DOI:
10.1039/D4SC03104K
(Edge Article)
Chem. Sci., 2024,
15, 11965-11971
Multi-electron redox reactivity of a samarium(II) hydrido complex†
Received
12th May 2024
, Accepted 14th June 2024
First published on 20th June 2024
Abstract
Well-defined low-valent molecular rare-earth metal hydrides are rare, and limited to Yb2+ and Eu2+ centers. Here, we report the first example of the divalent samarium(II) hydrido complex [(CpAr5)SmII(μ-H)(DABCO)]2 (4) (CpAr5 = C5Ar5, Ar = 3,5-iPr2-C6H3; DABCO = 1,4-diazabicyclooctane) supported by a super-bulky penta-arylcyclopentadienyl ligand, resulting from the hydrogenolysis of the samarium(II) alkyl complex [(CpAr5)SmII{CH(SiMe3)2}(DABCO)] (3). Complex 4 exhibits multi-electron redox reactivity toward a variety of substrates. Exposure of complex 4 to CO2 results in the formation of the trivalent samarium(III) mixed-bis-formate/carbonate complex [(CpAr5)SmIII(μ-η2:η1-O2CH)(μ-η2:η2-CO3)(μ-η1:η1-O2CH)SmIII(CpAr5)(DABCO)] (8), mediated by hydride insertion and reductive disproportionation reactions. Complex 4 shows four-electron reduction toward four equivalents of CS2 to afford the trivalent samarium(III) bis-trithiocarbonate complex [(CpAr5)SmIII(μ-η2:η2-CS3)(DABCO)]2 (9). A mechanistic study of the formation of complex 8 was carried out using DFT calculations.
Introduction
The chemistry of molecular hydrides of rare-earth metals has been extensively studied in the past four decades.1 However, despite their significant roles in various stoichiometric and catalytic reactions, such complexes are dominated by trivalent rare-earth metal centers.2 The difficulties in isolating discrete divalent rare-earth metal hydrides are mainly attributed to their greater ionic radii and higher reactivity levels, compared with trivalent rare-earth metals. So far, structurally characterized divalent rare-earth metal hydride species are scarce, consisting of a handful of ytterbium(II) hydrides3–9 and three examples of europium(II) hydride complexes,10 although all lanthanides(II), except for the radioactive promethium, are accessible in soluble molecules.11 The Takats group reported the first example of a divalent ytterbium hydride complex [(TptBu,Me)Yb(μ-H)]2 (TptBu,Me = hydrotris(3-tert-butyl-5-methyl-pyrazolyl)borate) (Fig. 1, I).3 The first mononuclear divalent ytterbium hydride complex bearing a terminal hydrido ligand—namely [(TpAd,iPr)Yb(H)(THF)] (TpAd,iPr = hydrotris(3-adamantyl-5-isopropyl-pyrazolyl)borate) (Fig. 1, II)—was produced.4 Three other ancillary ligand types (Fig. 1, III–VI) have been shown to stabilize neutral dimeric ytterbium hydrides, including the bulky ketiminate (BDIDipp = (2,6-iPr2C6H3)NC(Me)C(H)C(Me)N(2,6-iPr2C6H3)),5,6 amidinate (tBuC(N-Dipp)2 = (2,6-iPr2C6H3)NC(tBu)N(2,6-iPr2C6H3)),7 and β-diketiminato-based tetradentate ligand (MeC(NDipp)CHC(Me)NCH2CH2N(Me)CH2CH2NMe2, Dipp = 2,6-iPr2-C6H3).8 Recently, Okuda and co-worker disclosed the first examples of cationic divalent ytterbium hydrides [(Me4TACD)2Yb2(μ-H)(2+n)](2−n)+ (n = 0, 1) (Me4TACD = 1,4,7,10-tetramethyl-1,4,7,10-tetraazacyclododecane) (Fig. 1, VII).9 Very recently, the bulky β-diketiminate ligand frameworks {BDIDCHP}− and [BDIDipp/Ar]− (BDI = [HC{C(Me)2N-Dipp/Ar}2]− (Dipp = 2,6-iPr2-C6H3; Ar = 2,6-Cy2-C6H3 (DCHP) or 2,4,6-Cy3-C6H2 (TCHP)) were developed for the kinetic stabilization of the first europium(II) hydride complexes (Fig. 1, VIII).10
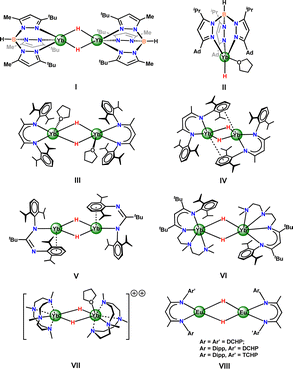 |
| Fig. 1 Selected examples of divalent rare-earth metal hydride complexes. | |
Divalent ytterbium hydrides have been used in a variety of organic transformations, and the oxidation state of ytterbium center remains unchanged in most cases.3–9,12 A more versatile chemistry can confidently be predicted for rare-earth metal hydrides with a highly reducing metal center.
