DOI:
10.1039/D4SC00954A
(Edge Article)
Chem. Sci., 2024,
15, 11374-11381
Chiral three-dimensional organic–inorganic lead iodide hybrid semiconductors†
Received
8th February 2024
, Accepted 13th June 2024
First published on 14th June 2024
Abstract
Chiral hybrid metal halides (CHMHs) have received a considerable amount of attention in chiroptoelectronics, spintronics, and ferroelectrics due to their superior optoelectrical properties and structural flexibility. Owing to limitations in synthesis, the theoretical prediction of room-temperature stable chiral three-dimensional (3D) CHFClNH3PbI3 has not been successfully prepared, and the optoelectronic properties of such structures cannot be studied. Herein, we have successfully constructed two pairs of chiral 3D lead iodide hybrids (R/S/Rac-3AEP)Pb2I6 (3R/S/Rac, 3AEP = 3-(1-aminoethyl)pyridin-1-ium) and (R/S/Rac-2AEP)Pb2I6 (2R/S/Rac, 2AEP = 2-(1-aminoethyl)pyridin-1-ium) through chiral introduction and ortho substitution strategies, and obtained bulk single crystals of 3R/S/Rac. The 3R/S exhibits optical activity and bulk photovoltaic effect induced by chirality. The 3R crystal device exhibits stable circularly polarized light performance at 565 nm with a maximum anisotropy factor of 0.07, responsivity of 0.25 A W−1, and detectivity of 3.4 × 1012 jones. This study provides new insights into the synthesis of chiral 3D lead halide hybrids and the development of chiral electronic devices.
Introduction
Chirality is one of the most essential characteristics in living organisms and nature universally,1,2 and is necessary for complex biometric recognition and replication functions in living systems.3 An object is chiral when its mirror image cannot be superimposed with the original object.4,5 In materials science, chirality is often used to represent an important non-centrosymmetric structural feature that can be obtained by introducing chiral molecules.6 The introduction of chirality usually brings optical rotation,7 circular dichroism (CD),8 and circularly polarized light (CPL) emission4,9 in materials. In some cases, it can even induce non-centrosymmetry-related properties such as the bulk photovoltaic effect (BPVE),10–12 second-harmonic generation,13–15 piezoelectricity,16,17 ferroelectricity,18–20 Rashba splitting,21,22 and topological quantum properties.23 Although organic chiral materials exhibiting strong chiral activity in the near-ultraviolet region are widely available, the charge transfer capability of actual polarized optoelectronic devices is poor,5 and there is an urgent need to develop new chiral lead iodide hybrids with strong absorption capacity and a broad light spectrum.
Metal halide hybrids, especially three-dimensional (3D) lead halide hybrids, have attracted great attention from researchers due to their excellent properties such as long carrier diffusion lengths,24–27 high carrier mobility,28,29 bandgap tunability,30 low trap densities,31 and high power conversion efficiency.32 The continuous breakthrough of the record photoelectric conversion record efficiency of single-junction lead halide hybrid solar cells has completely triggered the research boom of lead halide hybrid semiconductor materials.32 In addition, the structural diversity of organic cations in metal halides and the ionic properties of the inorganic framework make it possible to directly obtain chiral metal halides by introducing chiral