DOI:
10.1039/D4SC03111C
(Edge Article)
Chem. Sci., 2024,
15, 12598-12605
Nonplanar structure accelerates reverse intersystem crossing of TADF emitters: nearly 40% EQE and relieved efficiency roll off†
Received
13th May 2024
, Accepted 2nd July 2024
First published on 3rd July 2024
Abstract
Exploring strategies to enhance reverse intersystem crossing (RISC) is of great significance to develop efficient thermally activated delayed fluorescent (TADF) molecules. In this study, we investigate the substantial impact of nonplanar structure on improving the rate of RISC (kRISC). Three emitters based on spiroacridine donors are developed to evaluate this hypothesis. All molecules exhibit high photoluminescent quantum yields (PLQYs) of 96–98% due to their rigid donor and acceptor. Leveraging the synergistic effects of heavy element effect and nonplanar geometry, S2-TRZ exhibits an accelerated kRISC of 24.2 × 105 s−1 compared to the 11.1 × 105 s−1 of S1-TRZ, which solely incorporates heavy atoms. Additionally, O1-TRZ possesses a further lower kRISC of 9.42 × 105 s−1 because of the absence of these effects. Remarkably, owing to the high PLQYs and suitable TADF behaviors, devices based on these emitters exhibit state-of-the-art performance, including a maximum external quantum efficiency of up to 40.1% and maximum current efficiency of 124.7 cd A−1. More importantly, devices utilizing S2-TRZ as an emitter achieve a relieved efficiency roll-off of only 7% under 1000 cd m−2, in contrast to the 12% for O1-TRZ and 11% for S1-TRZ, respectively. These findings advance our fundamental understanding of TADF processes for high-performance electroluminescent devices.
1. Introduction
In the last few decades, thermally activated delayed fluorescent (TADF) emitters have emerged as highly promising materials for organic light emitting diodes (OLEDs) owing to their capability of boosting 75% triplet excitons to emit via reverse intersystem crossing (RISC) without the help of noble metals.1,2 The advent of multi-resonance (MR) type emitters, characterized by narrow band emission, has positioned them as a burgeoning option aligning well with BT.2020 standards, a wide color gamut standard for the next generation of high resolution displays.3–7 Nevertheless, MR emitters usually possess prolonged delayed fluorescence due to the large energy difference (ΔEST) between the first singlet (S1) and triplet excited state (T1), which impedes swift RISC processes. In response, sensitization techniques have been applied to augment the efficiency and durability of MR-emitter-based devices.8,9 These sensitizers play a critical role in effectively recycling all excitons, thereby offering a new opportunity for leveraging conventional donor–acceptor (D–A) type TADF compounds. Developing efficient D–A TADF emitters remains a paramount objective for realizing high performance OLEDs.
The primary task to craft an efficient TADF emitter lies in establishing a rapid RISC pathway, which necessitates a minimized ΔEST and a large spin–orbit coupling (SOC), as delineated by Fermi's golden rule.10–12 Various structural motifs, such as twisted D–A arrangements,13–19 through-space charge transfer (TSCT),20–24 or multi-resonance (MR) architectures,25–31 have successfully achieved diminutive ΔEST in pristine organic compounds. Indeed, the attainment of near-zero ΔEST has been achieved in several works, which propelled the rate of RISC (kRISC) to a maximum of 108 s−1.32 However, conventional TADF emitters encounter an intrinsic limitation in adjusting kRISC solely through ΔEST manipulation, as its minimum value is constrained to zero. To further enhance kRISC, attention must be directed to optimizing SOC. Hence, heavy elements such as sulfur (S),33–37 selenium (Se),38–42 or noble metals43–46 have been incorporated, which elevate the kRISC value and mitigate efficiency roll-off in devices. Notably, the introduction of heavy atoms will amplify the rate of intersystem crossing (kISC) simultaneously, which will compete with the radiative decay process and potentially compromise photoluminescent quantum yield (PLQY). Therefore, despite the advantage of Se, its utilization in D–A type TADF compounds remains limited due to the inherent suppression of radiative decay caused by the diminished overlap between frontier molecular orbitals (FMOs). Exploring alternative strategies to enhance SOC represents a promising avenue for the design of efficient TADF emitters.
Conformational twisting has long been recognized to trigger SOC in a conjugated π-electron system.47 Nonplanar molecules usually possess a larger kISC than their planar counterparts. The spin–orbit Hamiltonian in central-field approximation could be written as48
| 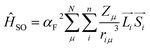 | (1) |
where
αF represents the fine-structure constant.
Zμ denotes the effective nuclear charge at nucleus
μ, and
S and
L are the spin and orbital angular momenta of electron
i, respectively. Notably, in coplanar molecules, the SOC between π–π* orbitals yields relatively small values, whereas the ‘out-of-plane’ component
Lz of the angular momentum operator
L contributes to a non-negligible SOC. This phenomenon has been observed in polymer systems as well.
49 Recently, twisting geometry has been applied in boosting phosphorescence emission in pure organic molecules.
50–53 SOC could be effectively improved by a nonplanar structure. Additionally, You
et al. reported that the nonplanar benzothiophene could accelerate
kRISC by reducing the Δ
EST.
54 Enlightened by these valuable experiences, the exploration of such strategies will provide a new perspective in assembling practical TADF emitters.
