DOI:
10.1039/D3SD00325F
(Paper)
Sens. Diagn., 2024,
3, 1028-1038
Active loading of cyanine 5.5 derivatives into liposomes for deep self-quenching and their applications in deep tissue imaging†
Received
9th December 2023
, Accepted 1st April 2024
First published on 3rd May 2024
Abstract
Visualizing liposome release profiles in small animals is important for evaluating the pharmacokinetic influence of vesicles. Encapsulating near-infrared (NIR) fluorescent dyes to visualize and report liposomal cargo release in vivo, which necessitates high encapsulation with deep self-quenching, is highly desirable in advanced (such as targeting or trigger-release) liposome development. However, passive loading of NIR dyes usually yields low encapsulation efficiencies (1–5%), causing significant wastage and cost-ineffectiveness while using expensive NIR fluorescent dyes. It would be highly beneficial if an active loading method, which typically has an encapsulation efficiency of nearly 100%, is developed. This research describes an active loading approach for two cyanine 5.5 (Cy5.5) derivatives. We discovered that using ammonium sucrose octasulfate (ASO) as a trapping agent allows for nearly 100% encapsulation for both Cy5.5 dyes, accompanied by the formation of nanoprecipitates inside the liposome, as evidenced by cryogenic electron microscopy. Fluorescence spectroscopy confirmed deep fluorescence self-quenching after active loading and a 60–100-fold fluorescence enhancement upon full content release via liposome rupture. Cellular uptake experiments showed that the fluorescence of Cy5.5-loaded liposomes recovered and plateaued after 9 hours of incubation with cells. In vivo fluorescence imaging (IVIS) demonstrated the same fluorescence activation in tumor-bearing mice intratumorally injected with the liposome. We believe that the developed active loading method will enable Cy5.5-loaded liposomes to be a deep tissue-compatible and cost-effective NIR fluorescence release-reporting platform.
Liposomes have proven to be successful drug delivery systems for clinical applications.1 Unlike the free form of chemotherapeutics, liposomal drug formulation prevents toxic chemotherapeutics from prematurely damaging healthy tissues and gives the encapsulated drug more time to circulate in the bloodstream and accumulate in malignant lesions through the enhanced permeability and retention (EPR) effect.2 This advantage greatly enhances the safety of chemotherapeutics and reduces undesirable adverse effects.3 A good example is that doxorubicin has severe cardiotoxicity, but the liposome-formulated doxorubicin, Doxil®, completely suppresses the side effects.4 Although liposome formulation makes drugs safer, it does not make the drugs more effective other than providing better biodistribution by EPR effect.5,6 More advanced liposome designs such as smart liposomes that can be trigger-released at the intended site are needed. Developing such trigger-release liposome systems that work in animals thus requires an appropriate in vivo reporting system to allow scientists/developers to visualize liposome release profiles such as release extent and location, at least in small animals.7–9 Because liposome release is an action of dilution, fluorescence imaging can generate higher signal enhancement/contrast upon liposome rupture and dilution of deep self-quenched fluorescent dyes.10,11 Therefore, it would be beneficial if a highly concentrated, deep self-quenched NIR fluorescent dye can be encapsulated into liposomes for this purpose.
Fluorescence imaging has been utilized as a diagnostic and tracking/labeling tool in vitro and in vivo for decades.12 Liposomes with unquenched fluorophores can facilitate tracking, whereas liposomes with highly self-quenched fluorescent dyes can report content release by fluorescence dequenching if liposome trigger release is designed to respond to biochemical cues. However, the concentration of the loaded dye severely affects the quenching efficiency.13 The dynamic range of signal enhancement is defined as unquenched fluorescence over self-quenched fluorescence and, thus, is governed by how low the fluorescence intensity can be quenched. One would prefer to have 20-fold or more fluorescence enhancement upon liposome release. Hence, liposome loading is critical in order to drive the loaded dye to a high concentration toward the self-quenching regime.
Conventional visible-light fluorescent dyes (such as fluorescein) were loaded into liposomes using the very inefficient method called passive loading for some in vitro assays to estimate liposome release.13–17 Fluorescein (50–100 mM) in a physiologically isotonic buffer was prepared to mix with lipid films to generate dye-encapsulated liposomes with an encapsulation efficiency normally lower than 5% without laborious recycling of the unencapsulated (>95%) dye. Such an inefficient method was used for decades simply because of the low cost of the dyes (the bulk retail price for fluorescein is approximately 0.34 USD per g in Sigma-Aldrich). As the demand for expensive near-infrared (NIR) fluorescent dyes with absorption/emission wavelengths of 650–900 nm (the first biological window) grows for in vivo applications,18 an efficient near-infrared fluorescent dye loading method for liposomes is needed. Otherwise the dye encapsulation would be extremely costly and could hinder the progress of this research field (for example, indocyanine green is 1876 USD per g from Sigma-Aldrich, DY-676 is 17
100 EUR per g from Dyomics GmbH, and the lowest unit price for Cy5.5 carboxylic acid is approximately 11
900 USD per g from Lumiprobe to date). Recently, liposomes encapsulating highly concentrated and self-quenched NIR fluorophores to estimate in vivo liposome trigger release have been reported.8,9,19–21 However, all of those works used inefficient passive loading and resulted in significant reagent costs. If an active loading option is available, one can drive the intraliposome dye concentration extremely high and even force the dye to precipitate. This will guarantee the self-quenching fluorescence intensity to the lowest possible level to maximize the signal enhancement after liposome rupture and maximize the fluorescence signal-to-noise (S/N) ratio and make the in vivo application reliable (Scheme 1).
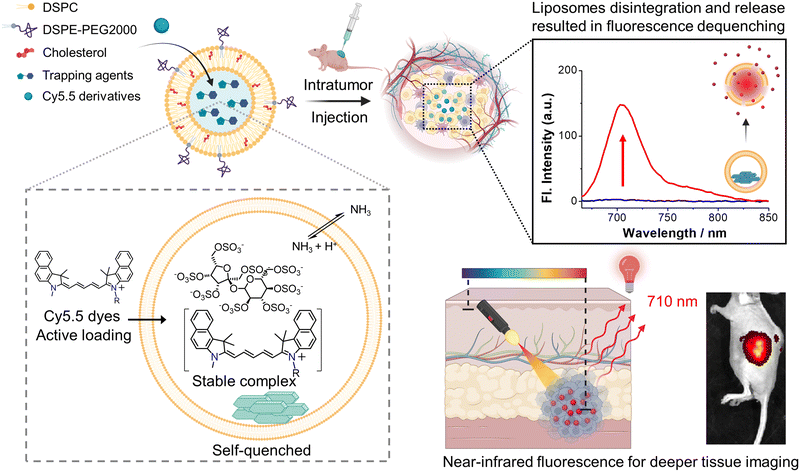 |
| Scheme 1 Schematic illustration of the active loading mechanism of Cy5.5 dye derivatives into the liposome using a cross-membrane ammonium sucrose octasulfate (ASO) gradient as a trapping agent, and in small-animal experiments, self-quenched Cy5.5-loaded liposomes exhibit significant fluorescence enhancement with an excellent signal-to-noise ratio in a specific tissue depth after liposome degradation causes dye release. Cartoons in the graphic scheme were created with https://BioRender.com. | |
Active (or remote) loading can efficiently encapsulate a cargo into a liposome, normally to an oversaturation and cause cargo precipitation.22–25 In most cases, the cargo being loaded has nearly 100% loading efficiency, and intraliposomal cargo amorphous precipitation is observed using a cryogenic electron microscope (cryo-EM). The Achilles heel of active loading, however, is that only the cargo having the right charge, hydrophobicity/hydrophilicity and right functional groups can be loaded using appropriate trapping agents.25,26 The exploration of active loading methods for a new class of cargo is usually complicated and time-consuming.
