DOI:
10.1039/D4SC04125A
(Edge Article)
Chem. Sci., 2024,
15, 13853-13863
Realizing altermagnetism in two-dimensional metal–organic framework semiconductors with electric-field-controlled anisotropic spin current†
Received
22nd June 2024
, Accepted 25th July 2024
First published on 27th July 2024
Abstract
Altermagnets exhibit momentum-dependent spin-splitting in a collinear antiferromagnetic order due to their peculiar crystallographic and magnetic symmetry, resulting in the creation of spin currents with light elements. Here, we report two two-dimensional (2D) metal–organic framework (MOF) semiconductors, M(pyz)2 (M = Ca and Sr, pyz = pyrazine), which exhibit both altermagnetism and topological nodal point and line by using first-principles calculations and group theory. The altermagnetic 2D MOFs exhibit unconventional spin-splitting and macroscopic zero magnetization caused by 4-fold rotation in crystalline real space and 2-fold rotation in spin space, leading to the generation and control of anisotropic spin currents when an in-plane electric field (E) is applied. In particular, pure spin current with the spin Hall effect occurs when E is applied along the angular bisector of the two spin arrangements. Our work indicates the existence of altermagnetic MOF systems and a universal approach to generate electric-field-controlled spin currents for potential applications in antiferromagnetic spintronics.
Introduction
Spintronics, using only the spin freedom of electrons to store, transport, and process information, offers the opportunity to reduce the generation of Joule heat in conventional electronics by utilizing spin alignment.1 There are two primary phases of spin alignment in the collinear form, i.e., ferromagnetic and antiferromagnetic order with the spins aligned in parallel and antiparallel directions, respectively. Ferromagnets are disturbed by the stray fields and antiferromagnets are normally absent of spin-splitting in energy bands, posing a formidable challenge in their practical applications in spintronics.2 In addition, the generation of pure spin current in spintronics as a substitute for electron current is highly desirable due to its potential for reduced power consumption, but it still faces big challenges.
Recently, altermagnets that exhibit a spontaneous spin-splitting of energy bands and lack macroscopic magnetism in the collinear antiferromagnetic form have been proposed based on crystalline and magnetic symmetry.3–6 Compared to conventional antiferromagnets, altermagnets possess unique characteristics, including unconventional spin-splitting due to the valley-dependent spin–momentum coupling, as well as adjustable charge and spin currents through anisotropic spin–momentum coupling.7 These features make them suitable for various applications such as spintronics,7,8 thermo-electric response,9 field-effect electronics,10 multiferroics,11 superconductivity,12etc. For example, the rutile metal RuO2 bulk shows unconventional broken time-reversal (T) symmetry transport anomalies and spintronic effects, including the anomalous Hall effect,13–15 charge–spin conversion,8,16 spin-torque phenomena,17–19 and giant and tunneling magnetoresistance.7,20 By doping, insulating perovskite La2CuO4 transforms into a high-temperature d-wave superconductor.3,21 Two-dimensional (2D) V2Se2O crystal is a multifunctional inorganic material that displays giant piezomagnetism, which enables manipulation of both spin and valley.22 Furthermore, 2D CrO crystal exhibits large spin-splitting and spin–momentum-locked transport, together with a high magnetic transition temperature.23 Theoretically, high-throughput screening from existing databases has enriched the quantity of altermagnets in both 2D and 3D systems.24–26 Experimentally, spin-splitting in altermagnets can be detected from photoemission spectroscopy and ARPES measurements.27–29 However, it is highly desirable to explore experimentally feasible altermagnets in the nanoscale that are beyond the limiting range of existing inorganic altermagnetic materials.
Metal–organic frameworks (MOFs), assembled with metal nodes and organic linkers, provide a versatile chemical platform that exhibits peculiar magnetism in 2D forms.30 By modulating the coordination modes, the spin-related properties of 2D MOFs can be created and regulated. For instance, the orthorhombic [Fe(pyz)2(NCS)2]n (pyz = pyrazine) polymeric sheet shows antiferromagnetic behavior below 8.5 K and the neutron powder diffraction showed a three-dimensional (3D) long-range order below 6.8 K.31,32 The antiferromagnetic triclinic M(NCS)2(pzdo)2 (M = Mn or Co, pzdo = pyrazine dioxide) maintain its magnetic order at the temperature below 8.4 and 11.2 K for Mn and Co, respectively.33 Non-crystal-symmetry compensated magnets, such as the ferromagnetic orthorhombic Cr(ptl)2 (ptl = pentalene),34 the ferrimagnetic tetragonal Cr(pyz)2,35–37 and the pentafunctional ferrimagnetic Cr(tdz)2 (tdz = 1,2,5-thiadiazole),38 have high magnetic transition temperatures of 560, 342, and 378 K, respectively, because of the strong d–p direct exchange interaction between the metal and N atoms.
Here, we present two 2D MOF altermagnetic semiconductors, namely M(pyz)2 (M = Ca and Sr), by assembling the alkaline earth metal atoms as nodes and pyrazine (1,4-diazine) as organic linkers based on first-principles calculations. The 2D MOFs exhibit altermagnetism at their ground state, characterized by unconventional spin-splitting and macroscopic zero magnetization caused by the 4-fold rotation (C4z) in crystalline real space and 2-fold rotation (C2) in spin space. Particularly, spin current (Js) can be generated and reversely regulated by the in-plane electric field (E) direction. The spin Hall effect (SHE)39 generates pure transverse Js when E is applied along the angular bisector of two spin configurations.
Results and discussion
Conventional antiferromagnets lack macroscopic magnetism and have degenerate energy bands due to opposing spin channels, typically displaying similar electric behaviors to nonmagnetic materials. Altermagnet is a novel type of antiferromagnet that exhibits additionally unconventional spin polarization and spin-splitting in reciprocal momentum space, which is determined by specific crystallographic symmetry and magnetic ground state.3,4,9,13,22,23,27,28,40 As shown in Fig. 1a, in conventional antiferromagnets, opposite-spin sublattices are connected by inversion (i) or translation (t). These connections can be represented as the notation [C2‖i] or [C2‖t], where the transformation on the left side of the double vertical bars affects only the spin space, while the one on the right side affects only the crystalline real space. Spin valleys are isotropic, and sublattices of conventional antiferromagnets belong to the same crystallographic point group G as the material. In the case of altermagnets, as shown in Fig. 1b, the α and β spins are connected by rotation (R), but inversion and translation are prohibited. The spin symmetry notation is denoted as [C2‖R], and the point group of sublattice H is a subgroup of G with an index of 2. The α and β spin channels in altermagnets are nondegenerate, equally occupied, and anisotropic.
