DOI:
10.1039/D4SC03008G
(Edge Article)
Chem. Sci., 2024,
15, 10945-10953
Self-assembly-integrated tumor targeting and electron transfer programming towards boosting tumor type I photodynamic therapy†
Received
7th May 2024
, Accepted 5th June 2024
First published on 18th June 2024
Abstract
Type I photodynamic therapy (PDT) is attracting increasing interest as an effective solution to the poor prognosis of patients with hypoxic tumors. The development of functional type I photosensitizers is limited by a lack of feasible strategies to systematically modulate electron transfer (ET) in photosensitization. Herein, we present an easily accessible approach for the preparation of nanophotosensitizers with self-assembly-integrated tumor-targeting and ET programming towards boosting tumor type I PDT. Specifically, a dual functional amphiphile PS-02 was designed with a ligand (6-NS) that had the ability to not only target tumor cell marker carbonic anhydrase IX (CAIX) but also regulate the ET process for type I PDT. The amphiphile PS-02 tended to self-assemble into PS-02 nanoparticles (NPs), which exhibited a local “ET-cage effect” due to the electron-deficient nature of 6-NS. It is noteworthy that when PS-02 NPs selectively targeted the tumor cells, the CAIX binding enabled the uncaging of the inhibited ET process owing to the electron-rich characteristic of CAIX. Therefore, PS-02 NPs integrated tumor targeting and CAIX activation towards boosting type I PDT. As a proof of concept, the improved PDT performance of PS-02 NPs was demonstrated with tumor cells under hypoxic conditions and solid tumor tissue in mouse in vivo experiments. This work provides a practical paradigm to develop versatile type I PDT nano-photosensitizers by simply manipulating ET and easy self-assembling.
Introduction
Photodynamic therapy (PDT) for tumor treatment has drawn considerable attention for its merits of non-invasiveness, high cure rate, and minimal side effects compared to chemotherapy, surgery, and radiotherapy.1,2 Although tumor PDT has made lots of progress in clinical trials and application,3–6 the full promise of PDT in these merits has not yet been realized in practice. It is revealed that the complex tumor biological environment, for example, abnormal vasculature and tumor heterogeneity, compromises PDT efficiency.7,8 These unfavorable factors in tumors not only create barriers to the effective delivery of photosensitizer (PS) reagents but also cause the hypoxic environment in tumors.9–13 In terms of tumor PDT, the effective tumor enrichment of PSs is the first prerequisite,14 because increasing tumor PS enrichment can reduce the toxic side effects on normal tissues by cutting down the amounts of PSs injected or the intake of normal tissues.15,16 Besides, it is well known that the hypoxic environment of the tumor directly limits the efficacy of PDT.1,17–20 This is because most of the photosensitizers (PSs) proved in clinical trials are based on the type II PDT mechanism by sensitizing O2 to produce singlet oxygen (1O2). And type II PSs are severely dependent on the O2 supplement. In order to achieve the desired therapeutic outcomes, advanced PDT treatment protocols call for a combination of tumor targeting and circumvention hypoxic treatment modalities.
Further studies have found that type I PDT, in which PSs could sensitize O2 to mainly produce the superoxide anion (O2˙−), was less oxygen-dependent. It was praised for performing well in the treatment of hypoxic tumors.3,21–25 So far, several organic PSs, for example, triarylmethanes,26 benzophenothiazine derivatives,21 1,8-naphthalimide-dialkyl-triphenylamine27,28 and aggregation-induced emission luminogens,29,30 have been reported to follow the type I PDT mechanism. However, the rational molecular design of novel type I PSs is still in its infancy.31,32 Considering electron transfer (ET) as the core photophysical process of type I photosensitization, it is believed that the electron-rich environment around PSs could significantly enhance the ET-based type I process. Following this idea, some traditional type II PSs could transform into dominant type I processes in the electron-rich substrate environment.33–36 Despite these exciting advances, the therapeutic performance of these type I PDT processes still needed to be improved. For example, these previously reported type I nanophotosensitizers mainly relied on the passive targeting of nanoparticles (enhanced permeability and retention (EPR) effect). The enrichment of the drug in the tumor area remained unsatisfactory. To enable a better PS delivery, tumor-selective antibody or ligand modification and tumor-associated factor activation functionalization have been proposed.37,38 But until now, these active targeting techniques were rarely fully employed in these previous type I nanophotosensitizers. It is desirable to design versatile PSs which integrate the enhancement of type I PDT with the endowment of tumor targeting.
Recently, amphiphilic molecule self-assembly has drawn great interest in nanomedicine preparation because it provides a facile and “bottom-up” method to manipulate therapeutic building blocks at a molecular level.39,40 In this work, by rationally tailoring amphiphilic small molecules, self-assembly was leveraged to the bespoke production of nanophotosensitizers with both tumor cell targeting and type I PDT enhancement (Scheme 1). In detail, we have designed and synthesized two amphiphilic small molecules PS-01 and PS-02 from a thermally activated delayed fluorescence (TADF) characteristic photosensitizer molecule PS developed earlier in our laboratory.35,41 In the aqueous environment, PS, PS-01, and PS-02 could self-assemble into nanoparticles PS NPs, PS-01 NPs, and PS-02 NPs, respectively. In PS-01, the piperazine unit was covalently attached to the PS as an electron donor group to provide the local “electron-rich environments” in self-assembled nanoparticles for boosting the type I process. In PS-02, a tumor biomarker carbonic anhydrase IX (CAIX)-targeting ligand saccharin derivative (6-NS) was further modified to the piperazine unit for two purposes. First, by targeting CAIX, PS-02 NPs can achieve tumor-targeting recognition and enrichment.42,43CAIX is known to be an overexpressed membrane protein in most aggressive forms of cancer such as in cancer stem cells.44 And the CAIX-targeting approach could improve and advance the tumor therapeutic treatments.45–47 Second, we assumed that the electron-deficient nature of 6-NS enabled the weakening of the electron donor effect provided by piperazine and turned off the “electron-rich environments” unit by forming an “electron transfer cage” in PS-02 NPs. When PS-02 NPs were targeted to tumors, electron-rich CAIX could uncage the electron transfer for the type I process. Therefore, PS-02 NPs could achieve the integration functions of tumor targeting and type I photosensitization enhancement. As a proof of concept, we have proved the feasibility of this design by photosensitization in solution and detected by nanosecond transient absorption that the ET process occurred in the excited state of PS-01 NPs but not in PS NPs and PS-02 NPs. Meaningfully, PS-02 NPs were devised to effectively cure tumor cells in vitro under hypoxia and tumor tissues in mice in vivo. This strategy of self-assembly-integrated tumor targeting and electron transfer programming should become a universal protocol towards boosting tumor type I PDT.