In this work, a variety of trapping agents were screened to create liposomal transmembrane gradients to actively load near-infrared fluorophores, two cyanine 5.5 (Cy5.5) derivatives. Cy5.5 is a commonly used NIR fluorophore for in vivo imaging due to its excellent optical tissue penetration depth. It is a hybrid of one benzoindoline and one benzoindolium connected by a long polyalkene for large dipole (highly delocalized) resonance for long-wavelength emission, and a nonaromatic benzoindolium amine27 that can counteract with proper trapping agents such as ammonium sulfate28,29 or sucrose octasulfate ammonium salt30–33 to precipitate. We also tested calcium acetate34 and lanthanum acetate to precipitate Cy5.5 derivatives since one of the derivatives has an auxiliary acid group. Our work demonstrated that Cy5.5 can be successfully active-loaded and precipitated inside liposomes to a deep self-quenched concentration, which can generate up to 60- to 100-fold fluorescence enhancement and high absolute fluorescence intensity upon liposomal release in test tubes. The fluorescence properties of the active-loaded Cy5.5 liposomes were further analyzed by fluorescence spectroscopy before and after liposome rupture. We also discovered that Cy5.5 derivatives exhibit a significant quantum yield jump when bound to protein.35 The strong protein binding property and quantum yield enhancement are much valuable in strong reporting intensity and immobilized location with accumulation effects in small-animal whole-body imaging experiments.
Experimental section
Materials and reagents
3H-Indolium, 2-[5-[1-(5-carboxypentyl)-1,3-dihydro-3,3-dimethyl-2H-indol-2-ylidene]-1,3-pentadien-1-yl]-1,3,3-trimethyl-chloride (Cy5.5 acid) was purchased from Lumiprobe. 3-(6-Aminohexyl)-1,1-dimethyl-2-((1E,3E,5E)-5-(1,1,3-trimethyl-1,3-dihydro-2H-benzo[e]indol-2-ylidene)penta-1,3-dien-1-yl)-1H-benzo[e]indol-3-ium iodide (Cy5.5 amine) was synthesized (full description is given in the ESI,† and 1H-NMR spectra are shown in Fig. S5†). Both Cy5.5 dyes were dissolved in DMSO as stock solutions. 1,2-Distearoy-sn-glycero-3-phosphatidylcholine (DSPC) and 1,2-distearoyl-sn-glycero-3-phosphatiylethanol-amine-N-[methoxy(polyethyleneglycol)-2000] (DSPE-PEG2000) were purchased from Avanti Polar Lipids. Sucrose octasulfate ammonium salt was purchased from Toronto Research Chemical. Cholesterol, sucrose, calcium(II) acetate (Ca(OAc)2), ammonium sulfate ((NH4)2SO4), and lanthanum(III) acetate hydrate (La(OAc)3) were obtained from Sigma-Aldrich and J.T. Baker. 4-(2-Hydroxyethyl)-1-piperazineethanesulfonic acid (HEPES) was purchased from Acros Organic (Thermo Fisher Scientific Inc). Bovine serum albumin (BSA) was supplied by VWR Life Science. All other generic chemicals and organic solvents were of analytical grade and were purchased from Sigma-Aldrich.
Evaluation of trapping agents by a solubility test
Trapping agents were evaluated by measuring the solubility of Cy5.5 dyes in the solution, and are listed in Fig. 1a. Approximately 2.5 μL of 10 mM Cy5.5 acid or Cy5.5 amine stock solution was added to 250 μL of the following solutions: (1) HEPES buffer (50 mM, pH 7.4), (2) 10% sucrose solution (in the presence of 5% DMSO, 5 mM HEPES, pH 7.4) as an extraliposomal solution, (3) deionized water, (4) calcium(II) acetate (100 mM, pH 7.4), (5) lanthanum(III) acetate (100 mM, pH 6.2), (6) ammonium sulfate (250 mM, pH 5.5), and (7) ammonium sucrose octasulfate (100 mM, pH 7.4). Each Cy5.5 dye solution/suspension was incubated and ultrasonicated at room temperature for 30 minutes. Insoluble precipitates were removed through centrifugation at 17
000 × g for further 30 minutes, and the supernatant was obtained for solubility quantification by comparing the HPLC peak absorbance areas with the calibration curves.
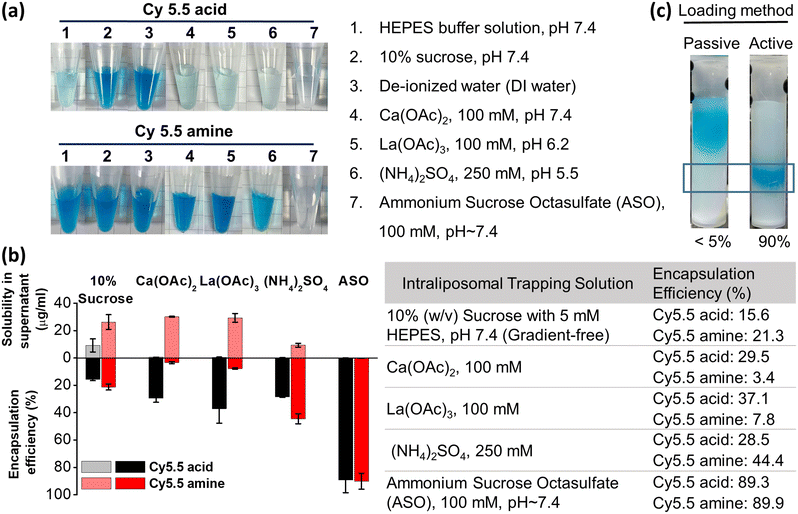 |
| Fig. 1 Trapping agent screening for the active loading of Cy5.5 dyes into liposomes. (a) Supernatants of Cy5.5 dyes in different trapping agent solutions after centrifugation at 17 000 × g for 30 minutes. (b) Cy5.5 derivative solubility in different trapping agents (upper bars) and encapsulation efficiency in liposomes containing different trapping agents (lower bars) in an extraliposomal buffer solution containing 10% (292 mM) sucrose, 5 mM HEPES, and 5% DMSO with a D/L ratio of 0.02 at pH 7.4. (c) Size exclusion column photos of the Cy5.5-loaded liposome by using the passive and active loading (ASO as trapping agent) methods presented different encapsulation efficiencies and the circled band indicates the dye-loaded liposome fraction. | |
Preparation of liposomes with different trapping agents
The lipid mixtures consisting of DSPC, cholesterol, and DSPE-PEG2000 at a molar ratio of 45/50/5 (∼17.5 μmoles of total lipids) were dissolved in chloroform in a round-bottomed flask. To form a lipid thin film, the lipid mixtures were dried at 60 °C in a rotatory evaporator and kept under vacuum overnight to remove the residual solvent. The thin film obtained was hydrated with 1 mL of the trapping agents listed in Fig. 1b at 60 °C, followed by 10 freeze–thaw cycles for the formation of unilamellar liposomes. The liposome suspension was extruded through a 100 nm pore-sized polycarbonate membrane at 65 °C using a Mini Extruder (Avanti Polar Lipids) to obtain small unilamellar vesicles. The extraliposomal phase of the liposomes was replaced with a 10% (w/v) sucrose solution (containing 5 mM HEPES, pH 7.4) by a Sepharose CL-4B-packed size-exclusion column (GE Healthcare) to generate a cross-membrane trapping agent gradient. A basified ammonium sucrose octasulfate (ASO, 100 mM) solution was prepared by adjusting the pH to approximately 7.4 with a 6 N NaOH solution. The phospholipid concentration was colorimetrically determined by the Bartlett assay.