Sm2+, Yb2+ and Eu2+ have been classically regarded as the three most common Ln2+ examples in molecular lanthanide complexes in solution.13 The Ln3+/Ln2+ redox potentials for Sm2+, Yb2+ and Eu2+ are, respectively, −1.55, −1.15 and −0.35 V versus NHE.14 Divalent samarium complexes, for example, SmIII2(THF)x (ref. 15) and (Cp*)2SmII(THF)x (Cp* = C5Me5),16 have been widely used as powerful electron-transfer reagents in various organic transformations. Compared with divalent ytterbium hydrides, the synthesis and structural characterization of divalent samarium hydrides are more challenging, because of the larger ionic radius (Yb2+ 1.02 Å, Sm2+ 1.17 Å; CN = 6),17 and more reducing metal center and paramagnetic nature (Yb2+: diamagnet; Sm2+: 3.5–3.8 μB) of divalent samarium.18 Molecular divalent samarium hydride complexes [SmIIH2(THF)2]19 and [(Cp*)SmIIH(THF)2]20 have been studied for decades, but no crystal structure has to date been obtained. Gambarotta et al. described an anionic divalent samarium hydride species, namely tetranuclear {([Ph2C(C4H3N)2]SmII)4(H)(THF)2}−, obtained serendipitously from the reduction of trivalent samarium precursor {[Ph2C(C4H3N)2]SmIIICl} with sodium in THF. However, the hydride position in the crystal structure is elusive.21 No further reactivity studies have been presented, as far as we know.
As the ionic radii of Sr2+ and Sm2+ are very similar (Sr2+ 1.18 Å, Sm2+ 1.17 Å; CN = 6),17 there are strikingly similar interatomic parameters in their crystal structures.22 Recently, our group found that the sterically demanding penta-arylcyclopentadienyl ligand CpAr5 (CpAr5 = C5Ar5, Ar = 3,5-iPr2-C6H3)23 can serve as an excellent ligand with the dinuclear structure.23a Encouraged by this success, we turned our attention to the synthesis of divalent samarium hydrides. Herein, we report the first example of a structural characterization of a neutral divalent samarium(II) hydrido complex, namely [(CpAr5)SmII(μ-H)(DABCO)]2 (4), generated from a moderate-pressure (20 atm) hydrogenolysis of the corresponding samarium(II) alkyl precursor. Preliminarily determined reactivities of divalent samarium(II) hydrides with Se, azobenzene (PhN
NPh), CO2, and CS2 are presented as well.
Results and discussion
As the precursor of reported strontium hydrides, the strontium alkyl complex [(CpAr5)Sr{CH(SiMe3)2}(THF)]23a was generated from the reaction of (CpAr5)H with [Sr{CH(SiMe3)2}2(THF)2].24 However, the samarium analogue “[SmII{CH(SiMe3)2}2(THF)2]” is inaccessible. Protonolysis reactions of (CpAr5)H with samarium benzyl complexes, for example [SmII2(CH2Ph)4(THF)3]25 and [(DMAT)2SmII(THF)] (DMAT = 2-Me2N-α-Me3Si-benzyl),24,26 failed. Thus, two-step salt metathesis reactions were carried out (Scheme 1). Treatment of (CpAr5)K(THF)2 (1) with [SmIII2(THF)5]27 in tetrahydropyran (THP), followed by the exchange of coordinated THP with DABCO, afforded the heteroleptic divalent samarium(II) iodide complex [(CpAr5)SmII(μ-I)(DABCO)]2 (2) as dark-green crystals in 92% isolated yield. Reaction of complex 2 with KCH(SiMe3)2 (ref. 28) in benzene gave the half-sandwich samarium(II) alkyl complex [(CpAr5)SmII{CH(SiMe3)2}(DABCO)] (3) as a dark-brown solid in 86% isolated yield. Due to the high solubility of complex 3 in hydrocarbon solvents such as hexane, no crystallographically definable product could be isolated.