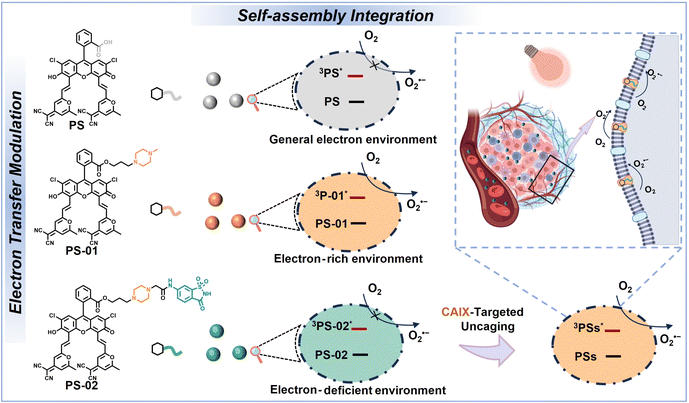 |
| Scheme 1 A brief illustration of the scheme of chemically modifying the structure of amphiphiles' functional molecules and the related self-assembly for nanoparticles. | |
Results and discussion
Molecular synthesis and self-assembly
PS-01 and PS-02 were synthesized by two steps of substitution reaction (Scheme S1†). First, PS and 1,3-dibromopropane were connected by nucleophilic substitution reaction to produce the intermediate PS-Br. Then, PS-Br underwent a second substitution reaction with N-methyl piperazine or the saccharin derivatives (6-NS) to obtain the product PS-01 or PS-02, respectively. The specific synthesis process and molecular structure characterization are documented in the ESI.†
The amphiphilic molecular structure of PS, PS-01, and PS-02 could endow them with the ability to self-assemble into nanoparticles in the aqueous environment.48 The UV-Vis absorption spectra of the amphiphiles in an aqueous solution showed no obvious characteristic peak compared with those in an organic solvent (Fig. 1a and S1†). This indicated that self-assembly may occur in the aqueous solution.49,50 At the same time, the fluorescence intensity of these amphiphiles in the aqueous solution was significantly different (Fig. 1b). PS-01 decreased to 43% of PS in relative fluorescence quantum efficiency, which was consistent with the electron transfer quenching process from the electron donor piperazine (Table S1†). In contrast, PS-02 exhibited a restored fluorescence quantum efficiency comparable to that of PS. From the molecular design, the electron-deficient ligand 6-NS was expected to work as an “ET cage” to weaken the electron-donor effect of piperazine. So, the electron transfer process of piperazine in PS-02 should be inhibited to some extent. Furthermore, when CAIX was added, the fluorescence intensity of PS-02 decreased again. This may be explained by the fact that the electron-rich characteristics of CAIX could uncage the “electron transfer cage” by forming the PS-02/CAIX complex. The transmission electron microscope (TEM) and dynamic light scattering (DLS) experiments were conducted to prove the formation of nanoparticles by self-assembly. PS NPs, PS-01 NPs, and PS-02 NPs had a spherical structure with an average hydrodynamic size of around 150 nm (Fig. 1c and S2†). Moreover, from the hydrodynamic size change of PS-02 NPs after adding CAIX, we inferred that it should effectively disassemble in the presence of CAIX. To verify this, we performed molecular docking simulation calculations. It can be seen that the 6-NS ligand group part of PS-02 could enter into the pocket of CAIX and bring about a tight binding effect with a free energy of binding of −4.35 kcal mol−1 (Fig. 1d). These results indicate that these amphiphiles could self-assemble into functional NPs.
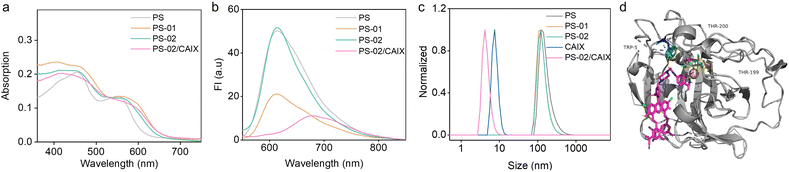 |
| Fig. 1 The basic steady-state spectral properties and self-assembly evaluation in aqueous solutions. (a) The UV-Vis absorption spectra of PS, PS-01, PS-02, and PS-02/CAIX (10 μM) in aqueous solution. (b) The fluorescence emission spectra of PS, PS-01, PS-02, and PS-02/CAIX (10 μM) in aqueous solution. (c) The PS, PS-01, PS-02, and PS-02/CAIX (10 μM) hydrodynamic particle size measured by DLS in aqueous solution. (d) AUTODOCK simulations of binding sites of PS-02/CAIX (PDB code: 3IAI). | |
CAIX-activated photosensitization for boosted O2˙− generation
Next, we investigated whether the binding effect between CAIX and PS-02 could regulate the type I photosensitization process. We tested the photosensitization ability of PS NPs, PS-01 NPs, and PS-02 NPs in aqueous solutions. The results indicated that PS-01 NPs had 18.0 times larger ROS amounts than PS NPs (Fig. 2a and d). Meanwhile, compared with PS-01 NPs, PS-02 NPs showed significantly reduced ROS amounts which were 20% that of PS-01 NPs (Fig. 2b and d). Importantly, when CAIX was added, it could recover the ability of ROS generation (Fig. 2c and d). In order to make clear the CAIX effect in ROS generation capacity, we analyzed the ROS components by a single ROS species quenching experiment using specific ROS scavengers NaN3 (for 1O2),51 vitamin C (for O2˙−)21 and isopropyl alcohol (for OH˙)52 (Fig. 2e). This suggests that O2˙− dominated the ROS generated in the PS-02 NPs/CAIX system. This explained that CAIX mainly affects the type I photosensitization process. To confirm this result, another indicator, dihydrorhodamine 123 (DHR 123), was used for confirming O2˙− generation. As can be seen from Fig. 2f and g, the O2˙− generation capacity of PS-01 NPs has been increased