DOI:
10.1039/D4SC04519J
(Edge Article)
Chem. Sci., 2024, Advance Article
Ambient catalyst-free oxidation reactions of aromatic amines using water radical cations†
Received
8th July 2024
, Accepted 5th September 2024
First published on 6th September 2024
Abstract
Water radical cations play a pivotal role in various scientific and industrial fields due to their unique reactivity and capacity to drive complex chemical transformations. Here we explored the formation of quaternary ammonium cations through the direct oxidation reaction of aromatic amines, facilitated by water radical cations within water microdroplets. This process was monitored via in situ mass spectrometry and occurs under ambient conditions, negating the need for traditional chemical catalysts or oxidants and achieving an impressive yield of approximately 80%. Additionally, we employed a multi-channel spray system and enhanced both the reactant concentration and flow rate, thereby enabling gram-scale synthesis. These findings not only demonstrate the effectiveness and eco-friendliness of microdroplet chemistry but also provide a new understanding of heterogeneous ˙OH generation channels, thereby boosting the synthetic efficiency and sustainability of chemical processes.
Introduction
The oxidation of organic compounds is an essential reaction widely studied in academia as well as in the chemical industry.1,2 Despite numerous research efforts, the selective oxidation of organic substrates remains a critical challenge. It would be of great value to be able to hydroxylate aromatic amines in a regio-controlled manner under mild conditions, using inexpensive oxidants and avoiding the use of transition metals, thereby preparing synthetically significant structures.3 There are few reported strategies for the synthesis of amine N-oxides4,5 and quaternary ammonium cations.6,7 Quaternization, forming the quaternary ammonium cation, prevents inversion and locks the configuration of the nitrogen stereocenter. The synthesis of quaternary ammonium compounds is of great significance for drug development. Therefore, developing a general strategy for constructing quaternary ammonium compounds has always been a major challenge in synthetic chemistry.
Mass spectrometry (MS) has been widely applied to the study of chemical reactions in recent years, enabling the in situ capture of short-lived intermediates during reactions.8–10 In particular, the emergence of microdroplet chemistry in MS has garnered much attention due to the “catalytic” role of the interface in accelerating reactions, making it a unique platform for investigating and conducting chemical reactions.11–13 Recently, it has been discovered that simple water microdroplets can significantly accelerate the rate of organic reactions.14,15 For instance, Lee et al. reported spontaneous oxidation and reduction reactions in microdroplets without the addition of reducing or oxidizing agents, where the reactive species involved are the hydroxyl radical and hydrogen peroxide.16 Gong et al. hypothesized that electrons at the microdroplets' air–water interface are responsible for the reduction of ethyl viologen.17 Cooks and colleagues suggested that water radical cations, generated in organic-solvent microdroplets containing trace amounts of water, play a key role in the oxidation of aromatic sulfones to sulfonic acids.18,19 Our group has also demonstrated the strong reactivity of (H2O)2+˙, which can transform C
C bonds into epoxides20,21 and C
O bonds to generate [M + H2O]+˙.22 Most recently, we discovered that (H2O)2+˙ can even convert nitrogen (N2), a highly inert molecule, into NH2OH and HNO.23 The diverse chemistry of (H2O)2+˙ has been attributed to the co-existence of two distinct structures: [H3O+⋯˙OH] and [H2O⋯OH2]+˙,24 which can exhibit the properties of ˙OH, H3O+, and H2O+˙.
The electrochemical oxidation of N,N-dimethylaniline and its derivatives has been studied extensively.25,26 A common feature in all cases is that the oxidation of aniline begins with the nitrogen atom losing one electron to yield the radical cation.25,26 From this point, the oxidation mechanism varies depending on the level of substitution at the nitrogen atom and the basicity of the reaction medium. Yoshida and co-workers reported a highly chemoselective metal-free method for the aryl C–H amination of aromatic compounds with pyridine and imidazole, resulting in the formation of N-arylpyridinium and N-arylimidazolium ions, respectively, through electrochemical oxidation.27,28 Functional groups such as N–O bonds are crucial for biological functions. In this work, we report the synthesis of quaternary ammonium cations through the direct N-hydroxylation of aromatic amine in water microdroplets, notably without the need for applying an additional external voltage to the sample solution. The quaternary ammonium product was characterized by MS and nuclear magnetic resonance (NMR) experiments, demonstrating good universality in the reaction.
Experimental procedures
Chemicals and materials
D2O was purchased from Cambridge Isotope Laboratories, Inc. (Andover, MA, USA). Ultra-purity Ar (>99.999%) and ultra-purity helium (>99.999%) were obtained from Jiangxi Guoteng Gas Co. Ltd (Nanchang, China). N,N-Dimethylaniline (DMA, 1), 4-methoxyl-N,N-dimethylaniline (4-MeO-DMA, 2), 4-methyl-N,N-dimethylaniline (4-Me-DMA, 3), 4-chloro-N,N-dimethylaniline (4-Cl-DMA, 4), 4-bromo-N,N-dimethylaniline (4-Br-DMA, 5), N,N-diethylaniline (DTA, 6), 3-hydroxy-N,N-dimethylaniline (3-OH-DMA, 7), 2-hydroxy-N,N-dimethylaniline (2-OH-DMA), and 4-hydroxy-N,N-dimethylaniline (4-OH-DMA) were purchased from Shanghai Sun Chemical Technology Co., Ltd (China), each with a purity of over 99%. N,N-Dimethylaniline N-oxide was purchased from Shanghai Laibo Chemical Technology Co., Ltd (China), also with a purity exceeding 99%. Water used in all experiments was purified using a Milli-Q system (Millipore, USA).