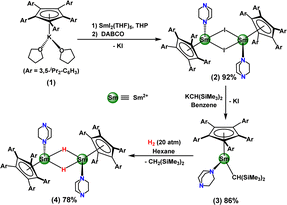 |
| Scheme 1 Synthesis of the divalent samarium(II) hydride 4. | |
Hydrogenolysis of complex 3 in hexane under 20 atm H2 successfully afforded the corresponding hydride complex [(CpAr5)SmII(μ-H)(DABCO)]2 (4) as dark-brown crystals in 78% isolated yield (Scheme 1), concomitant with CH2(SiMe3)2. Complex 4 was found to be slightly soluble in hexane, and quite soluble in benzene. Complex 4 decomposed completely in C6D6 within two days and converted quickly to samarocene [(CpAr5)2SmII] (5) in THF through ligand redistribution at room temperature. The molecular structure of complex 5 (ESI, Fig. S32†) was observed to be similar to those of other deca-arylsamarocenes [{C5(4-R-C6H4)5}2SmII] (R = Et, n-Bu).22,29
The 1H NMR spectrum of complex 4 showed very broad signals, attributed to the paramagnetic nature of the Sm2+ ion, and hence could not be fully assigned. However, a signal attributed to bridging hydrides was observed at 1.63 ppm, corroborated by a 2H NMR spectrum for the deuterated analogue [(CpAr5)SmII(μ-D)(DABCO)]2 (4-D). Based on magnetic measurements, the magnetic moments of complexes 2 and 4 at 300 K were measured to be 3.2 μB and 3.9 μB, respectively, consistent with divalent samarium derivatives.18
The dimeric nature of complex 4 in the solid state was revealed by X-ray crystallographic studies to be isostructural to [(CpAr5)Sr(μ-H)(DABCO)]2. Each Sm2+ center coordinated with one η5-CpAr5 ligand, one DABCO ligand and two bridging hydrido ligands. The bridging hydrido ligand H1 was located using difference Fourier syntheses and refined isotopically (Fig. 2). Analysis of the structure showed Sm1–N1 (2.652(4) Å), Sm1–Cent1 (2.594 Å) and Sm1–Sm1i (3.7966(5) Å) bond distances almost identical to those in [(CpAr5)Sr(μ-H)(DABCO)]2,23a and showed Sm–H distances, of 2.26(6) Å and 2.48(5) Å, in the range (2.26(5)–2.86(6) Å) found in the molecular strontium hydride complexes.23a,30 Thus, our work with complex 4 yielded the first example of a structurally characterized Sm(II) hydride complex, in comparison to the dozen previously reported examples of trivalent samarium(III) hydrides.31
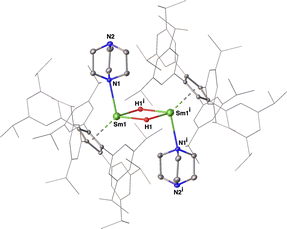 |
| Fig. 2 Molecular structure of complex 4. All the hydrogen atoms except the bridging hydrides (H1 and H1i) are omitted for clarity. Selected interatomic distances [Å] and angles [deg]: Sm1–H1 2.26(6), Sm1–N1 2.652 (4), Sm1–Cent1 2.594, Sm1–Sm1i 3.7966 (5), Sm1–H1–Sm1i 106 (2), H1–Sm1–H1i 74 (2). Cent: center of the CpAr5 ring. | |
As shown in Scheme 2, complex 4 readily reduced two equivalents of elemental Se in an apparent four-electron reduction to afford the corresponding trivalent samarium complex [(CpAr5)SmIII(μ-Se)(DABCO)]2 (6) in 77% isolated yield, with the four-electron reduction formally involving two one-electron hydride reductions and two one-electron Sm2+/Sm3+ reductions. Here, a distinct change in the color of the benzene solution from dark brown to pale brown was observed instantly, in company wih the release of H2.
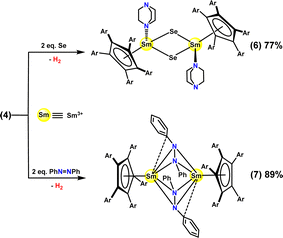 |
| Scheme 2 Reactions of complex 4 with Se and azobenzene. | |
Structural analyses showed the molecular framework of complex 6 to be similar to that of complex 2, except for the bridging atoms and the oxidation state of Sm centers (ESI, Fig. S30 and S33†), and showed the bond lengths of Sm–Se (2.7349(7)∼2.7524(6) Å) in complex 6 to be comparable to those in samarium complex [(Cp*)2SmIII(THF)]2(μ-Se) (2.782(1) and 2.779(1) Å).32
Complex 4 also apparently acted as a four-electron reductant in the reduction of 2 equivalents of PhN
NPh to give [(CpAr5)SmIII(μ-PhN-NPh)]2 (7) as a red-brown solid in a measured 89% isolated yield. As shown in Fig. S34,† the N1–N2 bond length (1.462(3) Å) in the [PhN-NPh]2− unit was found to be comparable to that of the previously reported samarium(III) complex [(Cp*)SmIII(μ-PhN-NPh)(THF)]2 (1.44(1) Å).33a In contrast, Evans and coworkers reported the reductive cleavage of azobenzene into uranium(VI) bis(imido) complex (Cp*)2U(NPh)2.33b
Rare-earth metal complexes have been found to engage in extensive chemistry with carbon dioxide (CO2)34 and carbon disulfide (CS2).35 Exposure of a solution of complex 4 in toluene to 1 atm of CO2 resulted in a decolorization to pale yellow in 30 minutes. The trivalent-samarium mixed-bis-formate/carbonate complex [(CpAr5)SmIII(μ-η2:η1-O2CH)(μ-η2:η2-CO3)(μ-η1:η1-O2CH)SmIII(CpAr5)(DABCO)] (8) was isolated from a hexane solution as pale-yellow crystals in 75% yield (Scheme 3).