Liposomal active loading test of Cy5.5 dyes
For all active loading testing experiments, liposomes (a final lipid concentration of 5 mM) with various trapping agents were co-incubated with Cy5.5 acid or Cy5.5 amine (at a dye-to-lipid molar ratio, D/L, of 0.02) for 40 minutes at 65 °C in an extraliposomal phase solution containing 10% sucrose and 5% DMSO.26,36–38 To purify Cy5.5-loaded liposomes, unencapsulated dyes were removed using a CL-4B-packed column pre-equilibrated with a HEPES buffer solution (50 mM HEPES, 100 mM NaCl, pH 7.4). The liposome solution (0.7–0.8 mM in total lipid concentration) was normally obtained after size exclusion chromatography.
For loading kinetic assays, a solution of Cy5.5 acid or Cy5.5 amine was incubated with the as-prepared ASO liposomes at a D/L ratio of 0.02 and maintained at 65 °C for the required time (5 to 60 minutes). The loading of Cy5.5 was quenched at each time point by placing the liposome–dye mixture on ice for a few minutes and then in the ambient atmosphere. The unencapsulated dyes were removed using a CL-4B-packed column.
For loading capacity tests, the dye solution was incubated with the as-prepared ASO liposomes at different D/L molar ratios ranging from 0.02 to 0.20 at 65 °C for 40 minutes to ensure complete loading, and then the unencapsulated dyes were removed using a CL-4B-packed column. All of these freshly prepared Cy5.5-encapsulated liposomes were stored at 4 °C for further characterization and experiments.
Quantification of Cy5.5-loaded liposomes
The dye content of all Cy5.5-loaded liposomes was quantified by measuring the absorbance at the maximal wavelength of Cy5.5 at 684 nm. Cy5.5-loaded liposomes were first ruptured for complete dye release by adding a 50% (v/v) ethanol/HEPES buffer solution followed by 30 minutes of ultrasonication using a bath-type sonicator. Dilutions of liposome-encapsulated dyes were used as needed to stay within the range of the calibration curve. The Cy5.5 encapsulation efficiency was reported as the percentage of the D/L molar ratio after purification over the D/L molar ratio before purification: |  | (1) |
UV-vis absorption and fluorescence of Cy5.5-loaded liposomes
The absorption and fluorescence spectra of Cy5.5-loaded liposomes before and after rupture were recorded using a Varian Cary 50 UV-vis spectrophotometer and a Varian Cary Eclipse fluorescence spectrometer (Agilent Technologies) with a micro quartz cuvette. To stay within the detection range, all Cy5.5-loaded liposomes were diluted 10-fold with a HEPES solution containing 3% bovine serum albumin (BSA). BSA was added to the buffer to bind and rigidify the structure of the released Cy5.5 dyes for fluorescence enhancement purposes. For intact liposomes, diluted liposome solutions were directly measured by UV-vis and fluorometry. For ruptured liposomes, Triton X-100 was first added to each Cy5.5 liposome solution and heated at 70 °C for 3 minutes to complete dye release. Fluorescence spectra were then recorded from 655 nm to 850 nm, the excitation wavelength was set to 650 nm, and the excitation and emission slit widths were fixed at 5.0 nm with the PMT voltage set to 600 V.
Cell culture and live-cell fluorescence microscopy imaging
Human cervical cancer cells (KB) and human lung adenocarcinoma cells (A549) were purchased from the American Type Culture Collection. KB cells and A549 cells were cultured in Dulbecco's modified Eagle's medium (Gibco) supplemented with 10% heat-inactivated fetal bovine serum (Biological Industries, Beit HaEmek), 100 unit per mL penicillin, and 100 μg mL−1 streptomycin (Gibco) in 100 mm culture dishes. The cell lines were kept at 37 °C in a humidified incubator with 5% CO2 until cells reached 90% confluency for subculturing and animal experiments.
For living cell imaging, the time trace fluorescence of A549 cells treated with Cy5.5-loaded liposomes was visualized using a Confocal Quantitative Image Cytometer CQ1 (Yokogawa Electric Corporation) at an excitation wavelength of 640 nm. A549 cells were seeded into a 96-well culture plate (1 × 104 cells per well) with DMEM overnight before liposome incubation. The cells were treated with both Cy5.5 dye liposomes for 30 minutes at a final lipid concentration of 200 μM, equal to ∼3.6 μM of Cy5.5 dyes, after refreshing DMEM. The cells were washed with PBS and replaced with fresh DMEM after 30 minutes of cellular uptake. The fluorescence of treated cells was measured at different time intervals at 37 °C for up to 24 hours and the fluorescence images were quantified using the CQ1 software (version 1.04.03.01).
In vivo fluorescence imaging of KB xenografted mice
For this experiment, 6- to 8-week-old female BALB/c nude mice (BioLASCO, Taiwan) were raised in a 12-hour light/12-hour darkness cycle with limitless food and water under standard facilities at the Institute of Biomedical Sciences, Academia Sinica, Taiwan. All animal procedures were performed in accordance with the Guidelines for Care and Use of Laboratory Animals of Academia Sinica, Taiwan and approved by the Institutional Animal Care and Use Committee of Academia Sinica (IACUC NO. 19-12-1413). In vivo mouse models were established by subcutaneous injection of KB cells (∼5 × 105 cells/100 μL Matrigel/PBS, 50/50 v/v). The tumor size was estimated using digital calipers, and the body weight was checked twice a week. After the tumor had grown to >500 mm3, Cy5.5 acid liposomes were directly injected (30 μL liposome with a lipid concentration of ∼1 mM and ∼0.5 nmoles Cy5.5 acid) into the intratumor site. Mice were anesthetized with isoflurane (Minrad) using a vaporizer system. An in vivo imaging system (IVIS) for epifluorescence imaging (excitation, 675 nm; emission, 720 nm) was used to monitor the fluorescence enhancement of Cy5.5 acid liposomes at 0, 1, 2, 3, 4, 6, and 24 hours after injection. The fluorescence imaging parameters are as follows: exposure time = 0.5 s, f/stop = 4, medium binning, and field of view = 13.2 cm. The regions of interest (ROI) of tumor locations were circled and evaluated using the Living Image 3.1 software (PerkinElmer).