Experimental setup
The experimental setup to study the N-hydroxylation reaction between DMA and (H2O)2+˙ in water microdroplets is depicted in Fig. 1a. Argon gas was introduced at a flow rate between 0.3 and 1.0 MPa. The produced H2O/DMA solution was directed to a fused silica capillary situated inside a larger capillary through which argon gas flows at high pressure. The distance from the inlet of the mass spectrometer capillary to the tip of the ion source ranged from 5 to 20 mm.
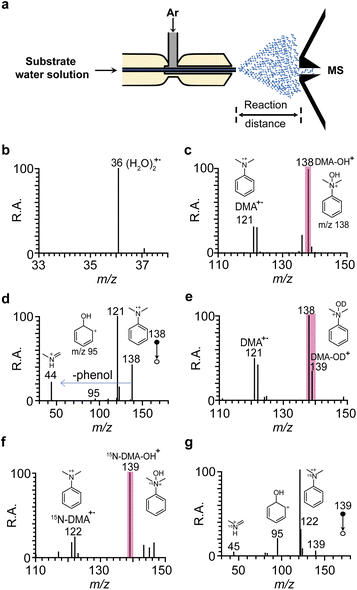 |
| Fig. 1 Mass spectrometric analysis of the spontaneous hydroxylation reaction between DMA and (H2O)2+˙ in water microdroplets. (a) A schematic drawing of the experimental setup. (b) A typical mass spectrum showing the generation of (H2O)2+˙ at m/z 36 when spraying pure water. (c) A typical mass spectrum demonstrating the spontaneous generation of DMA+˙ at m/z 121 and DMA–OH+ at m/z 138 when spraying a DMA–water solution. (d) A tandem mass spectrum of the product ion at m/z 138. (e) A typical mass spectrum illustrating the spontaneous generation of DMA+˙ at m/z 121 and the DMA–OD+ product at m/z 139 when spraying a D2O solution with DMA. (f) A typical mass spectrum illustrating the spontaneous generation of 15N-DMA+˙ at m/z 122 and the 15N-DMA–OH+ product at m/z 139 when spraying 15N-DMA solution. (g) A tandem mass spectrum of the product ion at m/z 139 in Fig. 1f. DMA : N,N-dimethylaniline. | |
MS settings
MS detection was performed using an LTQ-XL ion trap mass spectrometer (LTQ-XL, Thermo Scientific, San Jose, CA, USA). The temperature of the ion transfer capillary was set at 250 °C. The capillary voltage was 20 V, and the tube lens voltage was set at 30.0 V. The pressure in the ion trap was maintained at 1 × 10−5 torr. High-purity helium (99.999%) was used as the collision gas. Collision-induced dissociation (CID)-MS experiments were conducted by applying an alternating current excitation voltage to the end caps of the ion trap to induce collisions of the isolated ions. CID-MS spectra were obtained by activating the precursor ions at a normalized collision energy ranging from 0% to 50%. Ion detection was conducted in the positive ion mode. Other LTQ-XL parameters were automatically optimized by the system.
High-resolution MS experiments were conducted using an Orbitrap-XL mass spectrometer (Thermo Scientific, San Jose, CA, USA) equipped with a homemade ESI setup. The instrument operated at a resolution of up to 300
000. Mass spectra were averaged over 30 scans during a sampling period of 0.5 minutes, covering a mass-to-charge (m/z) range of 15 to 200. Other Orbitrap-XL parameters were consistent with the LTQ-XL parameters previously mentioned.
Yield calculation
The method for yield calculation in this work was derived from the rough estimates of the yields of microdroplet synthesis reactions reported by the Cooks group and Zare group.29,30 This was obtained by measuring the conversion ratio: the ratio of the intensity of the product (P) to the sum of the intensities of the reactant (R) and product (P), e.g., [P]/([R] + [P]).
Scale-up experiments
Synthesis of DMA–OH was carried out through the scale-up microdroplet reaction of DMA aqueous solution. First, a capillary tube with an inner diameter of 150 μm was placed in a nebulizer with an argon pressure of 0.4 MPa. A DMA aqueous solution with a concentration of 1000 μg mL−1 was placed in a syringe and pumped into the capillary tube at a rate of 20 μL min−1 to form microdroplets. These droplets were sprayed into a three-necked flask with a condenser (Fig. S1a†). An electrode was placed at one end of the flask to neutralize excess positive charge, while the other end was vented. In the venting section, ethyl acetate was used to absorb the product that may be contained in the carrier gas. The droplets were collected continuously for 10 h, combined with the contents of the flask and ethyl acetate absorption solution, and then evaporated to obtain yellow oil crude products. The crude product was further purified by thin layer chromatography using petroleum ether and ethyl acetate as eluents. The purified target product was obtained and characterized by MS and NMR.