by 16.1 times compared to that of PS NPs. This favors the design purpose that electron donor piperazine's effect as an electron-rich environment inclined to the occurrence of ET of the type I process in PS-01 NPs. However, for PS-02 NPs, the O2˙− generation amounts decreased a lot to only 0.1 compared to that of PS-01 NPs, which was consistent with the proposed “ET cage” to turn off the type I process. So, when CAIX was added, the boosted O2˙− generation recovered (10.7 times that of PS NPs), which meant that the “ET cage” built by the ligand 6-NS was uncaged by CAIX. These results confirmed that the strategy of ET programming through the modification of functionalized groups enabled the regulation of the type I process. In order to identify these different ET processes in PS-01 NPs and PS-02 NPs, the long-lived excited state properties of the PS NPs, PS-01 NPs and PS-02 NPs were studied by transient absorption spectroscopy (Fig. 3). PS NPs, PS-01 NPs, and PS-02 NPs showed a similar triplet excited state absorption at the 640 nm and 400 nm peaks and ground state depletion at the 565 nm and 465 nm peaks (Fig. 3a–c). The differences mainly lie in the kinetic decay process of the triple excited state of 3(PS-01)* that a new rapid decay process with a fitted lifetime τ1 = 35.05 ns appeared compared with PS NPs and PS-02 NPs (Fig. 2d–f). It should be assigned to the ET quenching process derived from the modified electron piperazine in PS-01. Correspondingly, we did detect the single-electron reduction excited state species (PS-01)˙− at 420 nm only in PS-01 NPs but not in PS NPs and PS-02 NPs (Fig. 3g–i).35,53 This long-lived radical anion species (τ2 = 43.96 μs) determined the effective O2 to O2˙− photosensitization. Furthermore, this disappeared ET quenching process in PS-02 NPs meant the ET from piperazine was inhibited. And the absence of the intermediate photosensitizer free radical anion resulted in the off state of the type I process in PS-02 NPs, which was consistent with the significant drop in O2˙− generation. We speculate that in PS-02 NPs the ligand 6-NS should form a local cage to block the ET process.
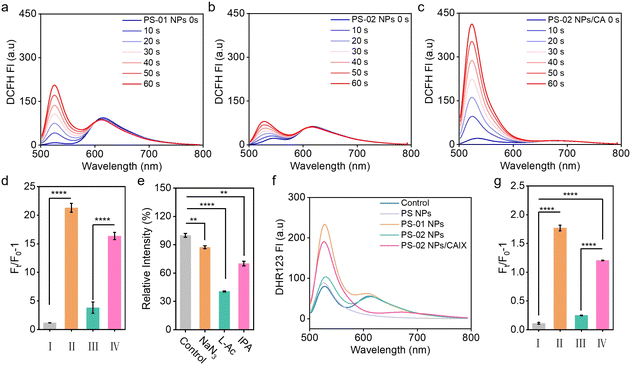 |
| Fig. 2 The photosensitization evaluation in aqueous solutions. General ROS detection by using DCFH (5 μM) for PS-01 NPs (a), PS-02 NPs (b) and PS-02 NPs/CAIX (10 μM) (c). (d) The histogram of relative fluorescence intensity of DCFH of PS NPs (I), PS-01 NPs (II), PS-02 NPs (III), and PS-02 NPs/CAIX (IV) separately after 60 s of irradiation (white light, 2 mW cm−2). (e) The histogram of the normalized relative integration fluorescence intensity ratio of DCFH in the presence of different ROS scavengers for PS-02 NPs/CAIX. (f) The curve graph of relative fluorescence intensity of DHR 123 in PS NPs, PS-01 NPs, PS-02 NPs and PS-02 NPs/CAIX aqueous solution after light irradiation. (g) The histogram of relative fluorescence intensity of DHR 123 of PS NPs (I), PS-01 NPs (II), PS-02 NPs (III), and PS-02 NPs/CAIX (IV) separately after irradiation (white light, 2 mW cm−2). Statistical significance was analyzed by the Student's t-test. ****p < 0.0001; **p < 0.01. | |
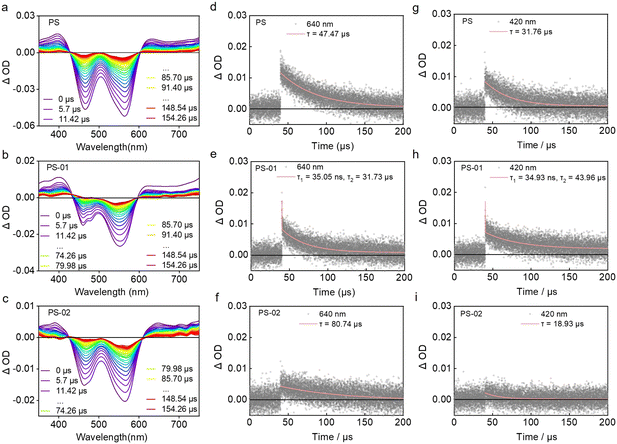 |
| Fig. 3 The transient species and kinetic characterization by nanosecond transient absorption spectroscopy. Time-resolved transient absorption spectra of PS NPs (a), PS-02 NPs (b), and PS-02 NPs (c) (10 μM) in anaerobic aqueous solutions by nitrogen bubbling. The decay curve at the absorption wavelength of 640 nm of PS NPs (d), PS-02 NPs (e), and PS-02 NPs (f) (10 μM) in anaerobic aqueous solutions by nitrogen bubbling. The decay curve at the absorption wavelength of 420 nm of PS NPs (g), PS-01 NPs (h), and PS-02 NPs (i) (10 μM) in anaerobic aqueous solutions by nitrogen bubbling. All samples were excited with a nanosecond pulsed laser, λex = 532 nm. | |
CAIX-integrated tumor cell targeting and type I PDT boosting in vitro
In the above, we have confirmed that the functional nanoparticle NP-02 NPs possessed the ability for CAIX-activation for boosted O2˙− generation in solution. Next, we evaluated the type I PDT performance afforded by this merit in living tumor cells. First, the targeting capability of PS-02 NPs oriented by CAIX was verified by confocal laser scanning microscopy (CLSM) imaging. In the CAIX high-expressive human breast cancer cell of MDA-MB-231, the distribution of PS-02 NPs in the cell membrane was observed. And it was further checked by a colocalization experiment with a commercial membrane dye DiO (Fig. 4a). In contrast, under the same conditions, PS NPs and PS-01 NPs with no target ligand