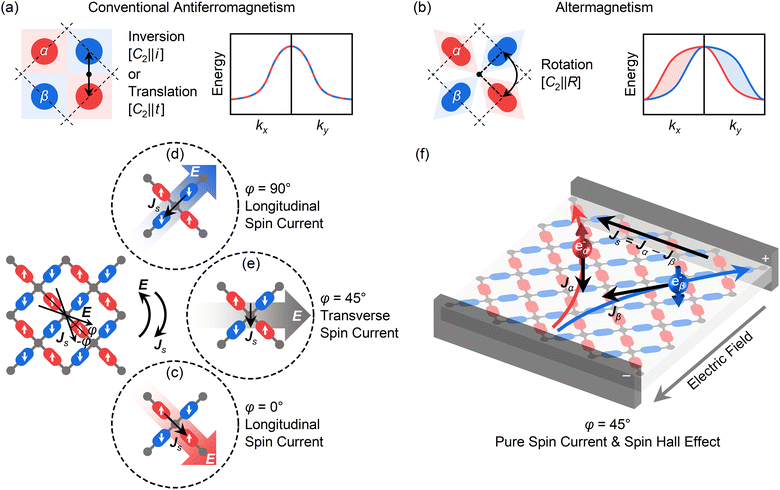 |
| Fig. 1 Characteristics of altermagnetism and schematic illustrations of the anisotropic spin current (Js) regulation. (a) Opposite-spin sublattices (light-colored quadrilaterals, same below) in conventional antiferromagnets are connected by inversion (i) or translation (t) and spin valleys are isotropic (left panel), which is reflected in the band degeneracy of α and β spins (right panel). (b) For altermagnets, opposite-spin sublattices are connected by rotation (R) rather than inversion and spin valleys are anisotropic (left panel), which is reflected in the spin-splitting of energy bands (right panel). In altermagnets, Js can be directly regulated by the in-plane electric field (E) direction due to the anisotropic spin channels, and as E rotates, Js will rotate in the opposite direction at the same angle (φ). (c and d) When the electric field follows the direction of a certain spin arrangement, longitudinal spin currents will appear and exhibit opposite directions under different spins. (e and f) When the electric field follows the angular bisector of two spin arrangements, pure transverse Js with no Hall charge current will be observed, and the charge–spin conversion ratio will reach maximum. The carriers with α and β spins undergo the same degree of deflection but in opposite directions under the in-plane E on the angular bisector, resulting in the spin Hall effect (SHE). Particularly, for the altermagnetic M(pyz)2 (M = Ca and Sr) with C4z rotation between sublattices, the longitudinal and transverse Js will alternate in cycles of 45°, and the pure transverse J and SHE will appear at φ = 45°. Red and blue denote opposite spins. Dashed boxes in (a) and (b) are the unit cells in spin space. | |
The time-reversal symmetry arises from the multiplication of [C2‖X] and the spin-only group symmetry [C2⊥‖T], i.e., [T‖TX]. Here, X is a set of symmetric elements, and C2⊥ represents a 180° rotation around an axis perpendicular to spins. In conventional antiferromagnets, X is the crystalline point group G including the identity element (E). Thus, the T-symmetry is an element of its spin point group and is reflected in the band degeneracy of α and β spins (Fig. 1a). In the case of altermagnets, X = G ⊖ H, where the coset G ⊖ H does not include E. As a result, the T-symmetry is broken, leading to the spin-splitting of energy bands and spin polarization in momentum space (Fig. 1b, and see details in ESI Note S1†).
Due to the anisotropic spin channels, the spin current (Js = Jα − Jβ) in altermagnets can be regulated by directly controlling in-plane E direction. As E rotates, Js will undergo a rotation in the opposite direction, maintaining the same angle (φ). When the in-plane E is applied along a certain spin valley configuration of α or β (Fig. 1c and d), the spin current will be parallel to the direction of the electric field and will flow in the opposite direction depending on the spin types along which E is applied. When E is applied at the angular bisector of two spin arrangements (Fig. 1e and f), carriers with α and β spins will undergo equal deflection but in opposite directions, leading to pure transverse Js and SHE (see details in ESI Note S2†).39 Anisotropies, spin-splitting, and spin current in altermagnets arise from their unique symmetries independent of spin–orbit coupling (SOC), enabling their realization in materials containing only light elements.27,28 The primary rules for identifying altermagnetic phases of crystals have been proposed in previous reports, which serve as a foundation for the rational design of such materials.3,4
Benefitting from the abundant ligands, unpaired electrons, and various topologies, it is highly promising to construct MOFs with targeted magnetic order, such as altermagnetism, by selecting the core metal, organic linker, and topology.
Structure and stabilities
In 2D MOFs, metal atoms are tetra-coordinated with four adjacent pyrazine ligands via metal–nitrogen bonds, forming a square plane. The pyrazine ligand exhibits a C4z rotational symmetry with the metal atom as its center (Fig. 2a–c). The Be(pyz)2 and Mg(pyz)2 undergo a phase transition from tetragonal to orthorhombic, which is caused by the small radius of alkaline earth metal atoms, the significant steric hindrance between hydrogen atoms in neighboring pyrazine ligands, and a substantial torsion angle along the Be(Mg)–N bonds. However, Ca(pyz)2, Sr(pyz)2, and Ba(pyz)2 can exhibit tetragonal phases with the space group of P4/nbm (#125). The calculated lattice parameters, bond lengths between the metal and N atoms, sheet thicknesses, torsion angles, Bader charge transfer from the metal atoms to pyrazine ligands, formation energies per stoichiometric formula, and electronic band gaps are summarized in Table 1. The lattice parameters and bond lengths between metal and nitrogen atoms increase when the period of metal atoms increases, while torsion angles and monolayer thicknesses decrease.
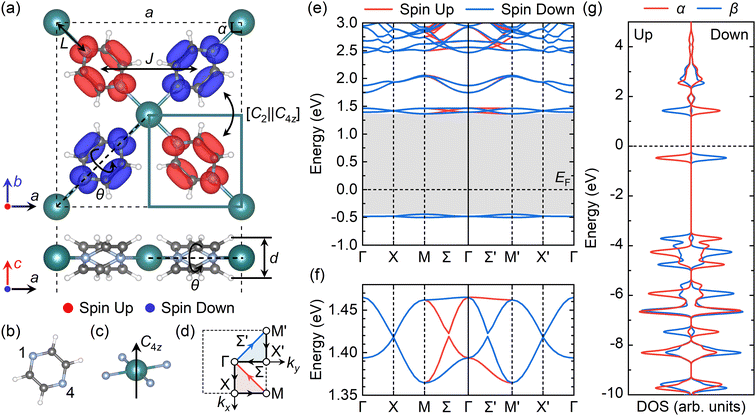 |
| Fig. 2 Altermagnetic 2D Ca(pyz)2 with unconventional spin-splitting and valley-dependent spin-polarized sublattices. (a–c) Structure and spin valleys of the altermagnetic 2D Ca(pyz)2. (a) The P4/nbm (#125) Ca(pyz)2 is connected by (b) the pyrazine (1,4-diazine) ligands and Ca atoms with (c) Ca–N tetra-coordination, where the sublattices with different spins obtain the C4z rotational symmetry in the planar square field. The isosurface value of spin density in (a) is 0.005 e bohr−3, where the red and blue colors represent up and down spins, respectively. Green, gray, blue, and white balls represent Ca, C, N, and H atoms, respectively. Structural parameters in Table 1 are labeled in (a). (d) The first Brillouin zone (BZ) of the altermagnetic 2D Ca(pyz)2 with high symmetry k-points and k-paths. The red and blue arrows represent the different spin-splitting paths and the corresponding triangle areas are the two irreducible parts of the first BZ. (e) Band structure of the altermagnetic 2D Ca(pyz)2 with HSE06 functional via the k-path in (d), with (f) spin-splitting of the bands around CBM. The gray shadows in (e) represent the band gap (g) Sublattice and spin resolved density of states (DOS) of the altermagnetic 2D Ca(pyz)2. The α sublattice is framed with solid green lines in (a) while the β lattice is the corresponding opposite one. The Fermi level is set to 0 eV. | |
Table 1 The calculated lattice parameters (a for length and α for angle, in Å and °, respectively), bond lengths between metal and N atoms (L, in Å), sheet thicknesses (d, in Å), torsion angles (θ, in °), Bader charge transfer from the metal atoms to pyrazine ligands (C, in e−), formation energies
, and electronic band gaps
of the tetragonal M(pyz)2 (M = Ca, Sr, and Ba). The values of
are calculated with HSE06 hybrid functional
|
Ca(pyz)2 |
Sr(pyz)2 |
Ba(pyz)2 |
a
|
10.86 |
11.34 |
11.84 |
α
|
90.00 |
90.00 |
90.00 |
L
|
2.39 |
2.56 |
2.74 |
d
|
2.39 |
2.21 |
2.13 |
θ
|
34.66 |
31.71 |
30.49 |
C
|
1.36 |
1.63 |
1.66 |
|
−4.65 |
−4.22 |
−4.15 |
|
1.81 |
1.79 |
1.70 |
To examine the stability of 2D MOFs, the average formation energy per stoichiometric formula
of the two thermally stable sheets is calculated using the following equation:
| 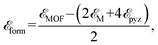 | (1) |
where
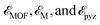
denote the energies of 2D MOFs, metal atoms, and pyrazine rings, respectively.