Herein, to validate this hypothesis, we designed and synthesized a new emitter, named 10-(4-(4,6-diphenyl-1,3,5-triazin-2-yl)phenyl)-2,7-dimethyl-10H-spiro[acridine-9,12′-benzo-[b]fluoreno[1,2-d]thiophene] (S2-TRZ), with a properly bent acridine (Fig. 1). The structurally fixed benzothiophene unit provides a substantial steric hindrance between the hydrogen atom and the acridine plane. Hence, a hooked acridine with a dihedral angle of 166° was acquired. In comparison, an isomer, 10-(4-(4,6-diphenyl-1,3,5-triazin-2-yl)phenyl)-2,7-dimethyl-10H-spiro[acridine-9,7′-benzo[b]fluoreno-[3,2-d]thiophene] (S1-TRZ), with the same benzothiophene substitution but a more planar acridine was assembled. Additionally, the oxygen (O) equipped analogs of S1-TRZ, 10-(4-(4,6-diphenyl-1,3,5-triazin-2-yl)phenyl)-2,7-dimethyl-10H-spiro[acridine-9,7′-fluoreno[2,3-b]benzofuran] (O1-TRZ), was also forged to unveil the effect of heavy atoms. All emitters exhibit high PLQY of 96–98%, relevant with the rigid D and A units. Benefiting from the synergy of the heavy element effect and nonplanar donor structure, S2-TRZ possesses the highest kRISC of 24.2 × 105 s−1. Without the nonplanar structure, S1-TRZ with a solely heavy element effect holds a moderate kRISC of 11.1 × 105 s−1. Furthermore, lacking the nonplanar structure of the sulfur-containing analogs, O1-TRZ displays a lower kRISC of 9.42 × 105 s−1. Notably, electroluminescent devices based on these emitters achieved a state-of-the-art external quantum efficiency (EQE) of 39.6% for S2-TRZ, 39.2% for S1-TRZ and even 40.1% for O1-TRZ thanks to the exceptional molecular properties. More importantly, S2-TRZ enables a significantly reduced efficiency roll-off of only 7% under 1000 cd m−2, outperforming both S1-TRZ (11%) and O1-TRZ (12%).
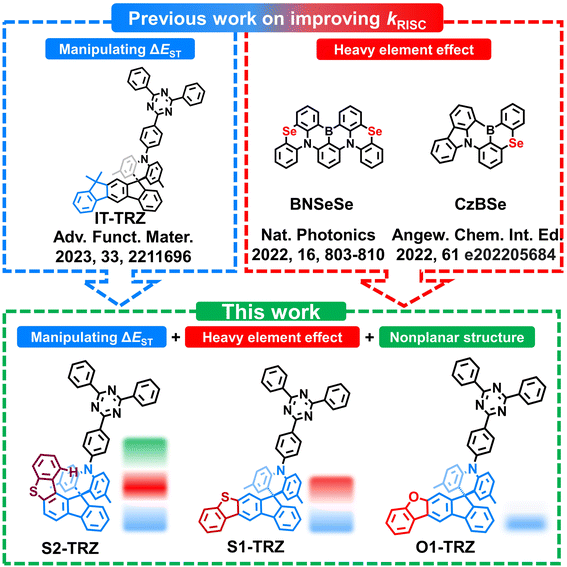 |
| Fig. 1 Comparison of the reported work on the methods of enhancing kRISC, molecular design and chemical structures of the related emitters. | |
2. Results and discussion
2.1. Molecular design, synthesis and thermal properties
To validate the impact of nonplanar structures on kRISC, a delicate molecular design is imperative. Nonplanar motifs within D or A groups may affect their electron-donating or -withdrawing capabilities, potentially hindering charge transfer (CT) interactions. In some cases, such motifs could lead to unfavorable conformations, such as the quasi-axial (QA) geometry, which is generally recognized to result in less efficient TADF properties. Thus, there arises a need for a building block composed of a fixed geometry with controllable modulation. Enlightened by our previous works, spiro-acridines featuring a rigid crisscross geometry could be an excellent platform to establish a model compound. To further reveal the insights for manipulating the TADF properties, the heavy element effect is also taken into consideration. Therefore, S2-TRZ, S1-TRZ and O1-TRZ are designed. All the compounds were synthesized via conventional Buchwald–Hartwig coupling between the bromo triazine acceptor and corresponding acridine donors (Scheme S1†). The donor parts were synthesized using a previously reported one-pot reaction method in good yields.55 All the final compounds were identified by 1H NMR, 13C NMR, mass spectra and elemental analysis. Excellent thermal stability is demonstrated with decomposition temperatures of 390–445 °C as evidenced by thermal gravimetric analysis (TGA) measurements (Fig. S1†). No obvious glass transition temperatures (Tgs) are detected in the range of 50–400 °C.