Results and discussion
Evaluation of trapping agents and preparation of Cy5.5-loaded liposomes via different trapping agent gradients
To develop a release-reporting fluorescent liposome for animal studies, Cy5.5 was selected as a cargo for its NIR emission (λex: 684 nm/λem: 710 nm), which offers deeper tissue penetration for in vivo optical applications. Cy5.5 also provides strong protein binding to retain and accumulate in the tissue site where release occurs.35 Although there have been several publications on fluorescence-quenched liposomes using NIR dyes, the dyes of those liposomes were either passively loaded20 or membrane partitioned.21 Active (remote) loading methods of NIR dyes, if developed, can effectively load a large quantity of dyes into liposomes for deep fluorescence quenching and consequent strong fluorescence enhancement after liposomal release (in terms of both fluorescence fold increase and absolute intensity increase). More importantly, active loading of NIR dyes can significantly lower the cost of NIR dyes by avoiding excessive dye loss. Amphipathic cargo in active loading must contain at least one ionizable functional group to have good solubility (in the exterior water phase), membrane permeability, and interactions with trapping agents in liposomes.26 Therefore, the carboxylic acid and amine derivatives of Cy5.5 were used as cargos in this study. Their pKa and calculated log
D value versus pH value are shown in Fig. S1.† Their log
D values were all positive but not too high in the loading pH (3 to 7), providing optimized lipophilicity and hence membrane permeability without being trapped or anchored on the membrane.36
With an appropriate pKa and log
D of Cy5.5 derivatives, the only remaining critical factor for active loading is the trapping agents. We conducted solubility screening tests aiming to evaluate trapping agents that are capable of retaining Cy5.5 dyes via intraliposomal precipitation. The fully dissolved Cy5.5 dyes were mixed with solutions containing different trapping agents, incubated for 30 minutes and centrifuged. Translucent colorless supernatants indicate that the Cy5.5 dye-trapping agent complex precipitated effectively, as illustrated in Fig. 1a. Both the Cy5.5 dyes are much insoluble in ammonium sucrose octasulfate (ASO) (the most transparent supernatant). Cy5.5 acid was more insoluble in ammonium sulfate, Ca(OAc)2, and La(OAc)3 than Cy5.5 amine in general because Cy5.5 acid contains carboxylic acid that can complex with Ca2+ and La3+ and contains two amines that can complex with sulfate, but Cy5.5 amine can only be complexed with sulfate but not Ca2+ and La3+ (Fig. 1b, upper bars).
Liposomes with cross-membrane trapping agent gradients were used to screen the active loading efficiency of Cy5.5 dyes. As shown in Fig. 1b, lower bars and table, in a gradient-free liposome-entrapped 10% sucrose solution, the encapsulation efficiency (EE%) of Cy5.5 acid and Cy5.5 amine was very low (15.6% and 21.3%, respectively). This indicates that dissolved Cy5.5 dyes can partition partially into the liposome membrane due to their lipophilicity when lacking electrolytes.36,39,40 Ca(OAc)2- and La(OAc)3-encapsulated liposomes were also tested, assuming that metal ions could interact with Cy5.5 dyes, particularly Cy5.5 acid, by forming a stable complex inside liposomes,41 with acetic acid acting as a proton efflux shuttle to create a pH gradient (high inside).34
However, the Cy5.5 acid encapsulation efficiency of Ca(OAc)2- and La(OAc)3-encapsulated liposomes was only approximately 29–37%, while that of Cy5.5 amine was only approximately 3–8%. This low Cy5.5 amine encapsulation (even lower than the gradient-free liposome) can only be explained by the higher solubility of Cy5.5 amine in Ca(OAc)2 and La(OAc)3 than in 10% sucrose solution (Fig. 1b, upper and lower bars). For ammonium sulfate-encapsulated liposomes, the encapsulation efficiency of both dyes increased to 28.5% and 44.4% because the two benzoindolium amines in Cy5.5 dyes can be precipitated by ammonium sulfate. Cy5.5 amine has a better encapsulation efficiency than Cy5.5 acid because sulfate has an extra interaction with the extra auxiliary amine group. ASO solution appeared can significantly precipitate Cy5.5 benzoindolium cations (89.3% and 89.9%) regardless of the auxiliary group, which may be due to the multivalent (eight) sulfate.30 Passive encapsulation of Cy5.5 dyes was also compared to active loading (Fig. 1c). The photos of size exclusion columns in purification confirmed that the encapsulation efficiency of passive and active loading methods differed markedly. In conclusion, the interactions between Cy5.5 benzoindolium cations and multivalent sulfates from ASO are much stronger than ammonium sulfate on dye-retention capacity.33 The interactions between Ca2+/La3+ and Cy5.5 acid are weaker than those between ammonium sulfate and Cy5.5 benzoindolium. These results suggest that Cy5.5 dyes can be successfully actively loaded into liposomes using a transmembrane ASO gradient, the most effective trapping agent in this study.
Characterization of Cy5.5-loaded liposomes via an ammonium sucrose octasulfate gradient
The ASO-assisted Cy5.5 loading method was selected for the rest of the experiments for its high loading efficiency. The loading kinetics of Cy5.5 dyes into ASO-liposomes was studied at a D/L ratio of 0.02 at 65 °C. As shown in Fig. 2a, 80% and 70% EE were achieved within the first 10 minutes for Cy5.5 acid and Cy5.5 amine, respectively, and slowly increased and plateaued at ∼90% after 40 minutes in both cases. These results demonstrated very fast loading kinetics for the Cy5.5 loading, which can be beneficial because Cy5.5 solubility is relatively low, and a lengthy loading time will increase the probability that cargo prematurely precipitates outside liposomes.42
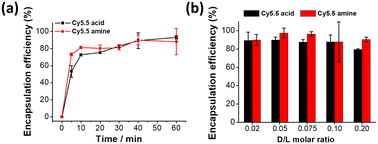 |
| Fig. 2 (a) Loading kinetics of Cy5.5 acid and Cy5.5 amine into liposomes at a drug-to-lipid molar ratio of 0.02. (b) Encapsulation efficiency experiments show that Cy5.5 dyes can be effectively loaded into liposomes from a D/L ratio of 0.02 to 0.20. | |
To further understand the Cy5.5 loading capacity limit of the ASO-liposomes, the D/L molar ratio of Cy5.5 was increased from 0.02 to 0.2, and the incubation time was set to 40 minutes to guarantee complete loading. As shown in Fig. 2b, the encapsulation efficiency of Cy5.5 acid did not change (remained at 90%) as the D/L ratio of dyes increased but slightly decreased to 80% at a D/L ratio of 0.20, whereas the encapsulation efficiency of Cy5.5 amine was always above 90%. The saturated loading capacity (D/L) of both dyes appears to be >0.20, and it is possible to actively load more when using ASO as trapping agents.
To verify whether Cy5.5 dyes can form a stable precipitate with ASO in the intraliposomal phase, cryo-EM was used to visualize the encapsulated Cy5.5 dyes in liposomes. As shown in Fig. 3, amorphous precipitates of both dyes were discovered in most liposomes, and the size of the dye precipitates increased with the increase in the amounts of the encapsulated Cy5.5 dye, indicating the formation of sucrose octasulfate–Cy5.5 complexes. These substantial findings verify the successful encapsulation of Cy5.5 dyes. We noticed that at high D/L ratios, such as 0.20, some populations of Cy5.5 amine-loaded liposomes were hemifused, and some were bilamellar, with aggregated dye deposits between two bilayers.
