instruments of R2 = 0.9967. The sensitivity coefficient (SC) of the biosensor was calculated from the slope of the calibration plots and found to equal −2.4 nA μM−1 UA with both instruments. Bending created natural mechanical stress on the wound dressings due to the human body's curvature, mobility, and flexing at the application site. The bandage was folded and released 80 times at 180° to test how mechanical deformation impacted the UA biosensor. Every 20 bends, the reaction to a 400 μM UA standard was monitored. It was found that after repeated bending stress, the smart bandage biosensor's electrochemical response did not change (RSD = 5.60%).74
The efficacy of paper microfluidics toward IoT/AI-based connected health platforms based on IoT/AI has recently been investigated with the development of a SARS-CoV-2 immunosensor, to monitor COVID-19 in possible patients. In 2020, COVID-19 transformed into a pandemic. Yakoh et al. created a paper-based lateral flow assay (LFA) immunosensor for detecting IgG, IgM, and spike protein.75 The hydrophobic sample boundaries were developed using a wax barrier on paper substrates. In addition to voltammetry and amperometry techniques, electrochemical impedance spectroscopy (EIS) has been extensively studied as a sensing tool. The use of small-amplitude sinusoidal signals at the electrode–electrolyte interface, within a frequency spectrum, enables distinguishing between bulk and interfacial processes. The calculation of the overall impedance follows Ohm's law and is given as the following equation (where the bold font in eqn (1) indicates the phasor notation):
|  | (1) |
where
Z(ω) = complex impedance generated over a defined frequency range,
= Re{Z(ω)} + jIm{Z(ω)}
V(ω) = sinusoidal voltage applied across an electrode system,
I(ω) = time-varying current flowing across the electrode system,
ω = angular frequency of the applied sinusoidal voltage signal.
In any electrode–electrolyte-based sensor, the bulk and interface regions yield unique impedance signatures at various frequencies that can aid analyzing the charge-transfer kinetics in greater detail, and ultimately sensor calibration with ultrahigh sensitivity and a low LOD.76
A wireless electronic health patch and piezoelectric energy harvesting and flexible sensing devices were developed with an integrated mechanism and fabricated in ultrathin devices for better usability77,78 (Fig. 4). In another work by Zhang et al., the development of a smartphone-based electrochemical sensor for the detection of bull serum albumin (BSA) and thrombin was investigated.79 The sensing strip for BSA detection was primarily a screen-printed carbon electrode based on a three-electrode setup.
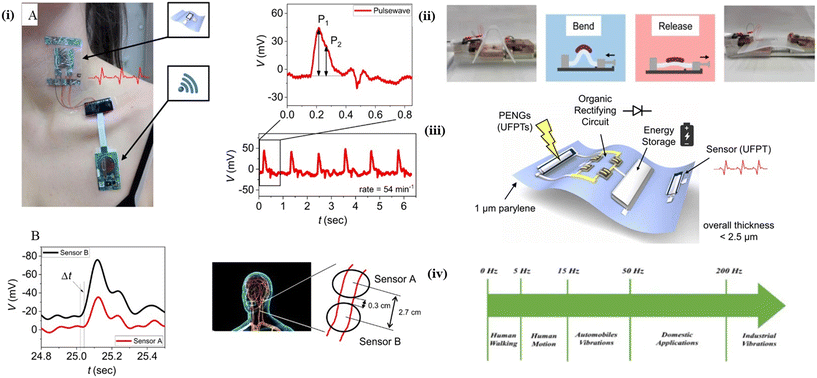 |
| Fig. 4 Wireless electronic health patch and self-powered wearable sensors. The wireless e-health patch's attachment points, where the ultraflexible transducer serves as an imperceptible sensor that adheres to the skin without adhesive. The patch has the ability to monitor (A) human pulse waves (with P1 and P2 peaks), from which the rate (here: 54 min−1 for a 32 year-old woman) and artery augmentation index AI (here: AI 56%) can be calculated. (B) Pulse wave velocity (PWV) is used to calculate the blood pressure of human arteries in the neck. The PWV is calculated by measuring the signal delay t for a given sensor distance x. PWV in this case was 9 m S−1 for a 34 year-old man.77 (i) Illustrations adapted from ref. 77, CC BY 4.0 (http://creativecommons.org/licenses/by/4.0/). (ii) Energy collection. Photographs and diagrams of the bending process.77 (ii) Illustrations adapted from ref. 77, CC BY 4.0 (http://creativecommons.org/licenses/by/4.0/). (iii) Piezoelectric energy harvesting and sensing device with extreme flexibility. A scheme depicting a variety of ultraflexible devices integrated on a 1 m thin parylene substrate, as well as photographs of the fabricated devices.77 (iii) Illustrations adapted from ref. 77, CC BY 4.0 (http://creativecommons.org/licenses/by/4.0/). (iv) Frequency levels of common mechanical energy sources for self-powered wearable sensors based on piezoelectric and triboelectric nanogenerators.78 (iv) Illustrations adapted from ref. 78, CC BY 4.0 (http://creativecommons.org/licenses/by/4.0/). | |
The carbon working electrode was modified with anti-BSA (antibody) and a layer of nitrocellulose membrane, relying on the typical immunoresponsive sensing of BSA in the 5 mM [Fe (CN)6]3−/4− redox probe. However, the sensing strip for the detection of thrombin was based on Au interdigitated electrodes. A characteristic of an electronic system indicates whether the sensor is properly associated, connected, or transmitted. The main constituent is any signal condition associated with the biosensor device that indicates the physical environment.
5. Wearable electrochemical biosensing performance
The electrosensing of thrombin was based on its ability to cleave the Arg–Gly bond of a defined octapeptide sequence linked to BSA, immobilized onto the Au interdigitated electrode surface. Higher concentrations of thrombin would then lead to a greater degree of Arg–Gly bonds and remove the linked BSA, which could be calibrated as a measure of enhanced charge-transfer kinetics at the electrode–electrolyte interface. The core of the smart biosensor was based on an Arduino microcontroller capable of communicating with an AD 5933 impedance analyzer chip. The latter sent clock signals through the ADF 4001 clock chip to generate a sinusoidal signal of 200 mV amplitude, within the frequency range 10 Hz to 10 kHz. The corresponding impedance spectra during BSA and thrombin detection, with the respective Rct values, were displayed on a smartphone by means of wireless data transmission. The LODs for BSA and thrombin, obtained by using the portable smartphone-based biosensor, were calculated to be 1.78 μg mL−1 and 2.97 ng ml−1, respectively, with a high degree of selectivity in a mixture of human serum albumin and an 11-peptide sequence.
Table 3 provides an overview of wearable sensors for biomedical applications. This table summarizes various wearable sensors designed for biomedical applications, highlighting their target analytes, sensing strategies, linear ranges, and sensitivities.
Table 3 List of recently developed wearable electrochemical biosensing platforms for healthcare monitoring applications
Sl. no. |
Wearable sensing surface |
Target analyte |
Sensing strategy |
Linear range |
Sensitivity |
Ref. |
1 |
PANI on bandage |
Wound pH |
Potentiometry |
2.69–8.00 |
56 mV pH−1 |
66
|
2 |
ZIF-67/C3N4 on cellulose paper |
S. aureus
|
Impedimetry |
1 fM–10 μM |
0.25 kΩ fM−1 mm2 |
80
|
3 |
Prussian blue carbon/uricase on PET sheets |
Uric acid |
Amperometry |
0–600 μM |
2.45 μA mM−1 |
81
|
4 |
Graphite on a cellulose-polyester cloth |
pH |
Amperometry |
6–9 |
4 mV pH−1 |
82
|
5 |
Graphene-AgNW on contact lens |
Intraocular pressure |
Frequency response analysis |
5–50 mm Hg |
2.64 MHz mm Hg−1 |
83
|
6 |
GOx/chit/CNTs on Prussian blue/Au electrodes |
Glucose |
Amperometry |
0–200 μM |
2.35 nA μM−1 |
84
|
LOx/chit/CNTs on Prussian blue/Au electrodes |
Lactate |
0–30 mM |
220 nA mM−1 |
7 |
Flavin/carbon-polyethylene film |
Wound pH |
Voltammetry |
2.55–8.12 |
60 mV pH−1 |
85
|
8 |
Laser-treated polyimide |
Wound pH |
Voltammetry |
2.8–8 |
56 mV pH−1 |
86
|
9 |
Uricase on carbon film |
Uric acid |
Amperometry, impedimetric |
0.2–1 mM |
Not specified |
87
|
Ag/PANI on bandage |
Wound pH |
5.5–8.5 |
10 |
LOx/chit/carbon electrodes-based eyeglasses |
Lactate |
Amperometry, potentiometry |
0.1–14 mM |
0.8 μA mM−1 |
88
|
Valinomycin/PVC/carbon electrodes-based eyeglasses |
Potassium |
0.1–100 mM |
60.6 mV mM−1 |
5.1. Wearable smart biomedical products
In the research and development section, the expert domain should contribute to the design and development of a conceptual sensor to measure physical entities. Improvement in performance requires innovation, technology, designs, prototypes, and testing for the development of sensor products, and support throughout the development cycle, from prototype to production. The reported wearable smart biomedical sensors mostly utilize separate battery-based power sources. When designing electronic circuits, this frequently necessitates a large number of empty slots, which can compromise the need for miniaturization. To produce effective miniaturized electronics, we need to follow Moore's law, which stipulates that the number of transistors per chip will double every 18 months. A large empty battery slot on the circuit board could be annoying. In addition, the disposability of batteries is one of the difficult issues, as the simple burning of electronic waste (e-waste) could produce biohazards. In recent years, extensive research has been conducted on the development of self-powered wearable or mobile biosensors to address these issues.
5.2. Self-powered sensors based on nanogenerators
This section highlight some fundamental self-powered nanogenerators and their applications in wearable biosensors based on artificial intelligence. In response to the drawbacks of implementing battery-powered smart wearable biosensors, the current strategy is to fabricate nanogenerators that can serve as a highly reliable source of renewable energy to generate electricity in order to power wearable sensors. As discovered by Wang and co-workers,89,90 nanogenerators employ suitable nanomaterials to transform mechanical energy (input) into electrical energy (output). The latter can be harnessed in two ways, either by piezoelectric effect or triboelectric effect, and the resulting devices are known, respectively, as piezoelectric nanogenerators (PENGs) or triboelectric nanogenerators (TENGs). These are essentially capacitive devices and therefore depend on the displacement current density JD for output power generation.91 The displacement current density is given as eqn (2): | 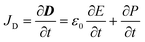 | (2) |
where D = electric displacement vector,
ε
0 = permittivity of free space,
E = electric field intensity,
P = polarization density.
Since there are no external electric fields while the latter is generated solely by induced polarization charges, the second term in eqn (2) becomes an essential factor in determining the output power density.91
The viability of self-powered nanogenerators lies in their ability to be integrated with miniaturized electronics for the development of personalized mobile healthcare devices. For example, Zhang and co-workers reported a PVDF-based flexible and implantable PVENG based on PVDF, which was capable of capturing energy from pulses generated in the ascending aorta.92 Their study also performed in vivo and in vitro studies of energy harvesting through vibrational artery pressure by employing a flexible and implantable PENG device, as shown in Fig. 6. The PVDF-based PENG device outputted 681 nW, 10.3 V, and 400 nA in vitro. One arterial pulse produced 7–9 nC. The PENG generated 1.5 V and 300 nA when implanted in a porcine's ascending aorta (in vivo). The PENG device's instantaneous output power peaked at 30 nW for 700 ms. The PVDF-based PENG could charge 1 F to 1.0 V in 40 s.
Another innovative research was carried out by Xue and co-workers on the development of a self-powered electronic skin to monitor glucose concentrations in body fluids.93 They employed a novel piezoenzymatic coupling reaction strategy employing GOx–ZnO nanowires, whereby mechanical pressure (due to bodily movements) could convert electric energy, which was in turn affected by the glucose concentration because of the electrocatalytic influence of GOx. The GOx enzyme was immobilized on an array of ZnO nanowires, while the latter were grown on titanium foil. The PENG device was manufactured by sandwiching an array of GOx–ZnO nanowires between two layers of 50 m thick aluminum foils covered with PDMS, the latter of which was used to prevent electrical leakage. The device's overall dimensions were 0.6 cm × 0.6 cm, allowing it to be easily integrated or implanted beneath the skin to monitor glucose levels. The developed PENG device yielded consistent variations in output voltage from 133–6 mV, as the glucose concentration increased from 0–0.125 ng L−1. The electrocatalytic oxidation of glucose molecules by GOx effectively increased the surface carrier density in the ZnO nanowire array, which eventually lowered the piezoelectric output voltage because of the piezoscreening effect. The PENG device also generated voltage responses to interferants, such as fructose and urea, that were significantly different from those of glucose, indicating that the PENG-based electronic skin was highly selective to the presence of glucose in bodily fluids.