2.2. Theoretical simulation
To examine the properties of ground and excited states, density functional theory (DFT) and time dependent DFT (TD-DFT) were performed at the PBE0/def2svp level. As shown in Fig. 2 and S2,†S1-TRZ and O1-TRZ hold a more planar acridine unit with a dihedral angle of 173° and 177°. Conversely, S2-TRZ exhibits a twisted acridine with a dihedral angle of 166°, which is attributed to the spatially close hydrogen atom forming a constrained interaction with the acridine plane. These interactions could be identified using reduced density gradient (RDG) analysis (Fig. S3†). Strong van der Waals interactions (green isosurface) and large hindrance (brown isosurface) are clearly presented. Despite the enforced bending of acridine in S2-TRZ, the rigid crisscross structure prevents excessive deformation, favoring a quasi-equational (QE) geometry. In contrast, both S1-TRZ and O1-TRZ exhibit predictable QE patterns. In return, all emitters show large twisting between D and A with torsion angles of 85° for S2-TRZ, 88° for S1-TRZ and 87° for O1-TRZ, respectively. Hence, well separated highest occupied molecular orbitals (HOMOs) and lowest unoccupied molecular orbitals (LUMOs) are established in all three molecules. LUMOs predominantly reside on triazine with similar energy levels of −2.15 eV for S2-TRZ and −2.12 eV for both S1-TRZ and O1-TRZ, while HOMOs mostly spread on acridine units with comparable values of −5.27 eV for both S1-TRZ and O1-TRZ, and −5.31 eV for S2-TRZ. All S1 and T1 of the three compounds exhibit CT features as revealed by the distribution of their natural transition orbitals (NTOs). Taking S2-TRZ for example, ‘hole’ NTOs localized on acridine with a small portion on the spiro-junction, while ‘particle’ NTOs extend over the acceptor unit. The good separation of ‘hole’ and ‘particle’ NTOs suggests small ΔEST, leading to predicted values of 9 meV for S2-TRZ, 8 meV for S1-TRZ and 9 meV for O1-TRZ, respectively, which may facilitate a good TADF feature. To distinguish the effect of the hooked donor, the SOC constant was calculated to be 0.00545 cm−1 for S2-TRZ, 0.00073 cm−1 for S1-TRZ and 0.00011 cm−1 for O1-TRZ, respectively (Table 1). S1-TRZ exhibits a slightly higher SOC than O1-TRZ, hinting at a weak heavy element effect. In sharp comparison, S2-TRZ manifests a much larger SOC value than that of S1-TRZ, highlighting the pronounced impact of nonplanar structure tin enhancing SOC. Theoretical simulation suggests that these emitters could exhibit efficient TADF properties, and more encouragingly, the nonplanar donor could provide an effective enhancement on SOC.
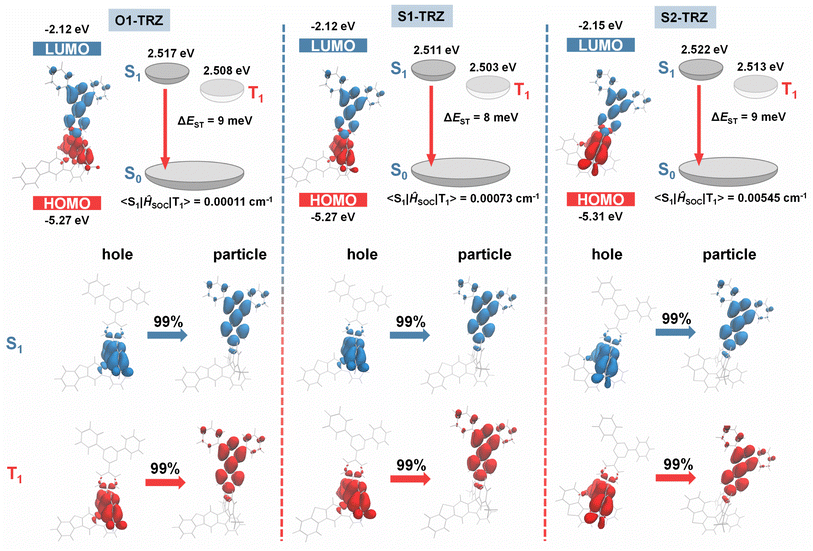 |
| Fig. 2 Theoretical simulated distribution and energy levels of HOMO/LUMO, and NTO distribution of the particle/hole of S1 and T1 of O1-TRZ, S1-TRZ and S2-TRZ at the PBE0/def2svp level. The figures are extracted using Multiwfn.56 | |
Table 1 Theoretical results and thermal properties of S2-TRZ, S1-TRZ and O1-TRZ
Compound |
HOMO (eV) |
LUMO (eV) |
S1 (eV) |
T1 (eV) |
ΔEST (meV) |
SOC (cm−1) |
T
d (°C) |
S2-TRZ
|
−5.31 |
−2.15 |
2.522 |
2.513 |
9 |
0.00545 |
445 |
S1-TRZ
|
−5.27 |
−2.12 |
2.511 |
2.503 |
8 |
0.00073 |
404 |
O1-TRZ
|
−5.27 |
−2.12 |
2.517 |
2.508 |
9 |
0.00011 |
390 |
2.3. Photophysical properties