modification showed little accumulation in MDA-MB-231 (Fig. S3†). As expected, the accumulation of PS-02 NPs in the CAIX low-expression one such as breast cancer cells of MCF-7 or normal breast cells COS-7 was rather low (Fig. 4b). Meanwhile, PS-02 NPs could be anchored in MDA-MB-231 cells for as long as 48 h (Fig. 4c). These results supported that the tight CAIX-anchoring features enabled PS-02 NPs to target tumor cells selectively. After confirming the CAIX-mediated tumor cell target function in PS-02 NPs, it is time to check whether CAIX could still boost type I photosensitization in living cells. We chose MDA-MB-231 tumor cells treated with PS-02 NPs as the experiment group and those treated with PS NPs and PS-01 NPs as control groups, separately. The total ROS generation amounts were captured and analyzed with an ROS indicator (DCFH-DA) in CLSM imaging. As shown in Fig. 5a and b, the experimental groups treated by PS-02 NPs maintained the highest ROS generation amounts compared to PS NPs and PS-01 NPs under both normoxic and hypoxic conditions. Then, the boosted O2˙− producing ability was evaluated again by using the O2˙− indicator (DHR 123) (Fig. 5c and d). The fluorescence imaging indicated that PS-02 NPs had the best performance of the type I process in the living cells. And the significantly enhanced O2˙− on tumor cells' killing effect was further evaluated by the live/dead cell co-staining assay (Fig. 5e). Obviously, PS-02 NPs showed pronounced phototoxicity to tumor cells. Owing to the firm anchoring effect of CAIX, PS-02 NPs maintained an excellent tumor cell killing effect even at 24 h after once staining compared with those of PS NPs and PS-01 NPs (Fig. S4†). In order to further elucidate the detailed CAIX-assisted PDT process induced by PS-02 NPs, the time series cell membrane morphology change was monitored by CLSM imaging (Fig. S5†). After light irradiation, lots of bubbles appeared around the plasma membrane and gradually grew out largely into rupture only in 8 min. Next, the phototoxicity of PS-02 NPs to tumor cells was quantitatively evaluated by the MTT assay. As shown in Fig. 5f and g, PS-02 NPs exhibited the best tumor cell killing effect compared to PS NPs and PS-01 NPs, and the half maximal inhibitory concentration (IC50) of PS-02 NPs was as low as 0.34 ± 0.05 μM under normoxic PDT conditions. Under hypoxic PDT conditions, PS-02 NPs still had a much lower IC50 of 0.52 ± 0.15 μM. At the same time, the almost no obvious cell viability change under dark conditions demonstrated all of the three nanoparticles were low biologically toxic (Fig. S6†). All the cellular experiments proved that PS-02 NPs could not only realize the tumor cell targeting but exert significantly boosted type I PDT even under hypoxia.
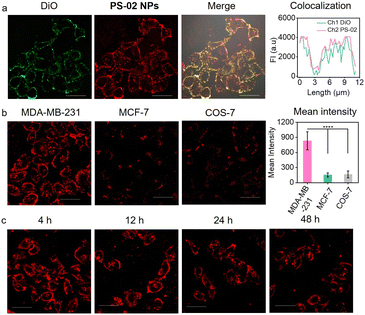 |
| Fig. 4 Photosensitizer staining assessment of live cells. (a) Cell membrane colocalization CLSM imaging of MDA-MB-231 cells stained with PS-02 NPs (5 μM) and DiO (10 μM), DiO: excitation light source 488 nm, detection channel 530 ± 20 nm; PS-02 NPs: excitation light source 559 nm, detection channel 620 ± 20 nm. (b) PS-02 NP distributions in three different human breast cells, breast cancer cell MDA-MB-231 is CAIX high-expression, breast cancer cell MCF-7 is CAIX low-expression and normal breast cell COS-7 is CAIX low-expression. (c) CLSM imaging of a cell membrane stained by PS-02 NPs at different times, excitation light source 559 nm, detection channel 620 ± 20 nm, scale bar = 50 μm. | |
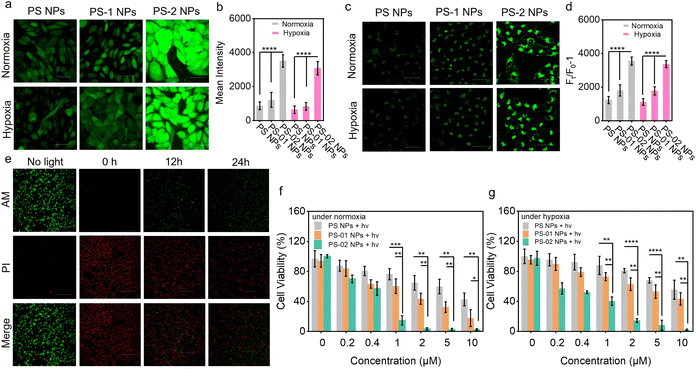 |
| Fig. 5 The ROS amount detection and tumor cell PDT experiment in vitro. (a) The ROS production of PS NPs, PS-01 NPs, and PS-02 NPs (5 μM) in MDA-MB-231 cells under light treatment and detection by DCFH (1 μM). Excitation light source 488 nm, detection channel wavelength = 530 ± 20 nm, scale bar = 50 μm. (b) The histogram of fluorescence intensity of DCFH in (a). (c) The O2˙− production of PS NPs, PS-01 NPs, and PS-02 NPs (5 μM) under light treatment and detection by DHR 123 (10 μM). Excitation light source 488 nm, detection channel wavelength 530 ± 20 nm, scale bar = 50 μm. (d) The histogram of fluorescence intensity of DHR 123 in (c). (e) CLSM imaging of live and dead cells treated with PS-02 NPs (5 μM) under light treatment by calcein AM (2 μM)/propidium iodide (PI) (4 μM) staining. After the cells were once stained by PS-02 NPs, the same light treatment was conducted at 0 h, 12 h, and 24 h, respectively. (Calcein AM: excitation light source 488 nm, detection channel wavelength = 530 ± 20 nm; PI: excitation light source 559 nm, detection channel wavelength = 650 ± 50 nm), scale bar = 300 μm. (f) Relative viabilities of MDA-MB-231 cells treated with different concentrations of PS NPs, PS-01 NPs and PS-02 NPs under light treatment under normoxic conditions. (g) Relative viabilities of MDA-MB-231 cells treated with different concentrations of PS NPs, PS-01 NPs, and PS-02 NPs under light treatment under hypoxic conditions (light treatment conditions: irradiation by white light, 20 mW cm−2, 0.5 h). All data in (b), (d) (f) and (g) are presented as mean ± SD. Statistical significance was analyzed by the Student's t-test. ****p < 0.0001; ***p < 0.001; **p < 0.01; *p < 0.05. | |