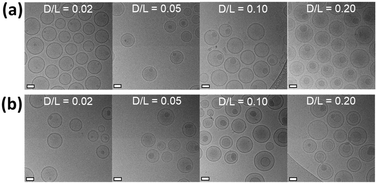 |
| Fig. 3 Cryo-EM images of (a) liposomes active-loaded with Cy5.5 acid and (b) liposomes active-loaded with Cy5.5 amine. Cy5.5 dyes precipitated in liposomes containing 100 mM ASO as a trapping agent clearly observed at D/L ratios from 0.02 to 0.20. Scale bars represent 50 nm. | |
The particle size and zeta potential results for all Cy5.5-loaded liposomes using different trapping agents are listed in Table S1,† and ASO-assisted Cy5.5-loaded liposomes with different loading D/L ratios are listed in Table S2.† There are no noticeable changes in particle size (110–120 nm) and near-neutral zeta potential for most liposomes before and after Cy5.5 dye loading with two exceptions. Ammonium sulfate-assisted Cy5.5 liposomes had a size of 140 nm, perhaps due to their higher osmolarity. Cy5.5 amine-loaded ASO-liposomes with a D/L ratio of 0.20 had a size of 218 nm with PDI (>0.2), which was related to the hemifusion liposome population we observed in this specific loading condition.
To determine how effectively the Cy5.5 dye fluorescence dequenched, the absorption and fluorescence spectra of these Cy5.5 dye-encapsulated liposomes before and after rupture were recorded. The measurements were performed in HEPES buffer solutions containing 3% BSA, and the background spectra were offset. As shown in Fig. 4a and b, the absorbance curves of both Cy5.5-loaded liposomes (D/L 0.02) shifted from a broadband to a distinct peak corresponding to the Cy5.5 monomer when the liposomes were ruptured by Triton X-100. Furthermore, the fluorescence of intact Cy5.5-loaded liposomes was rather low in intensity (deep self-quenching), and the fluorescence was turned on with a 60–100-fold enhancement when the Cy5.5 dyes were released in the presence of BSA. It should be noted that the liposome rupturing methods (Triton X-100 or sonication) did not change the final full fluorescence intensity (Fig. S2†). These changes in absorbance and fluorescence clearly show that ASO-mediated Cy5.5 dye encapsulation and precipitation inside liposomes has deep fluorescence self-quenching. The significant dynamic range of fluorescence fold enhancement and final absolute fluorescence value after release endows Cy5.5-loaded liposomes with a valuable and potential application in animal studies as an advanced contrast agent. Cy5.5 liposomes with higher D/L ratios were also investigated, and their spectra are shown in Fig. S3.† Cyanine dyes are notorious for their poor photostability, yet when encapsulated within liposomes, they could benefit from the liposome encapsulation, possibly due to the trapping agent precipitated Cy5.5 being photo bleaching-resistant. As depicted in Fig. 5, the fluorescence of Cy5.5 acid-loaded liposomes showed almost no photobleaching (remain >90% fluorescence intensity without further bleaching with longer UV irradiation). In contrast to free dye, only 25% fluorescence intensity remains after UV irradiation and bleaching worsens with longer irradiation durations. The stability of both Cy5.5-loaded liposomes in terms of dye release was carefully monitored in various solutions over seven days. As shown in Fig. S4,† the release of all liposomes was very low (<5%) in the initial 12 hours. The release of Cy5.5 acid liposomes was negligible in the HEPES buffer solution containing 3% BSA during 7 days of incubation. However, the release of Cy5.5 acid liposomes gradually increased to 15–35% in FBS and culture media starting from the third day of incubation. However, the release of Cy5.5 amine liposomes was negligible in all the solutions we tested during 7 days of incubation. These results show that both Cy5.5 liposomes can be stably stored for a long time in the HEPES buffer. Cy5.5 acid liposomes have slight release in FBS and culture media after 72 hours, and Cy5.5 amine liposomes can retain Cy5.5 dye for a longer time, possibly due to the extra alkyl amine, so that the interaction between sucrose octasulfate and Cy5.5 amine is stronger than the interactions between sucrose octasulfate and Cy5.5 acid.
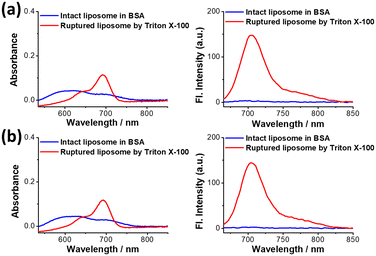 |
| Fig. 4 Absorption and fluorescence spectra of the Cy5.5-liposomes before and after rupture by Triton X-100. (a) Cy5.5 acid-loaded liposomes at a D/L ratio of 0.02 and (b) Cy5.5 amine-loaded liposomes at a D/L ratio of 0.02. | |
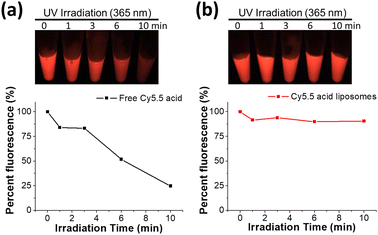 |
| Fig. 5 Photostability was evaluated under 365 nm UV irradiation for up to 10 minutes at a power density of 3 mW cm−2: (a) fluorescence of free Cy5.5 acid and (b) fluorescence of encapsulated Cy5.5 acid liposomes after UV exposure followed by liposome rupture. Fluorescence imaging was performed using a fluorescence in vivo imaging system (FOBI) with 630 nm excitation and a 725 nm long-pass emission filter, with the exposure time set at 200 ms. | |
Fluorescence enhancement after Cy5.5 released by cell internalization
To evaluate and expand the utilities of the deep self-quenched Cy5.5 liposomes, Cy5.5 dye release and dequenching due to liposome cellular uptake and the subsequent degradation were also examined. The lung cancer A549 cells were separately treated with Cy5.5 acid and Cy5.5 amine-loaded liposomes for 30 minutes for liposome cellular uptake, and excessive liposomes were washed off to observe dye release and fluorescence dequenching. Time-course living cell imaging revealed that the fluorescence of A549 cells treated with both Cy5.5-loaded liposomes was dark after 30 minutes for the uptake stage, implying that both Cy5.5-loaded liposomes remained intact without being digested in the intracellular environment (Fig. 6). Following cell internalization, subsequent fluorescence imaging shows growing fluorescence with increasing incubation time, indicating that the encapsulated dyes in liposomes were slowly released, and the released Cy5.5 dyes bound to abundant cellular proteins in the cytosol,35 resulting in significant fluorescence dequenching. The fluorescence enhancement can be observed within the first 3 hours of incubation, and it can increase and last over 24 hours, suggesting that the dye can be retained in the cytosol and is relatively degradation-resistant. The fluorescence release histograms of both Cy5.5-loaded liposomes following cellular uptake revealed an increase in intensity with prolonged incubation time, reaching a saturation point at the 9th hour (refer to Fig. S7†). This enhancement was quantitatively analyzed, as shown in Fig. 6b and c. This experiment demonstrates that Cy5.5 liposomes are effective release-reporting NIR fluorescence contrast agents with a high S/N ratio and are potentially useful in deep tissue bioimaging. This would be beneficial for future smart (trigger responsive) liposome design and development.43,44