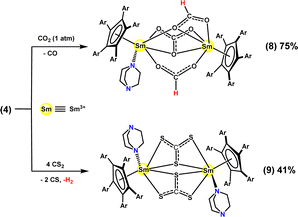 |
| Scheme 3 Reactions of complex 4 with CO2 and CS2. | |
Due to the paramagnetic nature of complex 8, no meaningful data could be obtained from its 1H and 13C NMR spectra. Accordingly, the solid-state structure of compound 8 was determined using X-ray crystallography and is highlighted in Fig. 3. Inspection of this structure showed two [(CpAr5)SmIII] fragments bridged by one carbonate [CO3]2− ligand and two formate [O2CH]− moieties. It specifically showed the bridging carbonate [CO3]2− unit symmetrically bonded to the metal centers in a μ-η2:η2 mode, with similar Sm–O bond distances (Sm1–O3, 2.397(3) Å; Sm2–O3, 2.398(3) Å) and Sm–O–C bond angles (Sm1–O3–C1, 88.2(3)°; Sm2–O3–C1, 91.0(3)°)—but showed unequal C–O bond lengths in the carbonate unit, specifically with C1–O1 (1.204(6) Å) and C1–O2 (1.251(6) Å) being significantly shorter than C1–O3 (1.349(8) Å). Two different coordination patterns for the [O2CH]− moieties were observed: one being a μ-η1:η1 mode, and the other a μ-η2:η1 mode, different from previously reported rare-earth metal formate complexes.36 The coordination sphere of Sm1 was completed by a DABCO ligand, to have the same coordination number as that of the Sm2 center. Other interatom bond distances, e.g., Sm1–N1 (2.605(3) Å) and Sm–Cent (2.523 and 2.452 Å), were found to be in agreement with trivalent samarium(III) complexes 6 and 7.
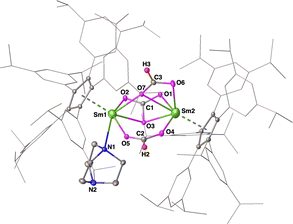 |
| Fig. 3 Molecular structure of complex 8. All the hydrogen atoms, except H2 and H3, are omitted for clarity. Selected interatomic distances [Å] and angles [deg]: Sm1–O2 2.354(2), Sm1–O3 2.397(3), Sm1–O5 2.338(2), Sm1–O7 2.426(2), Sm1–N1 2.605(3), Sm2–O1 2.373(3), Sm2–O3 2.398(3), Sm2–O4 2.346(2), Sm2–O6 2.406(3), Sm2–O7 2.644(2), C1–O1 1.251 (6), C1–O2 1.204(6), C1–O3 1.349(8), C2–O4 1.248(7), C2–O5 1.206(7), C3–O6 1.249(5), C3–O7 1.271(4), Sm1–Cent1 2.523, Sm2–Cent2 2.453, O2–C1–O1 133.6(6), O2–C1–O3 112.1(5), O1–C1–O3 113.8(5), O5–C2–O4 133.1(7), O6–C3–O7 121.7(4). Cent: center of the CpAr5 ring. | |
Apparently, four equivalents of CO2 were involved in this reaction. Two CO2 molecules inserted into Sm–H bonds to form two formate units with different bonding modes. The other two CO2 molecules likely underwent two-electron reduction and disproportionation to generate carbonate species [CO3]2− with the release of carbon monoxide (CO), which has been proposed in the formation of other rare-earth metal carbonate complexes.37
Treatment of complex 4 with CS2 in toluene gave a red-brown solution in 30 minutes. Trivalent samarium bis-trithiocarbonate complex [(CpAr5)SmIII(μ-η2:η2-CS3)(DABCO)]2 (9) was isolated as red-brown crystals in 41% yield. The whole molecule was determined according to X-ray crystallographic studies to adopt a binuclear structure composed of two CpAr5-supported samarium trithiocarbonate units (Fig. 4), with each Sm3+ center coordinated with one η5-CpAr5 ligand, one DABCO ligand and two bridging μ-η2:η2-CS3 ligands. It was obvious that this reaction occurred with the oxidation of both Sm(II) and hydrido centers, and the reductive disproportionation of four equivalents of CS2 to give two trithiocarbonate [CS3]2− units with the release of carbon monosulfide (CS). Note the generation of [CS3]2− species through a two-electron reduction in complex 4 being comparable to that previously reported for a rare-earth metal trithiocarbonate complex.38
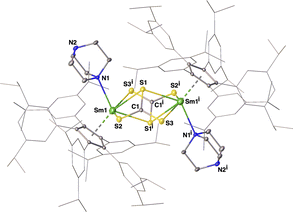 |
| Fig. 4 Molecular structure of complex 9. All the hydrogen atoms are omitted for clarity. Selected interatomic distances [Å] and angles [deg]: Sm1–S1 2.9774(8), Sm1–S2 2.8243(8), Sm1–S3i 2.8393(8), C1–S1 1.726(3), C1–S2 1.698(3), C1–S3 1.705(3), Sm1–N1 2.581(3), Sm1–Cent1 2.518, S1–C1–S2 118.35(17), S1–C1–S3 119.28(17), S2–C1–S3 122.29(19). Cent1: center of the CpAr5 ring. | |
In order to gain more insights into the previously unobserved reactivity of complex 4 toward CO2, DFT calculations (B3PW91) were carried out (Fig. 5). According to these calculations, the reaction begins with the insertion of a CO2 molecule into a Sm–H bond, viaTS1 and an activation barrier of 12.2 kcal mol−1, a value typical of an insertion of a double-bond into to a Ln–H bond. The reaction then yields a very stable formate-hydride di-samarium(II) intermediate (Int2), with the formate ligand in this complex μ2-bonded to the two Sm centers. A second CO2 molecule is readily inserted into this intermediate with a very low barrier (5.1 kcal mol−1) to form the bisformate di-samarium(II) intermediate (Int3), calculated to be thermodynamically highly stable (−113.4 kcal mol−1). Int3 then reacts with a third molecule of CO2 in an expected redox fashion. Indeed, each of the two Sm(II) centers undergoes a single electron transfer (SET) to CO2, which becomes doubly reduced, and each Sm center becomes oxidized to +III. This intermediate (Int4), usually called the “key intermediate” in SET reactions, adopts a dioxocarbene form as previously reported by Paparo et al. in titanium chemistry.39 This step is slightly endothermic, by 5.7 kcal mol−1, so that Int4 is expected to be quite reactive. Thus, Int4 readily reacts with a fourth molecule of CO2, with this reaction implying the release of one DABCO molecule to allow CO2 to bind to one Sm(III) center (Int5). This ligand exchange is endothermic by 9.9 kcal mol−1 from Int4, that is 15.6 kcal mol−1 from Int3. Then from Int5, a C–O bond formation occurs between the doubly reduced CO2 and the other CO2 molecule (bonded to Sm) viaTS3—with an associated barrier of 12.1 kcal mol−1 from Int5, that is 27.7 kcal mol−1 from Int3, in line with a kinetically accessible reaction. Finally according to the calculations, the formation of the C–O bond allows the formation of the carbonate ligand and the release of a CO molecule, as observed in complex 8, with the formation of this complex being highly exothermic (−131.6 kcal mol−1).