In addition to PENGs, TENGs have also been incorporated into self-powered wearable biosensing units. Recent advancements in implantable TENG are beneficial for self-powered biomedical devices that operate at personalized level. They are also very useful for tracking and evaluating various physiological movements, particularly for chronic conditions like arrhythmia, atherosclerosis, hypertension, and coronary heart disease.94 Implantable TENGs had biocompatible electrodes, triboelectric layers, and spacers, with 50 μm thick of nanostructured polytetrafluoroethylene (n-PTFE) as one triboelectric layer and Al foil (100 μm thick) acting as both another triboelectric layer and the electrode.94 To improve leak-proof performance, structural stability, and biocompatibility, PTFE and PDMS (patterned pyramid arrays) layers were applied to the entire device. The implantable TENG was inserted between the epicardium and the pericardium in the pericardial sac, which was then attached to the pericardium at each corner. The operation of the TENG-based sensor was carried out based on the contraction and separation of triboelectric layers in tandem with the relaxation and contraction of the heart, generating a continuous electric output by coupling electrostatic induction and contact electrification. In vivo investigation of the self-power generation showed open-circuit voltage and short-circuit current of 10 V and 4 μA respectively.94 The heart rate recorded by the developed self-powered sensor was compared with a conventional electrocardiogram (ECG) output and was found to be ∼99% accurate for point-of-care applications. Fig. 5 shows a personalized healthcare model based on advanced sensing and information technology devices.
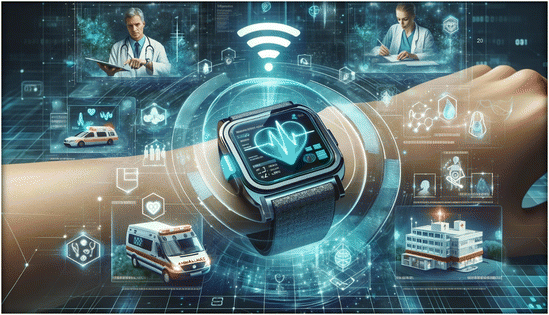 |
| Fig. 5 Outlining the features and operating principles of a wearable biosensing system, which can play a pivotal role in personalized healthcare models. This advanced device incorporates an array of sensors, utilizes artificial intelligence for data analysis, leverages cloud computing for storage and processing, and employs IoT technology for seamless connectivity. The system is designed to monitor vital health parameters in real-time, facilitating immediate detection and follow-up. It maintains a continuous, real-time connection with healthcare infrastructure, including hospitals, medical professionals, and ambulance services. This integrated approach can ensure timely medical intervention and enhances patient care by providing healthcare professionals with immediate access to critical health data. | |
5.3. Implantable sensor and digital health system
The digital health system has attracted special attention due to the explosive growth of biomedical applications and the rapid evolution of implantable devices and electronics. This technique involves the monitoring, tracking, and recording of a person's vital signs to collect information about the individual. Smartwatches, armbands, and glasses are examples of implantable or wearable sensors that have become an integral part of our daily lives. An implanted cardiac pacemaker was the first implantable health device manufactured for patients. Kaefer et al.95 created an implanted gold nanoparticle sensor for long-term body concentration monitoring. Fig. 6 shows the output performance of their implantable wearable sensor.
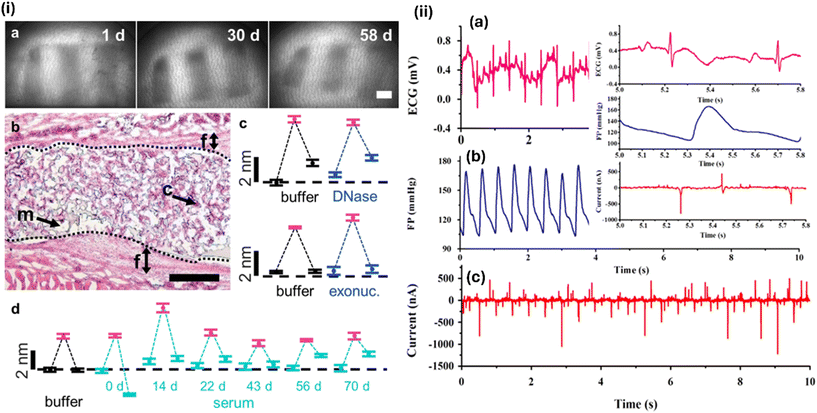 |
| Fig. 6 Long-term stability of an implanted sensor under physiological conditions: (i) (a) transcutaneous images over time (1, 30, and 58 days) post-implantation in rat skin, revealing the maintenance of integrity. (b) Histological section post 65 days, showing the well-integrated sensor architecture with minimal fibrous tissue formation, highlighting cellular integration into the sensor's macropores. Figures (c) and (d) demonstrate the sensor's consistent response to varying concentrations of kanamycin, even after exposure to DNA-degrading enzymes or long-term storage in rat serum, showcasing its reliable and reversible functionality. Reproduced with permission from ref. 95, Copyright © 2021 American Chemical Society. (ii) (a–c) Waveform of the output current, along with the fetal phonocardiography (FP) and electrocardiogram (ECG) signals, with the enlarged waveform presented in the inserted figure for a flexible polyvinylidene fluoride (PVDF) film coated by an aluminum layer piezoelectric implantable sensor. Reproduced with permission from ref. 92, Copyright © 2015 Elsevier Ltd. | |
Noninvasively, they evaluated tissue kanamycin levels in anesthetized rats implanted with a gold nanoparticle-based sensor. The implantable sensors showed comparable kanamycin tissue concentrations in the rats after two, three, and four weeks of implantation.95 The use of biosensors and digital health systems has improved diabetes control, which is a serious disease known as “the silent killer”. Such non-invasive glucose monitoring systems can track blood glucose continuously without uncomfortable finger-prick testing needed. This technique improves patient comfort and increases glucose level accuracy, decreasing diabetic complications. Biosensors and digital health systems can enable real-time data analysis and individualized healthcare. This integration empowers patients with personalized food, exercise, and medication advice for proactive diabetes treatment. By using biosensor data, machine learning algorithms can predict blood glucose changes and warn of hyperglycemic or hypoglycemic situations. These biosensor and digital system advancements improve diabetes control and promise a healthier future. They make diabetes care more effective, individualized, and less invasive by giving extensive insights and prediction tools.45,95
6. Recent advancements in sensor-based detection and diagnosis
Diverse disease categories caused by pathogenic microorganisms, genetic predispositions, or unhealthy behaviors are prevalent in society and many regions globally. They include infectious, non-infectious, lifestyle, and chronic diseases. Pandemic diseases, such as the SARS coronavirus, emerging diseases, such as monkeypox, and endemic diseases, such as visceral leishmaniasis, pose a threat to life and an economic burden on society and individuals. Several infectious or zoonotic diseases that can cause illness in humans have emerged in recent decades. The early detection or diagnosis promotes disease prevention directly. Early knowledge of the status of a disease or infection facilitates treatment and promotes a higher quality of life.
Integrated sensor device systems make the detection of diseases and the identification of microorganisms more accessible and accurate. Developed public health models can ensure adaptable, timely and quality support for eliminating and controlling healthcare-related infections. Table 4 presents some recent advancements in detection and diagnosis for biosensing applications.96–128 Sensors based on diverse functionalities and constituents, such as capacitive pressure sensing, healing wearable sensors, pressure, wireless, FRET-based genetically encoded sensors for silver ions, oxygen sensors on an optofluidic platform, location tracking, metamaterials based on soft tactile, electrospinning-based PVDF-TrFE nanofiber sensors, glycine–chitosan-based biodegradable piezoelectric sensors, and DNA-regulated CRISPR-Cas12a sensors, represent important advances in the biosensor field.96–106 Detection is based on diverse approaches, including paper-based devices, 3D-printing electrochemical, MoS2 quantum dots, 2D nanomaterial-enhanced plasmonic functionality, smartphone-integrated colorimetric, PVDF-TrFE nanofiber, electronic, machine learning, fluorescent DNA, non-enzymatic glucose, and silicon nanowire biosensing platforms for the (bio)detection of various analytes ranging from metal, pollutants, cells, genetic materials, and even post-translational modification.107–117 Biomedical real-time health evaluation is done to diagnose cancer, obesity, bipolar disorder, cardiac issues, SARS-CoV-2/COVID-19, cerebral palsy, diseased skin, etc., as well as for the detection of non-invasive signs, such as for sleep apnea.
Table 4 Recent advances in detection and diagnosis for biosensing platforms related to several applications
Category |
Detail |
Ref. |
Sensor |
Graphene porous foams for capacitive pressure sensing |
96
|
Transparent, healing, and adhesive hydrogel for wearable sensors |
97
|
High-performance pressure sensor for wearable electronics |
98
|
Battery-free wireless sensor for bacteria |
99
|
FRET-based genetically encoded sensor for silver ions |
100
|
Oxygen sensor on an optofluidic platform |
101
|
Enhanced location tracking in sensors |
102
|
Metamaterials enabling soft tactile sensors |
103
|
Electrospinning-based PVDF-TrFE nanofiber sensor |
104
|
Glycine–chitosan-based biodegradable piezoelectric sensor |
105
|
Functional DNA-regulated CRISPR-Cas12a sensor |
106
|
Detection |
Paper-based devices for environmental pollutants |
107
|
3D-printing electrochemical (bio)sensors |
108
|
MoS2 quantum dots for Fe3+ detection and cell imaging |
109
|
Electrochemical biosensor for miRNA detection |
110
|
2D nanomaterial-enhanced plasmonic biosensor |
111
|
Smartphone-integrated colorimetric sensor |
112
|
PVDF-TrFE nanofiber sensor |
113
|
Electronic nose sensor array signals and machine learning |
114
|
Fluorescent sensor for DNA detection |
115
|
Non-enzymatic glucose detection |
116
|
Silicon nanowire biosensing platforms for post-translational modification |
117
|
Diagnosis |
Biomedical REAI-Time Health Evaluation (BREATHE) kit |
118
|
Quantum sensors in diamond |
119
|
Mechanophore activation in hydrogels for cancer therapy |
120
|
Bioactive-functionalized gold nanoparticles for obesity control |
121
|
DNAzyme-based lithium-selective imaging for bipolar disorder patient understanding |
122
|
Cardiac signals for gastric fundus |
123
|
Electromyography sensors |
124
|
SARS-CoV-2/COVID-19 sensors |
125
|
Machine learning in cerebral palsy |
126
|
Non-invasive signs for sleep apnea |
127
|
Multisensor measurements in healthy and diseased skin |
128
|
6.1. Biomaterials compatibility, functionality, and diverse applications
Biocompatibility and functionality are studied through the testing and demonstration of human body adaptation, clinical characteristics, analytical chemistry, physicochemical behavior, in vitro experiments, animal models, and in vivo capabilities. Important compatibility testing for materials technology and biomedical devices is required to ensure their safe use with human physiological systems. Before launching a device, several regulatory issues, compliance, safety regulations, standards, and precautions must be addressed and passed to prevent any potential risk to the human body. In addition to other standards, biosensor products should offer a technological advantage based on sensitivity and specificity. The functionality of medical devices varies as a result of their distinctive characteristics. Due to a greater understanding of intricate biological mechanisms, various functionalities have emerged. The advancement of next-generation materials and materials is beneficial for biocompatibility testing and can accelerate the regulatory approval process for medical devices. Table 5 details the biocompatibilities of some sensor technologies, as well as their functionality and applications.129–158 Biocompatible materials for living cells, bone, biological fluid, yak hair, cellular loading, membrane systems for biomedical and electronics purposes have already studied.129–136 Functionalities related to cytotoxic, DNA binding, photocatalytic, H2O2 detection, antioxidant, transcutaneous measurement, eye-movement tracking, physiological conditions, mitochondria sensors for metabolic state, nanocomposites, gas permeability, DNA methylation, and cancer sensing are important research areas for healthcare devices.137–145 Applications include carboxymethylcellulose (CMC) optical fibers, silicon carbide sensors, smartphone-based colorimetric biosensors, molecularly imprinted sensors, flexible sensors, piezoelectric ceramics sensors, gas sensing, AI-based sensing, pattern recognition sensor arrays, BioMEMS sensor, electro-immunosensing, strain monitoring, and thermal biosensing application for biomedical healthcare.146–158
Table 5 Recently developed biosensing platforms, indicating both the biocompatibilities of sensor materials and technologies as well as the variety of the functions that these offer and the many different applications of the sensor technologies
Category |
Detail |
Ref. |
Biocompatibility |
Biocompatible carbon dots-decorated α-FeOOH nanohybrid sensor for wastewater and living cells |
129
|
Biocompatibility for bone replacement materials |
130
|
Biocompatible polymers for electronic applications |
131
|
Thin-film transistor for biological fluid sensing |
132
|
Bioplastic film derived from discarded yak hair |
133
|
Biomechanical cellular loading and compliance experiments |
134
|
Biological impact of bioconjugated gold nanoparticles |
135
|
A composite membrane system for biomedical purposes |
136
|
Functionality |
Cytotoxic, antimitotic, DNA binding, photocatalytic, H2O2 sensing, and antioxidant properties |
137
|
Transcutaneous measurement of essential vitamins |
138
|
Eye-movement tracking |
139
|
Monitoring regional body temperature |
140
|
Mitochondria sensors for the metabolic state in glioblastoma |
141
|
Chitosan–ZnO nanocomposites |
142
|
Gas-permeable sensors for heart monitoring |
143
|
Biosensor for detection of DNA methylation |
144
|
Graphene oxide for cancer sensing |
145
|
Application |
Carboxymethyl cellulose (CMC) optical fibers for environment |
146
|
Silicon carbide for healthcare applications |
147
|
Smartphone-based colorimetric biosensors |
148
|
Molecularly imprinted nanoparticles for biomedical applications |
149
|
Flexible sensors for biomedical applications |
150
|
Piezoelectric ceramics for biomedical applications |
151
|
Graphene nanoplatelets for gas sensing aerogels |
152
|
AI-based applications in medical technologies |
153
|
Pattern-recognition-based sensor arrays |
154
|
BioMEMS applications |
155
|
Hand-fabricated CNT/AgNPs electrodes for electro-immunosensing |
61
|
Strain monitoring and fracture recovery of human femur bone |
156
|
Thermal nanoimprint lithography for biosensing application |
157
|
Recently developed biosensing platforms offering both the biocompatibility of sensor materials and technologies as well as a variety of functions are discussed. Various sensor technologies' biocompatibility, functionality, and applications96–106,129–158 are outlined in Fig. 7.