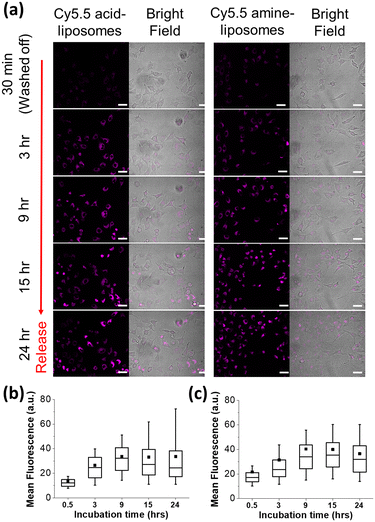 |
| Fig. 6 (a) Time-course of fluorescent cell imaging for self-quenched Cy5.5-loaded liposomes after 30 min incubation, followed by washing off and monitoring for every 6 h until 24 h. (Left) Treated with Cy5.5 acid-loaded liposomes and (right) treated with Cy5.5 amine-loaded liposomes. Scale bars represent 50 μm. Box plot illustrating the quantitative analysis of fluorescence release from (b) Cy5.5 acid-loaded liposomes and (c) Cy5.5 amine-loaded liposomes at different time points following cellular uptake. The whiskers represent the 10th to 90th percentiles, and (■) represents the mean. | |
Release-reporting fluorescence of Cy5.5-liposome in vivo imaging
To examine the fluorescence dequenching effect of Cy5.5-loaded liposomes in small animals, we conducted a time-course fluorescence imaging experiment on tumor-bearing (KB) mice intratumorally injected with Cy5.5 acid liposomes and reported liposomal content release extent by fluorescence (IVIS imaging). As shown in Fig. 7a, there was no detectable fluorescence during the first 2 hours after injection, but the fluorescence started increasing from the 3rd hour and reached the highest intensity (complete release) at 24 hours after injection, with a maximum (24 hours) intensity as bright as the rupture liposome phantom, suggesting that Cy5.5 acid liposomes were slowly released, and the released Cy5.5 dye bound to proteins and accumulated in the tumor over 24 hours. In addition, the protein binding property of Cy5.5 dyes could result in longer dye retention in tissues and intensify the release of signal, while dyes without protein/tissue binding property would be rapidly cleaned by blood circulation.20 As depicted in Fig. S8,† the Cy5.5 fluorescence released from intact liposomes peaked between 24 and 48 hours. Notably, the dye was observed to persist in the tumor region for at least 13 days in our observations, with a half intensity of the maximum. The quantitative analysis of the average radiance of the ROI in the tumor region is shown in Fig. 7b. The average radiance intensity increased by 20-fold from 10 minutes to 24 hours after injection in the tumor region. This fluorescence enhancement in the tumor can be explained by the gradual release observed in the stability experiments and the fluorescence enhancement after cell internalization and degradation of liposomes. Overall, these results demonstrated that the developed highly efficient Cy5.5 active loading method can be used to evaluate liposome release profile in small animals via deep-tissue NIR fluorescence.
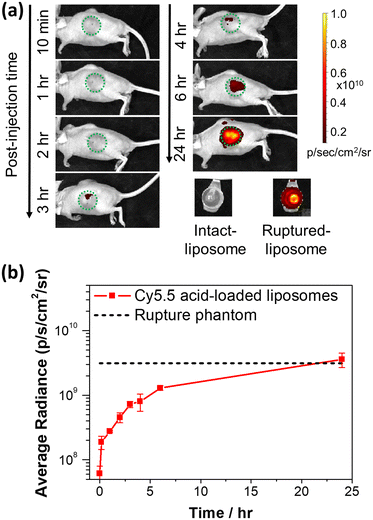 |
| Fig. 7
In vivo fluorescence imaging. (a) Time course of mouse imaging using an IVIS system after intratumor injection of Cy5.5 acid-loaded liposomes into KB tumor-bearing mice. (b) Quantification of the fluorescence of IVIS images from regions and time points of interest after intratumor injection of Cy5.5 acid-loaded liposomes. After injection, the initial radiance ranged from 1.87 × 108 at 10 min to 3.59 × 109 p s−1 cm−2 sr−1 at 24th hour. The Cy5.5 acid concentration of phantoms is ∼2.5 μM. | |
Conclusions
We reported an efficient method to actively load Cy5.5 NIR fluorescent dye derivatives (Cy5.5 acid and Cy5.5 amine) into liposomes. Ammonium sucrose octasulfate is the best trapping agent compared to ammonium sulfate and lanthanum and calcium acetate. The encapsulated Cy5.5 dyes in liposomes were stable for long-term storage in buffer solutions. The active loading method is very efficient and can be used to generate fluorescently deep self-quenched liposomes, which is a very important method to test and report release profiles of designed liposomes by fluorescence. The Cy5.5 dye liposomes showed excellent fluorescence dequenching after cell internalization and liposome degradation in cell and small animals. The developed active loading method allows nanoscientists to utilize the NIR emission and long tissue retention properties of Cy5.5 dyes without high costs. To conclude, we expect that, with the active loading method developed, Cy5.5 dye liposomes will become valuable but affordable tool kits to develop trigger-release or targeted delivery liposome vesicles. This methodology has the potential to be adapted for various high-value NIR-II fluorescent dyes, given the appropriate selection of trapping agents and tailored structural or functional groups of the dye itself. We foresee significant advancements in in vivo imaging applications by integrating this technique with NIR-II fluorescent dyes. Hopefully, the development of trigger-release liposomes that work in animals can be accelerated with the advantages of NIR emission, deep self-quenching, and a high S/N ratio of fluorescence in deep-tissue bioimaging in the future.
Author contributions
Conceptualization: C.-Y. C., C.-B. J., J.-J. S., and H.-M. L.; investigation: C.-Y. C., and C.-B. J.; methodology: H.-D. G., X.-E. Y., Y.-C. C., and S. K. L.; validation: C.-Y. C., and C.-B. J.; visualization: C.-Y. C.; writing – original draft preparation: C.-Y. C., and C.-B. J.; writing – review and editing: J.-J. S., and H.-M. L.; supervision and project administration: J.-J. S., and H.-M. L. All authors have read and agreed to the published version of the manuscript.
Conflicts of interest
The authors declare no competing financial interest.
Acknowledgements
This research was funded by Innovative Materials and Analysis Technology Exploration Program of Academia Sinica (grant number: As-Imate-109-21) and The Ministry of Science and Technology of Taiwan (grant number: 111-2113-M-001-005-). The cryo-EM experiments were performed at ASCEM. ASCEM is jointly supported by Academia Sinica Core Facility and Innovative Instrument Project (grant No. AS-CFII-108-110) and Taiwan Protein Project (grant no. AS-KPQ-109-TPP2). We thank the Animal Center at the Institute of Biomedical Sciences, Academia Sinica for their animal care. We also thank the Instrument Core Facilities at the Genomic Research Center, Academia Sinica for the IVIS imaging support.