Table 1 provides a summary of the calculated energies, which vary from −4.11 to −4.65 eV. These values indicate that the synthesis of three altermagnetic 2D MOFs using metal atoms and pyrazine ligands is feasible. As shown in Fig. S1,
† temperature, reaction time, solvent, and additive conditions can be predicted by the MOF Synthesis Prediction Tool.
41 It is shown that water is predicted as the solvent with neutral or no additive for 2D MOFs with high certainty, while temperature and reaction time are predicted with medium certainty. Furthermore, the Bader charge transfer from the metal atoms to pyrazine ligands increases as the period of metal atoms increases, correlating to their electronegativities (
Table 1).
The structural dynamical stability of 2D MOFs is examined by the calculated phonon spectrum. Fig. S2† shows the absence of imaginary frequencies in the phonon spectra of Ca(pyz)2 and Sr(pyz)2, indicating that they are dynamically stable. On the other hand, Ba(pyz)2 exhibits the presence of soft phonon modes at around 20i cm−1, corresponding to the relative vibration of Ba atoms along c-axis. This is partly due to the large atomic radius of Ba, which increases the lattice parameter and causes structural instability. Thus, only Ca(pyz)2 and Sr(pyz)2 are studied in the following (Tables S1 and S2†).
The thermal stabilities of the 2D MOFs are analyzed using ab initio molecular dynamic (AIMD) simulations. The simulations are performed at 300, 600, and 900 K for 5 ps with a time step of 1 fs. The structural snapshots are shown in Fig. S3 and S4.† 2D MOFs exhibit thermal stability by preserving their structural integrity at a temperature as high as 600 K. Additionally, it is shown that pyrazine rings can easily rotate between the metal nodes. To verify the stability with different torsion angles, the PBE self-consistent-field energies with different torsion angles are calculated, as shown in Fig. S5.† It is shown that the relaxed structures are the most stable with an energy minimum to a single value of torsion angles, indicating their stability. The energy variation is relatively small when the torsion angle is between 30° and 90°, corresponding to the pyrazine rotating in AIMD simulations. Additionally, due to the lattice parameter of Ca(pyz)2 being smaller than Sr(pyz)2, the steric hindrance between neighboring pyrazine ligands of flat Ca(pyz)2 is larger, thus with a larger energy difference to the stable phase.
The stress–strain method is used to analyze the mechanical stabilities by calculating the elastic constants. The values of C11, C12, and C66 for Ca(pyz)2 are 21.85, 21.63, and 17.61 N m−1. For Sr(pyz)2, the values are 15.91, 14.00, and 15.10 N m−1, respectively. Both Ca(pyz)2 and Sr(pyz)2 are mechanically stable, confirmed by their elastic constants (see details in Methods).42
Altermagnetism and magnetic transition temperature
The magnetic ground states of 2D MOFs are determined by considering several magnetic configurations as illustrated in Fig. S6 and S7.† As summarized in Table 1, the ligand becomes spin-polarized by gaining about one electron from the metal. The magnetism of Ca(pyz)2 and Sr(pyz)2 derives primarily from the N atoms located on the pyrazine rings, as indicated in Tables S3 and S4.† Fig. S6† demonstrates that Ca(pyz)2 is nonmagnetic when the spin orientations of N atoms within the same pyrazine are arranged in opposite directions. Thus, the spin polarization is accounted for in terms of the pyrazine ring. Four magnetic states are constructed using the D4 real space symmetry of Ca(pyz)2 and Sr(pyz)2, including altermagnetic, ferromagnetic, stripe antiferromagnetic, and nonmagnetic states, as shown in Fig. S7.† Both Ca(pyz)2 and Sr(pyz)2 prefer the altermagnetic state, where adjacent organic ligands tend to be arranged in antiparallel with each other (Fig. 2a). The energy differences between the altermagnetic and other magnetic states are summarized in Table 2.
Table 2 Magnetic energies with HSE06 functional (in meV per unit cell) of four different magnetic states relative to the ground altermagnetic state, along with the deduced magnetic exchange parameter (J, in meV) and magnetic anisotropy parameter (D, in μeV) of Ca(pyz)2 and Sr(pyz)2
|
Ca(pyz)2 |
Sr(pyz)2 |
Altermagnetic |
0 |
0 |
Ferromagnetic |
15.4 |
11.7 |
Stripe antiferromagnetic |
7.3 |
5.2 |
Nonmagnetic |
1485.3 |
1532.0 |
J
|
7.70 |
5.85 |
D
|
0.21 |
0.95 |
Fig. S8† displays the total and atomically resolved density of states
of the altermagnetic 2D MOFs. Both Ca(pyz)2 and Sr(pyz)2 are semiconductors with band gap values of 1.81 and 1.79 eV, respectively (Fig. 2e and S9†). The DOS around the Fermi level
is mainly contributed by the pz orbitals of N and C atoms, while the contribution from metal atoms is negligible, as shown in Fig. S8.† This can also be confirmed by the orbital-resolved band structures, as shown in Fig. S10.† To further understand the altermagnetic exchange, band centers
are calculated as
| 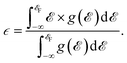 | (2) |
It is shown that in 2D MOFs, the exchange of N's p orbitals contributes to the magnetic exchange parameters (
J), resulting in an antiparallel alignment between adjacent ligands. For metal atoms without magnetism, there is almost no electron population on d orbitals, so there is no superexchange interactions mediated by them (Fig. S11
†). The
J values are small due to the weak π-interaction between pyrazine ligands (
Table 2), resulting in altermagnetic transition temperatures of 15.5 K for Ca(pyz)
2 and 11.5 K for Sr(pyz)
2 (Fig. S12
†). These values are lower than that of the altermagnetic metal RuO
2 containing d electrons (400 K),
4,13,43 but higher than that of
κ-(BETS)
2FeBr
4 (2.5 K).