Tumor-targeting type I PDT in vivo
Furthermore, the selective tumor therapeutic effect of PS-02 NPs was evaluated in mouse subcutaneous tumor models. Tumor-bearing BAL b/c mice were established and randomly divided into five groups. The group treated with saline with irradiation, PS-02 NPs without irradiation, PS NPs with irradiation, and PS-01 NPs with irradiation were separately set as control groups, and PS-02 NPs with irradiation were set as experiment groups. First, the tumor-selective accumulation of PS-02 NPs in mice was administrated by way of intravenous injection. From Fig. 6a and S7,†PS-02 NPs were observed to realize efficient accumulation in tumor areas for as long as 36 h, which provides a suitable window time for tumor treatment. Following the guidance of good tumor fluorescence imaging, we conducted PDT experiments in the tumor region at 12 h and 36 h after drug injection once. As can be seen from Fig. 6b and c, only the group treated with PS-02 NPs + light exhibited a significant tumor-suppressing efficacy, outperforming the other groups. During this process, all mice did show no noticeable abnormal body weight changes (Fig. 6d). Hematoxylin and eosin (H&E) histological analysis was applied to reveal the necrosis of tumor tissue induced by the PDT of PS-02 NPs (Fig. 6e). These results consistently suggested that PS-02 NPs can exert an excellent tumor curative effect in mice. Moreover, no noticeable cell necrosis and inflammation lesions including in the heart, liver, spleen, lungs, and kidneys appeared in H&E staining imaging (Fig. S8†). Collectively, PS-02 NPs hold impressive therapeutic potential for tumor PDT due to their excellent tumor inhibition efficiency and biocompatibility.
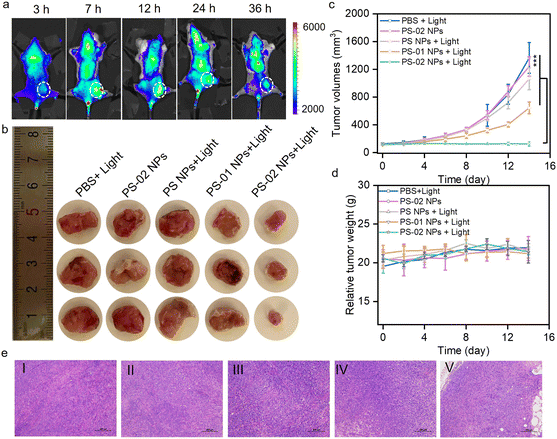 |
| Fig. 6 The evaluation of PDT treatment in tumor-bearing mice. (a) Fluorescence imaging by PS-02 NPs in tumor tissue. (b) The photographs of ex vivo tumors from tumor-bearing mice with different treatment groups. (c) The tumor volume growth curves from tumor-bearing mice with different treatment groups (n = 3). (d) The body weight curves of the mice with different treatments (n = 3). (e) H&E-stained tumors from BAL b/c mice after different treatments. Scale bar = 100 μm. I: PBS + light, II: PS-02 NPs, III: PS NPs + light, IV: PS-01 NPs + light and V: PS-02 NPs + light. All data in (c) and (d) are presented as mean ± SD. Statistical significance was analyzed by the Student's t-test. ***p < 0.001. | |
Conclusions
This work introduced a novel and feasible integrated system for the efficient treatment of hypoxic tumors by rationally leveraging the self-assembly and electron transfer programming strategy. In detail, we have developed two functional amphiphile PS-01 and PS-02 from TADF-type photosensitizer PS by importing an electron-rich group and additionally modifying tumor marker-targeting ligand 6-NS. These amphiphiles tend to assemble into nanoparticles of PS NPs, PS-01 NPs, and PS-02 NPs. PS-01 NPs showed augmented type I photosensitization (16.1 times O2˙− compared to that of PS NPs) owing to the local electron-rich environment provided by the electron donor. PS-02 NPs showed “off state” (0.1 times O2˙− compared to that of PS NPs) type I photosensitization, and we inferred that the electron-deficient ligand 6-NS would work as an “electron transfer cage” to block the ET process in PS-02 NPs. Consistently, when PS-02 NPs targeted and tightly bound to the tumor marker CAIX, the electron-rich nature of CAIX afforded the ability of uncaging the ET effect to boost type I photosensitization (10.7 times O2˙− compared to that of PS NPs). So, PS-02 NPs have integrated their tumor targeting ability with boosted type I photosensitization. It is noteworthy that the significantly improved therapeutic performance of PS-02 NPs was demonstrated with hypoxic tumor cells and solid tumor tissue in mice. We believe this accessible strategy would rejuvenate the development of more functionalized PSs for practical tumor treatments.