References
- T. M. Allen and P. R. Cullis, Liposomal drug delivery systems: From concept to clinical applications, Adv. Drug Delivery Rev., 2013, 65(1), 36–48 CrossRef CAS PubMed.
- T. O. B. Olusanya, R. R. Haj Ahmad, D. M. Ibegbu, J. R. Smith and A. A. Elkordy, Liposomal Drug Delivery Systems and Anticancer Drugs, Molecules, 2018, 23(4), 907 CrossRef PubMed.
- M. Slingerland, H. J. Guchelaar and H. Gelderblom, Liposomal drug formulations in cancer therapy: 15 years along the road, Drug Discovery Today, 2012, 17(3–4), 160–166 CrossRef CAS PubMed.
- A. Cabanes, D. Tzemach, D. Goren, A. T. Horowitz and A. Gabizon, Comparative study of the antitumor activity of free doxorubicin and polyethylene glycol-coated liposomal doxorubicin in a mouse lymphoma model, Clin. Cancer Res., 1998, 4(2), 499–505 CAS.
- K. M. Laginha, S. Verwoert, G. J. Charrois and T. M. Allen, Determination of doxorubicin levels in whole tumor and tumor nuclei in murine breast cancer tumors, Clin. Cancer Res., 2005, 11(19 Pt 1), 6944–6949 CrossRef CAS PubMed.
- M. E. O'Brien, N. Wigler, M. Inbar, R. Rosso, E. Grischke, A. Santoro, R. Catane, D. G. Kieback, P. Tomczak, S. P. Ackland, F. Orlandi, L. Mellars, L. Alland, C. Tendler and Group C. B. C. S., Reduced cardiotoxicity and comparable efficacy in a phase III trial of pegylated liposomal doxorubicin HCl (CAELYX/Doxil) versus conventional doxorubicin for first-line treatment of metastatic breast cancer, Ann. Oncol., 2004, 15(3), 440–449 CrossRef PubMed.
- W. S. Chen, Q. Y. Huang, W. Z. Ou, Y. Q. Hao, L. Q. Wang, K. Zeng, H. Y. Guo, J. Li and Y. N. Liu, Self-Reporting Liposomes for Intracellular Drug Release, Small, 2014, 10(7), 1261–1265 CrossRef CAS.
- T. J. Evjen, E. Hagtvet, A. Moussatov, S. Rognvaldsson, J. L. Mestas, R. A. Fowler, C. Lafon and E. A. Nilssen, In vivo monitoring of liposomal release in tumours following ultrasound stimulation, Eur. J. Pharm. Biopharm., 2013, 84(3), 526–531 CrossRef CAS PubMed.
- Y. Xia, C. Xu, X. Zhang, J. Gao, Y. Wu, C. Li and Z. Wang, An activatable liposomal fluorescence probe based on fluorescence resonance energy transfer and aggregation induced emission effect for sensitive tumor imaging, Colloids Surf., B, 2020, 188, 110789 CrossRef CAS PubMed.
- U. Chitgupi, S. Shao, K. A. Carter, W. C. Huang and J. F. Lovell, Multicolor Liposome Mixtures for Selective and Selectable Cargo Release, Nano Lett., 2018, 18(2), 1331–1336 CrossRef CAS PubMed.
- J. N. Weinstein, S. Yoshikami, P. Henkart, R. Blumenthal and W. A. Hagins, Liposome-cell interaction: transfer and intracellular release of a trapped fluorescent marker, Science, 1977, 195(4277), 489–492 CrossRef CAS PubMed.
- Y. Xia, C. Xu, X. Zhang, P. Ning, Z. Wang, J. Tian and X. Chen, Liposome-based probes for molecular imaging: from basic research to the bedside, Nanoscale, 2019, 11(13), 5822–5838 RSC.
- R. F. Chen and J. R. Knutson, Mechanism of fluorescence concentration quenching of carboxyfluorescein in liposomes: energy transfer to nonfluorescent dimers, Anal. Biochem., 1988, 172(1), 61–77 CrossRef CAS PubMed.
- J. F. Lovell, C. S. Jin, E. Huynh, H. L. Jin, C. Kim, J. L. Rubinstein, W. C. W. Chan, W. G. Cao, L. V. Wang and G. Zheng, Porphysome nanovesicles generated by porphyrin bilayers for use as multimodal biophotonic contrast agents, Nat. Mater., 2011, 10(4), 324–332 CrossRef CAS PubMed.
- H. Xu, T. Y. Ohulchanskyy, J. Qu, A. Yakovliev, R. Ziniuk, Z. Yuan and J. Qu, Co-encapsulating indocyanine green and CT contrast agent within nanoliposomes for trimodal imaging and near infrared phototherapy of cancer, Nanomedicine, 2020, 29, 102269 CrossRef CAS PubMed.
- Y. Wang, D. Liu, M. You, H. Yang and H. Ke, Liposomal cyanine dyes with enhanced nonradiative transition for the synergistic phototherapy of tumors, J. Mater. Chem. B, 2022, 10(16), 3016–3022 RSC.
- K. Kono, M. Takashima, E. Yuba, A. Harada, Y. Hiramatsu, H. Kitagawa, T. Otani, K. Maruyama and S. Aoshima, Multifunctional liposomes having target specificity, temperature-triggered release, and near-infrared fluorescence imaging for tumor-specific chemotherapy, J. Controlled Release, 2015, 216, 69–77 CrossRef CAS PubMed.
- G. S. Hong, A. L. Antaris and H. J. Dai, Near-infrared fluorophores for biomedical imaging, Nat. Biomed. Eng., 2017, 1(1), 0010 CrossRef CAS.
- M. H. Y. Cheng, K. M. Harmatys, D. M. Charron, J. Chen and G. Zheng, Stable J-Aggregation of an aza-BODIPY-Lipid in a Liposome for Optical Cancer Imaging, Angew. Chem., Int. Ed., 2019, 58(38), 13394–13399 CrossRef CAS PubMed.
- F. L. Tansi, R. Ruger, M. Rabenhold, F. Steiniger, A. Fahr, W. A. Kaiser and I. Hilger, Liposomal encapsulation of a near-infrared fluorophore enhances fluorescence quenching and reliable whole body optical imaging upon activation in vivo, Small, 2013, 9(21), 3659–3669 CrossRef CAS PubMed.
- Y. Xia, C. Xu, K. Wu, J. Wang, C. Cui, Y. Wu, C. Li and X. Zhang, Self-quenched liposomal probes for tumor imaging based on cellular on/off system, Mater. Today Commun., 2022, 30, 103207 CrossRef CAS.
- J. Gubernator, Active methods of drug loading into liposomes: recent strategies for stable drug entrapment and increased in vivo activity, Expert Opin. Drug Delivery, 2011, 8(5), 565–580 CrossRef CAS PubMed.
- C. B. Jian, X. E. Yu, H. D. Gao, H. A. Chen, R. H. Jheng, C. Y. Chen and H. M. Lee, Liposomal PHD2 Inhibitors and the Enhanced Efficacy in Stabilizing HIF-1alpha, Nanomaterials, 2022, 12(1), 163 CrossRef CAS PubMed.