cations. The introduction of chirality extends the excellent optoelectronic properties of metal halides to the field of chiral electronics, greatly expanding their application range and potential.4,33–35 Tang et al. reported a CPL detector based on a 1D (R/S-PEA)PbI3 (PEA = phenethylammonium) structure, providing an effective method for the direct detection of CPL.36 In 2019, Xiong et al. reported a pair of 2D chiral metal halide ferroelectrics (R/S-CMBA)2PbI4 (CMBA = 1-(4-chlorophenyl)ethylammonium).16 In 2020, Fu et al. reported the chiral ferroelectric (R/S-3-FPRD)MnBr3 (3-FPRD = 3-fluoropyrrolidinium) with CPL emission properties; the luminescence asymmetry factor |glum| is 6.1 × 10−3, and the photoluminescence quantum yield is 28.31%.37 Recently, Luo et al. constructed a pair of 3D chiral lead chloride hybrids (R/S-BPEA)EA6Pb4Cl15 (BPEA = 1-4-bromophenylethylammonium, EA = ethylammonium),38 but due to the large cavity of their inorganic framework, their band gap is much larger than that of the reported 3D lead iodide hybrids.39–41 However, until now, the chiral 3D lead iodide hybrids, which are theoretically predicted to be both thermodynamically and kinetically stable, have been rarely reported.2
Inspired by the organic-to-inorganic structural chirality transfer effect in chiral hybrid metal halides (CHMHs), we know that the introduction of chiral cations into the inorganic framework is the most direct and effective way to obtain CHMHs.5 Here, using the 3D lead iodide hybrid (3AMPY)Pb2I6 (3AMPY = 3-aminomethylpyridinium) as a template and introducing methyl and ortho substitution strategies in the divalent cation 3AMPY (Scheme 1a and b), two pairs of 3D chiral lead iodide hybrids (R/S-3AEP)Pb2I6 (3R/S/Rac, 3AEP = 3-(1-aminoethyl)pyridin-1-ium) and (R/S-2-AEP)Pb2I6 (2R/S/Rac, 2AEP = 2-(1-aminoethyl)pyridin-1-ium) were synthesized, and bulk single crystals of 3R/S/Rac were obtained. We find the BPVE under white-light irradiation in 3R/S. The sign of zero-bias photocurrent is altered depending on the configuration of the chirality R/S-3AEP. 3R/S also exhibits chiroptical properties and the Rashba effect with a Rashba coefficient (αR) of 0.23 eV Å.
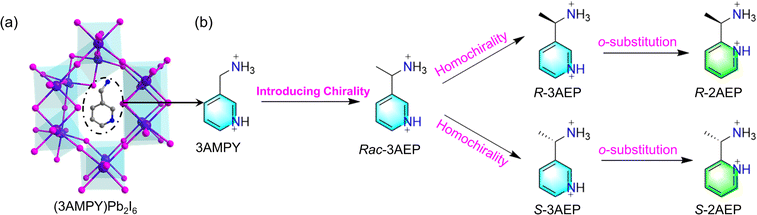 |
| Scheme 1 (a) Crystal structure of (3AMPY)Pb2I6. (b) Molecular design for 3D chiral lead iodide hybrids. | |
Results and discussion
Synthesis
We first referred to the synthesis method of (3AMPY)Pb2I6 reported in the literature (Fig. 1a)39 and tried to use a 4
:
1 ratio of Pb2+ to the 3-AMPY starting material to obtain the chiral 3D metal halide hybrids. However, we did not obtain the desired 3D structure, but only a 2D structure under this condition. We guessed that the introduction of the methyl group may increase the steric hindrance, leading to an increase in the synthesized energy barrier. This may be overcome by increasing the proportion of Pb2+. We then investigated the 5
:
1, 6
:
1, and 7
:
1 ratios of Pb2+ and the organic cation (Fig. 1b and c), and found that 3D structures can be obtained at 6
:
1 and higher ratios (Fig. 1c). However, a similar 3D lead iodide hybrid (M2pda)Pb2I6 (M2pda = N-methyl-1,3-propanediammonium) was found to be synthesized with a 3
:
1 ratio of starting materials,41 which inspired us that reaction conditions play an important role in the structural dimensions of metal halides, especially the proportion of starting materials and reaction temperature.