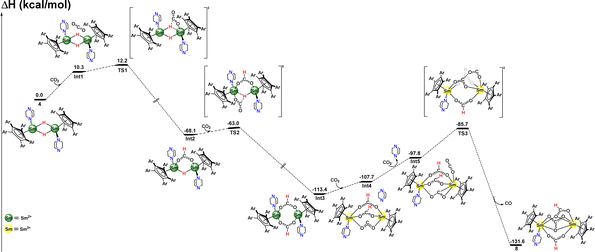 |
| Fig. 5 Computed enthalpy profile for the reaction of 4 with four equivalents of CO2 at room temperature. The energies are given in kcal mol−1. | |
Conclusions
In summary, we have demonstrated a synthesis, isolation and structural characterization of dimeric divalent samarium hydrido complex 4 bearing a super-bulky penta-arylcyclopentadienyl ligand. Complex 4 showed four-electron reduction toward various substrates, including Se, PhN
NPh and CS2, to afford the corresponding double Se2−, [PhN-NPh]2−, and [CS3]2− bridged trivalent samarium complexes, respectively, without suffering from ligand loss or ligand scrambling. For comparison, hydride insertion and two-electron reductive disproportionation of CO2 were observed in the formation of mixed-bis-formate/carbonate complex 8, corroborated by DFT calculations. These preliminarily determined reactivities indicate that complex 4 can provide an entry into an exciting new area involving the cooperation of a highly reactive hydride and a strong reducing metal center.
Data availability
All data (experimental procedures and characterization) that support the findings of this study are available within the article and its ESI.† Crystallographic data for complexes 2, 4–9 have been deposited with the Cambridge Crystallographic Data Centre under CCDC 2104460–2104466.
Author contributions
X. S. carried out the synthesis and characterization of complexes 1–9. P. D. assisted in the NMR spectroscopy experiment and crystal structure analysis. T. R. and L. M. performed the theoretical calculations. J. C. conceived this project and provided guidance. The manuscript was written with contributions from all the authors.
Conflicts of interest
The authors declare no conflict of interest.
Acknowledgements
This work was supported by the National Natural Science Foundation of China (No. 21901238 and 22271272). L. M. is a senior member of the Institut Universitaire de France. The Chinese Academy of Science is acknowledged for financial support (LM) and CalMip for a generous grant of computing time.
Notes and references
-
(a) M. Ephritikhine, Chem. Rev., 1997, 97, 2193 CrossRef CAS PubMed;
(b)
F. T. Edelmann in Comprehensive Organometallic Chemistry III, ed. R. H. Crabtree and D. M. P. Mingos, Elsevier, Oxford, 2006, vol. 4.01, p. 1 Search PubMed;
(c) M. Nishiura and Z. Hou, Nat. Chem., 2010, 2, 257 CrossRef CAS PubMed;
(d) A. A. Trifonov and D. M. Lyubov, Coord. Chem. Rev., 2017, 340, 10 CrossRef CAS;
(e)
T. Shima and Z. Hou, in Recent Development in Clusters of Rare Earths and Actinides: Chemistry and Materials, ed. Z. Zheng, Springer, Berlin, Heidelberg, 2017, p. 315 Search PubMed;
(f) W. J. Evans, J. H. Meadows, A. L. Wayda, W. E. Hunter and J. L. Atwood, J. Am. Chem. Soc., 1982, 104, 2008 CrossRef CAS.
