DOI:
10.1039/D4SC01804D
(Edge Article)
Chem. Sci., 2024,
15, 10135-10145
Copper/ruthenium relay catalysis enables 1,6-double chiral inductions with stereodivergence†
Received
18th March 2024
, Accepted 11th May 2024
First published on 16th May 2024
Abstract
The dual catalysis strategy is an efficient and powerful tool to fulfill the stereodivergent synthesis of stereoisomeric products from the same set of starting materials. Great attention has been given to the construction of chiral compounds with two contiguous stereocenters. However, the synthesis of two remote noncontiguous stereocenters is more challenging and is less developed, despite the high demand for synthetic tactics. We herein developed an unprecedented example of the stereodivergent preparation of synthetically useful and biologically important chiral ζ-hydroxy amino ester derivatives containing remote 1,6-noncontiguous stereocenters and a unique β,γ-unsaturation moiety. This cascade dehydrogenation/1,6-Michael addition/hydrogenation protocol between readily-available ketoimine esters and racemic branched dienyl carbinols was rationally realized with bimetallic copper/ruthenium relay catalysis. The key features of the process were atom economy, step economy, and redox-neutrality. All four stereoisomers of chiral ζ-hydroxy amino ester derivatives were easily achieved by the orthogonal permutations of a chiral copper catalyst and chiral ruthenium catalyst. Importantly, a much more challenging stereodivergent synthesis of all eight stereoisomers of chiral peptide products containing three remote stereocenters was accomplished with excellent results through the cooperation of two chiral catalyst pairs and substrate enantiomers.
Introduction
Chiral organic molecules containing multiple stereocenters are frequently found in many natural products, pharmaceuticals, proteins, and biologically active molecules.1,2 Due to the synthetic versatility and promising biological and pharmaceutical activities, they play an important role in modern synthetic chemistry, especially in medicinal chemistry. It is important and challenging to achieve the precise synthesis of each stereoisomer, which is significantly helpful in elucidating the relationship between molecular structure and biological activities, while facilitating the rapid pace of drug discovery.3,4 Therefore, the development of an efficient and facile strategy for the straightforward generation of all possible enantiopure stereoisomers has attracted a great deal of interest.5–11 The stereodivergent dual catalysis strategy, including synergistic catalysis12–17 and relay catalysis,18–23 is a powerful protocol that allows reliable synthesis of all stereoisomers from a common set of starting materials and conditions. This strategy can activate two substrates simultaneously or sequentially and control the formation of stereogenic centers independently during the reaction process by employing two distinct chiral catalysts. Owing to its great advantages, tremendous efforts have been devoted to the development of stereodivergent dual catalysis strategies in recent years. Despite significant achievements in stereodivergent reactions, the reported methodologies for stereodivergent synthesis mainly focus on the construction of 1,2-contiguous stereocenters with many elegant examples.24–53 However, very limited research works disclose the synthesis of chiral molecules containing noncontiguous stereocenters. Successful examples of the synthesis of challenging 1,3-/1,4-nonadjacent stereocenters are sparse.54–64 In sharp contrast, for the stereodivergent construction of remoter and much more arduous 1,6-nonadjacent stereocenters, it remains an unsolved and prominent synthetic challenge to achieve the full set of four stereoisomers with high yields and excellent stereoselectivities (Scheme 1a).
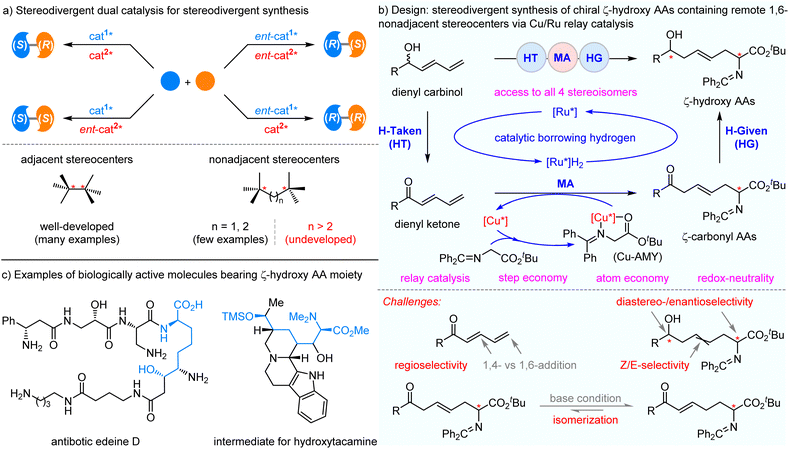 |
| Scheme 1 (a) Stereodivergent dual catalysis, (b) our design: stereodivergent synthesis of 1,6-noncontiguous stereocenters, (c) examples of biologically active molecules bearing ζ-hydroxy amino ester derivatives. | |
On the other hand, asymmetric borrowing-hydrogen catalysis65–69 has emerged as an important and efficient relay catalytic approach to access structurally valuable chiral molecules from easily accessible alcohols in a rapid and atom-economical fashion.70–72 Recently, with the strategy of relay catalysis, we successfully developed two methods of stereodivergent construction of 1,4-nonadjacent stereocenters via merging asymmetric borrowing-hydrogen reaction with asymmetric Michael addition.61,62 Considering the concerns of stereodivergent synthesis of privileged scaffolds with remote 1,6-nonadjacent stereocenters, we envisioned combining asymmetric borrowing-hydrogen catalysis and the azomethine ylides-involved Lewis acid catalysis73–75 to achieve this challenging goal. As shown in Scheme 1b, the nucleophilic metallated Cu–azomethine ylide73 (Cu–AMY) could be formed in situ by the coordination of glycine-derived diphenyl ketoimine ester with chiral copper(I) Lewis acid under basic conditions. Meanwhile, the racemic branched dienyl carbinol substrate could be oxidatively dehydrogenated to generate an α,β,γ,δ-unsaturated ketone intermediate and chiral Ru–hydride (Ru–H) species, via the chiral borrowing-hydrogen Ru-catalyst. The ensuing asymmetric 1,6-Michael addition, followed by chiral Ru–hydride-promoted asymmetric reduction, would result in the stereodivergent synthesis of highly functionalized ζ-hydroxy amino ester derivative containing remote 1,6-nonadjacent stereocenters and β,γ-unsaturation. These are pervasive structural units, not only in a plethora of natural products and bioactive molecules, but also work as key synthetic intermediates (Scheme 1c).76–78 The copper/ruthenium relay-catalyzed dehydrogenation/1,6-Michael addition/hydrogenation process, thus envisaged, has some challenges that must be overcome: (1) the competitive reactions among 1,6- vs. 1,4-addition lead to difficult control of regioselectivity (site selectivity). In most cases, the partial positive charge density is less at the δ-position than the β-position;79–84 meanwhile the undesired Ru–H reduction85,86 of C
C double bonds in α,β,γ,δ-unsaturated ketone intermediate must be suppressed to improve the reaction efficiency; (2) the control of stereoselectivity, as the initial-formed stereocenter is formed at the remote 6-position of the chiral hydroxy center (five bonds between the two stereocenters) formed later; (3) the control over the E/Z geometry87 of dienyl ketone-based asymmetric 1,6-addition reaction; (4) the potential base-promoted position isomerization of the β,γ-C
C double bond of Michael addition intermediate (chiral ketone) into the thermodynamically favored conjugated enone.88
Herein, we reported the stereodivergent synthesis of highly functionalized chiral ζ-hydroxy amino ester derivatives containing remote 1,6-nonadjacent stereocenters and a unique β,γ-unsaturation moiety through dehydrogenation/1,6-Michael addition/hydrogenation relay process between diphenyl ketimine ester/peptides and racemic branched dienyl carbinols enabled by bimetallic copper/ruthenium relay catalysis. This protocol takes advantage of orthogonal one-pot catalysis, omission of intermediate purification steps, full atom economy, redox-neutrality, and efficient access to all four stereoisomers with remote 1,6-nonadjacent stereocenters and predictable control of stereoselectivity.