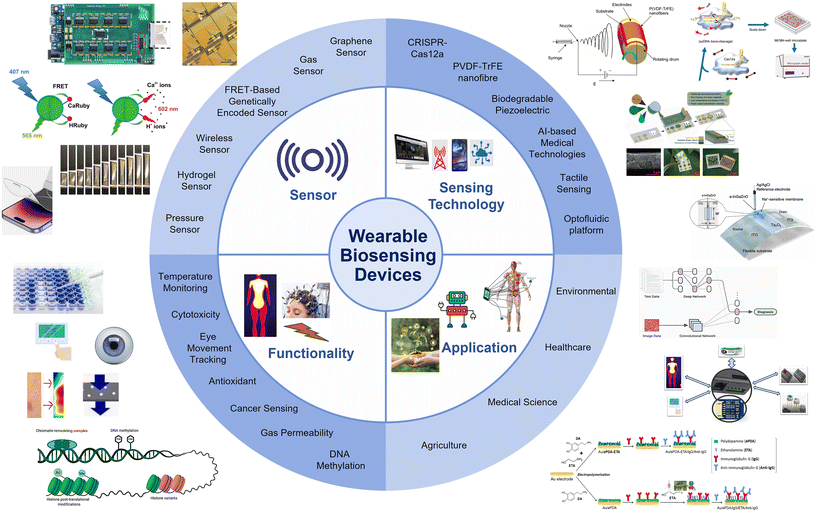 |
| Fig. 7 Biocompatibility of some reported sensor technologies, as well as their functionality and applications, are illustrated here, which can be found in ref. 96–106 and 129–158. Figures adapted from ref. 58–60, 71 and 158–162, CC BY 4.0 (http://creativecommons.org/licenses/by/4.0/). | |
Products that are based on analytes and are particularly well designed and novel can measure biological reactions in disease-specific mechanisms. By understanding the pathogenic mechanism, biomarkers, sensing technology, and the utilization of materials, it is possible to produce medical devices that can enable standard biocompatibility testing.
6.2. Future prospects in device industrialization
Industrialization of integrated biosensing-enabled digital healthcare devices appears promising due to various drivers. Sensor technology is enabling the development of more sensitive, accurate, and real-time systems that can detect a wide range of physiological and biochemical markers. This progress, along with the IoT and wearable technology, is facilitating seamless health data transmission and analysis, improving remote monitoring and patient care. The desire for personalized medicine highlights the need for healthcare solutions tailored to individual patients based on their unique health data, obtained by these modern biosensing technologies. There is a shift in focus toward preventive healthcare, which aims to maintain wellness and prevent diseases from requiring significant medical intervention, emphasizing the importance of early detection and continuous monitoring by these devices.64–68
AI and machine learning combined with biosensing data is changing the approaches to diagnosis and individualized treatment. These technologies are improving health data analysis, enabling more accurate diagnosis and individualized healthcare. Technology makes these devices more affordable and accessible, creating new opportunities for improving global health outcomes and expanding their use into emerging countries. Technology businesses, healthcare providers, and research institutes are collaborating to drive innovation and address population healthcare requirements.40,53
In order for the sector to be sustainable and scalable, the environmental effects and accessibility of healthcare equipment must be considered. More intelligent, integrated, and patient-centric healthcare solutions are projected to improve patient care and health outcomes as the sector matures. A new era of accessible, personalized, and preventive healthcare is emerging as digital healthcare devices support effective disease management and empower individuals with the tools and information needed for proactive health maintenance.42–44
6.2.1 Emerging device technologies.
Emerging technologies provide us with the opportunity for progress and to improve activities related to medical diagnosis and treatment. Table 5 outlines some health management advances in the biosensing field based on device development.163–174 Developments include devices and systems for drug delivery, an ultrahigh pressure sensor, implantable pressure sensor, non-invasive wearable sensor, seizure, stretchable piezoelectric, implanted pH sensor, stretchable hybrid electronics for a skin sensor, bioimaging and wearable light-touch contact, and systems for strokes, etc.163–174
6.2.2 Commercialization and management.
Electrochemical platforms, low-cost electromyography, non-invasive wearables for HbA1c and glucose etc., are used for biomarker sensing and commercialization.175–178 Health management schedules for oral wearable sensors, stretchable strain sensors for joint motion and respiration monitoring, finger-based pulse oximeters, glucose monitoring systems among adults with type 2 diabetes, bipolar disorder treatment systems etc., have become standard elements of intensive care and follow-up plans.179–183Table 6 outlines some health management advances in the biosensing field based on device development.175–183
Table 6 Health management platforms based on biosensing research for the commercialization of devices
Category |
Detail |
Ref. |
Device |
Soft implantable drug delivery device |
163
|
An ultrahigh sensitive paper-based pressure sensor |
164
|
Ultrathin composite coating for implantable pressure sensor |
165
|
A non-invasive wearable device for sweat pressure |
166
|
An edge-device for accurate seizure detection |
167
|
Stretchable piezoelectric sensing systems |
168
|
An implanted pH sensor read using radiography |
169
|
Stretchable hybrid electronics for skin |
170
|
Label-free serum albumin nanoparticles for bioimaging and drug delivery |
171
|
Excitation–emission matrix for UV-treated cell culture |
172
|
A wearable light-touch contact device |
173
|
Portable, open-source solutions for estimating stroke |
174
|
Commercial |
Electrochemical biosensing platform |
175
|
Low-cost electromyography |
176
|
Non-invasive wearables for HbA1c and glucose |
177
|
Silicon nanodisk huygens metasurfaces for biomarker sensing |
178
|
Health management |
Oral wearable sensors |
179
|
Stretchable strain sensor for joint motion and respiration monitoring |
180
|
Finger-based pulse oximeters |
181
|
Glucose monitoring systems among adults with type 2 diabetes |
182
|
Bipolar disorder treatment management |
183
|
Research progress has led to the conclusion that today's and future materials advances, optimizing the industrial structure, and building a modern environmental governance system integrating modern technologies (AI, IoT, cloud etc.) can help progress toward achieving global sustainability (Fig. 8).
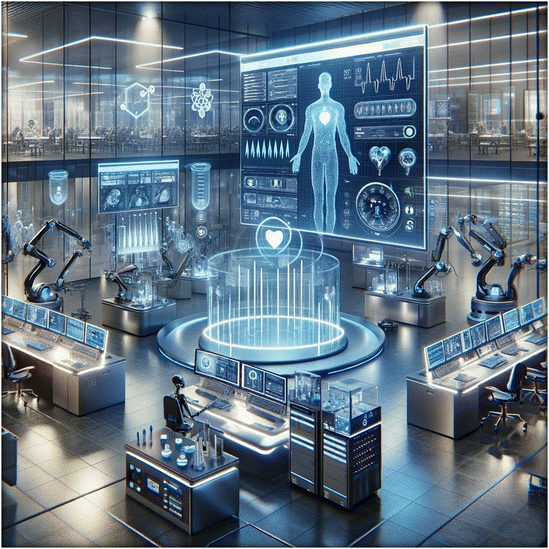 |
| Fig. 8 Design framework and methods used for applying sensing devices in an industrial setting, demonstrating the innovative concepts in sensor development that can lead to an effective healthcare management platform. The visuals present a futuristic laboratory equipped with advanced sensor technology, incorporating various innovative ideas in digital healthcare. | |
6.2.3 Effective biomaterials for indispensable health coverage.
Research developments have led to the conclusion that using cutting-edge materials with environmentally friendly functionality, optimizing industrial structure, establishing a contemporary environmental governance framework, and all of these things help to attain global sustainable healthcare. Biomaterials-induced sterile inflammation, mechanotransduction, and principles of biocompatibility control must be considered in biocompatibility pathways.184 Effective biomaterials should offer strength, biodegradation, biocompatibility, and an immunological benefit.185,186 Biofunctionalized composite scaffolds that can enhance osteonecrosis therapy and smart delivery platforms employing core–shell nanofibers for sequential drug release in wound healing are important applications.187,188
6.3. Next-generation technologies and future outlook
Future technologies that work together to improve productivity might benefit from more focus and an industrial point of view. Digital twins and quantum computing will ultimately change many processes in the healthcare industry's use of large-scale public health data. With a more intelligent approach, artificial intelligence is a fantastic field that will help improve public health and safety. The internet of things (IoT) can offer real-time display setups, data processes, and valuable information. Without human intervention, IoT devices can gather and transfer data over a wireless network. Furthermore, cloud computing offers internet-based services for data storage, servers, databases, networking, and software. A cost-effective solution for IT infrastructure is cloud-based systems. Through careful design and production, 3D printing technologies create prototypes to produce devices. The use of 3D printing for additive manufacturing requires the coordination of hardware, software, and materials. Through broadband cellular networks, the fifth generation of technology, or 5G, will expand the mobile ecosystem, opening new opportunities for infinitely usable data transfer and device functionality for real-time usage. By using the right applications, robotic process automation can mimic many human tasks and reduce risk due to the sterility, accuracy, and multiplicity of work functions. Using appropriate algorithms and platforms, data analytics uses machine learning and AI to help with data processing, preparation, analysis, prediction, and understanding. In a society free of disease, where the healthcare sector can produce and offer technical support for control management, well-characterized sensor devices are key. Innovative concepts were used to develop a variety of sensor applications to build platforms for effective healthcare management.13,67,68
This paper asserts that carbon neutrality has made some progress.189,190 In addition, it is necessary to establish a comprehensive standard for risk and climate neutrality assessment in healthcare management.18,62,190 There are approximately 2 million unique types of medical devices on the market today, which are categorized into over 7000 generic device groups.191 Access to high-quality, cost-effective, and appropriate health products is crucial for advancing universal health coverage, addressing health emergencies, and promoting healthier populations, even during pandemics.191,192 In recent decades, the sensor market in the healthcare sector has expanded significantly. Nanosensors developed by biomaterials can work efficiently in diagnostic devices for assessing anti-bacterial and anti-cancer activities based on electrochemistry communications.193–196 Advances in organ-on-a-chip materials and technologies through research in biosensors and bioelectronics with designed interfaces brings further possibilities for device development and their industrialization.197–199 The study provides a concise analysis of research tendencies in synthesis techniques as well as the difficulties associated with their implementation in industrial settings for layered materials, i.e., 2D materials, such as films, which can be used in the context of electronics, spintronics, optoelectronics, twistronics, or solar cells, while powders exhibit large surface areas and are employed in the construction of batteries, sensors, and catalysts. The three state-of-the-art mass production techniques refer to films. Films can be used in the context of electronics, spintronics, optoelectronics, twistronics, or solar cells.200 The pandemic and related global human health threats can be controlled by well-defined public health management; therefore, it is important to understand technological adaptation and carry out development on a regular basis.201,202 Integrated sensors now feature more cutting-edge technologies without sacrificing functionality. Herein, we have highlighted the key issues raised by new and pervasive diseases and provided recommendations for how the advanced materials community can address them. Therefore, future research should be conducted to improve health care management.
6.4. Healthcare transformation through wearables and AI
The personalized health ecosystem is transforming our lives through the use of wearable sensors that monitor our vital signs and habits. The advent of big data, AI, and micro-manufacturing has given rise to a broad spectrum of products, software, and services. These innovations offer healthcare solutions that address diverse issues, such as alarm fatigue, clinical variation, and the management of patient care transitions. To enhance personalized health, advancements in diverse sensors, 5G technology, and AI have led to the development of upgraded wearable sensors. These sensors can noninvasively monitor biochemical indicators in body fluids for disease prediction, diagnosis, and management.203
Non-invasive electrochemical sensors can detect biomarkers in tears, saliva, perspiration, and skin interstitial fluid (ISF), offering a comprehensive view of an individual's health status. Integrating AI into healthcare presents significant opportunities to improve disease diagnosis, treatment selection, and clinical laboratory testing.204 AI tools, by analyzing large datasets and identifying patterns, can outperform human capabilities in various healthcare domains. The benefits of AI include enhanced accuracy, cost reduction, time savings, and the minimization of human errors. AI has the potential to revolutionize personalized medicine, optimize medication dosages, boost population health management, establish care guidelines, provide virtual health assistants, support mental health care, enhance patient education, and foster trust between patients and physicians.
The benefits of AI have already been recognized in various healthcare sectors, including cardiac arrhythmia monitoring, diabetes management, and assisted surgeries.205 AI and machine learning (ML) techniques also enhance the analysis of analytical data from low-resolution or noisy datasets, improving signal strength, sensitivity, specificity, and measurement time.