Synthesis of 15N-labeled DMA
An efficient synthesis of 15N-labeled DMA, based on ref. 31, was conducted using 15NH4Cl and benzoyl chloride as starting materials. The synthesis involves the following steps: first, 15NH4Cl was reacted with benzoyl chloride to provide 15N-benzamide. Next, 15N-benzamide underwent Hofmann degradation to produce 15N-aniline. Finally, 15N-aniline was methylated using methyl 4-methylbenzenesulfonate to form 15N-DMA. The product, 15N-DMA, was also subjected to a scale-up experiment to confirm the structure of the hydroxylation product of DMA.
Density functional theory (DFT) calculations
Theoretical calculations were performed using the Dmol3 software package based on the linear combination of atomic orbitals method. Electron–ion interactions were described using all electron potentials. A double numerical polarized (DNP) basis set was employed to expand the wave functions with an orbital cutoff of 3.7 Å. For the electron–electron exchange and correlation interactions, the B3LYP functional was used throughout. The van der Waals interaction was described using the DFT-D2 method proposed by Grimme. During the geometry optimizations, all the atoms were allowed to relax. In this work, the convergence criterion for the electronic self-consistent field (SCF) loop was set to 10−6. The atomic structures were optimized until the residual forces were below 0.002 Ha Å−1.
Results and discussion
Hydroxyl quaternization of DMA
Fig. 1a illustrates the experimental setup for the microdroplet reaction. Briefly, substrate water solutions are propelled through a fused silica capillary using a syringe pump; this capillary is nested within a larger coaxial capillary through which high-pressure argon sheath gas flows. Fig. 1b presents a typical mass spectrum that demonstrates the spontaneous generation of the water dimer radical cation (H2O)2+˙ at m/z 36 when pure water is sprayed to form microdroplets, a phenomenon also reported by Xing et al.32 They observed the presence of (H2O)2+˙ at the air–liquid interface, which is essentially [˙OH⋯H3O+], where a hydroxyl radical is combined with a hydronium cation via hydrogen bonding. Our group has also reported the generation of (H2O)2+˙ using energy-tunable corona discharge ionization mass spectrometry, demonstrating its excellent reactivity.33 One form of (H2O)2+˙ (m/z 36), [˙OH⋯H3O+], acts as a carrier of the hydroxyl radical, facilitating numerous reactions.33,34
Initially, we explored the idea of extracting electrons from the substrates and further hydroxylation using microdroplets, selecting DMA (1) for mechanistic studies and optimization of reaction conditions. Fig. 1c displays a typical mass spectrum, highlighting the observation of the spontaneous hydroxylation product at m/z 138 (base peak) when spraying a DMA–water solution, which indicates that the reaction of DMA with ˙OH in (H2O)2+˙ may occur. Parent DMA+˙ radical cations are also present but with lower intensity. High-resolution mass spectrum data revealed a peak for DMA+˙ + ˙OH at m/z 138.09104 (error of −2.1 ppm), further confirming the hydroxylation product of DMA (Fig. S2†). The tandem MS spectrum of the hydroxylation product (m/z 138) yielded fragment ions at m/z 121, m/z 95 and m/z 44 (Fig. 1d), corresponding to the loss of ˙OH, CH3N
CH2, and phenol, respectively, consistent with its structural assignment (Fig. S3†). However, it remains uncertain whether the ˙OH is attached to the ortho- or para-position to form a phenol structure or connected to the nitrogen atom of DMA to form a quaternary ammonium structure.
To further determine the position of the ˙OH interaction with the DMA structure at m/z 138, tandem MS experiments for isomers N,N-dimethylaniline N-oxide, 2-OH-DMA, 3-OH-DMA, and 4-OH-DMA standards were conducted. The tandem MS spectrum of protonated N,N-dimethylaniline N-oxide displayed the same fragment ions at m/z 44, m/z 95 and m/z 121 (Fig. S4†) as those observed in the tandem MS spectrum of m/z 138 generated by the spraying of DMA solution, with consistent intensities. However, the tandem MS spectra of protonated 2-OH-DMA, protonated 3-OH-DMA, and protonated 4-OH-DMA showed major fragmentation ions at m/z 123, which differed from those of m/z 138 generated by spraying the DMA solution (Fig. S5†). These results confirmed that the structure of m/z 138 obtained from spraying of DMA solution corresponds to N-hydroxyl-N,N-dimethylanilinium, a quaternary ammonium structure. It was reported that N,N-dimethylaniline N-oxide and N-hydroxyl-N,N-dimethylanilinium can easily interconvert.5 Thus, the product at m/z 138 generated by the spraying of DMA solution is N-hydroxyl-N,N-dimethylanilinium. The formation process of fragment ions at m/z 121, m/z 95 and m/z 44 from the dissociation of protonated N,N-dimethylaniline N-oxide is clearly described in detail (Fig. S3†), and involves the OH transfer reaction. This is similar to the reactions reported in previous studies.35
When isotope-labeled D2O or 15N-DMA was used in the experiments, the corresponding deuterium labeled ions at m/z 139 (Fig. 1e) and 15N-labeled ions at m/z 139 (Fig. 1f) were both clearly observed. The dissociation of the m/z 139 ions from D2O and 15N-DMA displayed similar fragmentation patterns to those of m/z 138, with characteristic fragment ions at m/z 44, m/z 96, and m/z 121 (Fig. S6† and 1g). Consequently, the results of high-resolution MS experiments, tandem MS experiments of different standards for OH-DMA isomers, and isotope-labeling experiments all supported the hypothesis that the ion generated at m/z 138 is a quaternary structure known as N-hydroxyl-N,N-dimethylanilinium during the spraying of the DMA–water solution. This indicates that a large amount of quaternization products can obtained simply by spraying of the DMA–water solution.