Results and discussion
Optimization of reaction conditions
Initially, the design protocol was started with benzophenone-derived ketimine ester 1a and racemic branched dienyl carbinol 2a as the model substrates. This first step aimed to investigate the feasibility of dehydrogenation/1,6-Michael addition/hydrogenation reaction promoted by the cooperative Cu/Ru bimetallic relay catalysis at 20 °C. To our delight, the expected product, highly functionalized ζ-hydroxy amino ester trans-3a, bearing 1,6-nonadjacent stereocenters and a β,γ-unsaturation moiety, was accessible in high yield with moderate diastereoselectivity and excellent enantioselectivity with the combined Cu(I)/L1
89 and [Ru]-1
90,91 as the catalysts and K3PO4 as the base (93% yield, 4
:
1 dr, >99% ee, Table 1, entry 1). To be noted, the cascade reaction developed here exhibited excellent E/Z-geometry selectivity, and (E)-3a formed as the sole product. No regio-isomer formed by 1,4-Michael addition was detected by crude 1H NMR, suggesting that exclusive regioselectivity favored the less sterically hindered 1,6-Michael addition. Meanwhile, no side product with α,β-unsaturation was observed, indicating that the rate of base-promoted isomerization of the ketone intermediate was slower than that of 1,6-Michael addition. It was found that the subsequent solvent screening did not deliver better results in 2-Me-THF, Et2O and MTBE, but mess reaction systems were observed in 1,4-dioxane and toluene (Table 1, entries 2–6). A range of chiral ligands for copper complex were then examined, including the phosphoramidite ligand L2,92 (R)-BINAP L3, and ferrocene-based P,N ligands (L4–L7) (Table 1, entries 7–12); ligand L7 provided the best results in 99% yield with >20
:
1 dr and >99% ee (see ESI† for details on more screening on chiral ligands, base and solvent). To our delight, when switching the borrowing-hydrogen catalyst [Ru]-1 to the enantiomeric complex ent-[Ru]-1 under otherwise identical conditions, the diastereomeric ζ-hydroxy amino ester cis-3a was separated in 96% yield with exclusive diastereoselectivity and excellent enantioselectivity (Table 1, entry 13). In addition, control experiments verified that both copper and ruthenium catalysts are indispensable for this asymmetric cascade process, and the desired product 3a could not be generated without either of the two chiral metal complexes (Table 1, entries 14 and 15).
Table 1 Optimization of reaction conditionsa
Substrate scope study
Having established optimal reaction conditions, we focused on the investigation of the substrate generality of this cooperative bimetallic Cu/Ru relay-catalyzed dehydrogenation/1,6-Michael addition/hydrogenation reaction. As summarized in Scheme 2, a variety of racemic dienyl carbinols 2 were examined to react with benzophenone-derived ketimine ester 1a for the construction of densely functionalized chiral ζ-hydroxy amino esters. The racemic dienyl carbinols 2 bearing diverse substituted groups on the phenyl ring, such as electron-neutral (entry 1), electron-deficient (entries 2–6), and electron-donating (entries 7–10), at different positions served as compatible reaction partners and went through this cascade reaction efficiently to access the corresponding products, including trans-3a–3j bearing 1,6-nonadjacent stereocenters with excellent results (82–99% yields, 11
:
1 → 20
:
1 dr, exclusively >99% ee). In addition, the heteroaromatic ring-substituted dienyl carbinols, 2k and 2n, also worked as viable reactants, affording the desired products, trans-3k and trans-3n, in high yields with moderate diastereoselectivities and >99% ee (Scheme 2, entries 11 and 14). Notably, 1-naphthyl and 2-naphthyl-substituted dienyl carbinols with steric hindrance were examined, and the corresponding products, trans-3l and trans-3m, could be obtained in good yields with acceptable diastereoselectivities and excellent enantioselectivities (82% yield, 17
:
1 dr, >99% ee and 76% yield, 13
:
1 dr, >99% ee; respectively). When racemic methyl (2o) or ethyl (2p)-substituted dienyl carbinol was examined, the cascade reaction proceeded smoothly to generate the corresponding product, trans-3o or trans-3p, in good yields with excellent enantioselectivity albeit with lower diastereoselectivity93 (Scheme 2, entries 15 and 16). Remarkably, racemic cyclohexyl-substituted dienyl carbinol 2q was compatible to give the desired product trans-3q in high yield with good diastereoselectivity and excellent enantioselectivity (80% yield, 6
:
1 dr, >99% ee, Scheme 2, entry 17). Encouraged by these promising results, we extended this bimetallic Cu/Ru catalyst system to the benzophenone-derived ketimine dipeptide; the Gly–Gly–peptide-containing substrate 1b could be employed well in this relay catalytic system to deliver the desired product, trans-3r, in 76% yield with >20
:
1 dr and >99% ee (Scheme 2, entry 18). In addition, the influence of the substituted group on the position of the dienyl motif was also investigated. Although low diastereoselectivities were observed for the 1,6-adducts, 3s and 3u, the desired trans-3t-bearing methyl group on the γ-position could be obtained in 71% yield with >20
:
1 dr and >99% ee (Scheme 2, entries 19–21), and no 1,4-adducts were observed.