Understanding technology is crucial for optimal performance. Wi-Fi and Bluetooth serve as primary connectivity methods for interfacing IoMT devices with a central hub. Bluetooth connectivity, suitable for short-range communications up to 10 meters with a maximum speed of 3 Mbps, can link sensors to portable devices, like tablets, smartphones, and PCs. This method is particularly useful in operating rooms, intensive care units (ICUs), and other settings with numerous devices. Wi-Fi modules must incorporate optimized scanning algorithms to ensure persistent network connections for mobile devices in environments with high radio frequency (RF) noise.47
7. Potential challenges
The advent of integrated biosensing-enabled digital healthcare devices represents a substantial advancement in medical technology, with the potential to provide more individualized, efficient, and easily accessible healthcare solutions.9 Nevertheless, harnessing the complete capabilities of these advancements is not devoid of obstacles, with the key challenges shown in Fig. 9.
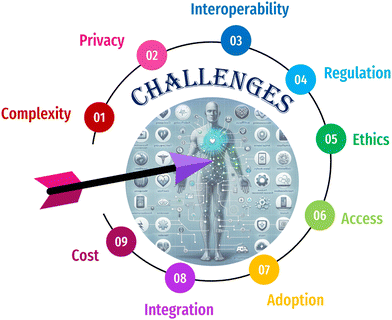 |
| Fig. 9 Challenges in the development of integrated biosensing digital healthcare devices. Nine primary challenges encountered in the evolution of integrated biosensing-enabled digital healthcare devices, with the figure depicting the complexity and interrelated nature of issues, ranging from technical and privacy concerns to regulatory compliance and cost considerations. | |
The intricate nature of creating devices capable of precisely and consistently monitoring real-time health indicators presents a substantial challenge. Another crucial concern is the need to guarantee the privacy and security of sensitive health data obtained and communicated by these devices. This necessitates the implementation of strong encryption and secure data handling methods to protect against unwanted access and cyberattacks.12
Ensuring interoperability and standardization among different healthcare systems and technologies is crucial for the efficiency of these devices, but remain difficult tasks. Intricate legal frameworks add more complexity to the process of bringing these technologies to the market, as strict approval procedures and compliance obligations can impede the pace of innovation and adoption. In order to ensure responsible use, it is imperative to address ethical considerations, specifically those related to permission, autonomy, and the possibility of surveillance.27
Moreover, there is a potential danger that this sophisticated healthcare technology could worsen current disparities in health, highlighting the importance of ensuring fair and equal access to their advantages. In order to achieve widespread adoption, it is also essential to overcome skepticism and opposition from healthcare providers and patients. Additionally, incorporating these new technologies into conventional healthcare practices requires substantial training and assistance. Ultimately, the development and deployment of these devices require significant investment.63 Therefore, it is important to carefully weigh the cost against the potential improvements in healthcare. This makes the process of integrating biosensing-enabled healthcare devices complex, but ultimately worthwhile.
8. Conclusions and future perspective
Recent years have witnessed significant advancements in disease management techniques, largely influenced by experiences gained during pandemics. These improvements in diagnostic and treatment methods have notably enhanced the success rates of both diagnosing and treating diseases compared to earlier practices. In envisioning the future of integrated biosensing and digital healthcare devices, this article anticipates significant advancements and transformations will further be made building on the progress already made. The field is poised to move beyond the current state-of-the-art, leveraging the lessons learned from pandemic experiences to enhance disease management. Future perspectives will likely focus on the development of ideal point-of-care devices, which, despite two decades of research, are yet to be fully materialized in the market. These devices, crucial for rapid and accurate diagnosis during pandemics, for instance, are expected to shift the paradigm toward patient-centered, non-laboratory testing. The article also highlights the increasing relevance of wearable sensors and systems in clinical applications. The past decade has seen these technologies evolve from research tools to integral components of public healthcare, improving monitoring, intervention, and outcomes. The future holds promise for more remote monitoring, particularly for older adults and chronic disease patients, though challenges in cost and reimbursement remain to be addressed. Another key area of advancement is in the realm of advanced materials and the internet of things integration. Biosensors are becoming increasingly vital in these technological landscapes, contributing to a more sustainable world.
The present review sheds light on how digital biosensors have revolutionized disease detection and biochemical analysis through their real-time, remote, and precise capabilities. The trends toward miniaturization, automation, and multiplexing in biosensing methods are particularly noteworthy. These trends have led to the creation of portable, flexible, and wearable devices that enable continuous health monitoring and point-of-care diagnostics. Digital automation and integrated multiplexing have enhanced the efficiency and accuracy of these devices, while the incorporation of smartphones and other digital devices as readout platforms has made biosensing more user-friendly. Artificial intelligence and machine learning are set to play a more pivotal role in the interpretation and analysis of complex biosensor data. The integration of these technologies into IoT platforms is expected to unlock tremendous potential and market value for personalized healthcare. The future of digital healthcare and biosensing is rich with possibilities. It encompasses a range of innovative biosensing methods and devices that are set to revolutionize the market. This article systematically discusses these advancements, emphasizing the need for intelligent point-of-care devices that synergize technological innovations with real-time healthcare management. This comprehensive discussion bridges technological progress with translational wisdom, outlining a vision for the future healthcare industry that is both ambitious and attainable.
Data availability statement
The data used in this review article was extracted from published literature and research. The article does not describe any new experimental or primary data. All data sources are cited in the reference section of this article.
Disclosure
The authors have utilized ChatGPT to enhance readability, graphics and language. Following this application, the authors meticulously reviewed and edited the content as necessary, assuming full responsibility for the publication of contents and adopted figures.
Author contributions
Anshuman Mishra, Pravin Kumar Singh, Nidhi Chauhan, Utkarsh Jain: writing, review, data collection, visualization; Souradeep Roy, Ayushi Tiwari, Shaivya Gupta, Santanu Patra, Trupti R. Das, Ahmad Soltani Nejad: data analysis, writing, reviewing & editing; Yogesh Kumar Shukla, Prashant Mishra, Ashutosh Tiwari: conceptualization of the work, assisting in writing, reviewing & editing.
Conflicts of interest
The authors declare no conflict of interest.
Acknowledgements
The authors acknowledge the International Association of Advanced Materials (IAAM), Sweden for providing financial support for this research work.
References
- D. Najjar, J. Rainbow, S. Sharma Timilsina, P. Jolly, H. De Puig, M. Yafia, N. Durr, H. Sallum, G. Alter, J. Z. Li and X. G. Yu, A lab-on-a-chip for the concurrent electrochemical detection of SARS-CoV-2 RNA and anti-SARS-CoV-2 antibodies in saliva and plasma, Nat. Biomed. Eng., 2022, 6(8), 968–978 CrossRef CAS PubMed.
-
B. B. Prasad and M. P. Tiwari, Molecularly imprinted nanomaterial-based highly sensitive and selective medical devices, Biomedical Materials and Diagnostic Devices, John Wiley & Sons, Inc., Hoboken, NJ, USA, 2012, pp. 339–391 Search PubMed.
- H. C. Ates, P. Q. Nguyen, L. Gonzalez-Macia, E. Morales-Narváez, F. Güder, J. J. Collins and C. Dincer, End-to-end design of wearable sensors, Nat. Rev. Mater., 2022, 7(11), 887–907 CrossRef PubMed.
-
Graphene bioelectronics, ed. A. Tiwari, Elsevier, 2017 Search PubMed.
-
Biosensors nanotechnology, ed. A. Tiwari and A. P. Turner, John Wiley & Sons, 2014 Search PubMed.
- J. Kim, A. S. Campbell, B. E. de Ávila and J. Wang, Wearable biosensors for healthcare monitoring, Nat. Biotechnol., 2019, 37(4), 389–406 CrossRef CAS PubMed.
- S. Bhatt, P. W. Gething, O. J. Brady, J. P. Messina, A. W. Farlow, C. L. Moyes, J. M. Drake, J. S. Brownstein, A. G. Hoen, O. Sankoh and M. F. Myers, The global distribution and burden of dengue, Nature, 2013, 496(7446), 504–507 CrossRef CAS PubMed.
- S. Cheng, Z. Gu, L. Zhou, M. Hao, H. An, K. Song, X. Wu, K. Zhang, Z. Zhao, Y. Dong and Y. Wen, Recent progress in intelligent wearable sensors for health monitoring and wound healing based on biofluids, Front. Bioeng. Biotechnol., 2021, 9, 765987 CrossRef.
- B. Nthubu, An overview of sensors, design and healthcare challenges in smart homes: future design questions, Healthcare, 2021, 9(10), 1329 CrossRef PubMed.
- M. Ashaduzzaman, A. A. Antony, N. A. Murugan, S. R. Deshpande, A. P. Turner and A. Tiwari, Studies on an on/off-switchable immunosensor for troponin T, Biosens. Bioelectron., 2015, 73, 100–107 CrossRef CAS PubMed.
- R. Snodgrass, A. Gardner, A. Semeere, V. L. Kopparthy, J. Duru, T. Maurer, J. Martin, E. Cesarman and D. Erickson, A portable device for nucleic acid quantification powered by sunlight, a flame or electricity, Nat. Biomed. Eng., 2018, 2(9), 657–665 CrossRef CAS PubMed.
- B. Heidt, W. F. Siqueira, K. Eersels, H. Diliën, B. van Grinsven, R. T. Fujiwara and T. J. Cleij, Point of care diagnostics in resource-limited settings: A review of the present and future of PoC in its most needed environment, Biosensors, 2020, 10(10), 133 CrossRef.
- S. Preetam, B. K. Nahak, S. Patra, D. C. Toncu, S. Park, M. Syväjärvi, G. Orive and A. Tiwari, Emergence of microfluidics for next generation biomedical devices, Biosens. Bioelectron.: X, 2022, 10, 100106 CAS.
- T. J. Morin, W. L. McKenna, T. D. Shropshire, D. A. Wride, J. D. Deschamps, X. Liu, R. Stamm, H. Wang and W. B. Dunbar, A handheld platform for target protein detection and quantification using disposable nanopore strips, Sci. Rep., 2018, 8(1), 14834 CrossRef PubMed.
- R. Dwivedi, D. Mehrotra and S. Chandra, Potential of Internet of Medical Things (IoMT) applications in building a smart healthcare system: A systematic review, J. Oral Biol. Craniofac. Res., 2022, 12(2), 302–318 CrossRef PubMed.
- J. T. Kelly, K. L. Campbell, E. Gong and P. Scuffham, The Internet of Things: Impact and implications for health care delivery, J. Med. Internet Res., 2020, 22(11), e20135 CrossRef PubMed.
- J. Lin, C. Jordi, M. Son, H. Van Phan, N. Drayman, M. F. Abasiyanik, L. Vistain, H. L. Tu and S. Tay, Ultra-sensitive digital quantification of proteins and mRNA in single cells, Nat. Commun., 2019, 10(1), 3544 CrossRef.
- A. Mishra, S. Patra, V. Srivastava, L. Uzun, Y. K. Mishra, M. Syväjärvi and A. Tiwari, Progress in paper-based analytical devices for climate neutral biosensing, Biosens. Bioelectron.: X, 2022, 11, 100166 CAS.
- E. Wilkinson, Reaching net zero carbon emissions in health systems, Lancet, 2021, 398(10315), 1953–1954 CrossRef CAS PubMed.
- S. Deng, Q. Jiang, Y. Zhang, X. Lu, X. Shi and Y. Zhang, Design and Implementation of Medical Ultrasound Remote Control Software Based on Sensor Design, Front. Bioeng. Biotechnol., 2021, 8, 620237 CrossRef PubMed.
- S. Prakash, B. K. Ashley, P. S. Doyle and U. Hassan, Design of a multiplexed analyte biosensor using digital barcoded particles and impedance spectroscopy, Sci. Rep., 2020, 10(1), 6109 CrossRef CAS PubMed.
- G. L. Cerone, A. Giangrande, M. Ghislieri, M. Gazzoni, H. Piitulainen and A. Botter, Design and validation of a wireless body sensor network for integrated EEG and HD-sEMG acquisitions, IEEE Trans. Neural Syst. Rehabil. Eng., 2022, 30, 61–71 CAS.
- S. M. Uddin, A. Sayad, J. Chan, E. Skafidas and P. Kwan, Design and Optimisation of Elliptical-Shaped Planar Hall Sensor for Biomedical Applications, Biosensors, 2022, 12(2), 108 CrossRef CAS PubMed.
- D. T. Bacheschi, E. Z. Strittmatter, S. Sawtelle and M. Nami, Designing Sensitivity: A Comparative Analysis of Microelectrode Topologies for Electrochemical Oxygen Sensing in Biomedical Applications, Micromachines, 2022, 13(1), 141 CrossRef PubMed.
- M. Herneisey, P. F. Salcedo, T. Domenech, C. Bagia, S. S. George, R. Tunney, S. Velankar, T. K. Hitchens and J. M. Janjic, Design of Thermoresponsive Polyamine Cross-Linked Perfluoropolyether Hydrogels for Imaging and Delivery Applications, ACS Med. Chem. Lett., 2020, 11(10), 2032–2040 CrossRef CAS PubMed.
- F. Chen, S. Gu, Q. Zhang, T. Liu, Z. Liu and T. Kuang, A comparison study of hyaluronic acid hydrogel exquisite micropatterns with photolithography and light-cured inkjet printing methods, e-Polym., 2022, 22(1), 332–341 CrossRef CAS.