Data availability
The crystal structure data of CAIX was deposited at the Protein Data Bank (PDB) with the PDB code 3IAI on the PDB website (https://www.rcsb.org/). All experimental supporting data and procedures are available in the ESI.†
Author contributions
Wenlong Chen and Zehui Wang contributed equally to this work and participated in all experimental processes. Gaobo Hong conducted the data curation; Jianju Du, Fengling Song, and Xiaojun Peng provided the conceptualization and supervision. Wenlong Chen, Zehui Wang, and Fengling Song conducted the writing – original draft and review & editing.
Conflicts of interest
There are no conflicts to declare.
Acknowledgements
This work was supported financially by the Guangdong Basic and Applied Basic Research Foundation (2024A1515012493), the National Key Research and Development Program of China (2023YFC3403000), the National Natural Science Foundation of China (22378231) and the Fundamental Research Funds of Shandong University.
Notes and references
- X. Li, J. F. Lovell, J. Yoon and X. Chen, Clinical development and potential of photothermal and photodynamic therapies for cancer, Nat. Rev. Clin. Oncol., 2020, 17, 657–674 CrossRef PubMed.
- T. C. Pham, V.-N. Nguyen, Y. Choi, S. Lee and J. Yoon, Recent Strategies to Develop Innovative Photosensitizers for Enhanced Photodynamic Therapy, Chem. Rev., 2021, 121, 13454–13619 CrossRef CAS PubMed.
- C. Willyard, The innovative therapies that could break the brain-cancer stalemate, Nature, 2018, 561, S59–S61 CrossRef CAS PubMed.
- P. Agostinis, K. Berg, K. A. Cengel, T. H. Foster, A. W. Girotti, S. O. Gollnick, S. M. Hahn, M. R. Hamblin, A. Juzeniene, D. Kessel, M. Korbelik, J. Moan, P. Mroz, D. Nowis, J. Piette, B. C. Wilson and J. Golab, Photodynamic Therapy of Cancer: An Update, Ca-Cancer J. Clin., 2011, 61, 250–281 CrossRef PubMed.
- J. Nam, S. Son, K. S. Park, W. Zou, L. D. Shea and J. J. Moon, Cancer nanomedicine for combination cancer immunotherapy, Nat. Rev. Mater., 2019, 4, 398–414 CrossRef.
- B. M. Vickerman, E. M. Zywot, T. K. Tarrant and D. S. Lawrence, Taking phototherapeutics from concept to clinical launch, Nat. Rev. Chem, 2021, 5, 816–834 CrossRef PubMed.
- R. Liu, Y. Xu, N. Zhang, S. Qu, W. Zeng, R. Li and Z. Dai, Nanomedicine, 2023, 99–156, DOI:10.1007/978-981-16-8984-0_8 , ch. Chapter 8..
- G. F. Beeghly, A. A. Shimpi, R. N. Riter and C. Fischbach, Measuring and modelling tumour heterogeneity across scales, Nat. Rev. Bioeng., 2023, 1, 712–730 CrossRef.
- S. Wilhelm, A. J. Tavares, Q. Dai, S. Ohta, J. Audet, H. F. Dvorak and W. C. W. Chan, Analysis of nanoparticle delivery to tumours, Nat. Rev. Mater., 2016, 1, 16014 CrossRef CAS.
- K. Hida, N. Maishi, Y. Sakurai, Y. Hida and H. Harashima, Heterogeneity of tumor endothelial cells and drug delivery, Adv. Drug Delivery Rev., 2016, 99, 140–147 CrossRef CAS PubMed.
- M. J. Mitchell, M. M. Billingsley, R. M. Haley, M. E. Wechsler, N. A. Peppas and R. Langer, Engineering precision nanoparticles for drug delivery, Nat. Rev. Drug Discovery, 2021, 20, 101–124 CrossRef CAS PubMed.
- S. K. Singh and R. Singh, Nanotherapy: targeting the tumour microenvironment, Nat. Rev. Cancer, 2022, 22, 258 CrossRef CAS PubMed.
- V. Bhandari, C. Hoey, L. Y. Liu, E. Lalonde, J. Ray, J. Livingstone, R. Lesurf, Y.-J. Shiah, T. Vujcic, X. Huang, S. M. G. Espiritu, L. E. Heisler, F. Yousif, V. Huang, T. N. Yamaguchi, C. Q. Yao, V. Y. Sabelnykova, M. Fraser, M. L. K. Chua, T. van der Kwast, S. K. Liu, P. C. Boutros and R. G. Bristow, Molecular landmarks of tumor hypoxia across cancer types, Nat. Genet., 2019, 51, 308–318 CrossRef CAS PubMed.
- G. He, Y. Li, M. R. Younis, L.-H. Fu, T. He, S. Lei, J. Lin and P. Huang, Synthetic biology-instructed transdermal microneedle patch for traceable photodynamic therapy, Nat. Commun., 2022, 13, 6238 CrossRef CAS PubMed.
- X. Liu, S. Viswanadhapalli, S. Kumar, T.-K. Lee, A. Moore, S. Ma, L. Chen, M. Hsieh, M. Li, G. R. Sareddy, K. Parra, E. B. Blatt, T. C. Reese, Y. Zhao, A. Chang, H. Yan, Z. Xu, U. P. Pratap, Z. Liu, C. M. Roggero, Z. Tan, S. T. Weintraub, Y. Peng, R. R. Tekmal, C. L. Arteaga, J. Lippincott-Schwartz, R. K. Vadlamudi, J.-M. Ahn and G. V. Raj, Targeting LIPA independent of its lipase activity is a therapeutic strategy in solid tumors via induction of endoplasmic reticulum stress, Nat. Cancer, 2022, 3, 866–884 CrossRef CAS PubMed.