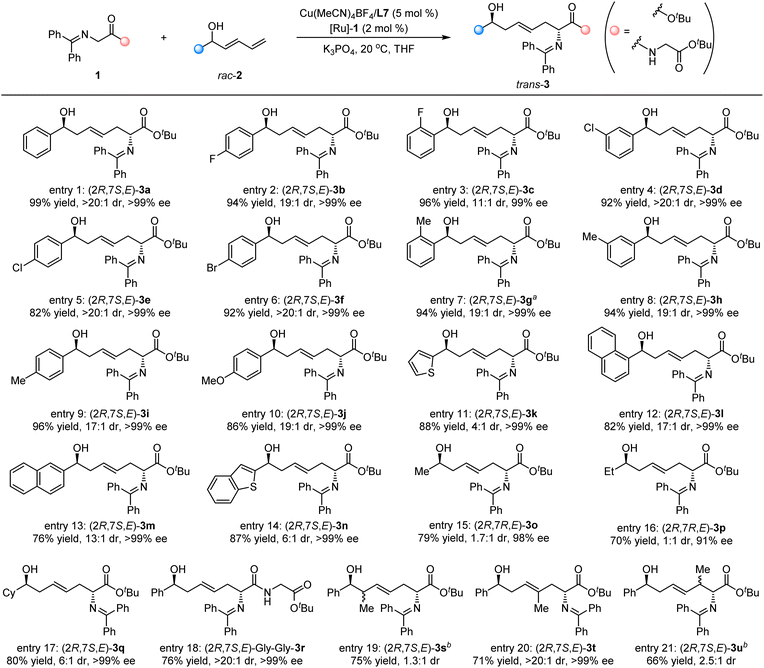 |
| Scheme 2 Substrate scope of the trans-selective dehydrogenation/1,6-Michael addition/hydrogenation reaction. All reactions were carried out with 0.60 mmol 2, 0.20 mmol 1, 0.01 mmol [Cu], 0.011 mmol L7, 0.004 mmol [Ru]-1, and 0.20 mmol K3PO4 in 2 mL of THF at 20 °C for 3–12 h. Isolated yield for the diastereomeric mixture. The dr and ee values were determined by HPLC analysis. a 40 °C for 12 h. b The ee values were not determined because the racemic samples could not be separated with all the chiral columns in the lab. | |
Considering the above success in the trans-selective synthesis of chiral ζ-hydroxy amino ester derivative bearing 1,6-nonadjacent stereocenters, we paid attention to the preparation of the diastereoisomers with cis-selectivity promoted by [Cu/L7]/ent-[Ru]-1 catalytic system. A wide range of racemic dienyl carbinols 2 reacted with ketimine ester 1a smoothly, giving the corresponding cis-selective products in good to high yields and excellent stereoselectivities. As summarized in Scheme 3, the electronic properties and positions of the substituted groups on the aryl ring of the dienyl carbinol substrates had a negligible impact on the reaction results. The corresponding products, cis-3a–3j, could be obtained in 75–97% yields with 11
:
1 → 20
:
1 dr and >99% ee (Scheme 3, entries 1–10). In addition, the naphthyl- or aromatic heterocyclic-substituted dienyl carbinols were observed to be suitable reaction partners to generate the desired products, cis-3k–3n, with excellent results (85–94% yields, 6
:
1–17
:
1 dr, 98% → 99% ee, Scheme 3, entries 11–14). The challenging alkyl substituted dienyl carbinols, 2o–2q, also showed good reactivity, delivering the desired product, cis-3o–3q, in good yields with low to moderate diastereoselectivity and excellent enantioselectivity (Scheme 3, entries 15–17). To our delight, the ketimine amide 1b bearing Gly–Gly–dipeptide also worked well to afford the expected product cis-3r in good yield with excellent stereoselectivity (Scheme 3, entry 18). In addition, the dienyl carbinols bearing methyl group on the different positions of the dienyl motif were also examined, and the 1,6-adducts, 3s–3u, were obtained as the only regioisomers in good yields (Scheme 3, entries 19–21). Excellent diastereoselectivity and excellent enantioselectivity were observed for the cis-3t-bearing methyl group on the γ-position (Scheme 3, entry 20).
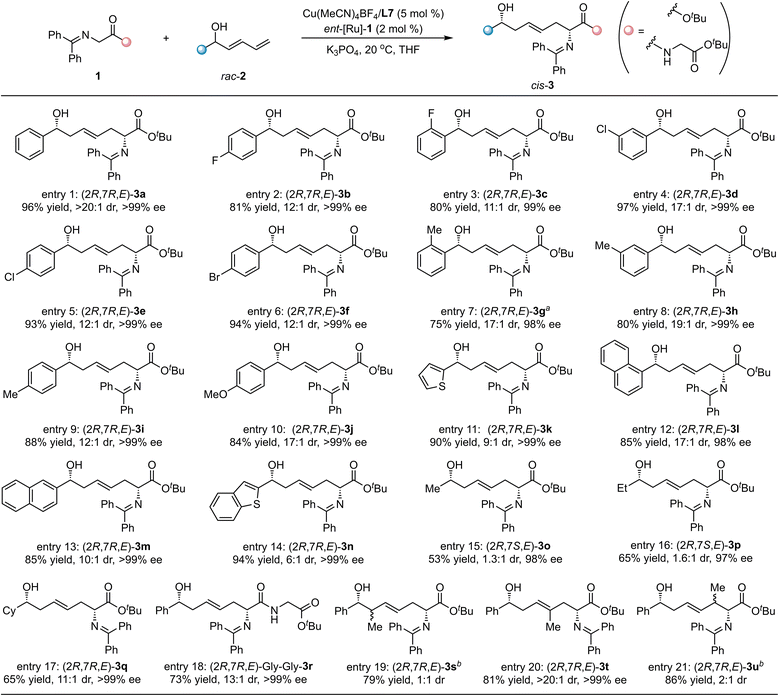 |
| Scheme 3 The substrate scope of the cis-selective dehydrogenation/1,6-Michael addition/hydrogenation reaction. All reactions were carried out with 0.60 mmol 2, 0.20 mmol 1, 0.01 mmol [Cu], 0.011 mmol L7, 0.004 mmol ent-[Ru]-1, and 0.20 mmol K3PO4 in 2 mL of THF at 20 °C for 3–12 h. Isolated yield for the diastereomeric mixture. The dr and ee values were determined by HPLC analysis. a 40 °C for 12 h. b The ee values were not determined because the racemic samples could not be separated with all the chiral columns in the lab. | |
Having investigated the substrate generality of dienyl carbinols, we are interested in examining whether trienyl carbinols could be tolerated in the cooperative Cu/Ru bimetallic relay catalytic system to provide otherwise inaccessible θ-hydroxy amino ester derivative bearing more remote 1,8-nonadjacent stereocenters. As shown in Scheme 4, when (2E,4E)-1-phenylhepta-2,4,6-trien-1-ol 4a was applied as the substrate, in combination with Cu(I)/L7/[Ru]-1 complexes as the relay catalyst, the corresponding trans-selective product, trans-5a, could be easily accessible in 94% yield with 16
:
1 dr and >99% ee. Trienyl carbinols containing electron-donating (Me, 4b) or electron-deficient (F, 4c)-substituted groups on the phenyl ring proceeded this asymmetric cascade dehydrogenation/1,8-Michael addition/hydrogenation reaction smoothly, and the desired products, trans-5b and trans-5c, were obtained in high yields with excellent diastereoselectivities and enantioselectivities (93% yield, >20
:
1 dr, >99% ee and 95% yield, 19
:
1 dr, >99% ee, respectively). It was found that ketimine amide 1b bearing Gly–Gly–dipeptide could react with 4a for the generation of the expected product, trans-5d, in 83% yield with >20
:
1 dr and >99% ee. To our delight, the cis-selective dehydrogenation/1,8-Michael addition/hydrogenation reaction was readily achieved, in combination with Cu(I)/L7/ent-[Ru]-1 catalytic system, and the corresponding products, cis-5a–5d, were obtained in high yields with high diastereoselectivities and excellent enantioselectivities.
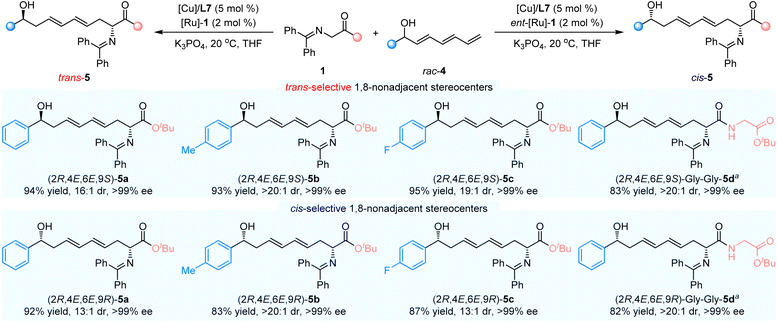 |
| Scheme 4 Cu/Ru-relay catalysis for the construction of cis- and trans-α-AAs and small peptides bearing 1,8-nonadjacent stereocenters with trienyl carbinols. All reactions were carried out with 0.60 mmol 4, 0.20 mmol 1, 0.01 mmol [Cu], 0.011 mmol L7, 0.004 mmol [Ru]-1 or ent-[Ru]-1, and 0.20 mmol K3PO4 in 2 mL of THF at 20 °C for 3–9 h. Isolated yield. The dr and ee values were determined by HPLC analysis. a 40 °C for 12 h. | |
Stereodivergent synthesis
To demonstrate the stereodivergence of this protocol, the transformation between ketimine ester 1a and racemic dienyl carbinol 2a was first carried out by four distinct catalyst systems via the orthogonal permutation of two chiral copper catalysts and two ruthenium catalysts. As shown in Scheme 5, all four stereoisomers of product-(2R,7S,E)-3a, (2R,7R,E)-3a, (2S,7S,E)-3a, and (2S,7R,E)-3a in optically pure form (>99% ee) with exclusive diastereoselectivities (>20
:
1 dr) could be achieved readily in 96–99% yields. In addition, the ketimine amide 1b bearing Gly–Gly–dipeptide and the trienyl carbinol 4a were respectively examined as the reaction partners to further exhibit the stereodivergent synthesis; the corresponding products 3s and 5a with four stereoisomers in each case could be obtained in good to high yields with excellent stereoselectivities (70–99% yield, 13
:
1 → 20
:
1 dr, generally above 99% ee). These results offered profitable evidence to confirm that each chiral catalyst could independently control the asymmetric induction of each step in this cooperative bimetallic Cu/Ru relay-catalyzed cascade dehydrogenation/1,6 or 1,8-Michael addition/hydrogenation reaction.
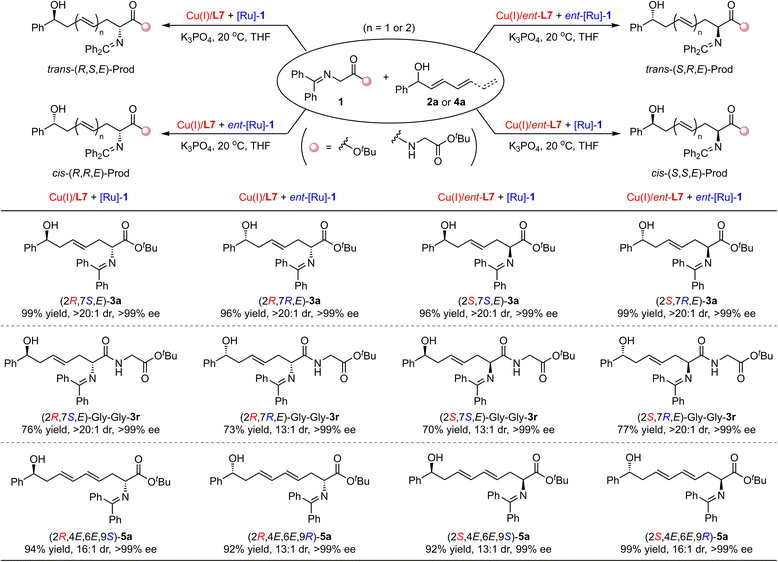 |
| Scheme 5 Representative examples of stereodivergent synthesis to access all four stereoisomers. | |
To our knowledge, the stereodivergent synthesis of chiral molecules with remote and more than two stereocenters has not yet been reported. To further display the excellent robustness of this dual catalytic system, we applied a ketimine amide compound bearing Gly–D-Ala and Gly–L-Ala units, i.e., 1c, respectively, in this cascade transformation. We aimed to achieve the stereodivergent synthesis of chiral hydroxy amino acid derivatives with three stereocenters. As displayed in Scheme 6, we were able to prepare all eight stereoisomers of the desired product 3v containing remote three stereocenters through the cooperation of chiral catalyst pair and substrate enantiomer in good yields with excellent stereoselectivities (45–60% yields, 20
:
1 → 20
:
1 dr). These reaction results demonstrated that the formation of these isomers was achieved well by the control chiral catalyst, but was not affected by the inherent chirality factors from the substrates.