- K. Lavrenyuk, D. Conway and K. N. Dahl, Imaging methods in mechanosensing: a historical perspective and visions for the future, Mol. Biol. Cell, 2021, 32(9), 842–854 CrossRef CAS PubMed.
- C. Yang, B. D. Ojha, N. D. Aranoff, P. Green and N. Tavassolian, Classification of aortic stenosis using conventional machine learning and deep learning methods based on multi-dimensional cardio-mechanical signals, Sci. Rep., 2020, 10(1), 17521 CrossRef CAS PubMed.
- X. Li, Z. Zhou, J. Wu and Y. Xiong, Human posture detection method based on wearable devices, J. Healthc. Eng., 2021, 2021, 8879061 Search PubMed.
- S. Wheaton, V. Lopez-Dominguez, H. Almasi, J. Cai, Z. Zeng, P. Khalili Amiri and H. Mohseni, A 3 pJ/bit free space optical interlink platform for self-powered tetherless sensing and opto-spintronic RF-to-optical transduction, Sci. Rep., 2021, 11(1), 8504 CrossRef CAS PubMed.
- S. Samimi Gharaie, A. Seyfoori, B. Khun Jush, X. Zhou, E. Pagan, B. Godau and M. Akbari, Silicate-based electro-conductive inks for printing soft electronics and tissue engineering, Gels, 2021, 7(4), 240 CrossRef CAS PubMed.
- C. McLean, B. Tiller, R. Mansour, K. Brown, J. Windmill and L. Dennany, Characterising the response of novel 3D printed CNT electrodes to the virulence factor pyocyanin, J. Electroanal. Chem., 2022, 909, 116149 CrossRef CAS.
- C. D. Raposo, A. B. Canelas and M. T. Barros, Human lectins, their carbohydrate affinities and where to find them, Biomolecules, 2021, 11(2), 188 CrossRef CAS PubMed.
- V. Montes-García and P. Samorì, Janus 2D materials via asymmetric molecular functionalization, Chem. Sci., 2022, 13(2), 315–328 RSC.
- R. A. Tromans, S. K. Samanta, A. M. Chapman and A. P. Davis, Selective glucose sensing in complex media using a biomimetic receptor, Chem. Sci., 2020, 11(12), 3223–3227 RSC.
- W. Jiang, J. Guo, W. Wen, Y. G. Jia and S. Liu, Nano-carriers based on pH-sensitive star-shaped copolymers for drug-controlled release, Materials, 2019, 12(10), 1610 CrossRef CAS.
- D. Kratzer, A. Ludwig-Husemann, K. Junges, U. Geckle and C. Lee-Thedieck, Nanostructured bifunctional hydrogels as potential instructing platform for hematopoietic stem cell differentiation, Front. Mater., 2019, 5, 81 CrossRef.
- J. Ye, F. Zhang, Z. Shen, S. Cao, T. Jin, X. Guo, Z. Li, L. Lin and Y. Zhang, Tunable seesaw-like 3D capacitive sensor for force and acceleration sensing, npj Flexible Electron., 2021, 5(1), 28 CrossRef.
- B. Mahanty, S. K. Ghosh, K. Maity, K. Roy, S. Sarkar and D. Mandal, All-fiber pyro-and piezo-electric nanogenerator for IoT based self-powered health-care monitoring, Mater. Adv., 2021, 2(13), 4370–4379 RSC.
- J. A. Moore and J. C. Chow, Recent progress and applications of gold nanotechnology in medical biophysics using artificial intelligence and mathematical modeling, Nano Express, 2021, 2(2), 022001 CrossRef.
- S. Singh, P. K. Singh, A. Umar, P. Lohia, H. Albargi, L. Castañeda and D. K. Dwivedi, 2D nanomaterial-based surface plasmon resonance sensors for biosensing applications, Micromachines, 2020, 11(8), 779 CrossRef.
- R. Yavari, A. Riensche, E. Tekerek, L. Jacquemetton, H. Halliday, M. Vandever, A. Tenequer, V. Perumal, A. Kontsos, Z. Smoqi and K. Cole, Digitally twinned additive manufacturing: Detecting flaws in laser powder bed fusion by combining thermal simulations with in-situ meltpool sensor data, Mater. Des., 2021, 211, 110167 CrossRef CAS.
- G. Gryglewski, R. Lanzenberger, G. S. Kranz and P. Cumming, Meta-analysis of molecular imaging of serotonin transporters in major depression, J. Cereb. Blood Flow Metab., 2014, 34(7), 1096–1103 CrossRef CAS PubMed.
- X. Peng, X. Kuang, D. J. Roach, Y. Wang, C. M. Hamel, C. Lu and H. J. Qi, Integrating digital light processing with direct ink writing for hybrid 3D printing of functional structures and devices, Addit. Manuf., 2021, 40, 101911 CAS.
- C. Fekiri, H. C. Kim and I. H. Lee, 3D-printable carbon nanotubes-based composite for flexible piezoresistive sensors, Materials, 2020, 13(23), 5482 CrossRef CAS PubMed.
- R. Shaw, M. Stroo, C. Fiander and K. McMillan, Selecting mobile health technologies for electronic health record integration: case study, J. Med. Internet. Res., 2020, 22(10), e23314 CrossRef PubMed.
- G. Tang, Y. Yan, C. Shen, X. Jia, M. Zinn, Z. Trivedi, A. Yingling, K. Westover and S. Jiang, Development of a real-time indoor location system using bluetooth low
energy technology and deep learning to facilitate clinical applications, Med. Phys., 2020, 47(8), 3277–3285 CrossRef PubMed.
- A. A. Bui, A. Hosseini, R. Rocchio, N. Jacobs, M. K. Ross, S. Okelo, F. Lurmann, S. Eckel, E. Dzubur, G. Dunton and F. Gilliland, Biomedical real-time health evaluation (BREATHE): toward an mHealth informatics platform, JAMIA Open, 2020, 3(2), 190–200 CrossRef PubMed.
- N. Richer, R. J. Downey, W. D. Hairston, D. P. Ferris and A. D. Nordin, Motion and muscle artifact removal validation using an electrical head phantom, robotic motion platform, and dual layer mobile EEG, IEEE Trans. Neural Syst. Rehabil. Eng., 2020, 28(8), 1825–1835 Search PubMed.
- K. Umetani, T. Okamoto, K. Saito, Y. Kawata and N. Niki, 36M-pixel synchrotron radiation micro-CT for whole secondary pulmonary lobule visualization from a large human lung specimen, Eur. J. Radiol. Open, 2020, 7, 100262 CrossRef PubMed.
- A. Shahshahani, Z. Zilic and S. Bhadra, An ultrasound-based biomedical system for continuous cardiopulmonary monitoring: A single sensor for multiple information, IEEE Trans. Biomed. Eng., 2019, 67(1), 268–276 Search PubMed.
- H. Nah, D. Lee, M. Heo, J. S. Lee, S. J. Lee, D. N. Heo, J. Seong, H. N. Lim, Y. H. Lee, H. J. Moon and Y. S. Hwang, Vitamin D-conjugated gold nanoparticles as functional carriers to enhancing osteogenic differentiation, Sci. Technol. Adv. Mater., 2019, 20(1), 826–836 CrossRef CAS.
- A. K. Kaushik, J. S. Dhau, H. Gohel, Y. K. Mishra, B. Kateb, N. Y. Kim and D. Y. Goswami, Electrochemical SARS-CoV-2 sensing at point-of-care and artificial intelligence for intelligent COVID-19 management, ACS Appl. Bio Mater., 2020, 3(11), 7306–7325 CrossRef CAS PubMed.
- R. Zhang, T. Belwal, L. Li, X. Lin, Y. Xu and Z. Luo, Nanomaterial-based biosensors for sensing key foodborne pathogens: Advances from recent decades, Compr. Rev. Food Sci. Food Saf., 2020, 19(4), 1465–1487 CrossRef PubMed.
- U. Jain, S. Gupta, S. Soni, M. P. Khurana and N. Chauhan, Triple-nanostructuring-based noninvasive electro-immune sensing of CagA toxin for Helicobacter pylori detection, Helicobacter, 2020, 25(4), e12706 CrossRef CAS.
- R. Mendes, N. Sousa, A. Almeida, J. Vilaça-Alves, V. M. Reis and E. B. Neves, Thermography: a technique for assessing the risk of developing diabetic foot disorders, Postgrad. Med. J., 2015, 91(1079), 538 CrossRef CAS PubMed.
- S. Roy, S. Nagabooshanam, S. Wadhwa, N. Chauhan, A. Mathur, S. A. Khan and J. Davis, Ultra-sensitive detection of l-tyrosine using molecularly imprinted electrochemical sensor towards diabetic foot ulcer detection, Electrochem. Commun., 2020, 117, 106782 CrossRef CAS.
- A. I. Zamaleeva, G. Despras, C. Luccardini, M. Collot, M. De Waard, M. Oheim, J. M. Mallet and A. Feltz, FRET-based nanobiosensors for imaging intracellular Ca2+ and H+ microdomains, Sensors, 2015, 15(9), 24662–24680 CrossRef CAS PubMed.
- M. Liang, Z. Li, W. Wang, J. Liu, L. Liu, G. Zhu, L. Karthik, M. Wang, K. F. Wang, Z. Wang and J. Yu, A CRISPR-Cas12a-derived biosensing platform for the highly sensitive detection of diverse small molecules, Nat. Commun., 2019, 10(1), 3672 CrossRef PubMed.
- M. Xue, C. Mackin, W. H. Weng, J. Zhu, Y. Luo, S. X. Luo, A. Y. Lu, M. Hempel, E. McVay, J. Kong and T. Palacios, Integrated biosensor platform based on graphene transistor arrays for real-time high-accuracy ion sensing, Nat. Commun., 2022, 13(1), 5064 CrossRef CAS PubMed.
- S. Chen, A. Z. Qamar, N. Asefifeyzabadi, M. Funneman, M. Taki, L. Elliot, M. E. Kinsel, G. R. Kinsel and M. H. Shamsi, Hand-fabricated CNT/AgNPs electrodes using wax-on-plastic platforms for electro-immunosensing application, Sci. Rep., 2019, 9(1), 6131 CrossRef PubMed.
- M. M. Monroe, L. G. Villanueva and D. Briand, Low-temperature processing of screen-printed piezoelectric KNbO3 with integration onto biodegradable paper substrates, Microsyst. Nanoeng., 2023, 9(1), 19 CrossRef CAS PubMed.
- N. Wongkaew, M. Simsek, C. Griesche and A. J. Baeumner, Functional nanomaterials and nanostructures enhancing electrochemical biosensors and lab-on-a-chip performances: recent progress, applications, and future perspective, Chem. Rev., 2018, 119(1), 120–194 CrossRef PubMed.
- S. Yao, P. Swetha and Y. Zhu, Nanomaterial-enabled wearable sensors for healthcare, Adv. Healthcare Mater., 2018, 7(1), 1700889 CrossRef CAS PubMed.
- Y. Fang, J. G. Hester, W. Su, J. H. Chow, S. K. Sitaraman and M. M. Tentzeris, A bio-enabled maximally mild layer-by-layer Kapton surface modification approach for the fabrication of all-inkjet-printed flexible electronic devices, Sci. Rep., 2016, 6(1), 39909 CrossRef CAS PubMed.
- T. Guinovart, G. Valdés-Ramírez, J. R. Windmiller, F. J. Andrade and J. Wang, Bandage-based wearable potentiometric sensor for monitoring wound pH, Electroanalysis, 2014, 26(6), 1345–1353 CrossRef CAS.
-
M. Pateraki, K. Fysarakis, V. Sakkalis, G. Spanoudakis, I. Varlamis, M. Maniadakis, M. Lourakis, S. Ioannidis, N. Cummins, B. Schuller and E. Loutsetis, Biosensors and Internet of Things in smart healthcare applications: Challenges and opportunities, Wearable and Implantable Medical Devices, 2020, pp. 25–53 Search PubMed.
- M. A. da Cruz, J. J. Rodrigues, J. Al-Muhtadi, V. V. Korotaev and V. H. de Albuquerque, A reference model for internet of things middleware, IEEE Internet Things J., 2018, 5(2), 871–883 Search PubMed.
- H. R. Lim, Y. Lee, K. A. Jones, Y. T. Kwon, S. Kwon, M. Mahmood, S. M. Lee and W. H. Yeo, All-in-one, wireless, fully flexible sodium sensor system with integrated Au/CNT/Au nanocomposites, Sens. Actuators, B, 2021, 331, 129416 CrossRef CAS.
- C. Wang, Z. Zhao, R. Wu, X. Shi, G. F. Payne and X. Wang, Fabrication of Robust Paper-Based Electronics by Adapting Conventional Paper Making and Coupling with Wet Laser Writing, ACS Sustainable Chem. Eng., 2023, 11(26), 9782–9791 CrossRef CAS.
- A. F. Coskun, A. E. Cetin, B. C. Galarreta, D. A. Alvarez, H. Altug and A. Ozcan, Lensfree optofluidic plasmonic sensor for real-time and label-free monitoring of molecular binding events over a wide field-of-view, Sci. Rep., 2014, 4(1), 6789 CrossRef CAS PubMed.