We conducted additional experiments using methanol, acetonitrile, and their mixed solutions with water to determine if similar phenomena and products could be observed. The effects of different solvents on the intensity of hydroxylation products are shown in Fig. S7.† Our results showed that no hydroxylation products were detected in methanol and acetonitrile solvents (Fig. S7†). However, hydroxylation products were observed in their mixed solutions with water, with the highest intensity observed when pure water was used as the solvent (Fig. S7†). This indicates that water plays a crucial role in promoting the reaction, likely due to its ability to facilitate the formation of water radical cations, which subsequently lead to hydroxylation products.
Optimization of conditions for hydroxylation of DMA
DMA was chosen as the model substrate for optimizing reaction conditions. We explored the experimental conditions affecting the yields of N-hydroxylation of DMA and found that gas pressure was a significant factor (Fig. 2a). Higher gas pressure facilitates the formation of smaller droplets, enhancing the electric field intensity on the surface of the droplets. This finding is consistent with a previous report by Xing et al.32 When the strength of the electric field exceeds the oxidation potential of the substrate, an electron is extracted from the substrate. However, when the argon pressure is increased beyond a certain point, the pressure at the spray outlet drops sharply. This disturbance affects the surrounding airflow, preventing the target substance from entering the mass spectrometer efficiently and resulting in decreased yields of the product ions.
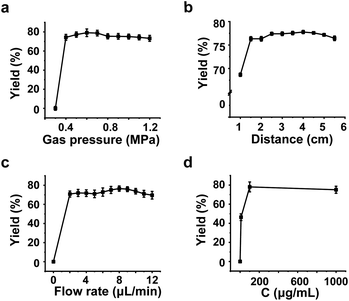 |
| Fig. 2 Effects of microdroplet working conditions on the yields of hydroxylation products of N,N-dimethylaniline (DMA) at m/z 138. (a) Yields as a function of gas pressure. (b) Yields as a function of the distance between the tip of the capillary tube and the MS inlet. (c) Yields as a function of the flow rate of the aqueous DMA solution. (d) Yields as a function of the concentration of the aqueous DMA solution. C: concentration. | |
We also examined the effects of the concentration of the aqueous DMA solution, the flow rate of the aqueous DMA solution, and the distance between the tip of the capillary tube and the MS inlet on the hydroxylation reaction. For the reaction distance, a noticeable increase in the yields of products at m/z 138 was observed as the distance increased from 1 to 6 cm (Fig. 2b), indicating that the reaction occurred within the microdroplets rather than in the gas phase inside the mass spectrometer. Similarly, when the sample flow rate was optimized to 8 μL min−1 (Fig. 2c), the products exhibited the highest yield. This may be because, at a fixed pressure, excessively increasing the flow rate results in the formation of larger droplets or even incomplete nebulization, thereby reducing the target ion signal. The yields of the products increased with the sample concentration and eventually stabilized (Fig. 2d). Considering that excessive sample concentration could impact the mass spectrometer, 100 μg mL−1 was ultimately selected for subsequent experiments.
Substrate scope for hydroxyl quaternization
Next, we evaluated the substrate scope for relatively electron-rich aromatics, encouraged by the accelerated hydroxylation of DMA in the water microdroplet. Hydroxylation reactions were found to be compatible with various 4-substituted functional groups on DMA, such as electron-donating groups like –OCH3 (4-MeO-DMA, 2) and –CH3 (4-Me-DMA, 3), as well as electron-withdrawing groups like –Cl (4-Cl-DMA, 4) and –Br (4-Br-DMA, 5). Other diverse functional compounds tested included DTA (6) and 3-OH-DMA (7). In addition to the reaction studied between DMA and ˙OH in water microdroplets shown in Fig. 1, we investigated six other hydroxylation reaction systems involving 4-MeO-DMA, 4-Me-DMA, 4-Cl-DMA, 4-Br-DMA, 3-OH-DMA, and DTA. Mass spectra supported the occurrence of these reactions (Fig. 3). Hydroxylation reaction products with different functional compounds—3-OH-DMA (m/z 154 in Fig. 3a), 4-Me-DMA (m/z 152 in Fig. 3b), 4-MeO-DMA (m/z 168 in Fig. 3c), DTA (m/z 166 in Fig. 3d), 4-Cl-DMA (m/z 172 in Fig. 3e), and 4-Br-DMA (m/z 216 in Fig. 3f)—were clearly observed in the corresponding mass spectra. Generally, electron-donating groups facilitate the formation of N-hydroxyl quaternary amine ions, while electron-drawing groups are slightly less effective, possibly because electron-donating groups help disperse the positive charge and increase the electron density on the nitrogen atom, resulting in more stable compounds. When the –CH3 group on the nitrogen atom was replaced by –C2H5, the N-hydroxyl quaternary amine ions were also efficiently produced. The corresponding N-hydroxylation products have been further elucidated using tandem MS spectra (Fig. S8†), which show similar cleavage patterns to those of the N-hydroxylation of DMA.