44
Symmetries, spin-splitting, and valley-dependent spin polarization
As depicted in Fig. 2a, the unit cell of 2D Ca(pyz)2 altermagnet consists of four magnetic pyrazine ligands. There is no inversion center between the sites occupied by the magnetic atoms from the opposite-spin sublattices. Based on the identification theories of spin point groups,45 it is shown that in Ca(pyz)2, the opposite spin sublattices are linked by a C4z transformation without any translation. The real-space crystallographic point group is G = 4/mmm and the molecular point group of the sublattice is H = mmm. Thus, the nontrivial spin Laue group, which represents the spin–momentum locking in the altermagnetic phase can be decomposed as follows: | 24/1m1m2m = [E‖mmm] + [C2‖C4z][E‖mmm], | (3) |
where the superscript on the upper left corner indicates the symmetry of the magnetic state at that place. The spin–momentum locking in altermagnetic 2D Ca(pyz)2 and Sr(pyz)2 is protected by the 24/1m1m2m (#15.8.193) spin symmetry, which shows an anisotropy characterized by d-wave symmetry (dxy) and two spin-degenerate nodal surfaces that pass through the k-point Γ (Γ–X and Γ–X′ in Fig. 2d).3 Based on eqn (3), T-symmetry is broken due to the absence of E in the coset 4/mmm ⊖ mmm.3 The lifted spin degeneracies in the altermagnetic phase are allowed for crystal momenta (k) and follow the equation AHk = k′ ≠ k, which leads to split energy isosurfaces
from spin up and spin down states with an equal number (Fig. 2d–f). Consequently, the spin (s) and k-dependent bands are represented as |  | (4) |
Note that spin-splitting only occurs on paths of spin channel arrangements, i.e., Γ–(Σ)–M for spin up and Γ–(Σ′)–M′ for spin down.4 Fig. S13† displays the HSE06 band structures including SOC of 2D Ca(pyz)2 and Sr(pyz)2. For both 2D MOFs with light elements, SOC has no contribution to spin-splitting, ruling out the SOC-induced weak ferromagnetism.27,46
Additionally, Ca(pyz)2 can be also manually constructed in a P4/nmm (#129) phase based on the symmetry, with the C4z rotation axis located at the edge center of the tetragonal lattice (Fig. S14a and Table S5†). This phase also satisfies the symmetry conditions for exhibiting altermagnetism. The distinctive spin-splitting nature can be observed in its energy band (Fig. S14b†). However, the P4/nmm (#129) phase of Ca(pyz)2 is found to be dynamically unstable (Fig. S14c†).
To summarize, the energy band of altermagnetic Ca(pyz)2 split in opposite directions along the Γ–(Σ)–M (red line in Fig. 2d) and M′–(Σ′)–Γ (blue line in Fig. 2d) path directions is opposite, leading to zero magnetization and non-polarization for Ca(pyz)2 and Sr(pyz)2 externally, as shown in Fig. 2f. Fig. 2g demonstrates that the sublattice in the altermagnetic 2D Ca(pyz)2, which possesses a specific momentum space valley exhibiting spin-polarization. The spin polarizations of different valleys lead to equal but opposite DOS, where g+(α) ≠ g−(α), g+(β) ≠ g−(β), and g±(α) = g∓(β), with the symbol “+” and “−” denoting spin up and down, respectively.
Topological points and lines
Fig. 2f displays a trivial nodal point at the k-point X in the energy band around CBM with a zero Chern number protected by a 2D irreps
3 ⊕
4 in little group 2/m,47 and the spin-splitting states along the Γ–(Σ)–M path leads to a narrow energy gap of 3.57 meV in Ca(pyz)2. A topological line is formed along the X–X′ path with a small gap at the mean value of 1.95 meV (Fig. 3a and S15†). Similar topological properties can also be presented in Sr(pyz)2 (Fig. S16†). The movable nodal line of altermgnetic 2D MOFs is protected by the magnetic space group P4′/nbm′ (#125.367).48 To confirm the existence of the nodal point at the k-point X, the edge states along the (100) and (110) directions of the first Brillouin zone (BZ) are calculated using the tight-binding approximation (TBA). The TBA Hamiltonian is derived from the p orbitals of N and C which contribute the most band structure around the Fermi level
as shown in Fig. S17 and S18.†Fig. 3b, c, and S16† display a quadruple degenerate Dirac cone edge state that is evident at the projected k-point
in 2D MOFs. On the
–
′ path, a topological line is observed with a negligible gap. The existence of topological points and lines can give rise to remarkable physical properties, such as the anomalous Hall effect and transportation.49,50
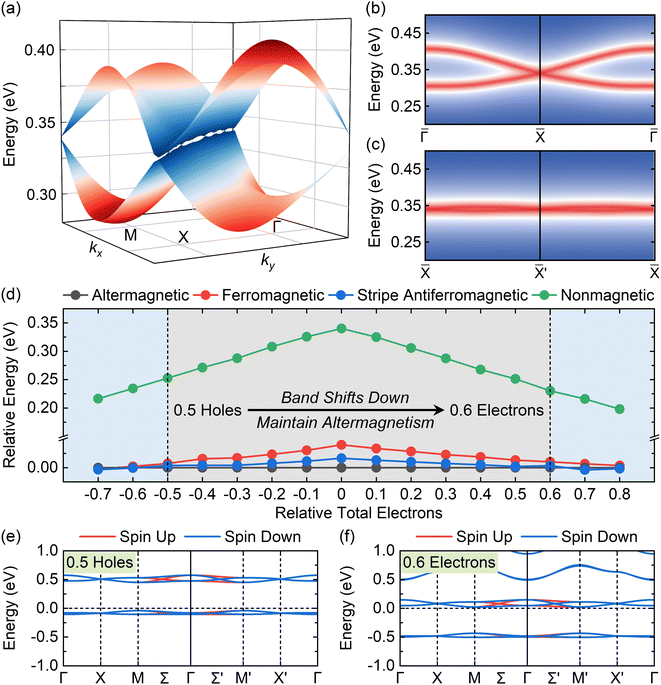 |
| Fig. 3 Topological points and lines in the altermagnetic 2D Ca(pyz)2. (a) Σ-centered 3D energy band structure of the altermagnetic spin-splitting near CBM for Ca(pyz)2. (b and c) Topological edge states on the (b) (100) and (c) (110) surfaces of BZ for Ca(pyz)2, where the k-points , , and ′ are the corresponding projections along the surfaces of BZ. The red color indicates a higher state along the edge. (d) Relative energy difference of different magnetic states according to altermagnetic phase when doping with electrons or holes. The altermagentic ground state can be maintained within a doping range of up to 0.5 holes and 0.6 electrons (gray shadow). When the range of 0.2 electrons or holes is exceeded, the ground state becomes stripe antiferromagnetic (blue shadows). (e and f) Band structures of doping with (e) 0.5 holes and (f) 0.6 electrons around . By doping with electrons, the topological points and lines above can move downwards towards the vicinity of , with an opposite direction when doping with holes. The topological properties are investigated with PBE functional and is set to 0 eV. | |
Regulations of doping with electrons and holes in Ca(pyz)2 are displayed in Fig. 3d–f, S19, and Table S6.† As shown in Fig. 3d and Table S6,† the relative energy difference according to altermagnetic phase decreases when more electrons or holes are doped. The altermagnetism ground state of Ca(pyz)2 can be maintained within a doping range of up to 0.5 holes and 0.6 electrons. When the range of 0.2 electrons or holes is exceeded, the ground state turns into stripe antiferromagnetic. Fig. S19† displays altermagnetic band structures of doping with different amounts of electrons and holes. When doping with holes, the energy band moves upwards as a whole, and the valence band moves near the
(Fig. 3e). When doping with electrons, the band moves downwards, and the conduction band along with topological points and lines moves near the
(Fig. 3f). Band edges further proves that the topological points and lines around CBM still exists when doping with electrons and holes (Fig. S20†).