- S. Roy, S. Nagabooshanam, K. Krishna, S. Wadhwa, N. Chauhan, U. Jain, R. Kumar, A. Mathur and J. Davis, Electroanalytical sensor for diabetic foot ulcer monitoring with integrated electronics for connected health application, Electroanalysis, 2020, 32(9), 2082–2089 CrossRef CAS.
- Q. Ge, A. H. Sakhaei, H. Lee, C. K. Dunn, N. X. Fang and M. L. Dunn, Multimaterial 4D printing with tailorable shape memory polymers, Sci. Rep., 2016, 6(1), 31110 CrossRef.
- P. Kassal, J. Kim, R. Kumar, W. R. de Araujo, I. M. Steinberg, M. D. Steinberg and J. Wang, Smart bandage with wireless connectivity for uric acid biosensing as an indicator of wound status, Electrochem. Commun., 2015, 56, 6–10 CrossRef CAS.
- A. Yakoh, U. Pimpitak, S. Rengpipat, N. Hirankarn, O. Chailapakul and S. Chaiyo, Paper-based electrochemical biosensor for diagnosing COVID-19: Detection of SARS-CoV-2 antibodies and antigen, Biosens. Bioelectron., 2021, 176, 112912 CrossRef CAS.
- S. Roy, S. Nagabooshanam, S. Wadhwa, N. Chauhan, A. Mathur, S. A. Khan and J. Davis, Ultra-sensitive detection of l-tyrosine using molecularly imprinted electrochemical sensor towards diabetic foot ulcer detection, Electrochem. Commun., 2020, 117, 106782 CrossRef CAS.
- A. Petritz, E. Karner-Petritz, T. Uemura, P. Schäffner, T. Araki, B. Stadlober and T. Sekitani, Imperceptible energy harvesting device and biomedical sensor based on ultraflexible ferroelectric transducers and organic diodes, Nat. Commun., 2021, 12(1), 2399 CrossRef CAS PubMed.
- A. Rayegani, M. Saberian, Z. Delshad, J. Liang, M. Sadiq, A. M. Nazar, S. A. Mohsan and M. A. Khan, Recent advances in self-powered wearable sensors based on piezoelectric and triboelectric nanogenerators, Biosensors, 2022, 13(1), 37 CrossRef PubMed.
- D. Zhang, Y. Lu, Q. Zhang, L. Liu, S. Li, Y. Yao, J. Jiang, G. L. Liu and Q. Liu, Protein detecting with smartphone-controlled electrochemical impedance spectroscopy for point-of-care applications, Sens. Actuators, B, 2016, 222, 994–1002 CrossRef CAS.
- S. Roy, K. Bisaria, S. Nagabooshanam, A. Selvam, S. Chakrabarti, S. Wadhwa, R. Singh, A. Mathur and J. Davis, An electroanalytical paper-based wound dressing using ZIF-67/C 3 N 4 nanocomposite towards the monitoring of staphylococcus aureus in diabetic foot ulcer, IEEE Sens. J., 2020, 21(2), 1215–1221 Search PubMed.
- J. Kim, S. Imani, W. R. de Araujo, J. Warchall, G. Valdés-Ramírez, T. R. Paixão, P. P. Mercier and J. Wang, Wearable salivary uric acid mouthguard biosensor with integrated wireless electronics, Biosens. Bioelectron., 2015, 74, 1061–1068 CrossRef CAS PubMed.
- L. Manjakkal, W. Dang, N. Yogeswaran and R. Dahiya, Textile-based potentiometric electrochemical pH sensor for wearable applications, Biosensors, 2019, 9(1), 14 CrossRef CAS PubMed.
- K. H. Maier-Hein, P. F. Neher, J. C. Houde, M. A. Côté, E. Garyfallidis, J. Zhong, M. Chamberland, F. C. Yeh, Y. C. Lin, Q. Ji and W. E. Reddick, The challenge of mapping the human connectome based on diffusion tractography, Nat. Commun., 2017, 8(1), 1349 CrossRef PubMed.
- W. Gao, S. Emaminejad, H. Y. Nyein, S. Challa, K. Chen, A. Peck, H. M. Fahad, H. Ota, H. Shiraki, D. Kiriya and D. H. Lien, Fully integrated wearable sensor arrays for multiplexed in situ perspiration analysis, Nature, 2016, 529(7587), 509–514 CrossRef CAS PubMed.
- A. McLister, C. Casimero, A. McConville, C. M. Taylor, C. L. Lawrence, R. B. Smith, A. Mathur and J. Davis, Design of a smart sensor mesh for the measurement of pH in ostomy applications, J. Mater. Sci., 2019, 54(14), 10410–10419 CrossRef CAS.
- R. Barber, S. Cameron, A. Devine, A. McCombe, L. K. Pourshahidi, J. Cundell, S. Roy, A. Mathur, C. Casimero, P. Papakonstantinou and J. Davis, Laser induced graphene sensors for assessing pH: Application to wound management, Electrochem. Commun., 2021, 123, 106914 CrossRef CAS.
- A. Pal, D. Goswami, H. E. Cuellar, B. Castro, S. Kuang and R. V. Martinez, Early detection and monitoring of chronic wounds using low-cost, omniphobic paper-based smart bandages, Biosens. Bioelectron., 2018, 117, 696–705 CrossRef CAS PubMed.
- J. R. Sempionatto, T. Nakagawa, A. Pavinatto, S. T. Mensah, S. Imani, P. Mercier and J. Wang, Eyeglasses based wireless electrolyte and metabolite sensor platform, Lab Chip, 2017, 17(10), 1834–1842 RSC.
- Z. L. Wang and J. Song, Piezoelectric nanogenerators based on zinc oxide nanowire arrays, Science, 2006, 312(5771), 242–246 CrossRef CAS PubMed.
- P. K. Singh, G. A. Kaur, M. Shandilya, P. Rana, R. Rai, Y. K. Mishra, M. Syväjärvi and A. Tiwari, Trends in piezoelectric nanomaterials towards green energy scavenging nanodevices, Mater. Today Sustain., 2023, 100583 CrossRef.
- Z. L. Wang, On Maxwell's displacement current for energy and sensors: the origin of nanogenerators, Mater. Today, 2017, 20(2), 74–82 CrossRef.
- H. Zhang, X. S. Zhang, X. Cheng, Y. Liu, M. Han, X. Xue, S. Wang, F. Yang, A. S. Smitha, H. Zhang and Z. Xu, A flexible and implantable piezoelectric generator harvesting energy from the pulsation of ascending aorta: in vitro and in vivo studies, Nano Energy, 2015, 12, 296–304 CrossRef CAS.
- X. Xue, Z. Qu, Y. Fu, B. Yu, L. Xing and Y. Zhang, Self-powered electronic-skin for detecting glucose level in body fluid basing on piezo-enzymatic-reaction coupling process, Nano Energy, 2016, 26, 148–156 CrossRef CAS.
- S. Zhang, M. Bick, X. Xiao, G. Chen, A. Nashalian and J. Chen, Leveraging triboelectric nanogenerators for bioengineering, Matter, 2021, 4(3), 845–887 CrossRef CAS.
- K. Kaefer, K. Krüger, F. Schlapp, H. Uzun, S. Celiksoy, B. Flietel, A. Heimann, T. Schroeder, O. Kempski and C. Sönnichsen, Implantable sensors based on gold nanoparticles for continuous long-term concentration monitoring in the body, Nano Lett., 2021, 21(7), 3325–3330 CrossRef CAS PubMed.
- L. A. Kurup, C. M. Cole, J. N. Arthur and S. D. Yambem, Graphene porous foams for capacitive pressure sensing, ACS Appl. Nano Mater., 2022, 5(2), 2973–2983 CrossRef CAS.
- K. Chen, M. Liu, F. Wang, Y. Hu, P. Liu, C. Li, Q. Du, Y. Yu, X. Xiao and Q. Feng, Highly transparent, self-healing, and self-adhesive double network hydrogel for wearable sensors, Front. Bioeng. Biotechnol., 2022, 10, 846401 CrossRef.
- Q. Du, L. Liu, R. Tang, J. Ai, Z. Wang, Q. Fu, C. Li, Y. Chen and X. Feng, High-performance flexible pressure sensor based on controllable hierarchical microstructures by laser scribing for wearable electronics, Adv. Mater. Technol., 2021, 6(9), 2100122 CrossRef CAS.
- P. Kalimuthu, J. F. Gonzalez-Martinez, D. Jakubauskas, M. Cardenas, T. Ruzgas and J. Sotres, Battery-free radio frequency wireless sensor for bacteria based on their degradation of gelatin-fatty acid composite films, Electrochim. Acta, 2021, 381, 138275 CrossRef CAS.
- N. Agrawal, N. Soleja, R. Bano, R. Nazir, T. O. Siddiqi and M. Mohsin, FRET-based genetically encoded sensor to monitor silver ions, ACS Omega, 2021, 6(22), 14164–14173 CrossRef CAS PubMed.
- F. Wang, L. Chen, J. Zhu, X. Hu and Y. Yang, A phosphorescence quenching-based intelligent dissolved oxygen sensor on an optofluidic platform, Micromachines, 2021, 12(3), 281 CrossRef PubMed.
- J. D. Prieto Prada, J. Im, H. Oh and C. Song, Enhanced location tracking in sensor fusion-assisted virtual reality micro-manipulation environments, PLoS One, 2021, 16(12), e0261933 CrossRef CAS.
- A. K. Teo, Y. Choudhury, I. B. Tan, C. Y. Cher, S. H. Chew, Z. Y. Wan, L. T. Cheng, L. L. Oon, M. H. Tan, K. S. Chan and L. Y. Hsu, Saliva is more sensitive than nasopharyngeal or nasal swabs for diagnosis of asymptomatic and mild COVID-19 infection, Sci. Rep., 2021, 11(1), 3134 CrossRef CAS PubMed.
- F. Kibria, W. Rahman and S. N. Patra, Electrospinning-based high-sensitive PVDF-TrFE nanofibre sensor with sensitivity dependence on pore diameter, Curr. Sci., 2020, 119(5), 841–849 CrossRef CAS.
- E. S. Hosseini, L. Manjakkal, D. Shakthivel and R. Dahiya, Glycine–chitosan-based flexible biodegradable piezoelectric pressure sensor, ACS Appl. Mater. Interfaces, 2020, 12(8), 9008–9016 CrossRef CAS.
- Y. Xiong, J. Zhang, Z. Yang, Q. Mou, Y. Ma, Y. Xiong and Y. Lu, Functional DNA regulated CRISPR-Cas12a sensors for point-of-care diagnostics of non-nucleic-acid targets, J. Am. Chem. Soc., 2019, 142(1), 207–213 CrossRef PubMed.
- N. Colozza, V. Caratelli, D. Moscone and F. Arduini, Based devices as new smart analytical tools for sustainable detection of environmental pollutants, Case Stud. Chem. Environ. Eng., 2021, 4, 100167 CrossRef CAS.
- J. S. Stefano, L. R. Silva and B. C. Janegitz, New carbon black-based conductive filaments for the additive manufacture of improved electrochemical sensors by fused deposition modeling, Microchim. Acta, 2022, 189(11), 414 CrossRef CAS PubMed.
- B. Xu, Z. Zhang, P. Zhang, L. Wang, R. Yuan, Z. Ju and W. Liu, High-Yield Production of Water-Soluble MoS2 Quantum Dots for Fe3+ Detection and Cell Imaging, Nanomaterials, 2020, 10(11), 2155 CrossRef CAS PubMed.
- Z. Wan, M. Umer, M. Lobino, D. Thiel, N. T. Nguyen, A. Trinchi, M. J. Shiddiky, Y. Gao and Q. Li, Laser induced self-N-doped porous graphene as an electrochemical biosensor for femtomolar miRNA detection, Carbon, 2020, 163, 385–394 CrossRef CAS.
- Y. Wang, S. Zeng, A. Crunteanu, Z. Xie, G. Humbert, L. Ma, Y. Wei, A. Brunel, B. Bessette, J. C. Orlianges and F. Lalloué, Targeted sub-attomole cancer biomarker detection based on phase singularity 2D nanomaterial-enhanced plasmonic biosensor, Nano-Micro Lett., 2021, 13, 1 CrossRef CAS.
- G. Chellasamy, S. R. Ankireddy, K. N. Lee, S. Govindaraju and K. Yun, Smartphone-integrated colorimetric sensor array-based reader system and fluorometric detection of dopamine in male and female geriatric plasma by bluish-green fluorescent carbon quantum dots, Mater. Today Bio, 2021, 12, 100168 CrossRef PubMed.
-
Z. Wang, Manufacturing and characterization of blended PVDF/PVDF-TrFE polymers: a study towards future sensor applications, MSc, University of Alberta, USA, 2022, DOI:10.7939/r3-hx9h-5m71.
- C. Y. Chen, W. C. Lin and H. Y. Yang, Diagnosis of ventilator-associated pneumonia using electronic nose sensor array signals: solutions to improve the application of machine learning in respiratory research, Respir. Res., 2020, 21, 1–2 CrossRef.
- I. Iwe and Z. Li, An isothermal, non-enzymatic, and dual-amplified fluorescent sensor for highly sensitive DNA detection, Rev. Anal. Chem., 2021, 40(1), 312–322 CrossRef CAS.
- L. P. Shivangani, P. K. Singh, S. Singh and D. K. Dwivedi, Design and modeling of reconfigurable surface plasmon resonance refractive index sensor using Al2O3, nickel, and heterostructure BlueP/WSe2 nanofilms, J. Opt., 2023, 52(3), 1358–1369 CrossRef.