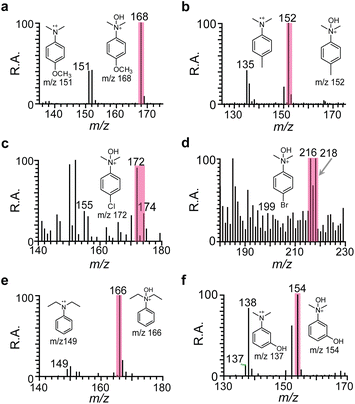 |
| Fig. 3 Reaction scope of the N-hydroxylation reaction of DMA derivatives in water microdroplets. (a) 4-Methoxyl-N,N-dimethylaniline. (b) 4-Methyl-N,N-dimethylaniline. (c) 4-Chloro-N,N-dimethylaniline. (d) 4-Bromo-N,N-dimethylaniline. (e) N,N-Diethylaniline. (f) 3-Hydroxy-N,N-dimethylaniline. | |
Our results demonstrated that these six different reactions are all spontaneous and ultrafast in microdroplets, compared to similar reactions facilitated by undivided electrochemical cells or strong oxidants.5 Notably, the radical cations of 4-OMe-DMA, 4-Me-DMA, 4-Cl-DMA, 4-Br-DMA, 3-OH-DMA, and DEA were all observed in the mass spectra; however, the intensity of the radial cations varied, which might be a result of the differing oxidation potentials of these functional compounds. Therefore, the reactions in water microdroplets are characterized as radical/radical coupling. A yield of up to 80% for DMA hydroxylation reactions was calculated as the ratio of product intensity to the sum of reactant and product intensities, a method commonly used in MS-based micro-synthetic studies.29,30,36 This relatively high product yield could be attributed to a relatively faster reaction rate of radical/radical coupling reactions. Our method showed very high yields for N,N-dimethylaniline compounds, which have low oxidation potentials. However, it resulted in low yields (<2%) for other alkylamines and piperidines, which have higher oxidation potentials (Fig. S9†). This indicates the limitations of our method when applied to these types of amines.
Scale-up reaction
To demonstrate the feasibility of our strategy for preparative synthesis, a scale-up reaction was performed. We modified the microdroplet reaction platform by extending the capillary into an electrically grounded collection vial (Fig. S1†). This vial contained a grounded device to provide favorable ion soft landing conditions. A DMA solution was used as the substrate, aiming to collect quaternary ammonium cations. The product yield for the DMA hydroxylation reaction was 90%, with approximately 10 mg collected over a 10 hour period using a concentration of 1 mg mL−1 and a flow rate of 20 μL min−1. This yield is higher than the 80% yield reported from MS analysis, further confirming the reliability and reproducibility of our maximum yield. Additionally, by employing a four-channel spray system simultaneously (Fig. S1b†), with a concentration of 10 mg mL−1 and a flow rate of 100 μL min−1, gram-scale synthesis (0.8 g) can be achieved. The method was also independently replicated in the laboratory of Jiangxi University of Traditional Chinese Medicine, where other researchers achieved consistent results, underscoring the robustness and reproducibility of our method. The formation of quaternary ammonium cations was further verified by 13C-NMR spectroscopy, 1H-NMR spectroscopy, and MS experiments. The 13C-NMR spectrum and H-NMR spectrum recorded after the scale-up spraying of DMA experiments are shown in Fig. S10 and S11,† respectively. The signal at 68 ppm is assigned to the methyl group of N-hydroxyl-N,N-dimethylanilinium. This assignment was confirmed by comparing it with the 13C-NMR and 1H-NMR spectra of the corresponding N-oxide standard (Fig. S12 and S13†), where the signal at 63 ppm is assigned to the methyl groups. In addition, 15N-DMA was synthesized and analyzed using 1H-NMR and 13C-NMR analysis (Fig. S14 and S15†). A scale-up microdroplet reaction of 15N-DMA was then conducted, and the subsequent 1H-NMR and 13C-NMR analysis of the reaction products confirmed the hydroxylation on the nitrogen atom (Fig. S16 and S17†). These isotopically labeled experiments provided clear evidence of the hydroxylation process and the precise position of the hydroxyl groups.
Elucidation of the hydroxylation mechanism
With the successful detection of DMA+˙ and its corresponding hydroxylated product, N-hydroxyl-N,N-dimethylanilinium, we advanced our investigation into the mechanism of the DMA+˙ hydroxylation reaction. The reaction is crucial for transforming DMA into valuable pharmaceuticals and synthetic intermediates. Direct hydroxylation of hydrocarbon bonds in aromatic compounds is a straightforward and desirable method, but it remains challenging, especially from a sustainability perspective.2 Cheng et al. described the dimerization process between two DMA+˙ molecules through a radical cation–radical cation coupling mechanism.37 The aqueous microdroplet environment can generate highly reactive species, including water radical cations and hydroxyl radicals.25,32 The high surface area-to-volume ratio and the unique electric field effects at the microdroplet interface can facilitate the formation of these reactive species. In our system, the interaction of DMA with (H2O)2+˙, which is produced in situ within the microdroplets, leads to the oxidation of DMA to DMA+˙. The (H2O)2+˙ acts as a strong oxidant, abstracting an electron from DMA and resulting in the formation of the DMA radical cation. As previously discussed, the proposed mechanism for the formation of m/z 138 when spraying DMA solution in microdroplets may involve the reaction of DMA+˙ with ˙OH from the water dimer radical cation, which has the [˙OH⋯H3O+] structure. Theoretically, as the radical cation is very unstable, it could couple with either another radical cation or with a neutral radical, with the latter pathway being more energetically favored. This is because the former process involves unfavorable charge–charge repulsion between two radical cations. We hypothesize that MS could elucidate the true reaction mechanism, as it allows us to capture and detect the radical cation of DMA and its derivatives, as shown in Fig. 3. Furthermore, control experiments reveal that the extracted ion chromatogram data show an increase in ion intensity of the peak at m/z 138 after spraying DMA samples, while the intensity of the peak at m/z 36 noticeably decreases (Fig. 4a). This observation suggests that the signals at m/z 138 are produced by the hydroxylation reaction between DMA+˙ and (H2O)2+˙.