Angle-dependent anisotropies and spin current
Further investigation is conducted to examine the anisotropic behavior of mechanical, magnetic, and electrical properties of Ca(pyz)2 and Sr(pyz)2. Young's modulus and Poisson's ratio of Ca(pyz)2 and Sr(pyz)2 display anisotropy in relation to angle, as shown in Fig. S21 and S22.† The Young's modulus values of Ca(pyz)2 and Sr(pyz)2 are 38.91 and 30.06 N m−1, equivalent to 162.80 and 136.02 GPa by using sheet thickness, respectively. These values are comparable to those of the inorganic monolayers α-P (164 GPa)51 and MoS2 (270 ± 100 GPa),52 and larger to the value of Cr(tdz)2 sheet (39 GPa).38 Sr(pyz)2 has a minimal Poisson's ratio of zero, which suggests its excellent resistance to fracture and resilience.
Magnetic anisotropy energy (MAE) values reveal the preferred direction of magnetization in the ground state and demonstrate the relative resistance of the long-range magnetic order to thermal disturbance at temperatures above zero. Table S7† summarized that the easy axes of the altermagnetic 2D Ca(pyz)2 and Sr(pyz)2 are both orientated out-of-plane with the corresponding MAE values of 0.21 and 0.95 μeV per unit cell.
The conductivities in the longitudinal and transverse directions exhibit periodic oscillation in response to the steady in-plane E direction. This results in the generation of an anisotropic spin current (Js, see details in ESI Note S2†). Using the classic Boltzmann transport theory and nearly free electron model, the energy
of the band edge in a 2D gapped system can be expressed as
| 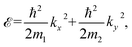 | (5) |
where
m1 and
m2 are the effective masses in the
kx and
ky directions. The charge (
σ) and spin (
σs) conductivity tensors for an in-plane
E along
φ direction in M(pyz)
2 (M = Ca and Sr) can be derived as
|  | (6) |
and
|  | (7) |
where
σα and
σβ are the conductivity originating from different spins, and
n and
τ denote carrier density and relaxation time, respectively, which are assumed constant at 0 K (see details in ESI Note S2
†).
As shown in Fig. 4a, the longitudinal charge current remains constant, with angle-dependent Jα and Jβ. In contrast, the transverse charge current continuously remains at zero, confirming the absence of Hall charge current in M(pyz)2. In Fig. 4b, the alternating period of longitudinal and transverse Js is 45°, as indicated by the red and blue lines. The longitudinal and transverse Js exhibit alternative positive and negative values every 90° with a phase difference of 45°, as indicated by the solid and dashed lines of each color. When an in-plane E is applied along a certain spin valley configuration of α or β, the resulting Js will be longitudinal and will flow in the opposite direction depending on the spin types along which E is applied. When E is applied at the angular bisector of two spin arrangements, carriers with α and β spins will undergo equal deflection but in opposite directions. This leads to the generation of SHE and pure transverse Js with no Hall charge current (J). The charge–spin conversion ratio (δ) achieves its maximal value of
Based on the spin-splitting from eqn (4) and anisotropy analysis, it is found that the effective mass in the kx direction
of α valley is equal to that in the ky direction
of β valley, and
of α valley is equal to
of β valley. By fitting the band structure with a quadratic function from the k-point M to Σ′ and Σ (Fig. 2d), we can determine the proportions of charge and spin currents from up and down spins, as well as the value of δ (Fig. S23†). The calculated δ of M(pyz)2 varies with angle, showing a periodicity of 45°. The largest δ values observed are 56.85% for Ca(pyz)2 and 86.04% for Sr(pyz)2 (Fig. 4c, and see details in ESI Note S2†). The corresponding angles between the two spin transport channels are 59.24° and 84.42°, respectively, which are significantly greater than those reported from SOC (12% for β-Ta (ref. 53) and 30% for β-W/CoFeB (ref. 54)), as well as higher than the values of recently reported altermagnets (28% for RuO2 (ref. 8) and 70% for V2Se2O (ref. 22)). Fig. 4d displays the spin Hall conductivity (SHC) calculated by Kubo formula with Qiao method (see details in Methods and ESI Note S2†).55 The semiconducting MOFs with light elements have a largest SHC value of 100 to 101ħ e−1 S cm−1 at about 3 eV above
. It is important to emphasize that Js is consistently found in M(pyz)2 (M = Ca and Sr), and the pure Js is aligned in a specific E direction.
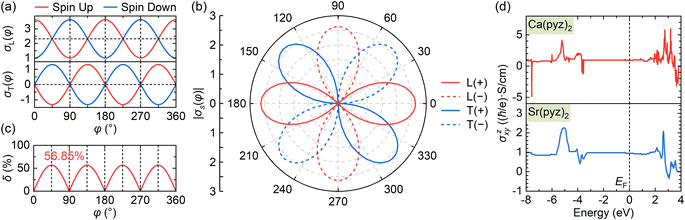 |
| Fig. 4 Anisotropic charge and spin current and spin-current conversion ratio in the altermagnetic 2D MOFs. (a) Angle-dependent anisotropy of the longitudinal (L, top panel) and transverse (T, bottom panel) conductivities (σ) contributed from spin up and down pyrazine ligands with the electric field direction (φ) as mentioned in Fig. 1c–e. The relative values of conductivities are taken near CBM of Ca(pyz)2. The total transverse charge current (σT) is always zero due to the opposite contributions from different spins. (b) Angle-dependent anisotropy of the longitudinal and transverse spin conductivities (σs) with the alternation in cycles of 45°, where the solid and dashed lines represent the positive and negative values of σ. (c) Spin-current conversion ratio (δ) as a trigonometric function of φ, with the maximal value of 56.85% for Ca(pyz)2 at 45°. (d) Spin Hall conductivities of altermagnetic 2D MOFs where is set to 0 eV. | |
Conclusions
In summary, we report two 2D MOFs, namely M(pyz)2 (M = Ca and Sr), which possess an altermagnetic ground state. These MOFs exhibit unique properties, including non-degenerate spins within zero macroscopic moments, π-magnetism, topological points and lines, and angle-dependent anisotropies by using first-principles calculations and group theory. It is demonstrated that magnetism is contributed by pyrazine rings in a certain arrangement, forming a magnetic symmetry of 24/1m1m2m (#15.8.193), which leads to the broken T-symmetry and spin-polarized bands in reciprocal momentum space. Both Ca(pyz)2 and Sr(pyz)2 are semiconductors with band gaps of 1.81 and 1.79 eV, respectively. An electric-field-controlled spin current can be achieved by making use of the anisotropic in-plane spin distribution. In particular, the spin Hall effect generates a pure spin current when an electric field is applied along the angular bisector of two spin configurations. Our study presents a rational design strategy to achieve multifunctional altermagnets based on 2D MOFs with electric-field-controlled spin currents.