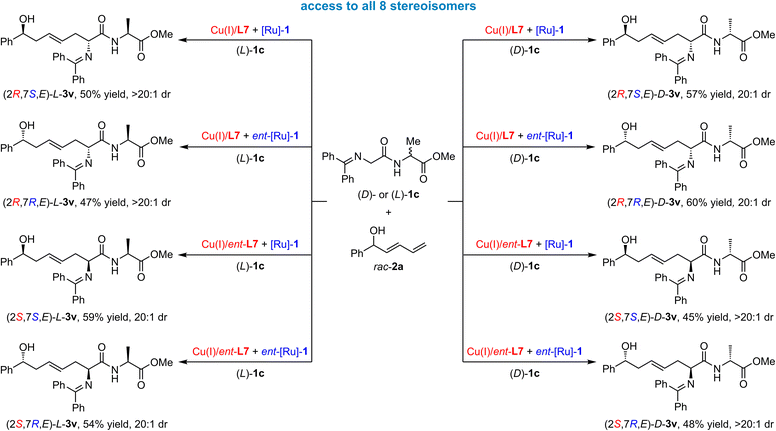 |
| Scheme 6 Stereodivergent synthesis of peptide products with three stereocenters to access all eight stereoisomers. | |
Scale-up experiments and synthetic application
The highly functionalized chiral hydroxy amino ester derivative product renders this protocol a promising tool to access other useful optically active molecules through further synthetic elaborations. As depicted in Scheme 7a, the gram-scale reactions between ketimine ester 1a and racemic dienyl carbinol 2a were carried out in the presence of a [Cu(I)/L7]/[Ru]-1 or [Cu(I)/L7]/ent-[Ru]-1 catalytic system to examine the synthetic practicability; trans-product (2R,7S,E)-3a and cis-product (2R,7R,E)-3a were respectively obtained in high yields and maintained stereoselectivities even under reduced catalyst loading. Product 6 containing a 1,2,3-triazole unit could be efficiently constructed in good yield without loss of stereoselectivities through the Mitsunobu reaction followed by Cu-catalyzed click reaction with phenylacetylene (Scheme 7b). The sequential Mitsunobu reaction/Staudinger reduction of (2R,7S,E)-3a could finally furnish a high yield of enantio-enriched ζ-amino derivative 7 in 76% yield and diastereoselectivity. Acidic hydrolysis of 7 delivered the chiral 1,6-diamine 8 in high yield. The direct hydrogenation of (2R,7S,E)-3a promoted by Pd/C could furnish chiral α-amino ζ-alcohol derivative 9 in 80% yield with >20
:
1 dr. In addition, treatment of (2R,7S,E)-3a with 15% citric acid, followed by amino protection with p-toluenesulfonyl chloride (TsCl), delivered the chiral compound, trans-10, in 78% overall yield with >20
:
1 dr. In addition, I2-enabled endo-trig cyclization of (2R,7S,E)-3a provided polysubstituted tetrahydrofurans, 11 and 11′, in 45% and 27% yield, respectively. The absolute configuration of (2R,7S,E)-trans-10 and its diastereoisomer, (2R,7R,E)-cis-10, were unambiguously determined by X-ray diffraction analysis94 (CCDC 2328948 and 2328949), and those of trans- and cis-products (3 and 5) were deduced based on these results.
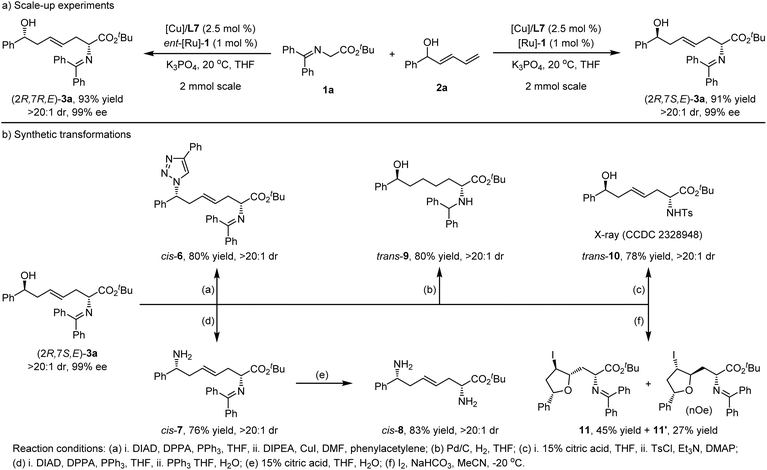 |
| Scheme 7 (a) Scale-up experiments and (b) synthetic elaborations. | |
Kinetic resolution studies
As racemic dienyl carbinol 2a was employed in this cascade dehydrogenation/1,6-Michael addition/hydrogenation reaction, we wondered whether kinetic resolution or dynamic kinetic resolution process was involved in this relay catalytic system. A series of control experiments were carried out to investigate this aspect. As displayed in Scheme 8, the reaction with 0.38 mmol of racemic dienyl carbinol 2a and 0.2 mmol of ketimine ester 1a was carried out under standard reaction conditions; product (2R,7S,E)-3a was obtained in 90% yield (based on 1a) with >20
:
1 dr and 99% ee, and the remaining (R)-2a was recovered in 31% yield (based on racemic 2a) with 93% ee. Meanwhile, the reaction with 0.2 mmol of racemic dienyl carbinol 2a and 0.3 mmol of ketimine ester 1a led to only 45% yield of the product, (2R,7S,E)-3a, with excellent stereoselectivity, along with 43% yield of unreacted (R)-2a with 89% ee. Similar results were observed when [Cu/L7] and ent-[Ru]-1 complexes were used as the cooperative catalysts. These experimental results demonstrate that racemic dienyl carbinol 2a should go through a kinetic resolution in this relay catalysis protocol.