Photophysical properties were assessed in dilute toluene solution (10−5 M) and doped films (10 wt% in dibenzo[b,d]furan-2,8-diylbis(diphenylphosphine oxide) (PPF)). As shown in Fig. 3, the intense absorption below 320 nm is assigned to the π–π* transition. The peaks between 320 and 370 nm are attributed to the n–π* transition of the donor. Different from O1-TRZ and S1-TRZ that exhibit structural and sharp peaks, S2-TRZ shows a broadened absorption in this region. To gain a deep insight into this phenomenon, we have recorded the absorption of the donor unit. As shown in Fig. S5,† the n–π* transition of these emitters fits well with the absorption of their donors, which confirms that these differences are related to the donor. It is reported that the molecular absorption becomes structureless and wide as the rigidity and planarity decrease.57 Therefore, the broadened n–π* transition of S2-TRZ could probably be attributed to the decrease of the planarity and rigidity of the acridine part, which confirms the bending of the acridine as revealed by theoretical analysis. The weak peaks at 410 nm originate from the CT absorption, which suggests the tight correlation between the D and A units. Structureless blueish green emissions around 504–513 nm are observed in doped films. The energy levels of S1 (ES1)/T1 (ET1) are calculated to be 2.72/2.67 eV for O1-TRZ, 2.69/2.63 eV for S1-TRZ and 2.73/2.67 eV for S2-TRZ, resulting in small ΔESTs values of 0.05, 0.06 and 0.06 eV, respectively. Moreover, Fl of these emitters was also recorded at room temperature. As shown in Fig. S4,†O1-TRZ and S1-TRZ show slightly redshifted spectra and smaller ES1 of 2.66 eV for O1-TRZ and 2.67 eV for S1-TRZ, respectively. Meanwhile, S2-TRZ exhibits the same ES1 at room temperature and 77 K, indicating S2-TRZ possesses less relaxation of S1 in films. Proper TADF is corroborated via transient PL measurement, revealing distinct prompt (PF) and DF components in the decay profiles (Fig. 3b). Fitting well with two-exponential decay, lifetimes of PF (τp)/DF (τd) are deduced to be 28 ns/2.1 μs, for O1-TRZ, 24 ns/1.7 μs for S1-TRZ and 25 ns/2.3 μs for S2-TRZ, respectively. And the ratios of PF/DF (Rp/Rd) are 48%/52% for O1-TRZ, 49%/51% for S1-TRZ and 15%/82% for S2-TRZ. The higher Rp/Rd may be attributed to the higher SOC value of S2-TRZ, which endows it with fast ISC and RISC processes. ISC competes with radiative decay and leads to more singlet excitons converting to triplet ones and enlarges the component of DF. Additionally, all emitters show high PLQY ranging from 96 to 98%. Rate constants are further calculated using the equations provided in the ESI† and summarized in Table 2. Compared to O1-TRZ, S1-TRZ exhibits a shortened τp and τd, demonstrating higher kr of 1.95 × 107 s−1 and kRISC of 11.1 × 105 s−1, respectively. This enhancement could be related to the introduction of S. Since S is introduced far from the D–A center, the heavy element effect could be weak as predicted by theoretical simulation. In contrast, S2-TRZ displays a DF-dominated TADF feature with Rp/Rd of 18%/82%, leading to significantly improved kRISC of 24.2 × 105 s−1. This substantial difference is attributed to the formation of the nonplanar donor, underscoring the significance of structural factors in TADF emitters. The HOMO energy levels of these compounds were determined using cyclic voltammetry (CV) and calculated to be 5.17 eV for S2-TRZ, 5.18 eV for S1-TRZ and 5.20 eV for O1-TRZ, respectively, suggesting that the nonplanar donor show minimal impact on electron donating ability. Thus, the enhanced kRISC is mainly contributed by the bended structure. Photophysical analysis confirms the formation of a nonplanar donor and the accelerated RISC channels as suggested by theoretical simulation.
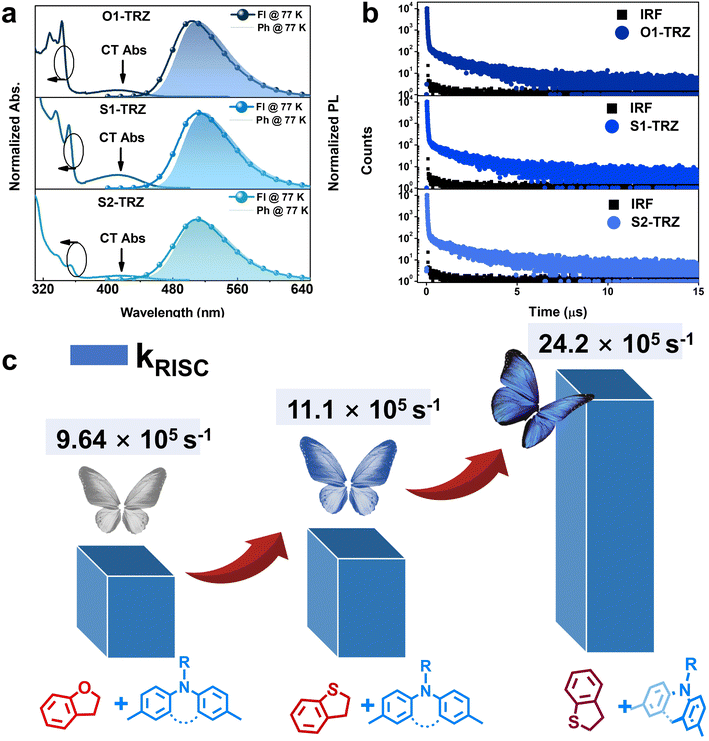 |
| Fig. 3 (a) Absorption in toluene (10−5 M), fluorescence (Fl) and phosphorescence (Ph) at 77 K in doped films (10 wt% in PPF) of O1-TRZ, S1-TRZ and S2-TRZ. (b) Transient PL decay in doped films (10 wt% in PPF) of O1-TRZ, S1-TRZ and S2-TRZ. (c) Comparison of kRISC and structure characteristics of O1-TRZ, S1-TRZ and S2-TRZ. | |