Methods
The first-principles calculations are performed by density functional theory (DFT)56 implemented in the Vienna Ab initio Simulation Package (VASP).57,58 The DFT-D3 approach with the Becke–Johnson damping function is applied for the π–π type van der Waals (vdW) interactions between the neighboring pyrazine rings around the metal atoms.59,60 The Perdew–Bruke–Ernzerhof (PBE) functional61 is adopted in geometric optimization and the corrected Heyd–Scuceria–Ernzerhof (HSE06) hybrid functional62 is applied for describing the 2D band structure and density of states. The projector augmented-wave (PAW) pseudopotential63,64 is employed with the plane-wave energy cutoff of 500 eV. Monkhorst–Pack k-point meshes of 6 × 6 × 1 are investigated for structure optimization and property calculations with the convergence criteria of energy and force of 10−6 eV and 0.01 eV Å−1, respectively.65 Bader charge analyses are investigated to characterize the charge transfer between the metal atoms and pyrazine ligands.66–70 Spin–orbit coupling (SOC) is considered non-self-consistently for different spin orientations in the magnetic anisotropy energy (MAE) calculations with the energy convergence criteria of 10−8 eV, which is calculated as MAE = E[100] − E[001].71 The 3D band structure is carried out via the PBE functional with the grid spacing of 1.85 × 10−3 Å−1, corresponding to the Monkhorst–Pack k-point meshes of 50 × 50 × 1 in a reciprocal unit cell.65 The edge states are performed using the WannierTools72 code based on the tight-binding approximation (TBA) model constructed by Wannier90,73,74 where the p orbitals of N and C are investigated to simulate the TBA Hamiltonian, including a total of 72 bands of frozen window. The topological Chern numbers and band irreducible representations are calculated by VASPBERRY75 and IrRep package,76 respectively. The spin Hall conductivity (SHC) is calculated by the Kubo formula with the Qiao method55 implemented in Wannier90 with Berry k-point meshes of 25 × 25 × 1. All structures obtain a vacuum layer with a thickness of at least 15 Å to avoid mirror interactions between periodic layers.
To investigate the dynamic and thermal stabilities, phonon spectra are simulated within the density functional perturbation theory (DFPT)77,78 by the Phonopy code,79–81 and ab initio molecular dynamic (AIMD) simulations are performed for 5 ps with the time step of 1 fs at 300, 600, and 900 K in the canonical (NVT) ensemble by using Nose–Hoover thermostats.82–85 All calculations are performed in the 2 × 2 × 1 supercell with 168 atoms and the k-meshes of 3 × 3 × 1 and 1 × 1 × 1 are investigated in the phonon spectrum and AIMD simulations, respectively. The acoustic sum rule is included in phonon spectrum calculations to cure the tiny imaginary frequencies of pure translations.
The mechanical stability and properties are calculated with the stress–strain method.42 For a tetragonal 2D crystal in the linear elastic region, the stress–strain (σ–ε) relationship follows Hooke's law and can be represented by the Voigt notation, i.e.,
| 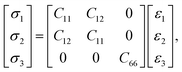 | (8) |
where
C11,
C12, and
C66 are the second-order in-plane elastic constants. In order to calculate the
Cij values, the strain range −2% ≤ Δ
ε ≤ 2% with the increment of 0.5% is investigated. The elastic stability conditions of a tetragonal 2D crystal is
C11 > 0,
C66 > 0, and
C11 > |
C12|.
42 The Young's modulus and Poisson's ratio are implemented with the VASPKIT code.
86
The estimated magnetic transition temperature is performed by the Monte Carlo simulation based on the Heisenberg model with a 24 × 24 × 1 supercell and a temperature step of 0.5 K from 1 to 50 K, as implemented in the SEU-mtc package.87 Due to the D4 symmetry and the magnetism completely provided by the pyrazine rings, the spin Hamiltonian (H) can be expressed as
| 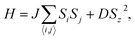 | (9) |
where
J and
S = 1/2 represent the nearest neighbor exchange parameter and the unit spin vector along the
c-axis, respectively. For M(pyz)
2 (M = Ca and Sr),
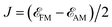
.
D denotes the magnetic anisotropy parameter calculated from the MAE value per pyrazine ligand. For M(pyz)
2 (M = Ca and Sr),
DS2 = MAE/4.
The anisotropy of spin conductivities is derived based on the theories in solid state physics and condensed matter with the DFT calculations of band structures, and more derivation details are summarized in ESI Note S2.†
Data availability
The data supporting the findings of this study are available within the article and its ESI.†
Author contributions
Y. C. carried out the calculations, derivations, and visualizations. H. L. and X. W. convinced the idea and supervised the work. All authors discussed the results and contributed to the manuscript.
Conflicts of interest
There are no conflicts to declare.
Acknowledgements
This research is supported by the National Natural Science Foundation for Distinguished Young Scholars (Grant No. 22225301), the National Natural Science Foundation of China (Grant No. 22303092), the Strategic Priority Research Program of the Chinese Academy of Sciences (Grant No. XDB0450101), the Innovation Program for Quantum Science and Technology (Grant No. 2021ZD0303302), the National College Student Innovation and Entrepreneurship Training Program (Grant No. 202110358035), and Super Computer Center of USTCSCC and SCCAS.
References
- S. A. Wolf, D. D. Awschalom, R. A. Buhrman, J. M. Daughton, S. von Molnár, M. L. Roukes, A. Y. Chtchelkanova and D. M. Treger, Science, 2001, 294, 1488–1495 CrossRef CAS PubMed.
- X. Li, X. Wu, Z. Li and J. Yang, Phys. Rev. B: Condens. Matter Mater. Phys., 2015, 92, 125202 CrossRef.
- L. Šmejkal, J. Sinova and T. Jungwirth, Phys. Rev. X, 2022, 12, 031042 Search PubMed.
- L. Šmejkal, J. Sinova and T. Jungwirth, Phys. Rev. X, 2022, 12, 040501 Search PubMed.
- I. Mazin and T. P. R. X. Editors, Phys. Rev. X, 2022, 12, 040002 CAS.
- I. Mazin, Physics, 2024, 17, 4 CrossRef.
- L. Šmejkal, A. B. Hellenes, R. González-Hernández, J. Sinova and T. Jungwirth, Phys. Rev. X, 2022, 12, 011028 Search PubMed.
- R. González-Hernández, L. Šmejkal, K. Výborný, Y. Yahagi, J. Sinova, T. Jungwirth and J. Železný, Phys. Rev. Lett., 2021, 126, 127701 CrossRef PubMed.
- I. I. Mazin, K. Koepernik, M. D. Johannes, R. González-Hernández and L. Šmejkal, Proc. Natl. Acad. Sci. U. S. A., 2021, 118, e2108924118 CrossRef CAS PubMed.
- R. D. Gonzalez Betancourt, J. Zubáč, R. Gonzalez-Hernandez, K. Geishendorf, Z. Šobáň, G. Springholz, K. Olejník, L. Šmejkal, J. Sinova, T. Jungwirth, S. T. B. Goennenwein, A. Thomas, H. Reichlová, J. Železný and D. Kriegner, Phys. Rev. Lett., 2023, 130, 036702 CrossRef CAS PubMed.
- S. Bhattacharjee, E. Bousquet and P. Ghosez, Phys. Rev. Lett., 2009, 102, 117602 CrossRef PubMed.
- Q. Si, R. Yu and E. Abrahams, Nat. Rev. Mater., 2016, 1, 16017 CrossRef CAS.
- L. Šmejkal, R. González-Hernández, T. Jungwirth and J. Sinova, Sci. Adv., 2020, 6, eaaz8809 CrossRef PubMed.
- Z. Feng, X. Zhou, L. Šmejkal, L. Wu, Z. Zhu, H. Guo, R. González-Hernández, X. Wang, H. Yan, P. Qin, X. Zhang, H. Wu, H. Chen, Z. Meng, L. Liu, Z. Xia, J. Sinova, T. Jungwirth and Z. Liu, Nat. Electron., 2022, 5, 735–743 CrossRef CAS.