- P. C. Su, B. H. Chen, Y. C. Lee and Y. S. Yang, Silicon nanowire field-effect transistor as biosensing platforms for post-translational modification, Biosensors, 2020, 10(12), 213 CrossRef CAS PubMed.
- H. Hao, S. P. Eckel, A. Hosseini, E. D. Van Vliet, E. Dzubur, G. Dunton, S. Y. Chang, K. Craig, R. Rocchio, T. Bastain and F. Gilliland, Daily associations of air pollution and pediatric asthma risk using the biomedical REAI-Time Health Evaluation (BREATHE) Kit, Int. J. Environ. Res. Public Health, 2022, 19(6), 3578 CrossRef CAS PubMed.
- E. Y. Cramer, E. L. Ray, V. K. Lopez, J. Bracher, A. Brennen, A. J. Castro Rivadeneira, A. Gerding, T. Gneiting, K. H. House, Y. Huang and D. Jayawardena, Evaluation of individual and ensemble probabilistic forecasts of COVID-19 mortality in the United States, Proc. Natl. Acad. Sci. U. S. A., 2022, 119(15), e2113561119 CrossRef CAS PubMed.
- Y. Rui, R. D. Jackson, M. F. Cotrufo, G. R. Sanford, B. J. Spiesman, L. Deiss, S. W. Culman, C. Liang and M. D. Ruark, Reply to Lajtha and Silva: Agriculture and soil carbon persistence of grassland-derived Mollisols, Proc. Natl. Acad. Sci. U. S. A., 2022, 119(30), e2204142119 CrossRef CAS.
- V. K. Sharma, S. C. Gupta, B. N. Singh, C. V. Rao and S. K. Barik, Cinnamomum verum-derived bioactives-functionalized gold nanoparticles for prevention of obesity through gut microbiota reshaping, Mater. Today Bio, 2022, 13, 100204 CrossRef CAS PubMed.
- C. E. McGhee, Z. Yang, W. Guo, Y. Wu, M. Lyu, C. J. DeLong, S. Hong, Y. Ma, M. G. McInnis, K. S. O'Shea and Y. Lu, DNAzyme-based lithium-selective imaging reveals higher lithium accumulation in bipolar disorder patient-derived neurons, ACS Cent. Sci., 2021, 7(11), 1809–1820 CrossRef CAS PubMed.
- H. Areiza-Laverde, C. Dopierala, L. Senhadji, F. Boucher, P. Y. Gumery and A. Hernández, Analysis of cardiac vibration signals acquired from a novel implant placed on the gastric fundus, Front. Physiol., 2021, 12, 748367 CrossRef PubMed.
- J. Fu, S. Cao, L. Cai and L. Yang, Finger gesture recognition using sensing and classification of surface electromyography signals with high-precision wireless surface electromyography sensors, Front. Comput. Neurosci., 2021, 15, 770692 CrossRef PubMed.
- S. Hassantabar, N. Stefano, V. Ghanakota, A. Ferrari, G. N. Nicola, R. Bruno, I. R. Marino, K. Hamidouche and N. K. Jha, CovidDeep: SARS-CoV-2/COVID-19 test based on wearable medical sensors and efficient neural networks, IEEE Trans. Consum. Electron., 2021, 67(4), 244–256 Search PubMed.
- S. Khaksar, H. Pan, B. Borazjani, I. Murray, H. Agrawal, W. Liu, C. Elliott, C. Imms, A. Campbell and C. Walmsley, Application of inertial measurement units and machine learning classification in cerebral palsy: Randomized controlled trial, JMIR Rehabil. Assist. Technol., 2021, 8(4), e29769 CrossRef PubMed.
- H. Zhang, W. Zhu, S. Ye, S. Li, B. Yu, Z. Pang and R. Nie, Monitoring of non-invasive vital signs for detection of sleep apnea, J. Mech. Med. Biol., 2021, 21(05), 2140007 CrossRef.
- Y. H. Kwon, J. Fernandes, J. J. Kim, J. Chen and H. Jiang, Micro-Actuated Tunable Hierarchical Silver Nanostructures to Measure Tensile Force for Biomedical Wearable Sensing Applications, Micromachines, 2021, 12(5), 476 CrossRef PubMed.
- B. Ghosh, S. Roy, S. Bardhan, D. Mondal, I. Saha, S. Ghosh, R. Basu, P. Karmakar, K. Das and S. Das, Biocompatible carbon dot decorated α-FeOOH nanohybrid for an effective fluorometric sensing of Cr (VI) in wastewater and living cells, J. Fluoresc., 2022, 32(4), 1489–1500 CrossRef CAS PubMed.
- K. K. Poon, M. C. Wurm, D. M. Evans, M. A. Einarsrud, R. Lutz and J. Glaum, Biocompatibility of (Ba, Ca)(Zr, Ti) O3 piezoelectric ceramics for bone replacement materials, J. Biomed. Mater. Res., Part B, 2020, 108(4), 1295–1303 CrossRef CAS PubMed.
- Y. Cao and K. E. Uhrich, Biodegradable and biocompatible polymers for electronic applications: A review, J. Bioact. Compat. Polym., 2019, 34(1), 3–15 CrossRef CAS.
- K. Ito, H. Satake, Y. Mori, A. C. Tseng and T. Sakata, Biocompatible and Na+−sensitive thin-film transistor for biological fluid sensing, Sci. Technol. Adv. Mater., 2019, 20(1), 917–926 CrossRef CAS.
- R. Wang and H. Tong, Preparation methods and functional characteristics of regenerated keratin-based biofilms, Polymers, 2022, 14(21), 4723 CrossRef CAS.
- K. Uysal, T. Creutz, I. S. Firat, G. M. Artmann, N. Teusch and A. A. Temiz, Bio-functionalized ultra-thin, large-area and waterproof silicone membranes for biomechanical cellular loading and compliance experiments, Polymers, 2022, 14(11), 2213 CrossRef CAS PubMed.
- J. L. Pichardo-Molina, A. P. Andrade-Pérez, J. Torres-Ramírez, J. C. Martínez-Espinosa, J. C. Villagómez-Castro, J. P. Castruita-Domínguez, I. S. Tránsito and L. L. Flores-Villavicencio, Biological Impact of the Interaction of Functionalized and Bioconjugated Gold Nanoparticles of Different Sizes on HeLa and SH-SY5Y Human Cell Lines, J. Nanomater., 2022, 2022, 9711723 Search PubMed.
- A. Kwiatkowska, M. Drabik, A. Lipko, A. Grzeczkowicz, R. Stachowiak, A. Marszalik and L. H. Granicka, Composite membrane dressings system with metallic nanoparticles as an antibacterial factor in wound healing, Membranes, 2022, 12(2), 215 CrossRef CAS PubMed.
- S. K. Chandraker, M. Lal, P. Dhruve, R. P. Singh and R. Shukla, Cytotoxic, antimitotic, DNA binding, photocatalytic, H2O2 sensing, and antioxidant properties of biofabricated silver nanoparticles using leaf extract of Bryophyllum pinnatum (Lam.) Oken, Front. Mol. Biosci., 2021, 7, 593040 CrossRef PubMed.
- X. He, H. Jiang, J. Li, Y. Ma, B. Fu and C. Hu, Dipole-moment induced phototaxis and fuel-free propulsion of ZnO/Pt Janus micromotors, Small, 2021, 17(31), 2101388 CrossRef CAS.
- S. Z. Homayounfar, S. Rostaminia, A. Kiaghadi, X. Chen, E. T. Alexander, D. Ganesan and T. L. Andrew, Multimodal smart eyewear for longitudinal eye movement tracking, Matter, 2020, 3(4), 1275–1293 CrossRef.
- D. Lu, Y. Yan, R. Avila, I. Kandela, I. Stepien, M. H. Seo, W. Bai, Q. Yang, C. Li, C. R. Haney and E. A. Waters, Bioresorbable, wireless, passive sensors as temporary implants for monitoring regional body temperature, Adv. Healthcare Mater., 2020, 9(16), 2000942 CrossRef CAS.
- L. Gómez-Caudillo, A. J. Ortega-Lozano, Á. G. Martínez-Batallar, H. Rosas-Vargas, F. Minauro-Sanmiguel and S. Encarnación-Guevara, Principal component analysis on LC-MS/MS and 2DE-MALDI-TOF in glioblastoma cell lines reveals that mitochondria act as organelle sensors of the metabolic state in glioblastoma, Oncol. Rep., 2020, 44(2), 661–673 CrossRef.
- S. Singh, S. Singh, P. K. Singh, R. K. Yadav, P. Lohia and D. K. Dwivedi, Theoretical study of malaria detection in blood samples using bimetal layer and zinc telluride nanomaterial-based surface plasmon resonance biosensor, Plasmonics, 2023, 18(6), 2125–2136 CrossRef CAS.
- M. O. Nayeem, S. Lee, H. Jin, N. Matsuhisa, H. Jinno, A. Miyamoto, T. Yokota and T. Someya, All-nanofiber–based, ultrasensitive, gas-permeable mechanoacoustic sensors for continuous long-term heart monitoring, Proc. Natl. Acad. Sci. U. S. A., 2020, 117(13), 7063–7070 CrossRef PubMed.
- S. Zhang, J. Huang, J. Lu, M. Liu, Y. Li, L. Fang, H. Huang, J. Huang, F. Mo and J. Zheng, A novel fluorescent biosensor based on dendritic DNA nanostructure in combination with ligase reaction for ultrasensitive detection of DNA methylation, J. Nanobiotechnol., 2019, 17, 1 CrossRef PubMed.
- E. Campbell, M. T. Hasan, C. Pho, K. Callaghan, G. R. Akkaraju and A. V. Naumov, Graphene oxide as a multifunctional platform for intracellular delivery, imaging, and cancer sensing, Sci. Rep., 2019, 9(1), 416 CrossRef CAS.
- A. K. Jaiswal, A. Hokkanen, M. Kapulainen, A. Khakalo, N. Nonappa, O. Ikkala and H. Orelma, Carboxymethyl cellulose (CMC) optical fibers for environment sensing and short-range optical signal transmission, ACS Appl. Mater. Interfaces, 2022, 14(2), 3315–3323 CrossRef CAS PubMed.
- S. E. Saddow, Silicon carbide technology for advanced human healthcare applications, Micromachines, 2022, 13(3), 346 CrossRef PubMed.
- S. Qian, Y. Cui, Z. Cai and L. Li, Applications of smartphone-based colorimetric biosensors, Biosens. Bioelectron.: X, 2022, 11, 100173 CAS.
- N. Cennamo, A. M. Bossi, F. Arcadio, D. Maniglio and L. Zeni, On the effect of soft molecularly imprinted nanoparticles receptors combined to nanoplasmonic probes for biomedical applications, Front. Bioeng. Biotechnol., 2021, 9, 801489 CrossRef PubMed.
- G. Shen, Recent advances of flexible sensors for biomedical applications, Prog. Nat. Sci.: Mater. Int., 2021, 31(6), 872–882 CrossRef CAS.
- M. Rotan, M. Zhuk, P. Boughton and J. Glaum, The influence of low-temperature sterilization procedures on piezoelectric ceramics for biomedical applications, Open Ceram., 2021, 7, 100143 CrossRef CAS.
- F. Pinelli, T. Nespoli, A. Fiorati, S. Farè, L. Magagnin and F. Rossi, Graphene nanoplatelets can improve the performances of graphene oxide–polyaniline composite gas sensing aerogels, Carbon Trends, 2021, 5, 100123 CrossRef CAS.
- T. Wittenberg, M. Benz, A. Foltyn, R. Hackner, J. Hetzel, V. Wiesmann and T. Eixelberger, Acquisition of Semantics for AI-based Applications in Medical Technologies: An overview, Curr. Dir. Biomed. Eng., 2021, 7(2), 515–518 CrossRef.
- H. Sugai, S. Tomita and R. Kurita, Pattern-recognition-based sensor arrays for cell characterization: From materials and data analyses to biomedical applications, Anal. Sci., 2020, 36(8), 923–934 CrossRef CAS PubMed.
- D. Rotake, A. Darji and N. Kale, Fabrication, calibration, and preliminary testing of microcantilever-based piezoresistive sensor for BioMEMS applications, IET Nanobiotechnol., 2020, 14(5), 357–368 CrossRef PubMed.
- A. Najafzadeh, D. Serandi Gunawardena, Z. Liu, T. Tran, H. Y. Tam, J. Fu and B. K. Chen, Application of fibre bragg grating sensors in strain monitoring and fracture recovery of human femur bone, Bioengineering, 2020, 7(3), 98 CrossRef PubMed.
- A. Zanut, A. Cian, N. Cefarin, A. Pozzato and M. Tormen, Nanoelectrode arrays fabricated by thermal nanoimprint lithography for biosensing application, Biosensors, 2020, 10(8), 90 CrossRef CAS PubMed.
- D. Hardman, T. George Thuruthel and F. Iida, Self-healing ionic gelatin/glycerol hydrogels for strain sensing applications, NPG Asia Mater., 2022, 14(1), 11 CrossRef CAS.
- S. P. Nunes, R. Henrique, C. Jerónimo and J. M. Paramio, DNA methylation as a therapeutic target for bladder cancer, Cells, 2020, 9(8), 1850 CrossRef CAS.