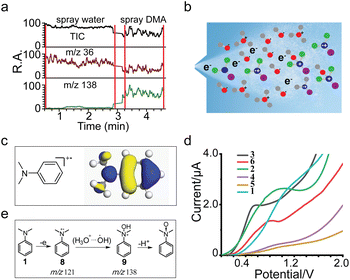 |
| Fig. 4 Mechanistic studies and proposal. (a) Typical total ion chromatogram (TIC) and extracted ion chromatogram (EIC) showing reactants at m/z 36 and products at m/z 138 before and after spraying the DMA solution in microdroplets. (b) Schematic for the formation of N-hydroxyl-N,N-dimethylanilinium in microdroplets. (c) Computation of the singly occupied molecular orbital of the DMA radical cation at the B3LYP level of theory. (d) Cyclic voltammograms obtained in H2O. 1: DMA, 2: 4-OMe-DMA, 3: 4-Me-DMA, 4: 4-Cl-DMA, 5: 4-Br-DMA, and 6: DEA. (e) Mechanistic proposal. DMA : N,N-dimethylaniline. | |
A recent study indicates that at the water–oil interface, water dimers exist predominantly as H2O+–H2O− couples, with a preference for the negative end being closer to the hydrophobic medium,38 potentially contributing to the electric double layer. The water radical cation has been directly observed as the ionized water dimer (H2O)2+˙ in microdroplets by Cooks and colleagues.18 The [˙OH⋯H3O+] structure, a conformation of (H2O)2+˙, facilitates proton transfer and hydroxyl radical chemistry with appropriate substrates.33 Our group has also prepared (H2O)2+˙ ions via low-energy corona discharge ionization MS and investigated their interaction with DMA. The experimental setup is depicted in Fig. S18,† where one channel generates (H2O)2+˙ ions while another channel produces DMA molecules. The results show a base peak at m/z 122 (DMA + H)+ and a smaller peak at m/z 138 (N-hydroxyl-N,N-dimethylanilinium) as seen in Fig. S19.† Additionally, extracted ion chromatogram data indicate an increase in the ion intensity of the peak at m/z 138 after introducing DMA samples, while the intensity of the peak at m/z 36 decreases (Fig. S20†). These results suggest that ˙OH radicals generated from [˙OH⋯H3O+] by corona discharge MS are responsible for N-hydroxyl-N,N-dimethylanilinium (m/z 138) formation. In our study, high intensities of (H2O)2+˙ ions and DMA+˙ were also obtained at the gas–liquid interface of microdroplets under the influence of a strong electric field (∼109 V m−1), as shown in Fig. 4b. At the gas–liquid interface, water dimer radical cations exist in the form of [˙OH⋯H3O+] couples, with the ˙OH radical being closer to the hydrophobic medium DMA+˙, while the H3O+ end, being positively charged, is repelled by DMA+˙ and moves away. Thus, N-hydroxyl-N,N-dimethylanilinium at m/z 138 is observed during DMA spraying.
The regional selectivity observed in our study aligns with the reactions of aromatic radical cations with nucleophiles and is supported by the single occupied molecular orbital (SOMO) distribution of DMA+˙. As depicted in Fig. 4c, the SOMO of DMA+˙ is significantly concentrated at the nitrogen atom site, as well as at the ortho and para positions relative to the N,N-dimethyl group. This distribution aligns with the experimental findings where m/z 138 corresponds to N-hydroxyl-N,N-dimethylanilinium. The cyclic voltammetry (CV) results for compounds 1–6, shown in Fig. 4d, reveal relatively low oxidation peak potentials (1.1 V for DMA, 0.9 V for 4-OMe-DMA, 0.5 V for 4-Me-DMA, 0.9 V for 4-Cl-DMA, 1.0 V for 4-Br-DMA, 1.1 V for DMA, and 0.8 V for DEA), indicating their readiness to be oxidized at the anode. Together, the mechanisms for N-hydroxylation of DMA, derived from the intermediates captured by MS and the products from the scale-up synthesis, are summarized in Fig. 4e. Initially, DMA is spontaneously oxidized at the gas–liquid interface of water microdroplets, yielding the DMA+˙ radical cation at m/z 121. This cation then reacts with the ˙OH from the water dimer radical cation, with the [˙OH⋯H3O+] structure, to produce N-hydroxyl-N,N-dimethylanilinium. This intermediate can further react to form N-hydroxyl-N,N-dimethylaniline N-oxide under certain conditions.