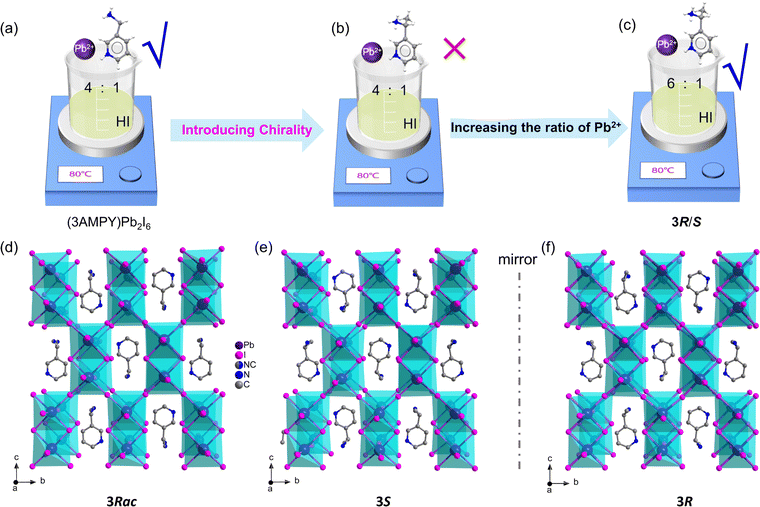 |
| Fig. 1 (a) Synthesis of (3AMPY)Pb2I6. (b and c) Synthesis of 3R/S. Crystal structures of (d) 3Rac, (e) 3S, and (f) 3R. | |
Two pairs of chiral 3D lead iodide compounds 2R/S/Rac and 3R/S/Rac were thus synthesized with bivalent chiral cations as shown in Scheme 1b and Fig. S1.† Red block single crystals of 3R/S/Rac with a size of 5 × 3 × 3.0 mm3 were obtained by slowly evaporating the solutions at 80 °C (Fig. 1c and S2†). However, due to the solubility of 2R/S/Rac and growth temperature constraints, a pure phase cannot be obtained, so the structures and properties of 3R/S/Rac are studied below.
The purity of the phase was confirmed by powder X-ray diffraction (Fig. S3†). The infrared spectrum of the compounds shows several vibrational peaks at 1480, 3000, and 3500 cm−1, which are attributed to the vibration absorption of N–H, C–H, C
C, and C
N bonds, respectively, demonstrating the successful introduction of the Rac/R/S-3AEP divalent cation into the 3D inorganic lattices (Fig. S4†). Thermogravimetric analysis shows the thermal stability of 3R/S/Rac up to about 575 K (Fig. S5†), comparable to that of (M2pda)Pb2I641and (Dmpz)Pb2I6.40
Crystal structure
Single-crystal XRD analysis shows that 3Rac crystallizes in the centrosymmetric space group Cmca, with cell parameters a = 12.9279(4) Å, b = 18.7439(6) Å, c = 17.6358(5) Å, and α = β = γ = 90° (Table 1). In the 3Rac structure, a mirror passes through the cation to result in a static disorder of the substituents of the pyridine ring (Fig. S6† and 1b). Due to the existence of the structural phase transition in 3R/S, we first analyze the structural characteristics of the low-temperature ordered state. Compounds 3S and 3R are all crystallized in the chiral space group C2221 at 240 K with the cell parameters of a = 12.9335(5) Å, b = 18.7439(8) Å, c = 17.6358(8) Å, and α = β = γ = 90° (Table 1). Due to the introduction of enantiomerically chiral cations, the crystal packing structures of the 3S and 3R exhibit mirror symmetry (Fig. 1e and f). To our knowledge, this is the first report of chiral 3D hybrid lead halides formed from lead halide octahedral dimers (Table S1†). The structure of 2R/S/Rac adopts the same space group as 3R/S/Rac (Table 1).