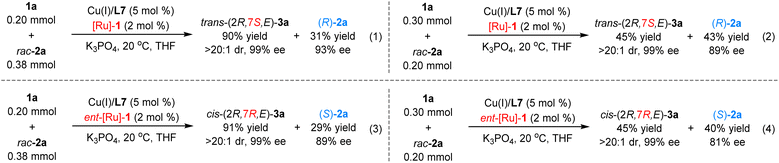 |
| Scheme 8 Kinetic resolution studies. | |
Conclusions
In summary, we have successfully developed the first example of the stereodivergent synthesis of synthetically useful and biologically important chiral molecules containing remote 1,6-nonadjacent stereocenters. The dual Cu/Ru-relay catalysis enabled cascade dehydrogenation/1,6-Michael addition/hydrogenation protocol between ketimine esters and racemic dienyl carbinols, providing an efficient approach to synthesizing a wide range of chiral ζ-hydroxy amino ester derivatives with remote 1,6-nonadjacent stereocenters and a unique β,γ-unsaturation in good to high yields with excellent diastereoselectivities and enantioselectivities. The stereodivergent syntheses of all four stereoisomers of chiral ζ-hydroxy amino ester derivatives could be readily achieved by the orthogonal permutations of a chiral copper catalyst and a chiral ruthenium catalyst. Moreover, the more challenging synthesis among all eight stereoisomers of the desired dipeptide products containing three remote stereocenters was readily accomplished with excellent results through the current cascade relay catalytic system by the cooperation of two chiral catalyst pairs and substrate enantiomers. The synthetic utility of this protocol was demonstrated by a scale-up reaction and further synthetic transformations. Based on the results of the control experiment, the kinetic resolution of racemic dienyl carbinols was involved in this Cu/Ru-relay catalyzed cascade reaction. This current cascade borrowing hydrogen/Michael addition protocol may provide a general avenue for the stereodivergent synthesis of chiral amino acid derivatives bearing remote 1,6-nonadjacent stereocenters with the key features of atom economy, step economy, and redox-neutrality.
Data availability
All experimental procedures, characterisation data, mechanistic investigations, NMR spectra, and HPLC spectra can be found in the ESI.†
Author contributions
C. J. W. conceptualized the project. C. J. W. and X. Q. D. supervised the investigation. H. R. Y., X. C., X. C., and Z. F. W. performed the research. C. J. W. and X. Q. D. co-wrote the paper. All authors analyzed the data, discussed the results, and commented on the manuscript.
Conflicts of interest
There are no conflicts to declare.
Acknowledgements
This work was supported by the National Key R&D Program of China (2023YFA1506700), the National Natural Science Foundation of China (22071186, 22071187, 22101216, 22271226, and 22371216), the National Youth Talent Support Program, and Natural Science Foundation of Hubei Province (2021CFA069). The authors thank Dr Ran Zhang from the Core Facility of Wuhan University for his generous support in the X-ray structures analysis.
Notes and references
- J. W. H. Li and J. C. Vederas, Science, 2009, 325, 161–165 CrossRef PubMed.
- D. J. Newman and G. M. Cragg, Nat. Prod. J., 2007, 70, 461–477 CrossRef CAS PubMed.
- F. Lovering, J. Bikker and C. Humblet, J. Med. Chem., 2009, 52, 6752–6756 CrossRef CAS PubMed.
-
K. Jozwiak, W. J. Lough and I. W. Wainer, Drug Stereochemistry: Analytical Methods and Pharmacology, CRC Press, Boca Raton, 3rd edn, 2012 Search PubMed.
- L. Wei, X. Chang, Z. Zhang, Z.-F. Wang and C.-J. Wang, Chin. Sci. Bull., 2023, 68, 3956–3968 Search PubMed.
- X. Huo, G. Li, X. Wang and W. Zhang, Angew. Chem., Int. Ed., 2022, 61, e202210086 CrossRef CAS PubMed.
- L. Wei and C.-J. Wang, Chin. J. Chem., 2021, 39, 15–24 CrossRef CAS.
- I. P. Beletskaya, C. Nájera and M. Yus, Chem. Rev., 2018, 118, 5080–5200 CrossRef CAS PubMed.
- L. Wei, C. Fu, Z.-F. Wang, H.-Y. Wang and C.-J. Wang, ACS Catal., 2024, 14, 3812–3844 CrossRef CAS.
- L. Lin and X. Feng, Chem.–Eur. J., 2017, 23, 6464–6482 CrossRef CAS PubMed.
- S. Krautwald and E. M. Carreira, J. Am. Chem. Soc., 2017, 139, 5627–5639 CrossRef CAS PubMed.
- L. Wei and C.-J. Wang, Chem Catal., 2023, 3, 100455 CrossRef CAS.
- Y. Wu, X. Huo and W. Zhang, Chem.–Eur. J., 2020, 26, 4895–4916 CrossRef CAS PubMed.
- U. B. Kim, D. J. Jung, H. J. Jeon, K. Rathwell and S.-g. Lee, Chem. Rev., 2020, 120, 13382–13433 CrossRef CAS PubMed.
- G. Zhan, W. Du and Y.-C. Chen, Chem. Soc. Rev., 2017, 46, 1675–1692 RSC.
- Z. Du and Z. Shao, Chem. Soc. Rev., 2013, 42, 1337–1378 RSC.
- A. E. Allen and D. W. C. MacMillan, Chem. Sci., 2012, 3, 633–658 RSC.
- Y. Liu, Y. Chen, A. Yihuo, Y. Zhou, X. Liu, L. Lin and X. Feng, ACS Catal., 2022, 12, 1784–1790 CrossRef CAS.
- D.-X. Zhu, J.-G. Liu and M.-H. Xu, J. Am. Chem. Soc., 2021, 143, 8583–8589 CrossRef CAS PubMed.
- X.-J. Liu, S. Jin, W.-Y. Zhang, Q.-Q. Liu, C. Zheng and S.-L. You, Angew. Chem., Int. Ed., 2020, 59, 2039–2043 CrossRef CAS PubMed.
- Y. Chen, Y. Liu, Z. Li, S. Dong, X. Liu and X. Feng, Angew. Chem., Int. Ed., 2020, 59, 8052–8056 CrossRef CAS PubMed.
- B. M. Trost, C.-I. Hung, T. Saget and E. Gnanamani, Nat. Catal., 2018, 1, 523–530 CrossRef CAS.