- Y. Jiang, L. Gong, X. Hu, Y. Zhao, H. Chen, L. Feng and D. Zhang, Aligned P (VDF-TrFE) nanofibers for enhanced piezoelectric directional strain sensing, Polymers, 2018, 10(4), 364 CrossRef PubMed.
- A. Esteva, K. Chou, S. Yeung, N. Naik, A. Madani, A. Mottaghi, Y. Liu, E. Topol, J. Dean and R. Socher, Deep learning-enabled medical computer vision, NPJ Digit. Med., 2021, 4(1), 5 CrossRef PubMed.
- L. C. Almeida, T. Frade, R. D. Correia, Y. Niu, G. Jin, J. P. Correia and A. S. Viana, Electrosynthesis of polydopamine-ethanolamine films for the development of immunosensing interfaces, Sci. Rep., 2021, 11(1), 2237 CrossRef CAS PubMed.
- H. Joo, Y. Lee, J. Kim, J. S. Yoo, S. Yoo, S. Kim, A. K. Arya, S. Kim, S. H. Choi, N. Lu and H. S. Lee, Soft implantable drug delivery device integrated wirelessly with wearable devices to treat fatal seizures, Sci. Adv., 2021, 7(1), eabd4639 CrossRef CAS PubMed.
- L. Gao, J. Yu, Y. Li, P. Wang, J. Shu, X. Deng and L. Li, An ultrahigh sensitive paper-based pressure sensor with intelligent thermotherapy for skin-integrated electronics, Nanomaterials, 2020, 10(12), 2536 CrossRef CAS PubMed.
- J. Yao, W. Qiang, H. Wei, Y. Xu, B. Wang, Y. Zheng, X. Wang, Z. Miao, L. Wang, S. Wang and X. Yang, Ultrathin and Robust Micro–Nano Composite Coating for Implantable Pressure Sensor Encapsulation, ACS Omega, 2020, 5(36), 23129–23139 CrossRef CAS PubMed.
- P. H. Lin, W. L. Chang, S. C. Sheu and B. R. Li, A Noninvasive Wearable Device for Real-Time Monitoring of Secretion Sweat Pressure by Digital Display, iScience, 2020, 23(11), 101658 CrossRef PubMed.
- M. A. Sayeed, S. P. Mohanty, E. Kougianos and H. P. Zaveri, eSeiz: An edge-device for accurate seizure detection for smart healthcare, IEEE Trans. Consum. Electron., 2019, 65(3), 379–387 Search PubMed.
- R. Sun, S. C. Carreira, Y. Chen, C. Xiang, L. Xu, B. Zhang, M. Chen, I. Farrow, F. Scarpa and J. Rossiter, Stretchable piezoelectric sensing systems for self-powered and wireless health monitoring, Adv. Mater. Technol., 2019, 4(5), 1900100 CrossRef.
- M. Arifuzzaman, P. W. Millhouse, Y. Raval, T. B. Pace, C. J. Behrend, S. B. Behbahani, J. D. DesJardins, T. R. Tzeng and J. N. Anker, An implanted pH sensor read using radiography, Analyst, 2019, 144(9), 2984–2993 RSC.
- B. Lee, H. Cho, S. Jeong, J. Yoon, D. Jang, D. K. Lee, D. Kim, S. Chung and Y. Hong, Stretchable hybrid electronics: combining rigid electronic devices with stretchable interconnects into high-performance on-skin electronics, J. Inf. Disp., 2022, 23(3), 163–184 CrossRef.
- B. Patra, A. K. Mishra and R. S. Verma, Label-free serum albumin nanoparticles for bioimaging and Trojan horse-like drug delivery, J. Sci.: Adv. Mater. Devices, 2022, 7(1), 100406 CAS.
- K. Głowacz, S. Skorupska, I. Grabowska-Jadach and P. Ciosek-Skibińska, Excitation–emission matrix fluorescence spectroscopy for cell viability testing in UV-treated cell culture, RSC Adv., 2022, 12(13), 7652–7660 RSC.
- K. Shima, K. Shimatani and M. Sakata, A wearable light-touch contact device for human balance support, Sci. Rep., 2021, 11(1), 7324 CrossRef CAS PubMed.
- C. P. Adans-Dester, C. E. Lang, D. J. Reinkensmeyer and P. Bonato, Wearable sensors for stroke rehabilitation, Neurorehabilitation Technology, 2022, 467–507 CrossRef.
- U. Zupančič, J. Rainbow, P. Estrela and D. Moschou, Utilising commercially fabricated printed circuit boards as an electrochemical biosensing platform, Micromachines, 2021, 12(7), 793 CrossRef.
- P. Tecchio, A. Monte and P. Zamparo, Low-cost electromyography: validity against a commercial system depends on exercise type and intensity, Eur. J. Transl. Myol., 2021, 31(2), 9735 CrossRef.
- B. Bent, P. J. Cho, A. Wittmann, C. Thacker, S. Muppidi, M. Snyder, M. J. Crowley, M. Feinglos and J. P. Dunn, Non-invasive wearables for remote monitoring of HbA1c and glucose variability: proof of concept, BMJ Open Diabetes Res. Care, 2021, 9(1), e002027 CrossRef PubMed.
- I. O. Oguntoye, B. K. Simone, S. Padmanabha, G. Z. Hartfield, P. Amrollahi, T. Y. Hu, A. J. Ollanik and M. D. Escarra, Silicon nanodisk Huygens metasurfaces for portable and low-cost refractive index and biomarker sensing, ACS Appl. Nano Mater., 2022, 5(3), 3983–3991 CrossRef CAS PubMed.
- Y. Li, H. Tang, Y. Liu, Y. Qiao, H. Xia and J. Zhou, Oral wearable sensors: health management based on the oral cavity, Biosens. Bioelectron.: X, 2022, 10, 100135 CAS.
- C. Shi, Z. Zou, Z. Lei, P. Zhu, G. Nie, W. Zhang and J. Xiao, Stretchable, Rehealable, Recyclable, and Reconfigurable Integrated Strain Sensor for Joint Motion and Respiration Monitoring, Research, 2021, 9846036 CAS.
- N. Mark, A. Lyubin, R. Gerasi, D. Ofir, A. M. Tsur, J. Chen and T. Bader, Comparison of the Effects of Motion and Environment Conditions on Accuracy of Handheld and Finger-Based Pulse Oximeters, Mil. Med., 2021, 186(Supplement_1), 465–472 CrossRef PubMed.
- M. Zheng, A. Patel, A. Khoja, Y. Luo, W. Lin, Q. He, X. Zhao, J. Wang, S. Yang and P. Hu, Barriers and facilitators of diabetes management by continuous glucose monitoring systems among adults with type 2 diabetes: a protocol of qualitative systematic review, BMJ Open, 2021, 11(10), e046050 CrossRef PubMed.
- M. Qassem, L. Constantinou, I. F. Triantis, M. Hickey, E. Palazidou and P. A. Kyriacou, A method for rapid, reliable, and low-volume measurement of lithium in blood for use in bipolar disorder treatment management, IEEE Trans. Biomed. Eng., 2018, 66(1), 130–137 Search PubMed.
- D. F. Williams, Biocompatibility pathways: biomaterials-induced sterile inflammation, mechanotransduction, and principles of biocompatibility control, ACS Biomater. Sci. Eng., 2017, 3(1), 2–35 CrossRef CAS.
- Q. Wei, Y. Su, H. Xin, L. Zhang, J. Ding and X. Chen, Immunologically effective biomaterials, ACS Appl. Mater. Interfaces, 2021, 13(48), 56719–56724 CrossRef CAS.
- D. Zhu, I. Cockerill, Y. Su, Z. Zhang, J. Fu, K. W. Lee, J. Ma, C. Okpokwasili, L. Tang, Y. Zheng and Y. X. Qin, Mechanical strength, biodegradation, and in vitro and in vivo biocompatibility of Zn biomaterials, ACS Appl. Mater. Interfaces, 2019, 11(7), 6809–6819 CrossRef CAS PubMed.
- B. Singh, J. Kim, N. Shukla, J. Lee, K. Kim and M. H. Park, Smart Delivery Platform Using Core–Shell Nanofibers for Sequential Drug Release in Wound Healing, ACS Appl. Bio Mater., 2023, 6(6), 2314–2324 CrossRef CAS PubMed.
- T. Zhu, M. Jiang, M. Zhang, L. Cui, X. Yang, X. Wang, G. Liu, J. Ding and X. Chen, Biofunctionalized composite scaffold to potentiate osteoconduction, angiogenesis, and favorable metabolic microenvironment for osteonecrosis therapy, Bioact. Mater., 2022, 9, 446–460 CAS.
- A. Tiwari, Advancement of Materials to Sustainable & Green World, Adv. Mater. Lett., 2023, 14(3), 23031724 Search PubMed.
- A. Tiwari, Climate Diplomacy to Attain Global Eco-Neutrality, Adv. Mater. Lett., 2022, 13(3), 22031697 Search PubMed.
-
J. J. Tobin and G. Walsh, Medical product regulatory affairs: pharmaceuticals, diagnostics, medical devices, John Wiley & Sons, 2023 Search PubMed.
- S. K. Shukla, S. Patra, T. R. Das, D. Kumar, A. Mishra and A. Tiwari, Progress in COVID research and developments during pandemic, View, 2022, 3(6), 20210020 CrossRef CAS PubMed.
- M. Ashaduzzaman, S. R. Deshpande, N. A. Murugan, Y. K. Mishra, A. P. Turner and A. Tiwari, On/off-switchable LSPR nano-immunoassay for troponin-T, Sci. Rep., 2017, 7(1), 44027 CrossRef CAS PubMed.
-
Biomedical materials and diagnostic devices, ed. A. Tiwari, M. Ramalingam, H. Kobayashi and A. P. Turner, John Wiley & Sons, 2012 Search PubMed.
- M. K. Hossain, A. Mishra, A. Tiwari, B. Pant, S. C. Dey, A. Tiwari, O. Saha, M. M. Rahaman, Y. R. Shukla, A. Tiwari and M. Ashaduzzaman, Thermally induced Fe2O3 spikes decorated Ag/Fe2O3 nanocomposite fabrication for anti-bacterial and anti-cancer activities, SN Appl. Sci., 2023, 5(12), 339 CrossRef CAS.
- N. Karimian, M. H. Zavar, M. Chamsaz, A. P. Turner and A. Tiwari, On/off-switchable electrochemical folic acid sensor based on molecularly imprinted polymer electrode, Electrochem. Commun., 2013, 36, 92–95 CrossRef CAS.
- B. K. Nahak, A. Mishra, S. Preetam and A. Tiwari, Advances in organ-on-a-chip materials and devices, ACS Appl. Bio Mater., 2022, 5(8), 3576–3607 CrossRef CAS PubMed.
- O. Parlak, A. İncel, L. Uzun, A. P. Turner and A. Tiwari, Structuring Au nanoparticles on two-dimensional MoS2 nanosheets for electrochemical glucose biosensors, Biosens. Bioelectron., 2017, 89, 545–550 CrossRef CAS.
- O. Parlak, S. Beyazit, B. Tse-Sum-Bui, K. Haupt, A. P. Turner and A. Tiwari, Programmable bioelectronics in a stimuli-encoded 3D graphene interface, Nanoscale, 2016, 8(19), 9976–9981 RSC.
- S. H. Choi, S. J. Yun, Y. S. Won, C. S. Oh, S. M. Kim, K. K. Kim and Y. H. Lee, Large-scale synthesis of graphene and other 2D materials towards industrialization, Nat. Commun., 2022, 13(1), 1484 CrossRef CAS PubMed.
-
A. Mishra, S. K. Shukla, S. Patra, S. Tripathi and A. Tiwari, Risk, Relevance and Roadmap for Sustainable Prognosis Model of COVID-19, Authorea Preprints, 2023 Search PubMed.
- A. Mishra, S. Basumallick, A. Lu, H. Chiu, M. A. Shah, Y. Shukla and A. Tiwari, The healthier healthcare management models for COVID-19, Journal of Infection and Public Health, 2021, 14(7), 927–937 CrossRef PubMed.
- B. Vural, İ. Uludağ, B. Ince, C. Özyurt, F. Öztürk and M. K. Sezgintürk, Fluid-based wearable sensors: a turning point in personalized healthcare, Turk. J. Chem., 2023, 47(5), 944–967 CrossRef PubMed.
- S. A. Alowais, S. S. Alghamdi, N. Alsuhebany, T. Alqahtani, A. I. Alshaya, S. N. Almohareb, A. Aldairem, M. Alrashed, K. Bin Saleh, H. A. Badreldin and M. S. Al Yami, Revolutionizing healthcare: the role of artificial intelligence in clinical practice, BMC Med. Educ., 2023, 23(1), 689 CrossRef PubMed.
- P. Manickam, S. A. Mariappan, S. M. Murugesan, S. Hansda, A. Kaushik, R. Shinde and S. P. Thipperudraswamy, Artificial intelligence (AI) and internet of medical things (IoMT) assisted biomedical systems for intelligent healthcare, Biosensors, 2022, 12(8), 562 CrossRef CAS.
Footnote |
† Equal contribution. |
|
This journal is © The Royal Society of Chemistry 2024 |
Click here to see how this site uses Cookies. View our privacy policy here.