Table 1 Crystal and refinement data for compounds reported here
Compound |
(R-3AEP)Pb2I6 |
(S-3AEP)Pb2I6 |
(Rac-3AEP)Pb2I6 |
R
1 = Σ| |Fo| – |Fc| |/|Fo|.
wR2 = [Σw(Fo2 – Fc2)2]/Σw(Fo2)2]1/2.
|
Formula |
C7H12N2Pb2I6 |
C7H12N2Pb2I6 |
C7H12N2Pb2I6 |
Formula weight |
1299.97 |
1299.97 |
1299.97 |
Crystal system |
Orthorhombic |
Orthorhombic |
Orthorhombic |
Space group |
C2221 |
C2221 |
Cmca
|
a/Å |
12.9493(3) |
12.9335(5) |
12.9279(4) |
b/Å |
18.7108(5) |
18.7439(8) |
18.7521(6) |
c/Å |
17.6439(5) |
17.6358(8) |
17.6600(5) |
α, β, γ/° |
90 |
90 |
90 |
V/Å3 |
4274.97(19) |
4273.3(3) |
4281.20(2) |
Flack parameter |
−0.016(16) |
0.00(3) |
— |
R
int
|
0.038 |
0.066 |
0.083 |
R
1
/wR2b (I > 2σ(I)) |
0.0606, 0.1713 |
0.0684, 0.1748 |
0.0561, 0.1520 |
R
1/wR2 (all data) |
0.0692, 0.1785 |
0.0900, 0.1865 |
0.0667, 0.1619 |
GOF |
1.064 |
1.02 |
1.036 |
Compound |
(R-2AEP)Pb2I6 |
(S-2AEP)Pb2I6 |
(Rac-2AEP)Pb2I6 |
Formula |
C7H12N2Pb2I6 |
C7H12N2Pb2I6 |
C7H12N2Pb2I6 |
Formula weight |
1299.97 |
1299.97 |
1299.97 |
Crystal system |
Orthorhombic |
Orthorhombic |
Orthorhombic |
Space group |
C2221 |
C2221 |
Cmca
|
a/Å |
12.9873(5) |
12.9972(3) |
12.9548(6) |
b/Å |
18.8387(7) |
18.8397(7) |
18.8648(9) |
c/Å |
17.5741(8) |
17.5687(6) |
17.6101(7) |
α, β, γ/° |
90 |
90 |
90 |
V/Å3 |
4299.7(3) |
4301.9(2) |
4303.7(3) |
Flack parameter |
0.006(8) |
0.002(15) |
— |
R
int
|
0.0289 |
0.0593 |
0.0394 |
R
1
/wR2b (I > 2σ(I)) |
0.0286, 0.0593 |
0.0681, 0.1653 |
0.0517, 0.1058 |
R
1/wR2 (all data) |
0.0361, 0.0612 |
0.0856, 0.1807 |
0.0762, 0.1151 |
GOF |
0.974 |
1.005 |
1.107 |
The basic structural motif of (AEP)Pb2I6 adopts a rare 3D lead iodide anion framework of AM2I6, where A is the bulky cation template for the inorganic lead iodide framework. The crystal structure contains 3D inorganic lead iodide inorganic frameworks of corner- and edge-sharing PbI6 octahedra with vacancies occupied by the 3AEP organic cations. Four edge-sharing dimers are connected by the corners of the individual layers to form a continuous layer with rectangular voids extending along the bc crystal plane. The layers are stacked along the a-axis by angle-to-angle connections to form an anionic 3D inorganic framework (Fig. 1c and S7†). The rectangular void in the inorganic skeleton differs from the parallelogram void induced by the chain alkyl diamine in the (M2pda)Pb2I6,41 which is caused by the template effect of the characteristic size and rigid shape of 3AEP. The asymmetric unit consists of one 3AEP divalent cation, two Pb ions, and six I ions (Fig. S8a†). The 3AEP cations are located in the plane of the layer, perpendicular to the stacking direction in the pores. They are bonded to the 3D inorganic framework by the hydrogen bond interaction of N–H⋯I and C–H⋯I, with the bond lengths of N–H⋯I ranging from 2.85 to 3.2 Å and C–H⋯I ranging from 3.93 to 4.16 Å (Fig. S8b and c†). Hirshfeld surface analysis reveals a predominant role of the hydrogen bonding interactions of H⋯I and additional van der Waals interactions of C⋯I between the cations and the I anions (Fig. S9†). Rich hydrogen bonding and van der Waals interactions promote effective chiral transfer of the chiral cations to the inorganic sublattices, with 21 screw axes crossing the corner-sharing octahedral bridging iodine atoms and twisted Pb–I planes along the a and c directions, respectively (Fig. S8d and Table S2†).