- T. Tschirner, P. Keßler, R. D. Gonzalez Betancourt, T. Kotte, D. Kriegner, B. Büchner, J. Dufouleur, M. Kamp, V. Jovic, L. Šmejkal, J. Sinova, R. Claessen, T. Jungwirth, S. Moser, H. Reichlova and L. Veyrat, APL Mater., 2023, 11, 101103 CrossRef CAS.
- H. Bai, Y. C. Zhang, Y. J. Zhou, P. Chen, C. H. Wan, L. Han, W. X. Zhu, S. X. Liang, Y. C. Su, X. F. Han, F. Pan and C. Song, Phys. Rev. Lett., 2023, 130, 216701 CrossRef CAS PubMed.
- A. Bose, N. J. Schreiber, R. Jain, D. Shao, H. P. Nair, J. Sun, X. S. Zhang, D. A. Muller, E. Y. Tsymbal, D. G. Schlom and D. C. Ralph, Nat. Electron., 2022, 5, 267–274 CrossRef CAS.
- H. Bai, L. Han, X. Y. Feng, Y. J. Zhou, R. X. Su, Q. Wang, L. Y. Liao, W. X. Zhu, X. Z. Chen, F. Pan, X. L. Fan and C. Song, Phys. Rev. Lett., 2022, 128, 197202 CrossRef CAS PubMed.
- S. Karube, T. Tanaka, D. Sugawara, N. Kadoguchi, M. Kohda and J. Nitta, Phys. Rev. Lett., 2022, 129, 137201 CrossRef CAS PubMed.
- Y. Jiang, Z. Wang, K. Samanta, S. Zhang, R. Xiao, W. J. Lu, Y. P. Sun, E. Y. Tsymbal and D. Shao, Phys. Rev. B: Condens. Matter Mater. Phys., 2023, 108, 174439 CrossRef CAS.
- P. W. Anderson, Science, 1987, 235, 1196–1198 CrossRef CAS PubMed.
- H. Ma, M. Hu, N. Li, J. Liu, W. Yao, J. Jia and J. Liu, Nat. Commun., 2021, 12, 2846 CrossRef CAS PubMed.
- X. Chen, D. Wang, L. Li and B. Sanyal, Appl. Phys. Lett., 2023, 123, 022402 CrossRef CAS.
-
Z. Gao, S. Qu, B. Zeng, Y. Liu, J. Wen, H. Sun, P. Guo and Z. Lu, arXiv, 2023, preprint, arXiv:2311.04418 Search PubMed.
- J. Sødequist and T. Olsen, Appl. Phys. Lett., 2024, 124, 182409 CrossRef.
-
L. Bai, W. Feng, S. Liu, L. Šmejkal, Y. Mokrousov and Y. Yao, arXiv, 2024, preprint, arXiv:2406.02123 Search PubMed.
- J. Krempaský, L. Šmejkal, S. W. D'Souza, M. Hajlaoui, G. Springholz, K. Uhlířová, F. Alarab, P. C. Constantinou, V. Strocov, D. Usanov, W. R. Pudelko, R. González-Hernández, A. Birk Hellenes, Z. Jansa, H. Reichlová, Z. Šobáň, R. D. Gonzalez Betancourt, P. Wadley, J. Sinova, D. Kriegner, J. Minár, J. H. Dil and T. Jungwirth, Nature, 2024, 626, 517–522 CrossRef PubMed.
- Y. Zhu, X. Chen, X. Liu, Y. Liu, P. Liu, H. Zha, G. Qu, C. Hong, J. Li, Z. Jiang, X. Ma, Y. Hao, M. Zhu, W. Liu, M. Zeng, S. Jayaram, M. Lenger, J. Ding, S. Mo, K. Tanaka, M. Arita, Z. Liu, M. Ye, D. Shen, J. Wrachtrup, Y. Huang, R. He, S. Qiao, Q. Liu and C. Liu, Nature, 2024, 626, 523–528 CrossRef CAS PubMed.
- O. Fedchenko, J. Minár, A. Akashdeep, S. W. D'Souza, D. Vasilyev, O. Tkach, L. Odenbreit, Q. Nguyen, D. Kutnyakhov, N. Wind, L. Wenthaus, M. Scholz, K. Rossnagel, M. Hoesch, M. Aeschlimann, B. Stadtmüller, M. Kläui, G. Schönhense, T. Jungwirth, A. B. Hellenes, G. Jakob, L. Šmejkal, J. Sinova and H. Elmers, Sci. Adv., 2024, 10, eadj4883 CrossRef CAS PubMed.
- H. Zhou, J. R. Long and O. M. Yaghi, Chem. Rev., 2012, 112, 673–674 CrossRef CAS PubMed.
- J. A. Real, G. De Munno, M. C. Munoz and M. Julve, Inorg. Chem., 1991, 30, 2701–2704 CrossRef CAS.
- H. N. Bordallo, L. Chapon, J. L. Manson, J. Hernández-Velasco, D. Ravot, W. M. Reiff and D. N. Argyriou, Phys. Rev. B: Condens. Matter Mater. Phys., 2004, 69, 224405 CrossRef.
- H. Sun, B. Ma, S. Gao and G. Su, Chem. Commun., 2001, 37, 2586–2587 RSC.
- X. Li and J. Yang, J. Am. Chem. Soc., 2019, 141, 109–112 CrossRef CAS PubMed.
- P. Perlepe, I. Oyarzabal, A. Mailman, M. Yquel, M. Platunov, I. Dovgaliuk, M. Rouzières, P. Négrier, D. Mondieig, E. A. Suturina, M.-A. Dourges, S. Bonhommeau, R. A. Musgrave, K. S. Pedersen, D. Chernyshov, F. Wilhelm, A. Rogalev, C. Mathonière and R. Clérac, Science, 2020, 370, 587–592 CrossRef CAS PubMed.
- X. Li, H. Lv, X. Liu, T. Jin, X. Wu, X. Li and J. Yang, Sci. China: Chem., 2021, 64, 2212–2217 CrossRef CAS.
- H. Lv, X. Li, D. Wu, Y. Liu, X. Li, X. Wu and J. Yang, Nano Lett., 2022, 22, 1573–1579 CrossRef CAS PubMed.
- X. Li, Q. Liu, Y. Tang, W. Li, N. Ding, Z. Liu, H. Fu, S. Dong, X. Li and J. Yang, J. Am. Chem. Soc., 2023, 145, 7869–7878 CrossRef CAS PubMed.
- J. Sinova, S. O. Valenzuela, J. Wunderlich, C. H. Back and T. Jungwirth, Rev. Mod. Phys., 2015, 87, 1213–1260 CrossRef.
- M. Naka, S. Hayami, H. Kusunose, Y. Yanagi, Y. Motome and H. Seo, Nat. Commun., 2019, 10, 4305 CrossRef PubMed.
- Y. Luo, S. Bag, O. Zaremba, A. Cierpka, J. Andreo, S. Wuttke, P. Friederich and M. Tsotsalas, Angew. Chem., Int. Ed., 2022, 61, e202200242 CrossRef CAS PubMed.
- V. Wang, G. Tang, Y. Liu, R. Wang, H. Mizuseki, Y. Kawazoe, J. Nara and W. T. Geng, J. Phys. Chem. Lett., 2022, 13, 11581–11594 CrossRef CAS PubMed.
- K. H. Ahn, A. Hariki, K. W. Lee and J. Kuneš, Phys. Rev. B, 2019, 99, 184432 CrossRef CAS.
- H. Fujiwara, E. Fujiwara, Y. Nakazawa, B. Z. Narymbetov, K. Kato, H. Kobayashi, A. Kobayashi, M. Tokumoto and P. Cassoux, J. Am. Chem. Soc., 2001, 123, 306–314 CrossRef CAS PubMed.