- Recent reviews of trivalent rare-earth metal hydrides, see:
(a) Z. Hou, M. Nishiura and T. Shima, Eur. J. Inorg. Chem., 2007, 2535 CrossRef CAS;
(b) M. Konkol and J. Okuda, Coord. Chem. Rev., 2008, 252, 1577 CrossRef CAS;
(c) A. A. Trifonov, Coord. Chem. Rev., 2010, 254, 1327 CrossRef CAS;
(d) W. Fegler, A. Venugopal, M. Kramer and J. Okuda, Angew. Chem., Int. Ed., 2015, 54, 1724 CrossRef CAS PubMed;
(e) J. Cheng, K. Saliu, M. J. Ferguson, R. McDonald and J. Takats, J. Organomet. Chem., 2010, 695, 2696 CrossRef CAS;
(f) J. Okuda, Coord. Chem. Rev., 2017, 340, 2 CrossRef CAS.
-
(a) G. M. Ferrence, R. McDonald and J. Takats, Angew. Chem., Int. Ed., 1999, 38, 2233 CrossRef CAS;
(b) G. M. Ferrence and J. Takats, J. Organomet. Chem., 2002, 647, 84 CrossRef CAS.
-
(a) X. Shi, P. Deng, T. Rajeshkumar, L. Zhao, L. Maron and J. Cheng, Chem. Commun., 2021, 57, 10047 RSC;
(b) X. Shi, T. Rajeshkumar, L. Maron and J. Cheng, Chem. Commun., 2022, 58, 1362 RSC.
- C. Ruspic, J. Spielmann and S. Harder, Inorg. Chem., 2007, 46, 5320 CrossRef CAS PubMed.
-
(a) G. M. Richardson, I. Douair, S. A. Cameron, J. Bracegirdle, R. A. Keyzers, M. S. Hill, L. Maron and M. D. Ankder, Nat. Commun., 2021, 12, 3147 CrossRef CAS PubMed;
(b) G. M. Richardson, I. Douair, S. A. Cameron, L. Maron and M. D. Ankder, Chem.–Eur. J., 2021, 27, 13144 CrossRef CAS PubMed;
(c) G. M. Richardson, J. Howarth, M. J. Evans, A. J. Edwards, S. A. Cameron and M. D. Anker, J. Coord. Chem., 2022, 75, 1954 CrossRef CAS.
-
(a) I. V. Basalov, D. M. Lyubov, G. K. Fukin, A. S. Shavyrin and A. A. Trifonov, Angew. Chem., Int. Ed., 2012, 51, 3444 CrossRef CAS;
(b) I. V. Basalov, D. M. Lyubov, G. K. Fukin, A. V. Cherkasov and A. A. Trifonov, Organometallics, 2013, 32, 1507 CrossRef CAS;
(c) D. M. Lyubov, I. V. Basalov, A. S. Shavyrin, A. V. Cherkasov and A. A. Trifonov, Russ. J. Coord. Chem., 2019, 45, 728 CrossRef CAS;
(d) D. O. Khristolyubov, D. M. Lyubov and A. A. Trifonov, Russ. Chem. Rev., 2021, 90, 529 CrossRef.
-
(a) Q. Wen, B. Feng, L. Xiang, X. Leng and Y. Chen, Inorg. Chem., 2021, 60, 13913 CrossRef CAS PubMed;
(b) Q. Wen, T. Rajeshkumar, L. Maron, X. Leng and Y. Chen, Angew. Chem., Int. Ed., 2022, 61, e202200540 CrossRef CAS.
- D. Schuhknecht, K. N. Truong, T. P. Spaniol, L. Maron and J. Okuda, Chem. Commun., 2018, 54, 11280 RSC.
- G. M. Richardson, M. J. Evans, T. Rajeshkumar, J. A. J. McCone, S. A. Cameron, L. Maron, C. Jones and M. D. Anker, Chem.–Eur. J., 2024, e202400681 CrossRef CAS PubMed.
- M. R. MacDonald, J. E. Bates, J. W. Ziller, F. Furche and W. J. Evans, J. Am. Chem. Soc., 2013, 135, 9857 CrossRef CAS PubMed.
-
(a) Y. Shi, J. Li and C. Cui, Dalton Trans., 2017, 46, 10957 RSC;
(b) X. Liu, L. Xiang, E. Louyriac, L. Maron, X. Leng and Y. Chen, J. Am. Chem. Soc., 2019, 141, 138 CrossRef CAS PubMed.
- W. J. Evans, Organometallics, 2016, 35, 3088 CrossRef CAS.
- W. J. Evans, Coord. Chem. Rev., 2000, 206–207, 263 CrossRef CAS.
-
(a)
D. J. Procter, R. A. I. I. Flowers and T. Skrydstrup, Organic Synthesis using Samarium Diiodide: A Practical Guide, RSC Publishing, Cambridge, 2009 Search PubMed;
(b) K. Gopalaiah and H. B. Kagan, Chem. Rec., 2013, 13, 187 CrossRef CAS PubMed;
(c) M. Szostak, N. J. Fazakerley, D. Parmar and D. J. Procter, Chem. Rev., 2014, 114, 5959 CrossRef CAS PubMed.