Table 2 TADF properties of O1-TRZ, S1-TRZ and S2-TRZ in doped films (10 wt% in PPF)
Emitter |
τ
p (ns) |
τ
d (μs) |
Φ
PL (%) |
Φ
p (%) |
Φ
d (%) |
k
r (107 s−1) |
k
ISC (107 s−1) |
k
RISC (105 s−1) |
k
nr (105 s−1) |
O1-TRZ
|
28 |
2.1 |
98 |
47 |
51 |
1.69 |
1.87 |
9.64 |
3.45 |
S1-TRZ
|
24 |
1.7 |
96 |
47 |
49 |
1.95 |
2.09 |
11.1 |
8.03 |
S2-TRZ
|
25 |
2.3 |
97 |
17 |
80 |
0.70 |
3.27 |
24.2 |
2.15 |
2.4. Electroluminescent properties
To evaluate the impact of such variation on electroluminescent (EL) properties, devices based on following structures of ITO/HATCN (5 nm)/TAPC (30 nm)/TCTA (15 nm)/mCBP (10 nm)/PPF:emitters (10–20 wt%, 25 nm)/PPF (10 nm)/ANT-BIZ (30 nm)/Liq (2 nm)/Al were fabricated. The corresponding EL profiles are listed in Fig. 4, S6† and Table 3. Initially, these emitters were examined at a doping concentration of 10 wt% to reduce the concentration-induced quenching. Single EL emission around 504–513 nm suggests the good confinement of excitons. All devices exhibit turn-on voltages of 3.2 V and high luminance exceeding 21
000 cd m−2. Maximum EQEs (EQEmaxs) of over 29.4% are achieved with the highest value of 34.1% in the O1-TRZ based device. Compared with O1-TRZ, the lower EQEmax of the S1-TRZ based device may be attributed to its lower PLQY. And the low EQEmax in the S2-TRZ based device could result from its low kr. Further probing the efficiency roll-off offers similar values of 6–8% under 100 cd m−2 and 26–29% under 1000 cd m−2, which are relatively large. The electron-withdrawing nature of PPF as the host matrix may have led to carrier imbalances in the emissive layer. Therefore, devices with concentration optimization were evaluated at 20 wt%. Remarkably, all devices achieve improved performance with a significantly enhanced luminance of 85
000 to 110
000 cd m−2 and increased EQEmax values of 39.6% for S2-TRZ, 39.2% for S1-TRZ and 40.1% for O1-TRZ. These results demonstrate their sufficient exciton utilization at a doping concentration of 20 wt%. Besides high PLQY and efficient TADF features, the excellent EL performances also benefit from high molecular horizontal dipole ratios, which will enhance the out-coupling efficiency. The p-polarized PL spectra are shown in Fig. S7,† suggesting that these emitters exhibit horizontal dipole ratios in the range of 80–97%. Such high values will greatly enhance the out-coupling efficiency and boost the EQE to nearly 40%. Notably, efficiency roll-off is substantially reduced with negligible roll-offs under 100 cd m−2. At a higher luminance level, O1-TRZ and S1-TRZ achieve similar roll-off of 12% and 11% under 1000 cd m−2, respectively, while S2-TRZ shows a small value of only 7%. Compared to O1-TRZ, S1-TRZ with slightly larger kRISC produces a comparable roll-off. S2-TRZ features a higher kRISC than S1-TRZ, experiencing a much gentler roll-off. The synergistic effect of the heavy element effect and nonplanar structure contributes to a significant enhancement of the RISC channel, facilitating the rapid consumption of excitons.
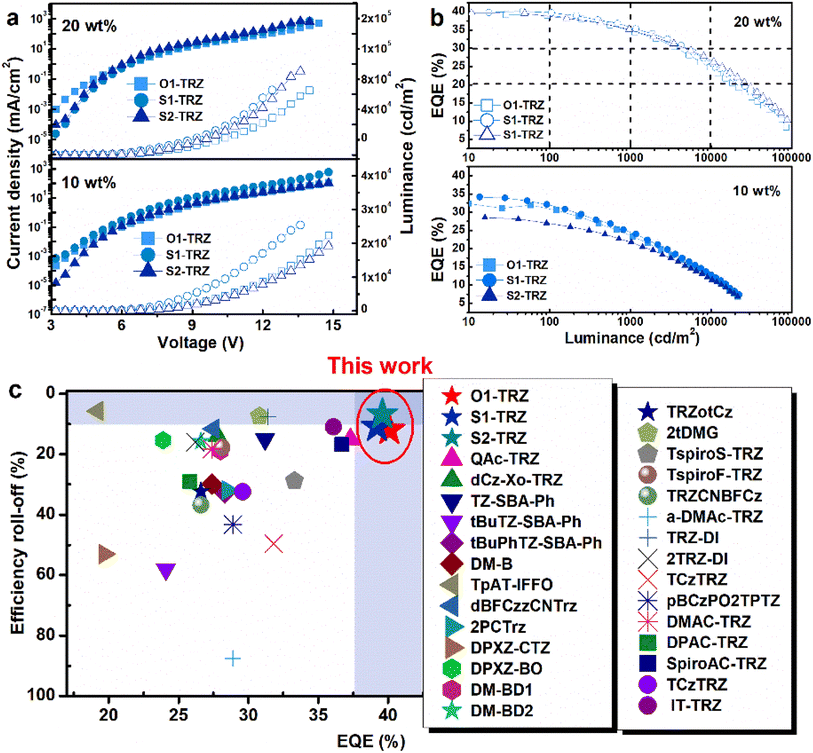 |
| Fig. 4 (a) Current density–voltage–luminance (J–V–L) profiles; (b) EQE vs. luminance characteristics. Inset of devices based on O1-TRZ, S1-TRZ and S2-TRZ at 10 and 20 wt% doping concentrations. (c) Comparison of EQE and efficiency roll-off under 1000 cd m−2 between reported emitters. | |
Table 3 EL data of O1-TRZ, S1-TRZ and S2-TRZ based devices at different doping concentrations
Emitting layer |
EL (nm) |
V
on (V) |
L
max (cd m−2) |
CEa (cd A−1) |
EQEa (%) |
Roll-offb (%) |
CIEc (x, y) |
Maximum values at 100/1000 cd m−2.
Efficiency roll-off under 100/1000 cd m−2.