- D. Litvin, Acta Crystallogr., Sect. A: Cryst. Phys., Diffr., Theor. Gen. Crystallogr., 1977, 33, 279–287 CrossRef.
- M. Milivojević, M. Orozović, S. Picozzi, M. Gmitra and S. Stavrić, 2D Mater., 2024, 11, 035025 CrossRef.
- B. Bradlyn, L. Elcoro, J. Cano, M. G. Vergniory, Z. Wang, C. Felser, M. I. Aroyo and B. A. Bernevig, Nature, 2017, 547, 298–305 CrossRef CAS PubMed.
- H. Watanabe, H. C. Po and A. Vishwanath, Sci. Adv., 2018, 4, eaat8685 CrossRef CAS PubMed.
- N. Nagaosa, J. Sinova, S. Onoda, A. H. MacDonald and N. P. Ong, Rev. Mod. Phys., 2010, 82, 1539–1592 CrossRef.
- W. Du, R. Peng, Z. He, Y. Dai, B. Huang and Y. Ma, npj 2D Mater. Appl., 2022, 6, 11 CrossRef CAS.
- Z. Zhuo, X. Wu and J. Yang, J. Am. Chem. Soc., 2016, 138, 7091–7098 CrossRef CAS PubMed.
- S. Bertolazzi, J. Brivio and A. Kis, ACS Nano, 2011, 5, 9703–9709 CrossRef CAS PubMed.
- L. Liu, C. Pai, Y. Li, H. W. Tseng, D. C. Ralph and R. A. Buhrman, Science, 2012, 336, 555–558 CrossRef CAS PubMed.
- C. Pai, L. Liu, Y. Li, H. W. Tseng, D. C. Ralph and R. A. Buhrman, Appl. Phys. Lett., 2012, 101, 122404 CrossRef.
- J. Qiao, J. Zhou, Z. Yuan and W. Zhao, Phys. Rev. B, 2018, 98, 214402 CrossRef CAS.
- R. G. Parr, Annu. Rev. Phys. Chem., 1983, 34, 631–656 CrossRef CAS.
- G. Kresse and J. Furthmüller, Comput. Mater. Sci., 1996, 6, 15–50 CrossRef CAS.
- G. Kresse and J. Furthmüller, Phys. Rev. B: Condens. Matter Mater. Phys., 1996, 54, 11169–11186 CrossRef CAS PubMed.
- S. Grimme, J. Antony, S. Ehrlich and H. Krieg, J. Chem. Phys., 2010, 132, 154104 CrossRef PubMed.
- S. Grimme, S. Ehrlich and L. Goerigk, J. Comput. Chem., 2011, 32, 1456–1465 CrossRef CAS PubMed.
- J. P. Perdew, K. Burke and M. Ernzerhof, Phys. Rev. Lett., 1996, 77, 3865–3868 CrossRef CAS PubMed.
- A. V. Krukau, O. A. Vydrov, A. F. Izmaylov and G. E. Scuseria, J. Chem. Phys., 2006, 125, 224106 CrossRef PubMed.
- P. E. Blöchl, Phys. Rev. B: Condens. Matter Mater. Phys., 1994, 50, 17953–17979 CrossRef PubMed.
- G. Kresse and D. Joubert, Phys. Rev. B: Condens. Matter Mater. Phys., 1999, 59, 1758–1775 CrossRef CAS.
- H. J. Monkhorst and J. D. Pack, Phys. Rev. B: Solid State, 1976, 13, 5188–5192 CrossRef.
-
R. Bader, Atoms in Molecules: A Quantum Theory, Oxford University Press, New York, 1990 Search PubMed.
- G. Henkelman, A. Arnaldsson and H. Jónsson, Comput. Mater. Sci., 2006, 36, 354–360 CrossRef.
- E. Sanville, S. D. Kenny, R. Smith and G. Henkelman, J. Comput. Chem., 2007, 28, 899–908 CrossRef CAS PubMed.
- W. Tang, E. Sanville and G. Henkelman, J. Phys.: Condens. Matter, 2009, 21, 084204 CrossRef CAS PubMed.
- M. Yu and D. R. Trinkle, J. Chem. Phys., 2011, 134, 064111 CrossRef PubMed.
- C. Zener, Phys. Rev., 1954, 96, 1335–1337 CrossRef CAS.
- Q. Wu, S. Zhang, H. Song, M. Troyer and A. A. Soluyanov, Comput. Phys. Commun., 2018, 224, 405–416 CrossRef CAS.
- A. A. Mostofi, J. R. Yates, G. Pizzi, Y. S. Lee, I. Souza, D. Vanderbilt and N. Marzari, Comput. Phys. Commun., 2014, 185, 2309–2310 CrossRef CAS.
- G. Pizzi, V. Vitale, R. Arita, S. Blügel, F. Freimuth, G. Géranton, M. Gibertini, D. Gresch, C. Johnson, T. Koretsune, J. Ibañez-Azpiroz, H. Lee, J. M. Lihm, D. Marchand, A. Marrazzo, Y. Mokrousov, J. I. Mustafa, Y. Nohara, Y. Nomura, L. Paulatto, S. Poncé, T. Ponweiser, J. Qiao, F. Thöle, S. S. Tsirkin, M. Wierzbowska, N. Marzari, D. Vanderbilt, I. Souza, A. A. Mostofi and J. R. Yates, J. Phys.: Condens. Matter, 2020, 32, 165902 CrossRef CAS PubMed.
- H. J. Kim, Zenodo, 2018, 1402592 Search PubMed.
- M. Iraola, J. L. Mañes, B. Bradlyn, M. K. Horton, T. Neupert, M. G. Vergniory and S. S. Tsirkin, Comput. Phys. Commun., 2022, 272, 108226 CrossRef CAS.
- P. Giannozzi, S. de Gironcoli, P. Pavone and S. Baroni, Phys. Rev. B: Condens. Matter Mater. Phys., 1991, 43, 7231–7242 CrossRef CAS PubMed.
- X. Gonze and C. Lee, Phys. Rev. B: Condens. Matter Mater. Phys., 1997, 55, 10355–10368 CrossRef CAS.
- A. Togo and I. Tanaka, Scr. Mater., 2015, 108, 1–5 CrossRef CAS.
- A. Togo, L. Chaput, T. Tadano and I. Tanaka, J. Phys.: Condens. Matter, 2023, 35, 353001 CrossRef CAS PubMed.
- A. Togo, J. Phys. Soc. Jpn., 2023, 92, 012001 CrossRef.
- S. Nosé, J. Chem. Phys., 1984, 81, 511–519 CrossRef.
- W. G. Hoover, Phys. Rev. A, 1985, 31, 1695–1697 CrossRef PubMed.
- S. Nosé, Prog. Theor. Phys. Suppl., 1991, 103, 1–46 CrossRef.
- D. M. Bylander and L. Kleinman, Phys. Rev. B: Condens. Matter Mater. Phys., 1992, 46, 13756–13761 CrossRef PubMed.
- V. Wang, N. Xu, J. Liu, G. Tang and W. Geng, Comput. Phys. Commun., 2021, 267, 108033 CrossRef CAS.
- Y. Zhang, B. Wang, Y. Guo, Q. Li and J. Wang, Comput. Mater. Sci., 2021, 197, 110638 CrossRef CAS.
|
This journal is © The Royal Society of Chemistry 2024 |