-
(a) W. J. Evans, I. Bloom, W. E. Hunter and J. L. Atwood, J. Am. Chem. Soc., 1981, 103, 6507 CrossRef CAS;
(b) W. J. Evans, L. A. Hughes and T. P. Hanusa, J. Am. Chem. Soc., 1984, 106, 4270 CrossRef CAS;
(c) W. J. Evans, Inorg. Chem., 2007, 46, 3435 CrossRef CAS;
(d) C. Schoo, S. Bestgen, A. Egeberg, J. Seibert, S. N. Konchenko, C. Feldmann and P. W. Roesky, Angew. Chem., Int. Ed., 2019, 58, 4386 CrossRef CAS;
(e)
A. J. Ryan and W. J. Evans, in Structure and Bonding, ed. D. M. P. Mingos, 2019, vol. 181, p. 197, and references therein Search PubMed.
- R. D. Shannon, Acta Crystallogr., Sect. A: Cryst. Phys., Diffr., Theor. Gen. Crystallogr., 1976, 32, 751 CrossRef.
- W. J. Evans and M. A. Hozbor, J. Organomet. Chem., 1987, 326, 299 CrossRef CAS.
- E. A. Fedorova, A. A. Trifonov, E. N. Kirillov and M. N. Bochkarev, Russ. Chem. Bull., 2000, 49, 946 CrossRef CAS.
- W. J. Evans, I. Bloom, W. E. Hunter and J. L. Atwood, Organometallics, 1985, 4, 112 CrossRef CAS.
- T. Dube, M. Ganesan, S. Conoci, S. Gambarotta and G. P. A. Yap, Organometallics, 2000, 19, 3716 CrossRef CAS.
-
(a) S. Harder, Angew. Chem., Int. Ed., 2004, 43, 2714 CrossRef CAS PubMed;
(b) L. Orzechowski, D. F. J. Piesik, C. Ruspic and S. Harder, Dalton Trans., 2008, 4742 RSC;
(c) S. Harder, D. Naglav, C. Ruspic, C. Wickleder, M. Adlung, W. Hermes, M. Eul, R. Pottgen, D. B. Rego, F. Poineau, K. R. Czerwinski, R. H. Herber and I. Nowik, Chem.–Eur. J., 2013, 19, 12272 CrossRef CAS PubMed.
-
(a) X. Shi, G. Qin, Y. Wang, L. Zhao, Z. Liu and J. Cheng, Angew. Chem., Int. Ed., 2019, 58, 4356 CrossRef CAS PubMed;
(b) Y. Wang and J. Cheng, New J. Chem., 2020, 44, 17333 RSC;
(c) Y. Wang, I. K. Del, G. Qin, L. Zhao, L. Maron, X. Shi and J. Cheng, Chem. Commun., 2021, 57, 7766 RSC;
(d) L. Zhao, P. Deng, X. Gong, X. Kang and J. Cheng, ACS Catal., 2022, 12, 7877 CrossRef CAS.
- M. R. Crimmin, A. G. M. Barrett, M. S. Hill, D. J. MacDougall, M. F. Mahon and P. A. Procopiou, Chem.–Eur. J., 2008, 14, 11292 CrossRef CAS PubMed.
- B. M. Wolf, C. Stuhl and R. Anwander, Chem. Commun., 2018, 54, 8826 RSC.
- N. J. C. van Velzen and S. Harder, Organometallics, 2018, 37, 2263 CrossRef CAS.
-
(a) P. Girard, J. L. Namy and H. B. Kagan, J. Am. Chem. Soc., 1980, 102, 2693 CrossRef CAS;
(b) W. J. Evans, T. S. Gummersheimer and J. W. Ziller, J. Am. Chem. Soc., 1995, 117, 8999 CrossRef CAS.
- P. B. Hitchcock, A. V. Khvostov and M. F. Lappert, J. Organomet. Chem., 2002, 663, 263 CrossRef CAS.
- C. Ruspic, J. R. Moss, M. Schurmann and S. Harder, Angew. Chem., Int. Ed., 2008, 47, 2121 CrossRef CAS PubMed.
-
(a) B. Maitland, M. Wiesinger, J. Langer, G. Ballmann, J. Pahl, H. Elsen, C. Färber and S. Harder, Angew. Chem., Int. Ed., 2017, 56, 11880 CrossRef CAS PubMed;
(b) C. N. de Bruin-Dickason, T. Sutcliffe, C. A. Lamsfus, G. B. Deacon, L. Maron and C. Jones, Chem. Commun., 2018, 54, 786 RSC;
(c) D. Mukherjee, T. Hollerhage, V. Leich, T. P. Spaniol, U. Englert, L. Maron and J. Okuda, J. Am. Chem. Soc., 2018, 140, 3403 CrossRef CAS PubMed;
(d) B. Rösch, T. X. Gentner, H. Elsen, C. A. Fischer, J. Langer, M. Wiesinger and S. Harder, Angew. Chem., Int. Ed., 2019, 58, 5396 CrossRef PubMed;
(e) J. Martin, J. Eyselein, J. Langer, H. Elsen and S. Harder, Chem. Commun., 2020, 56, 9178 RSC;
(f) T. Hollerhage, A. Carpentier, T. P. Spaniol, L. Maron, U. Englert and J. Okuda, Chem. Commun., 2021, 57, 6316 RSC;
(g) T. Hoellerhage, T. P. Spaniol, A. Carpentier, L. Maron and J. Okuda, Inorg. Chem., 2022, 61, 3309 CrossRef CAS PubMed;
(h) T. Hollerhage, P. Ghana, T. P. Spaniol, A. Carpentier, L. Maron, U. Englert and J. Okuda, Angew. Chem., Int. Ed., 2022, 61, e202115379 CrossRef CAS PubMed.