Structural phase transition properties
Structural properties were analyzed in detail by taking 3R as an example. The thermal properties of 3R during the structural phase transition were investigated. As shown in Fig. 2a, 3R exhibited two pairs of obvious endothermic and exothermic peaks at 293/275 K (T1) and 419/414 K (T2), indicating two reversible structural phase transitions. For the convenience of analysis, the phase below T1 is referred to as the low temperature phase (LTP), the phase between T1 and T2 as the intermediate temperature phase (ITP), and the phase above T2 as the high-temperature phase (HTP). At T1, there is a thermal hysteresis of 18 K with a phase transition centered at 284 K, while at T2 the thermal hysteresis is only 5 K with a phase transition center of 417 K. The ΔH at T1 is 5.88 kJ mol−1 and that at T2 is 0.71 kJ mol−1. As can be seen from Fig. 2b, 3Rac does not undergo any structural phase transition in the corresponding temperature range. The temperature-dependent dielectric constant of 3R crystalline powder was measured. As shown in Fig. 2c, the dielectric anomalies occur in the process of temperature change at different test frequencies. An obvious step-type dielectric anomaly can be seen from the 1 MHz curve, which confirms the DSC test results (Fig. 2a).
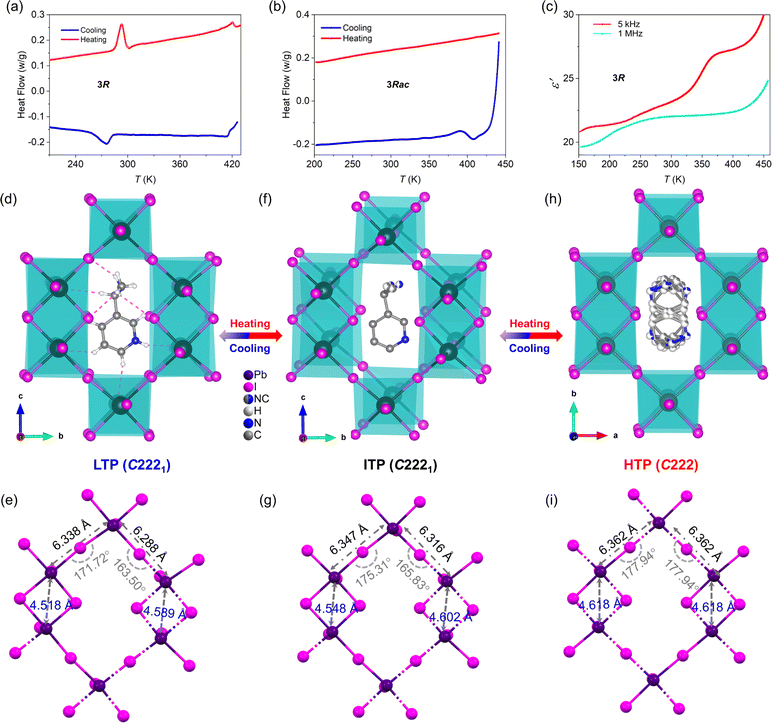 |
| Fig. 2 (a) DSC curves of 3R. (b) DSC curves of 3Rac. (c) Dielectric transition of 3R. Crystal structure of 3R in the ordered LTP (d), and disordered ITP (f), HTP (h) and the corresponding connection motifs (e, g, and i). | |
When the temperature increased to 298 K, the compound underwent an order–disorder phase transition due to the disordered substituents on the pyridine ring. The ordered and disordered cationic terminal groups did not cause changes in the space group (cell parameters, a = 12.9629(5) Å, b = 18.7955(5) Å, c = 17.6714(8) Å, α = β = γ = 90°) (Tables S3 and S4†). However, the bond length and bond angle of the inorganic anion skeleton are extended and enlarged with increasing temperature, which is a typical effect of thermal expansion and contraction. With the further increase of temperature to 425 K, the whole cations in the inorganic framework undergo a violent disorder, causing the space group to change to C222 with higher symmetry (cell parameters, a = 9.4786(5) Å, b = 17.723(10) Å, c = 6.5033(8) Å, and α = β = γ = 90°) (Tables S3 and S4†). In the HTP, there are three mutually perpendicular 2-fold axes passing through the 3-AEP cation (Fig. S10†), corresponding to the highly disordered state. The bond lengths and bond angles of the inorganic skeleton have further lengthened and become larger (Fig. 2e, g and i). The Pb–I–Pb bond angles belonging to the canonical angle shared bond have not yet reached the standard 180° (Table S5†), and there are still tiny distortions in the structure (Fig. 2i).