- M. Srinivasarao and P. S. Low, Ligand-Targeted Drug Delivery, Chem. Rev., 2017, 117, 12133–12164 CrossRef CAS PubMed.
- A. Vito, N. El-Sayes and K. Mossman, Hypoxia-Driven Immune Escape in the Tumor Microenvironment, Cells, 2020, 9, 992 CrossRef CAS PubMed.
- B. Pucelik, A. Sulek, A. Barzowska and J. M. Dabrowski, Recent advances in strategies for overcoming hypoxia in photodynamic therapy of cancer, Cancer Lett., 2020, 492, 116–135 CrossRef CAS PubMed.
- D. E. J. G. J. Dolmans, D. Fukumura and R. K. Jain, Photodynamic therapy for cancer, Nat. Rev. Cancer, 2003, 3, 380–387 CrossRef CAS PubMed.
- S. Singh, A. Aggarwal, N. V. Bhupathiraju, G. Arianna, K. Tiwari and C. M. Drain, Glycosylated Porphyrins, Phthalocyanines, and Other Porphyrinoids for Diagnostics and Therapeutics, Chem. Rev., 2015, 115, 10261–10306 CrossRef CAS PubMed.
- M. Li, J. Xia, R. Tian, J. Wang, J. Fan, J. Du, S. Long, X. Song, J. W. Foley and X. Peng, Near-Infrared Light-Initiated Molecular Superoxide Radical Generator: Rejuvenating Photodynamic Therapy against Hypoxic Tumors, J. Am. Chem. Soc., 2018, 140, 14851–14859 CrossRef CAS PubMed.
- J. Du, T. Shi, S. Long, P. Chen, W. Sun, J. Fan and X. Peng, Enhanced photodynamic therapy for overcoming tumor hypoxia: From microenvironment regulation to photosensitizer innovation, Coord. Chem. Rev., 2021, 427, 213604 CrossRef CAS.
- M. Li, Y. Xu, X. Peng and J. S. Kim, From Low to No O2-Dependent Hypoxia Photodynamic Therapy (hPDT): A New Perspective, Acc. Chem. Res., 2022, 55, 3253–3264 CrossRef CAS PubMed.
- W. Fan, P. Huang and X. Chen, Overcoming the Achilles' heel of photodynamic therapy, Chem. Soc. Rev., 2016, 45, 6488–6519 RSC.
- T. C. Pham, V. N. Nguyen, Y. Choi, S. Lee and J. Yoon, Recent Strategies to Develop Innovative Photosensitizers for Enhanced Photodynamic Therapy, Chem. Rev., 2021, 121, 13454–13619 CrossRef CAS PubMed.
- R. Docampo, S. N. J. Moreno, R. P. A. Muniz, F. S. Cruz and R. P. Mason, Light-Enhanced Free Radical Formation and Trypanocidal Action of Gentian Violet (Crystal Violet), Science, 1983, 220, 1292–1295 CrossRef CAS PubMed.
- L. Yu, Y. Xu, Z. Pu, H. Kang, M. Li, J. L. Sessler and J. S. Kim, Photocatalytic Superoxide Radical Generator that Induces Pyroptosis in Cancer Cells, J. Am. Chem. Soc., 2022, 144, 11326–11337 CrossRef CAS PubMed.
- Z. Shen, S. Zheng, Y. Fang, G. Zhang, C. Zhu, S. Liu and J. Hu, Overcoming the Oxygen Dilemma in Photoredox Catalysis: Near-Infrared (NIR) Light-Triggered Peroxynitrite Generation for Antibacterial Applications, Angew. Chem., Int. Ed., 2023, 62, e202219153 CrossRef CAS PubMed.
- K. Chen, P. He, Z. Wang and B. Z. Tang, A Feasible Strategy of Fabricating Type I Photosensitizer for Photodynamic Therapy in Cancer Cells and Pathogens, ACS Nano, 2021, 15, 7735–7743 CrossRef CAS PubMed.
- Y. Wang, J. Liao, Y. Lyu, Q. Guo, Z. Zhu, X. Wu, J. Yu, Q. Wang and W.-H. Zhu, An AIE Photosensitizer with Simultaneous Type I and Type II ROS Generation: Efficient Bacterial Elimination and Hypoxic Tumor Ablation, Adv. Funct. Mater., 2023, 33, 2301692 CrossRef CAS.
- D. Chen, Q. Xu, W. Wang, J. Shao, W. Huang and X. Dong, Type I Photosensitizers Revitalizing Photodynamic Oncotherapy, Small, 2021, 17, e2006742 CrossRef PubMed.
- K. X. Teng, W. K. Chen, L. Y. Niu, W. H. Fang, G. Cui and Q. Z. Yang, BODIPY-Based Photodynamic Agents for Exclusively Generating Superoxide Radical over Singlet Oxygen, Angew. Chem., Int. Ed., 2021, 60, 19912–19920 CrossRef CAS PubMed.
- D. Chen, Z. Wang, H. Dai, X. Lv, Q. Ma, D.-P. Yang, J. Shao, Z. Xu and X. Dong, Boosting O2˙− Photogeneration via Promoting Intersystem-Crossing and Electron-Donating Efficiency of Aza-BODIPY-Based Nanoplatforms for Hypoxic-Tumor Photodynamic Therapy, Small Methods, 2020, 4, 2000013 CrossRef CAS.
- H. Ding, H. Yu, Y. Dong, R. Tian, G. Huang, D. A. Boothman, B. D. Sumer and J. Gao, Photoactivation switch from type II to type I reactions by electron-rich micelles for improved photodynamic therapy of cancer cells under hypoxia, J. Controlled Release, 2011, 156, 276–280 CrossRef CAS PubMed.
- W. Chen, Z. Wang, M. Tian, G. Hong, Y. Wu, M. Sui, M. Chen, J. An, F. Song and X. Peng, Integration of TADF Photosensitizer as “Electron Pump” and BSA as “Electron Reservoir” for Boosting Type I Photodynamic Therapy, J. Am. Chem. Soc., 2023, 145, 8130–8140 CrossRef CAS PubMed.