Conclusions
In summary, we have developed a green and rapid method for achieving N-hydroxylation of aromatic amines with diverse electronic properties, without the use of any catalysts or chemical oxidants. The intrinsic oxidation properties of water microdroplets are demonstrated by the generation of water dimer radical cations. Importantly, the N-hydroxylation of aromatic amines to quaternary ammonium cations was observed in water microdroplets. This result supports the idea that the strong electric fields within water microdroplets help aromatic amines lose one electron to form the corresponding radical cations, which quickly capture the ˙OH radical generated from the dissociation of water radical cations to form quaternary ammonium cations. From this work, we anticipate that (H2O)2+˙ will open a wide range of opportunities for designing new chemical reactions.
Data availability
Experimental data have been made available as the ESI. The authors declare that all the data that support the findings of the study are included in the main text and ESI.† The source data are available from the corresponding authors on request.
Author contributions
Xiaoping Zhang and Huanwen Chen identified and characterized the reactions and designed the experiments. Pinghua Hua and Minmin Duana performed the experiments and analyzed the experimental results. Xiaoping Zhang and Xinglei Zhang co-wrote the manuscript. Konstantin Chingin and Roman Balabin performed the computation and described the computational section.
Conflicts of interest
The authors declare that they have no competing interests.
Acknowledgements
This work was supported by the National Natural Science Foundation of China (22104014, 22364002, 22376084, and 22164002), Jiangxi Provincial Natural Science Foundation (20232BAB213047 and 20242BAB25138), Jiangxi Province International Cooperation Project (20203BDH80W010 and 20232BBH80012), The Youth Talent Support Program of Jiangxi Association for Science and Technology (2025QT06), and Jiangxi University of Chinese Medicine School-level Science and Technology Innovation Team Development Program (CXTD22005 and 2004-5252300403).
References
- Z. Li, et al., A tautomeric ligand enables directed C-H hydroxylation with molecular oxygen, Science, 2021, 372, 1452–1457 CrossRef CAS PubMed.
- H. Long, T.-S. Chen, J. Song, S. Zhu and H.-C. Xu, Electrochemical aromatic C–H hydroxylation in continuous flow, Nat. Commun., 2022, 13, 3945 CrossRef CAS PubMed.
- J. Lv, B. Zhao, Y. Yuan, Y. Han and Z. Shi, Boron-mediated directed aromatic C–H hydroxylation, Nat. Commun., 2020, 11, 1316 CrossRef CAS PubMed.
- T. Baumeister, et al., Continuous flow synthesis of amine oxides by oxidation of tertiary amines, React. Chem. Eng., 2019, 4, 1270–1276 RSC.
- Y. Ogata, K. Tomizawa and H. Maeda, Kinetics of the tungstate-catalyzed H2O2 oxidation of amines in aqueous methanol. Acidity effect, Bull. Chem. Soc. Jpn., 1980, 53, 285–286 CrossRef CAS.
- M. P. Walsh, J. M. Phelps, M. E. Lennon, D. S. Yufit and M. O. Kitching, Enantioselective synthesis of ammonium cations, Nature, 2021, 597, 70–76 CrossRef CAS PubMed.
- W. Wang, A. Buchholz, I. I. Ivanova, J. Weitkamp and M. Hunger, Synthesis and immobilization of quaternary ammonium cations in acidic zeolites, Chem. Commun., 2003, 2600–2601 RSC.
- C.-Y. Liu, Y. Chen and J. Hu, Identification of the electrogenerated hidden nitrenium ions by in situ mass spectrometry, Anal. Chem., 2024, 96, 3354–3361 CrossRef CAS PubMed.
- Q.-Y. Cheng, T. Wang, J. Hu, H.-Y. Chen and J.-J. Xu, In situ probing the short-lived intermediates in visible-light heterogeneous photocatalysis by mass spectrometry, Anal. Chem., 2023, 95, 14150–14157 CrossRef CAS PubMed.
- Q. Wan, et al., Visible-light-activated aziridination reaction enables simultaneous resolving of C=C bond location and the sn-position isomers in lipids, Chin. Chem. Lett., 2024, 35, 108775 CrossRef CAS.
- X. Yan, Emerging microdroplet chemistry for synthesis and analysis, Int. J. Mass Spectrom., 2021, 468, 116639 CrossRef CAS.
- X. Yan, Y.-H. Lai and R. N. Zare, Preparative microdroplet synthesis of carboxylic acids from aerobic oxidation of aldehydes, Chem. Sci., 2018, 9, 5207–5211 RSC.
- L. Zhao, et al., Sprayed water microdroplets containing dissolved pyridine spontaneously generate pyridyl anions, Proc. Natl. Acad. Sci. U. S. A., 2022, 119, e2200991119 CrossRef CAS PubMed.
- H. Hao, I. Leven and T. Head-Gordon, Can electric fields drive chemistry for an aqueous microdroplet?, Nat. Commun., 2022, 13, 280 CrossRef CAS PubMed.
- M. T. C. Martins-Costa and M. F. Ruiz-López, Electrostatics and chemical reactivity at the air–water interface, J. Am. Chem. Soc., 2023, 145, 1400–1406 CrossRef CAS PubMed.
- J. K. Lee, et al., Spontaneous generation of hydrogen peroxide from aqueous microdroplets, Proc. Natl. Acad. Sci. U. S. A., 2019, 116, 19294–19298 CrossRef CAS PubMed.