- S. Sur, A. C. Fries, K. W. Kinzler, S. Zhou and B. Vogelstein, Remote loading of preencapsulated drugs into stealth liposomes, Proc. Natl. Acad. Sci. U. S. A., 2014, 111(6), 2283–2288 CrossRef CAS PubMed.
- D. Zucker, D. Marcus, Y. Barenholz and A. Goldblum, Liposome drugs' loading efficiency: a working model based on loading conditions and drug's physicochemical properties, J. Controlled Release, 2009, 139(1), 73–80 CrossRef CAS PubMed.
- G. Pauli, W. L. Tang and S. D. Li, Development and Characterization of the Solvent-Assisted Active Loading Technology (SALT) for Liposomal Loading of Poorly Water-Soluble Compounds, Pharmaceutics, 2019, 11(9), 465 CrossRef CAS PubMed.
- K. Xiao, Y. Zhao, J. Zhu and L. Zhao, Hyperconjugative aromaticity and protodeauration reactivity of polyaurated indoliums, Nat. Commun., 2019, 10(1), 5639 CrossRef CAS PubMed.
- Y. Barenholz, Liposome application: problems and prospects, Curr. Opin. Colloid Interface Sci., 2001, 6(1), 66–77 CrossRef CAS.
- G. Haran, R. Cohen, L. K. Bar and Y. Barenholz, Transmembrane ammonium sulfate gradients in liposomes produce efficient and stable entrapment of amphipathic weak bases, Biochim. Biophys. Acta, 1993, 1151(2), 201–215 CrossRef CAS PubMed.
- D. C. Drummond, C. O. Noble, Z. Guo, M. E. Hayes, J. W. Park, C. J. Ou, Y. L. Tseng, K. Hong and D. B. Kirpotin, Improved pharmacokinetics and efficacy of a highly stable nanoliposomal vinorelbine, J. Pharmacol. Exp. Ther., 2009, 328(1), 321–330 CrossRef CAS PubMed.
- D. C. Drummond, C. O. Noble, Z. Guo, K. Hong, J. W. Park and D. B. Kirpotin, Development of a highly active nanoliposomal irinotecan using a novel intraliposomal stabilization strategy, Cancer Res., 2006, 66(6), 3271–3277 CrossRef CAS PubMed.
- J. Liu, D. Chi, S. Pan, L. Zhao, X. Wang, D. Wang and Y. Wang, Effective co-encapsulation of doxorubicin and irinotecan for synergistic therapy using liposomes prepared with triethylammonium sucrose octasulfate as drug trapping agent, Int. J. Pharm., 2019, 557, 264–272 CrossRef CAS PubMed.
- W. Yang, Z. Yang, J. Fu, M. Guo, B. Sun, W. Wei, D. Liu and H. Liu, The influence of trapping agents on the antitumor efficacy of irinotecan liposomes: head-to-head comparison of ammonium sulfate, sulfobutylether-beta-cyclodextrin and sucrose octasulfate, Biomater. Sci., 2018, 7(1), 419–428 RSC.
- S. Clerc and Y. Barenholz, Loading of amphipathic weak acids into liposomes in response to transmembrane calcium acetate gradients, Biochim. Biophys. Acta, Biomembr., 1995, 1240(2), 257–265 CrossRef PubMed.
- A. Hansch, O. Frey, I. Hilger, D. Sauner, M. Haas, D. Schmidt, C. Kurrat, M. Gajda, A. Malich, R. Brauer and W. A. Kaiser, Diagnosis of arthritis using near-infrared fluorochrome Cy5.5, Invest. Radiol., 2004, 39(10), 626–632 CrossRef CAS PubMed.
- W. L. Tang, W. C. Chen, A. Roy, E. Undzys and S. D. Li, A Simple and Improved Active Loading Method to Efficiently Encapsulate Staurosporine into Lipid-Based Nanoparticles for Enhanced Therapy of Multidrug Resistant Cancer, Pharm. Res., 2016, 33(5), 1104–1114 CrossRef CAS PubMed.
- W. L. Tang, W. H. Tang, W. C. Chen, C. Diako, C. F. Ross and S. D. Li, Development of a Rapidly Dissolvable Oral Pediatric Formulation for Mefloquine Using Liposomes, Mol. Pharmaceutics, 2017, 14(6), 1969–1979 CrossRef CAS PubMed.
- W. L. Tang, W. H. Tang, A. Szeitz, J. Kulkarni, P. Cullis and S. D. Li, Systemic study of solvent-assisted active loading of gambogic acid into liposomes and its formulation optimization for improved delivery, Biomaterials, 2018, 166, 13–26 CrossRef CAS PubMed.
- A. Fahr, P. van Hoogevest, S. May, N. Bergstrand and M. L. Leigh, Transfer of lipophilic drugs between liposomal membranes and biological interfaces: consequences for drug delivery, Eur. J. Pharm. Sci., 2005, 26(3–4), 251–265 CrossRef CAS PubMed.
- X. Liu, B. Testa and A. Fahr, Lipophilicity and its relationship with passive drug permeation, Pharm. Res., 2011, 28(5), 962–977 CrossRef CAS PubMed.
- J. Yu, S. Zhou, J. B. Li, Y. L. Wang, Y. J. Su, D. X. Chi, J. M. Wang, X. Wang, Z. G. He, G. M. Lin, D. Liu and Y. J. Wang, Simple weak-acid derivatives of paclitaxel for remote loading into liposomes and improved therapeutic effects, RSC Adv., 2020, 10(46), 27676–27687 RSC.
- W. L. Zhang, G. J. Wang, J. R. Falconer, B. C. Baguley, J. P. Shaw, J. P. Liu, H. T. Xu, E. See, J. G. Sun, J. Y. Aa and Z. M. Wu, Strategies to Maximize Liposomal Drug Loading for a Poorly Water-soluble Anticancer Drug, Pharm. Res., 2015, 32(4), 1451–1461 CrossRef CAS PubMed.
- M. Ashrafizadeh, M. Delfi, A. Zarrabi, A. Bigham, E. Sharifi, N. Rabiee, A. C. Paiva-Santos, A. P. Kumar, S. C. Tan, K. Hushmandi, J. Ren, E. N. Zare and P. Makvandi, Stimuli-responsive liposomal nanoformulations in cancer therapy: Pre-clinical & clinical approaches, J. Controlled Release, 2022, 351, 50–80 CrossRef CAS PubMed.
- J. Nel, K. Elkhoury, É. Velot, A. Bianchi, S. Acherar, G. Francius, A. Tamayol, S. Grandemange and E. Arab-Tehrany, Functionalized liposomes for targeted breast cancer drug delivery, Bioact. Mater., 2023, 24, 401–437 CAS.
Footnotes |
† Electronic supplementary information (ESI) available: Cy5.5 amine synthesis routine and details; additional experimental details regarding particle size and zeta potential, cryo-EM imaging, and stability test of Cy5.5 liposomes; log D profile of Cy5.5 dyes; absorption and fluorescence spectra of Cy5.5-loaded liposomes; 1H and 13C-NMR spectra of Cy5.5 amine. See DOI: https://doi.org/10.1039/d3sd00325f |
‡ These authors contributed equally to this work. |
|
This journal is © The Royal Society of Chemistry 2024 |