- X. Li, D. Lee, J. D. Huang and J. Yoon, Phthalocyanine-Assembled Nanodots as Photosensitizers for Highly Efficient Type I Photoreactions in Photodynamic Therapy, Angew. Chem., Int. Ed., 2018, 57, 9885–9890 CrossRef CAS PubMed.
- D. Schrama, R. A. Reisfeld and J. C. Becker, Antibody targeted drugs as cancer therapeutics, Nat. Rev. Drug Discovery, 2006, 5, 147–159 CrossRef CAS PubMed.
- M. Gao, F. Yu, C. Lv, J. Choo and L. Chen, Fluorescent chemical probes for accurate tumor diagnosis and targeting therapy, Chem. Soc. Rev., 2017, 46, 2237–2271 RSC.
- D. Pochan and O. Scherman, Introduction: Molecular Self-Assembly, Chem. Rev., 2021, 121, 13699–13700 CrossRef CAS PubMed.
- Z. Xie, T. Fan, J. An, W. Choi, Y. Duo, Y. Ge, B. Zhang, G. Nie, N. Xie, T. Zheng, Y. Chen, H. Zhang and J. S. Kim, Emerging combination strategies with phototherapy in cancer nanomedicine, Chem. Soc. Rev., 2020, 49, 8065–8087 RSC.
- X. Q. Xiong, F. L. Song, J. Y. Wang, Y. K. Zhang, Y. Y. Xue, L. L. Sun, N. Jiang, P. Gao, L. Tian and X. J. Peng, Thermally Activated Delayed Fluorescence of Fluorescein Derivative for Time-Resolved and Confocal Fluorescence Imaging, J. Am. Chem. Soc., 2014, 136, 9590–9597 CrossRef CAS PubMed.
- C. Lomelino and R. McKenna, Carbonic anhydrase inhibitors: a review on the progress of patent literature (2011-2016), Expert Opin. Ther. Pat., 2016, 26, 947–956 CrossRef CAS PubMed.
- S. Zhang, C. Yang, W. Lu, J. Huang, W. Zhu, H. Li, Y. Xu and X. Qian, A highly selective space-folded photo-induced electron transfer fluorescent probe for carbonic anhydrase isozymes IX and its applications for biological imaging, Chem. Commun., 2011, 47, 8301–8303 RSC.
- J. H. Kim, P. Verwilst, M. Won, J. Lee, J. L. Sessler, J. Han and J. S. Kim, A Small Molecule Strategy for Targeting Cancer Stem Cells in Hypoxic Microenvironments and Preventing Tumorigenesis, J. Am. Chem. Soc., 2021, 143, 14115–14124 CrossRef CAS PubMed.
- H. S. Jung, J. Han, H. Shi, S. Koo, H. Singh, H. J. Kim, J. L. Sessler, J. Y. Lee, J. H. Kim and J. S. Kim, Overcoming the Limits of Hypoxia in Photodynamic Therapy: A Carbonic Anhydrase IX-Targeted Approach, J. Am. Chem. Soc., 2017, 139, 7595–7602 CrossRef CAS PubMed.
- H. S. Jung, S. Koo, M. Won, S. An, H. Park, J. L. Sessler, J. Han and J. S. Kim, Cu(ii)-BODIPY photosensitizer for CAIX overexpressed cancer stem cell therapy, Chem. Sci., 2023, 14, 1808–1819 RSC.
- J. H. Kim, S. Park, E. Jung, J. Shin, Y. J. Kim, J. Y. Kim, J. L. Sessler, J. H. Seo and J. S. Kim, A dual-action niclosamide-based prodrug that targets cancer stem cells and inhibits TNBC metastasis, Proc. Natl. Acad. Sci. U.S.A., 2023, 120, e2304081120 CrossRef CAS PubMed.
- D. Lombardo, M. A. Kiselev, S. Magazù and P. Calandra, Amphiphiles Self-Assembly: Basic Concepts and Future Perspectives of Supramolecular Approaches, Adv. Condens. Matter Phys., 2015, 2015, 1–22 CrossRef.
- C. Po, A. Y. Tam, K. M. Wong and V. W. Yam, Supramolecular self-assembly of amphiphilic anionic platinum(II) complexes: a correlation between spectroscopic and morphological properties, J. Am. Chem. Soc., 2011, 133, 12136–12143 CrossRef CAS PubMed.
- E. Feng, Y. Liu, S. Lv, D. Liu, S. Huang, Z. Li and F. Song, Fine-Tuning Cu (II)-Induced Self-Assembly of Hydrophilic Cyanine Dyes for Enhanced Tumor Photothermal Therapy, Adv. Funct. Mater., 2022, 32, 2209258 CrossRef CAS.
- H. Wu, F. Chen, C. You, Y. Zhang, B. Sun and Q. Zhu, Smart Porous Core-Shell Cuprous Oxide Nanocatalyst with High Biocompatibility for Acid-Triggered Chemo/Chemodynamic Synergistic Therapy, Small, 2020, 16, e2001805 CrossRef PubMed.
- S. Wang, M. Rong, H. Li, T. Xu, Y. Bu, L. Chen, X. Chen, Z. P. Yu, X. Zhu, Z. Lu and H. Zhou, Unveiling Mechanism of Organic Photogenerator for Hydroxyl Radicals Generation by Molecular Modulation, Small, 2022, 18, e2104857 CrossRef PubMed.
- T. Tachikawa, Y. Kobori, K. Akiyama, A. Katsuki, Y. Usui, U. E. Steiner and S. Tero-Kubota, Solvent effects on the intrinsic enhancement factors of the triplet exciplex generated by photoinduced electron transfer reaction between eosin Y and duroquinone, Mol. Phys., 2002, 100, 1413–1420 CrossRef CAS.
Footnotes |
† Electronic supplementary information (ESI) available. See DOI: https://doi.org/10.1039/d4sc03008g |
‡ Wenlong Chen and Zehui Wang contributed equally to this work. |
|
This journal is © The Royal Society of Chemistry 2024 |
Click here to see how this site uses Cookies. View our privacy policy here.