-
(a) W. J. Evans, I. Bloom, W. E. Hunter and J. L. Atwood, J. Am. Chem. Soc., 1983, 105, 1401 CrossRef CAS;
(b) Y. K. Gunko, B. M. Bulychev, G. L. Soloveichik and V. K. Belsky, J. Organomet. Chem., 1992, 424, 289 CrossRef;
(c) G. Desurmont, Y. Li, H. Yasuda, T. Maruo, N. Kanehisa and Y. Kai, Organometallics, 2000, 19, 1811 CrossRef CAS;
(d) A. A. Trifonov, G. G. Skvortsov, D. M. Lyubov, N. A. Skorodumova, G. K. Fukin, E. V. Baranov and V. N. Glushakova, Chem.–Eur. J., 2006, 12, 5320 CrossRef CAS PubMed;
(e) J. Cheng, K. Saliu, G. Y. Kiel, M. J. Ferguson, R. McDonald and J. Takats, Angew. Chem., Int. Ed., 2008, 47, 4910 CrossRef CAS PubMed;
(f) M. Nishiura, J. Baldamus, T. Shima, K. Mori and Z. Hou, Chem.–Eur. J., 2011, 17, 5033 CrossRef CAS PubMed;
(g) D. Martin, J. Kleemann, E. Abinet, T. P. Spaniol, L. Maron and J. Okuda, Eur. J. Inorg. Chem., 2013, 3987 CrossRef CAS;
(h) J. Liu, W. Chen, J. Li and C. Cui, ACS Catal., 2018, 8, 2230 CrossRef CAS.
- W. J. Evans, G. W. Rabe, J. W. Ziller and R. J. Doedens, Inorg. Chem., 1994, 33, 2719 CrossRef CAS.
-
(a) W. J. Evans, D. K. Drummond, L. R. Chamberlain, R. J. Doedens, S. G. Bott, H. M. Zhang and J. L. Atwood, J. Am. Chem. Soc., 1988, 110, 4983 CrossRef CAS;
(b) W. J. Evans, K. A. Miller, S. A. Kozimor, J. W. Ziller, A. G. DiPasquale and A. L. Rheingold, Organometallics, 2007, 26, 3568 CrossRef CAS.
- U. Bayer and R. Anwander, Dalton Trans., 2020, 49, 17472 RSC.
-
(a) D. Heitmann, C. Jones, D. P. Mills and A. Stasch, Dalton Trans., 2010, 39, 1877 RSC;
(b) D. Werner, G. B. Deacon and P. C. Junk, Inorg. Chem., 2019, 58, 1912 CrossRef CAS PubMed;
(c) D. Toniolo, A. R. Willauer, J. Andrez, Y. Yang, R. Scopelliti, L. Maron and M. Mazzanti, Chem.–Eur. J., 2019, 25, 7831 CrossRef CAS PubMed.
-
(a) D. Cui, M. Nishiura, O. Tardif and Z. Hou, Organometallics, 2008, 27, 2428 CrossRef CAS;
(b) D. W. Beh, W. E. Piers, I. del Rosal, L. Maron, B. S. Gelfand, C. Gendy and J. B. Lin, Dalton Trans., 2018, 47, 13680 RSC.
-
(a) N. W. Davies, A. S. Frey, M. G. Gardiner and J. Wang, Chem. Commun., 2006, 4853 RSC;
(b) G. B. Deacon, P. C. Junk, J. Wang and D. Werner, Inorg. Chem., 2014, 53, 12553 CrossRef CAS PubMed;
(c) M. Xemard, V. Goudy, A. Braun, M. Tricoire, M. Cordier, L. Ricard, L. Castro, E. Louyriac, C. E. Kefalidis, C. Clavaguera, L. Maron and G. Nocton, Organometallics, 2017, 36, 4660 CrossRef CAS;
(d) A. R. Willauer, D. Toniolo, F. Fadaei-Tirani, Y. Yang, M. Laurent and M. Mazzanti, Dalton
Trans., 2019, 48, 6100 RSC;
(e) A. R. Willauer, A. M. Dabrowska, R. Scopelliti and M. Mazzanti, Chem. Commun., 2020, 56, 8936 RSC.
- J. Andrez, G. Bozoklu, G. Nocton, J. Pecaut, R. Scopelliti, L. Dubois and M. Mazzanti, Chem.–Eur. J., 2015, 21, 15188 CrossRef CAS PubMed.
- A. Paparo, J. S. Silvia, C. E. Kefalidis, T. P. Spaniol, L. Maron, J. Okuda and C. C. Cummins, Angew. Chem., Int. Ed., 2015, 54, 9115 CrossRef CAS PubMed.
Footnote |
† Electronic supplementary information (ESI) available: Experimental details, spectroscopic characterization. CCDC 2104460–2104466. For ESI and crystallographic data in CIF or other electronic format see DOI: https://doi.org/10.1039/d4sc03104k |
|
This journal is © The Royal Society of Chemistry 2024 |