- C. Gong, et al., Spontaneous reduction-induced degradation of viologen compounds in water microdroplets and its inhibition by host–guest complexation, J. Am. Chem. Soc., 2022, 144, 3510–3516 CrossRef CAS PubMed.
- L. Qiu and R. G. Cooks, Simultaneous and spontaneous oxidation and reduction in microdroplets by the water radical cation/anion pair, Angew. Chem., Int. Ed., 2022, 61, e202210765 CrossRef CAS PubMed.
- L. Qiu, M. D. Psimos and R. G. Cooks, Spontaneous oxidation of aromatic sulfones to sulfonic acids in microdroplets, J. Am. Soc. Mass Spectrom., 2022, 33, 1362–1367 CrossRef CAS PubMed.
- X. Zhang, et al., Mass spectrometry distinguishing C=C location and cis/trans isomers: a strategy initiated by water radical cations, Anal. Chim. Acta, 2020, 1139, 146–154 CrossRef CAS PubMed.
- W. Yang, et al., Determination of C=C positions of unsaturated fatty acids in foods via ambient reactive desorption ionization with water dimer radical cations, J. Agric. Food Chem., 2024, 72, 845–856 CrossRef CAS PubMed.
- X. Zhang, X. Ren, Y. Zhong, K. Chingin and H. Chen, Rapid and sensitive detection of acetone in exhaled breath through the ambient reaction with water radical cations, Analyst, 2021, 146, 5037–5044 RSC.
- X. Zhang, et al., Efficient catalyst-free N2 fixation by water radical cations under ambient conditions, Nat. Commun., 2024, 15, 1535 CrossRef CAS PubMed.
- P.-R. Pan, Y.-S. Lin, M.-K. Tsai, J.-L. Kuo and J.-D. Chai, Assessment of density functional approximations for the hemibonded structure of the water dimer radical cation, Phys. Chem. Chem. Phys., 2012, 14, 10705–10712 RSC.
- Q. Wan, et al., Elucidating the underlying reactivities of alternating current electrosynthesis by time-resolved mapping of short-lived reactive intermediates, Angew. Chem., Int. Ed., 2023, 135, e202306460 CrossRef.
- T. A. Brown, H. Chen and R. N. Zare, Detection of the short-lived radical cation intermediate in the electrooxidation of N,N-dimethylaniline by mass spectrometry, Angew. Chem., Int. Ed., 2015, 127, 11335–11337 CrossRef.
- T. Morofuji, A. Shimizu and J.-i. Yoshida, Direct C–N coupling of imidazoles with aromatic and benzylic compounds via electrooxidative C–H functionalization, J. Am. Chem. Soc., 2014, 136, 4496–4499 CrossRef CAS PubMed.
- T. Morofuji, A. Shimizu and J.-i. Yoshida, Electrochemical C–H amination: synthesis of aromatic primary amines via N-arylpyridinium ions, J. Am. Chem. Soc., 2013, 135, 5000–5003 CrossRef CAS PubMed.
- J. K. Lee, S. Kim, H. G. Nam and R. N. Zare, Microdroplet fusion mass spectrometry for fast reaction kinetics, Proc. Natl. Acad. Sci. U. S. A., 2015, 112, 3898–3903 CrossRef CAS PubMed.
- M. Girod, E. Moyano, D. I. Campbell and R. G. Cooks, Accelerated bimolecular reactions in microdroplets studied by desorption electrospray ionization mass spectrometry, Chem. Sci., 2011, 2, 501–510 RSC.
- W. Yang, Q. Qi, W. Liu, M. Li and Y. Luo, Synthesis of isotope-labeled 15N-N,N-dimethylaniline, Chin. J. Org. Chem., 2012, 32, 145–148 CrossRef CAS.
- D. Xing, et al., Capture of hydroxyl radicals by hydronium cations in water microdroplets, Angew. Chem., Int. Ed., 2022, 61, e202207587 CrossRef CAS PubMed.
- M. Wang, et al., Abundant production of reactive water radical cations under ambient conditions, CCS Chem., 2022, 4, 1224–1231 CrossRef CAS.
- D. Mi, et al., Generation of phenol and molecular hydrogen through catalyst-free C-H activation of benzene by water radical cations, J. Am. Soc. Mass Spectrom., 2022, 33, 68–73 CrossRef CAS PubMed.
- M. Zheng, X. Zhang, Y. Cheng, L. Sun and X. Zhang, Hydroxyl transfer versus cyclization reaction in the gas phase: sequential loss of NH3 and CH2CO from protonated phenylalanine derivatives, Front. Chem., 2023, 10, 1094329 CrossRef.
- X. Zhang, et al., Demethylation C–C coupling reaction facilitated by the repulsive Coulomb force between two cations, Nat. Commun., 2024, 15, 5881 CrossRef CAS PubMed.
- H. Cheng, S. Tang, T. Yang, S. Xu and X. Yan, Accelerating electrochemical reactions in a voltage-controlled interfacial microreactor, Angew. Chem., Int. Ed., 2020, 59, 19862–19867 CrossRef CAS PubMed.
- S. Pullanchery, S. Kulik, B. Rehl, A. Hassanali and S. Roke, Charge transfer across C–H⋯O hydrogen bonds stabilizes oil droplets in water, Science, 2021, 374, 1366–1370 CrossRef CAS PubMed.
|
This journal is © The Royal Society of Chemistry 2024 |