CIE coordinates under 6 V.
|
O1-TRZ (10 wt%):PPF |
506 |
3.2 |
22 324 |
98.6/91.4/67.0 |
34.1/31.9/24.5 |
6/28 |
0.27, 0.50 |
S1-TRZ (10 wt%):PPF |
506 |
3.2 |
25 376 |
95.2/88.3/66.4 |
33.3/31.2/23.6 |
6/29 |
0.27, 0.50 |
S2-TRZ (10 wt%):PPF |
512 |
3.2 |
21 452 |
85.3/80.5/64.0 |
29.4/27.0/21.8 |
8/26 |
0.30, 0.53 |
O1-TRZ (20 wt%):PPF |
515 |
3.2 |
85 230 |
90.4/78.2/57.8 |
40.1/39.8/35.3 |
0.7/12 |
0.29, 0.53 |
S1-TRZ (20 wt%):PPF |
516 |
3.2 |
85 476 |
122.7/120.9/106.2 |
39.2/39.0/34.8 |
0.5/11 |
0.30, 0.54 |
S2-TRZ (20 wt%):PPF |
519 |
3.2 |
110 267 |
124.7/124.0/115.3 |
39.6/39.5/36.9 |
0.2/7 |
0.31, 0.55 |
3. Conclusion
In conclusion, we have disclosed the great impact of nonplanar structure on accelerating the RISC channel in TADF emitters. By combining cross-coupled spiroacridine and structurally fixed compulsory hindrance, S2-TRZ with a properly hooked acridine is successfully established. Analogs of S1-TRZ composed of a coplanar acridine and O1-TRZ with an O atom are designed to distinguish the effect of the nonplanar geometry and heavy element effect, respectively. All compounds exhibit high PLQYs of 96–98% and small ΔESTs of 8–9 meV, indicating excellent TADF behaviors. S2-TRZ with both heavy atoms and a nonplanar structure shows the highest kRISC of 24.2 × 105 s−1, whereas S1-TRZ possesses a lower kRISC of 11.1 × 105 s−1 due to the absence of a nonplanar acridine. Lacking these benefits, O1-TRZ displays the lowest kRISC of 9.64 × 105 s−1. Encouragingly, all emitters exhibit amazing EL performance with EQEmax of 39.6% for S2-TRZ, 39.2% for S1-TRZ and even 40.1% for O1-TRZ, respectively, alongside ultra-high luminance of up to 100
000 cd m−2. More importantly, S2-TRZ with a large value of kRISC exhibits the lowest roll-off of 7% under 1000 cd m−2, indicating the importance of the nonplanar structure in enhancing RISC for excellent EL performance. Overall, the effectiveness of nonplanar geometry in accelerating the RISC channel has been theoretically and experimentally validated. This strategy represents a valuable alternative to manipulating SOC besides the heavy atom effect. This work provides guidance for designing practical TADF emitters.
Data availability
All data supporting the findings of this study are presented in the article and ESI.† Additional data are available from the corresponding author upon reasonable request.
Author contributions
H. Liu designed the study. C. L. Yang supervised the project. Y. Liu and G. H. Chen performed the experiments. Y. Meng, H. Peng and J. S. Miao collected and analyzed the data. All the authors discussed the results and co-wrote the manuscript.
Conflicts of interest
There are no conflicts to declare.
Acknowledgements
This work was supported by the National Natural Science Foundation of China (No. 52373191 and 52130308), Natural Science Foundation of Guangdong Province (2023A1515030176), and the Shenzhen Science and Technology Program (ZDSYS20210623091813040, 20220809120915001). We thank the Instrumental Analysis Center of Shenzhen University for analytical support.
References
- H. Uoyama, K. Goushi, K. Shizu, H. Nomura and C. Adachi, Nature, 2012, 492, 234–238 CrossRef CAS PubMed
.
- Q. Zhang, B. Li, S. Huang, H. Nomura, H. Tanaka and C. Adachi, Nat. Photonics, 2014, 8, 326–332 CrossRef CAS
.
- Y. Kondo, K. Yoshiura, S. Kitera, H. Nishi, S. Oda, H. Gotoh, Y. Sasada, M. Yanai and T. Hatakeyama, Nat. Photonics, 2019, 13, 678–682 CrossRef CAS
.
- M. Yang, I. S. Park and T. Yasuda, J. Am. Chem. Soc., 2020, 142, 19468–19472 CrossRef CAS PubMed
.
- J. Liu, Y. Zhu, T. Tsuboi, C. Deng, W. Lou, D. Wang, T. Liu and Q. Zhang, Nat. Commun., 2022, 13, 4876 CrossRef CAS PubMed
.
- Y. Zou, J. H. Hu, M. X. Yu, J. S. Miao, Z. Y. Xie, Y. T. Qiu, X. S. Cao and C. L. Yang, Adv. Mater., 2022, 34, 2201442 CrossRef CAS PubMed
.
- X.-C. Fan, K. Wang, Y.-Z. Shi, Y.-C. Cheng, Y.-T. Lee, J. Yu, X.-K. Chen, C. Adachi and X.-H. Zhang, Nat. Photonics, 2023, 17, 280–285 CrossRef CAS
.
- S. O. Jeon, K. H. Lee, J. S. Kim, S.-G. Ihn, Y. S. Chung, J. W. Kim, H. Lee, S. Kim, H. Choi and J. Y. Lee, Nat. Photonics, 2021, 15, 208–215 CrossRef CAS
.
- C.-Y. Chan, M. Tanaka, Y.-T. Lee, Y.-W. Wong, H. Nakanotani, T. Hatakeyama and C. Adachi, Nat. Photonics, 2021, 15, 203–207 CrossRef CAS
.