- B. Simmons, A. M. Walji and D. W. C. MacMillan, Angew. Chem., Int. Ed., 2009, 48, 4349–4353 CrossRef CAS PubMed.
- J. Zhang, W. Zhu, Z. Chen, Q. Zhang and C. Guo, J. Am. Chem. Soc., 2024, 146, 1522–1531 CrossRef CAS PubMed.
- Y.-H. Wen, F. Yang, S. Li, X. Yao, J. Song and L.-Z. Gong, J. Am. Chem. Soc., 2023, 145, 4199–4207 CrossRef CAS PubMed.
- C. Sheng, Z. Ling, J. Xiao, K. Yang, F. Xie, S. Ma and W. Zhang, Angew. Chem., Int. Ed., 2023, 62, e202305680 CrossRef PubMed.
- Y. Luo, Y. Ma, G. Li, X. Huo and W. Zhang, Angew. Chem., Int. Ed., 2023, 62, e202313838 CrossRef CAS PubMed.
- J. Han, R. Liu, Z. Lin and W. Zi, Angew. Chem., Int. Ed., 2023, 62, e202215714 CrossRef CAS PubMed.
- Q. Zhang, M. Zhu and W. Zi, Chem, 2022, 8, 2784–2796 CAS.
- Y. Xu, H. Wang, Z. Yang, Y. Zhou, Y. Liu and X. Feng, Chem, 2022, 8, 2011–2022 CAS.
- L. Xiao, X. Chang, H. Xu, Q. Xiong, Y. Dang and C.-J. Wang, Angew. Chem., Int. Ed., 2022, 61, e202212948 CrossRef CAS PubMed.
- T. P. Le, S. Tanaka, M. Yoshimura, K. Sato and M. Kitamura, Nat. Commun., 2022, 13, 5876 CrossRef CAS PubMed.
- Z. He, L. Peng and C. Guo, Nat. Synth., 2022, 1, 393–400 CrossRef.
- M. Zhu, Q. Zhang and W. Zi, Angew. Chem., Int. Ed., 2021, 60, 6545–6552 CrossRef CAS PubMed.
- L. Xiao, L. Wei and C.-J. Wang, Angew. Chem., Int. Ed., 2021, 60, 24930–24940 CrossRef CAS PubMed.
- H. Wang, R. Zhang, Q. Zhang and W. Zi, J. Am. Chem. Soc., 2021, 143, 10948–10962 CrossRef CAS PubMed.
- Y. Peng, X. Huo, Y. Luo, L. Wu and W. Zhang, Angew. Chem., Int. Ed., 2021, 60, 24941–24949 CrossRef CAS PubMed.
- B. Kim, Y. Kim and S. Y. Lee, J. Am. Chem. Soc., 2021, 143, 73–79 CrossRef CAS PubMed.
- X. Huo, L. Zhao, Y. Luo, Y. Wu, Y. Sun, G. Li, T. Gridneva, J. Zhang, Y. Ye and W. Zhang, CCS Chem., 2021, 4, 1720–1731 CrossRef.
- S. Singha, E. Serrano, S. Mondal, C. G. Daniliuc and F. Glorius, Nat. Catal., 2020, 3, 48–54 CrossRef CAS.
- L. Peng, Z. He, X. Xu and C. Guo, Angew. Chem., Int. Ed., 2020, 59, 14270–14274 CrossRef CAS PubMed.
- R. He, X. Huo, L. Zhao, F. Wang, L. Jiang, J. Liao and W. Zhang, J. Am. Chem. Soc., 2020, 142, 8097–8103 CrossRef CAS PubMed.
- Q. Zhang, H. Yu, L. Shen, T. Tang, D. Dong, W. Chai and W. Zi, J. Am. Chem. Soc., 2019, 141, 14554–14559 CrossRef CAS PubMed.
- S.-M. Xu, L. Wei, C. Shen, L. Xiao, H.-Y. Tao and C.-J. Wang, Nat. Commun., 2019, 10, 5553 CrossRef PubMed.
- Z.-T. He, X. Jiang and J. F. Hartwig, J. Am. Chem. Soc., 2019, 141, 13066–13073 CrossRef CAS PubMed.
- L. Wei, Q. Zhu, S.-M. Xu, X. Chang and C.-J. Wang, J. Am. Chem. Soc., 2018, 140, 1508–1513 CrossRef CAS PubMed.
- X. Jiang, P. Boehm and J. F. Hartwig, J. Am. Chem. Soc., 2018, 140, 1239–1242 CrossRef CAS PubMed.
- X. Huo, J. Zhang, J. Fu, R. He and W. Zhang, J. Am. Chem. Soc., 2018, 140, 2080–2084 CrossRef CAS PubMed.
- X. Jiang, J. J. Beiger and J. F. Hartwig, J. Am. Chem. Soc., 2017, 139, 87–90 CrossRef CAS PubMed.
- F. A. Cruz and V. M. Dong, J. Am. Chem. Soc., 2017, 139, 1029–1032 CrossRef CAS PubMed.
- X. Huo, R. He, X. Zhang and W. Zhang, J. Am. Chem. Soc., 2016, 138, 11093–11096 CrossRef CAS PubMed.
- S. Krautwald, M. A. Schafroth, D. Sarlah and E. M. Carreira, J. Am. Chem. Soc., 2014, 136, 3020–3023 CrossRef CAS PubMed.
- S. Krautwald, D. Sarlah, M. A. Schafroth and E. M. Carreira, Science, 2013, 340, 1065–1068 CrossRef CAS PubMed.
- C. You, M. Shi, X. Mi and S. Luo, Nat. Commun., 2023, 14, 2911 CrossRef CAS PubMed.
- J.-H. Xie, Y.-M. Hou, Z. Feng and S.-L. You, Angew. Chem., Int. Ed., 2023, 62, e202216396 CrossRef CAS PubMed.
- J. Qi, T. Song, Z. Yang, S. Sun, C.-H. Tung and Z. Xu, ACS Catal., 2023, 13, 2555–2564 CrossRef CAS.
- J. Dai, L. Li, R. Ye, S. Wang, Y. Wang, F. Peng and Z. Shao, Angew. Chem., Int. Ed., 2023, 62, e202300756 CrossRef CAS PubMed.
- J. Zhang, X. Huo, J. Xiao, L. Zhao, S. Ma and W. Zhang, J. Am. Chem. Soc., 2021, 143, 12622–12632 CrossRef CAS PubMed.