- G. W. Robinson and R. P. Frosch, J. Chem. Phys., 1963, 38, 1187–1203 CrossRef CAS
.
- V. Lawetz, G. Orlandi and W. Siebrand, J. Chem. Phys., 1972, 56, 4058–4072 CrossRef CAS
.
- P. K. Samanta, D. Kim, V. Coropceanu and J. L. Bredas, J. Am. Chem. Soc., 2017, 139, 4042–4051 CrossRef CAS PubMed
.
- Y. Wada, H. Nakagawa, S. Matsumoto, Y. Wakisaka and H. Kaji, Nat. Photonics, 2020, 14, 643–649 CrossRef CAS
.
- W. Zeng, T. Zhou, W. Ning, C. Zhong, J. He, S. Gong, G. Xie and C. Yang, Adv. Mater., 2019, 31, e1901404 CrossRef PubMed
.
- T. A. Lin, T. Chatterjee, W. L. Tsai, W. K. Lee, M. J. Wu, M. Jiao, K. C. Pan, C. L. Yi, C. L. Chung, K. T. Wong and C. C. Wu, Adv. Mater., 2016, 28, 6976–6983 CrossRef CAS PubMed
.
- R. Pei, Y. Xu, J. Miao, H. Peng, Z. Chen, C. Zhou, H. Liu and C. Yang, Angew. Chem., Int. Ed., 2023, 135, 202217080 CrossRef
.
- W. Li, B. Li, X. Cai, L. Gan, Z. Xu, W. Li, K. Liu, D. Chen and S. J. Su, Angew. Chem., Int. Ed., 2019, 58, 11301–11305 CrossRef CAS PubMed
.
- L.-S. Cui, A. Gillett, S.-F. Zhang, H. Ye, Y. Liu, X.-K. Chen, Z.-S. Lin, E. Evans, W. Myers, T. Ronson, H. Nakanotani, S. Reineke, J. Bredas, C. Adachi and R. Friend, Nat. Photonics, 2020, 12, 636–642 CrossRef
.
- W. Han, J. Liu, C. Ran, Z. Huang, G. Gao, J. You and Z. Bin, Angew. Chem., Int. Ed., 2023, 62, e202312297 CrossRef CAS PubMed
.
- T. Huang, Q. Wang, G. Meng, L. Duan and D. Zhang, Angew. Chem., Int. Ed., 2022, 61, e202200059 CrossRef CAS PubMed
.
- X. J. Zheng, R. J. Huang, C. Zhong, G. H. Xie, W. M. Ning, M. L. Huang, F. Ni, F. B. Dias and C. L. Yang, Adv. Sci., 2020, 7, 1902087 CrossRef CAS PubMed
.
- C. Wu, W. Liu, K. Li, G. Cheng, J. Xiong, T. Teng, C. M. Che and C. Yang, Angew. Chem., Int. Ed., 2021, 60, 3994–3998 CrossRef CAS PubMed
.
- X. Tang, L. S. Cui, H. C. Li, A. J. Gillett, F. Auras, Y. K. Qu, C. Zhong, S. T. E. Jones, Z. Q. Jiang, R. H. Friend and L. S. Liao, Nat. Mater., 2020, 19, 1332–1338 CrossRef CAS PubMed
.
- S. Luo, J. Wang, N. Li, X. F. Song, X. Wan, K. Li and C. Yang, Angew. Chem., Int. Ed., 2023, 62, e202310943 CrossRef CAS PubMed
.
- Y. Xu, C. Li, Z. Li, Q. Wang, X. Cai, J. Wei and Y. Wang, Angew. Chem., Int. Ed., 2020, 59, 17442–17446 CrossRef CAS PubMed
.
- Z. Ye, H. Wu, Y. Xu, T. Hua, G. Chen, Z. Chen, X. Yin, M. Huang, K. Xu, X. Song, Z. Huang, X. Lv, J. Miao, X. Cao and C. Yang, Adv. Mater., 2023, 36, 2308314 CrossRef PubMed
.
- Y. Zhang, G. Li, L. Wang, T. Huang, J. Wei, G. Meng, X. Wang, X. Zeng, D. Zhang and L. Duan, Angew. Chem., Int. Ed., 2022, 61, e202202380 CrossRef CAS PubMed
.
- F. Liu, Z. Cheng, Y. Jiang, L. Gao, H. Liu, H. Liu, Z. Feng, P. Lu and W. Yang, Angew. Chem., Int. Ed., 2022, 61, e202116927 CrossRef CAS PubMed
.
- Y. Hu, M. Huang, H. Liu, J. Miao and C. Yang, Angew. Chem., Int. Ed., 2023, 62, e202312666 CrossRef CAS PubMed
.
- P. Jiang, J. Miao, X. Cao, H. Xia, K. Pan, T. Hua, X. Lv, Z. Huang, Y. Zou and C. Yang, Adv. Mater., 2021, e2106954 Search PubMed
.
- T. Hatakeyama, K. Shiren, K. Nakajima, S. Nomura, S. Nakatsuka, K. Kinoshita, J. Ni, Y. Ono and T. Ikuta, Adv. Mater., 2016, 28, 2777–2781 CrossRef CAS PubMed
.
- N. Aizawa, A. Matsumoto and T. Yasuda, Sci. Adv., 2021, 7, eabe5769 CrossRef CAS PubMed
.
- R. Braveenth, H. Lee, J. D. Park, K. J. Yang, S. J. Hwang, K. R. Naveen, R. Lampande and J. H. Kwon, Adv. Funct. Mater., 2021, 2105805 CrossRef CAS
.