- S.-Q. Yang, Y.-F. Wang, W.-C. Zhao, G.-Q. Lin and Z.-T. He, J. Am. Chem. Soc., 2021, 143, 7285–7291 CrossRef CAS PubMed.
- M.-M. Zhang, Y.-N. Wang, B.-C. Wang, X.-W. Chen, L.-Q. Lu and W.-J. Xiao, Nat. Commun., 2019, 10, 2716 CrossRef PubMed.
- C. Fu, L. He, X. Chang, X. Cheng, Z.-F. Wang, Z. Zhang, V. A. Larionov, X.-Q. Dong and C.-J. Wang, Angew. Chem., Int. Ed., 2024, 63, e202315325 CrossRef CAS PubMed.
- X. Chang, X. Cheng, X.-T. Liu, C. Fu, W.-Y. Wang and C.-J. Wang, Angew. Chem., Int. Ed., 2022, 61, e202206517 CrossRef CAS PubMed.
- J. Masson-Makdissi, L. Prieto, X. Abel-Snape and M. Lautens, Angew. Chem., Int. Ed., 2021, 60, 16932–16936 CrossRef CAS PubMed.
- T. Li, L. Luo, X. Cheng and Z. Lu, ACS Catal., 2024, 14, 3278–3286 CrossRef CAS.
- Y. Gao, G. Hong, B.-M. Yang and Y. Zhao, Chem. Soc. Rev., 2023, 52, 5541–5562 RSC.
- B. G. Reed-Berendt, D. E. Latham, M. B. Dambatta and L. C. Morrill, ACS Cent. Sci., 2021, 7, 570–585 CrossRef CAS PubMed.
- T. Irrgang and R. Kempe, Chem. Rev., 2019, 119, 2524–2549 CrossRef CAS PubMed.
- A. Corma, J. Navas and M. J. Sabater, Chem. Rev., 2018, 118, 1410–1459 CrossRef CAS PubMed.
- Q. Yang, Q. Wang and Z. Yu, Chem. Soc. Rev., 2015, 44, 2305–2329 RSC.
- N. Z. Burns, P. S. Baran and R. W. Hoffmann, Angew. Chem., Int. Ed., 2009, 48, 2854–2867 CrossRef CAS PubMed.
- P. A. Wender, V. A. Verma, T. J. Paxton and T. H. Pillow, Acc. Chem. Res., 2008, 41, 40–49 CrossRef CAS PubMed.
- B. M. Trost, Science, 1991, 254, 1471–1477 CrossRef CAS PubMed.
- L. Wei, X. Chang and C.-J. Wang, Acc. Chem. Res., 2020, 53, 1084–1100 CrossRef CAS PubMed.
- L. Wei, L. Xiao, Y. Hu, Z. Wang, H. Tao and C. Wang, Chin. J. Org. Chem., 2019, 39, 2119–2130 CrossRef CAS.
- Z.-Y. Xue, Q.-H. Li, H.-Y. Tao and C.-J. Wang, J. Am. Chem. Soc., 2011, 133, 11757–11765 CrossRef CAS PubMed.
- K. Ersmark, J. R. Del Valle and S. Hanessian, Angew. Chem., Int. Ed., 2008, 47, 1202–1223 CrossRef CAS PubMed.
- D. H. R. Barton, S. D. Gero, F. Lawrence, M. Robert-Gero, B. Quiclet-Sire and M. Samadi, J. Med. Chem., 1992, 35, 63–67 CrossRef CAS PubMed.
- H. Wojciechowska, A. Konitz and E. Borowski, Int. J. Pept. Protein Res., 1985, 26, 279–293 CrossRef CAS.
- Y.-C. Wang, Z.-X. Xiao, M. Wang, S.-Q. Yang, J.-B. Liu and Z.-T. He, Angew. Chem., Int. Ed., 2023, 62, e202215568 CrossRef CAS PubMed.
- P. H. Poulsen, K. S. Feu, B. M. Paz, F. Jensen and K. A. Jørgensen, Angew. Chem., Int. Ed., 2015, 54, 8203–8207 CrossRef CAS PubMed.
- Y. Hayashi, D. Okamura, S. Umemiya and T. Uchimaru, ChemCatChem, 2012, 4, 959–962 CrossRef CAS.
- L. Bernardi, J. López-Cantarero, B. Niess and K. A. Jørgensen, J. Am. Chem. Soc., 2007, 129, 5772–5778 CrossRef CAS PubMed.
- T. Nishimura, Y. Yasuhara and T. Hayashi, Angew. Chem., Int. Ed., 2006, 45, 5164–5166 CrossRef CAS PubMed.
- J. W. Ralls, Chem. Rev., 1959, 59, 329–344 CrossRef CAS.
- K. Ren, L. Zhang, B. Hu, M. Zhao, Y. Tu, X. Xie, T. Y. Zhang and Z. Zhang, ChemCatChem, 2013, 5, 1317–1320 CrossRef CAS.
- J. R. Zbieg, E. Yamaguchi, E. L. McInturff and M. J. Krische, Science, 2012, 336, 324–327 CrossRef CAS PubMed.
- A. Morita, T. Misaki and T. Sugimura, Chem. Lett., 2014, 43, 1826–1828 CrossRef.
- T. Nishimura, A. Noishiki and T. Hayashi, Chem. Commun., 2012, 48, 973–975 RSC.
- C. J. Richards and A. W. Mulvaney, Tetrahedron: Asymmetry, 1996, 7, 1419–1430 CrossRef CAS.
- F. Chen, D. He, L. Chen, X. Chang, D. Z. Wang, C. Xu and X. Xing, ACS Catal., 2019, 9, 5562–5566 CrossRef CAS.
- W. Baratta, E. Herdtweck, K. Siega, M. Toniutti and P. Rigo, Organometallics, 2005, 24, 1660–1669 CrossRef CAS.
- J. F. Teichert and B. L. Feringa, Angew. Chem., Int. Ed., 2010, 49, 2486–2528 CrossRef CAS PubMed.
- Asymmetric hydrogenation of simple dialkyl ketone is still a significant challenge.
For a recent example, see: F.-H. Zhang, F.-J. Zhang, M.-L. Li, J.-H. Xie and Q.-L. Zhou, Nat. Catal., 2020, 3, 621–627 CrossRef CAS.
- Deposition numbers 2328948 (for (2R,7S,E)-trans-10), and 2328949 (for (2R,7R,E)-cis-10) contain the supplementary crystallographic data for this paper.
|
This journal is © The Royal Society of Chemistry 2024 |