- X. Xiong, Y.-C. Cheng, K. Wang, J. Yu and X.-H. Zhang, Mater. Chem. Front., 2023, 7, 929–936 RSC
.
- T. Hua, L. Zhan, N. Li, Z. Huang, X. Cao, Z. Xiao, S. Gong, C. Zhou, C. Zhong and C. Yang, Chem. Eng. J., 2021, 426, 131169 CrossRef CAS
.
- I. S. Park, K. Matsuo, N. Aizawa and T. Yasuda, Adv. Funct. Mater., 2018, 28, 1802031 CrossRef
.
- F. Chen, L. Zhao, X. Wang, Q. Yang, W. Li, H. Tian, S. Shao, L. Wang, X. Jing and F. Wang, Sci. China: Chem., 2021, 64, 547–551 CrossRef CAS
.
- Y. X. Hu, J. Miao, T. Hua, Z. Huang, Y. Qi, Y. Zou, Y. Qiu, H. Xia, H. Liu, X. Cao and C. Yang, Nat. Photonics, 2022, 16, 803–810 CrossRef CAS
.
- X. S. Cao, K. Pan, J. S. Miao, X. L. Lv, Z. Y. Huang, F. Ni, X. J. Yin, Y. X. Wei and C. L. Yang, J. Am. Chem. Soc., 2022, 144, 22976–22984 CrossRef CAS PubMed
.
- J. Jin, S. Wang, H. Jiang, L. Wang and W. Y. Wong, Adv. Opt. Mater., 2024, 2302354 CrossRef CAS
.
- Y. X. Hu, J. S. Miao, C. Zhong, Y. Zeng, S. L. Gong, X. S. Cao, X. Zhou, Y. Gu and C. L. Yang, Angew. Chem., Int. Ed., 2023, 62, e202302478 CrossRef CAS PubMed
.
- I. S. Park, H. Min and T. Yasuda, Angew. Chem., Int. Ed., 2022, 61, e202205684 CrossRef CAS PubMed
.
- D. Di, A. Romanov, L. Yang, J. M. Richter, J. P. H. Rivett, S. Jones, T. H. Thomas, M. A. Jalebi, R. H. Friend, M. Bochmann and D. Credgington, Science, 2017, 356, 159–163 CrossRef CAS PubMed
.
- J. Yang, X. Feng, N. Li, J. Li, X. Song, M. Li, G. Cui, J. Zhang, C. Jiang, C. Yang and K. Li, Sci. Adv., 2023, 9, eadh0198 CrossRef CAS PubMed
.
- X. Feng, J. G. Yang, J. Miao, C. Zhong, X. Yin, N. Li, C. Wu, Q. Zhang, Y. Chen, K. Li and C. Yang, Angew. Chem., Int. Ed., 2022, 61, e202209451 CrossRef CAS PubMed
.
- J. J. Wang, N. Q. Li, C. Zhong, J. S. Miao, Z. Y. Huang, M. X. Yu, Y. X. Hu, S. Luo, Y. Zou, K. Li and C. L. Yang, Adv. Mater., 2023, 35, 2208378 CrossRef CAS PubMed
.
- K. Schmidt, S. Brovelli, V. Coropceanu, D. Beljonne, J. Cornil, C. Bazzini, T. Caronna, R. Tubino, F. Meinardi, Z. Shuai and J.-L. Brédas, J. Phys. Chem. A, 2004, 111, 10490–10499 CrossRef PubMed
.
- S. K. Lower and M. A. El-Sayed, Chem. Rev., 1966, 66, 199–241 CrossRef CAS
.
- D. Beljonne, Z. Shuai, G. Pourtois and J. L. Bredas, J. Phys. Chem. A, 2001, 105, 3899–3907 CrossRef CAS
.
- H. Liu, Y. Gao, J. Cao, T. Li, Y. Wen, Y. Ge, L. Zhang, G. Pan, T. Zhou and B. Yang, Mater. Chem. Front., 2018, 2, 1853–1858 RSC
.
- G. Pan, Z. Yang, H. Liu, Y. Wen, X. Zhang, Y. Shen, C. Zhou, S.-T. Zhang and B. Yang, J. Phys. Chem. Lett., 2022, 13, 1563–1570 CrossRef CAS PubMed
.
- X. Yang, S. Wang, K. Sun, H. Liu, M. Ma, S. T. Zhang and B. Yang, Angew. Chem., Int. Ed., 2023, 62, e202306475 CrossRef CAS PubMed
.
- X. Cai, Z. Qiao, M. Li, X. Wu, Y. He, X. Jiang, Y. Cao and S. J. Su, Angew. Chem., Int. Ed., 2019, 58, 13522–13531 CrossRef CAS PubMed
.
- Y. Shi, G. Yang, B. Shen, Y. Yang, L. Yan, F. Yang, J. Liu, X. Liao, P. Yu, Z. Bin and J. You, J. Am. Chem. Soc., 2021, 143, 21066–21076 CrossRef CAS PubMed
.
- H. Liu, Z. W. Liu, G. G. Li, H. N. Huang, C. J. Zhou, Z. M. Wang and C. L. Yang, Angew. Chem., Int. Ed., 2021, 60, 12376–12380 CrossRef CAS PubMed
.
- T. Lu and F. Chen, J. Comput. Chem., 2012, 33, 580–592 CrossRef CAS PubMed
.
- N. I. Nijegorodov and W. S. Downey, J. Phys. Chem., 1994, 98, 5639–5643 CrossRef CAS
.
|
This journal is © The Royal Society of Chemistry 2024 |