Semiconducting properties and the BPVE
DFT calculations were performed based on the 240 K crystal structure of 3R using the exchange–correlation functional PBE (Fig. 3a and b). Both the valence and conduction bands exhibit dispersion, but the degree of dispersion is smaller than that of CH3NH3PbI3 due to the relatively lower octahedral edge-sharing connectivity in the structure.42 The data show that 3R is a direct bandgap semiconductor with a bandgap value of 2.04 eV at the Y-point without spin–orbit coupling (SOC) (Fig. 3a), and turns to an indirect one with a bandgap value of 1.48 eV due to the Rashba spin splitting effect (Fig. 3b). Our PBE density of states (DOS) data show that Pb 6s and I 5p orbitals overlap to form the valence band maximum (VBM), and Pb 6p orbitals overlap to form the conduction band minimum (CBM), indicating that the energy band and semiconductor performance are mainly determined by the inorganic components (Fig. 3b). We find electronic states derived from the molecular sublattice ∼ 0.4 eV above the conduction band minimum. Higher accuracy electronic-structure approaches such as hybrid functionals or many-body perturbation theory and the inclusion of thermal broadening effects might lead to the participation of these molecular electronic states in the conduction band edge. Ultraviolet-visible diffuse-reflectance spectra of 3R, 3S, and 3Rac are shown in Fig. 3c. There is significant absorption in the ultraviolet region, and the edge of the band extends into the visible light region near 600 nm. Based on the Kubelka–Munk equation,43,44 the optical bandgap value is estimated to be 2.13 eV (Fig. 3a), which is smaller than the bandgap of common 2D lead iodide semiconductor materials,45 and is comparable to that of chiral quasi-2D lead iodide hybrid (R/S-MPA)2(MA)Pb2I7 (MA = methylammonium, MPA = methylphenethylammonium) (∼2.08 eV).10
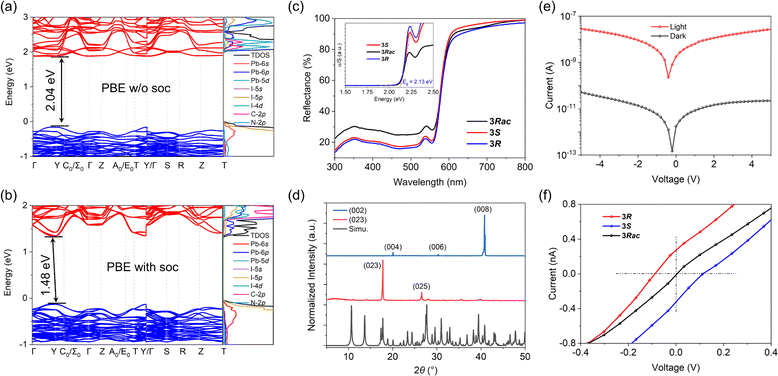 |
| Fig. 3 (a) DFT-PBE and (b) DFT-PBE + SOC band structures and projected density of states (PDOS) of 3R. (c) UV-vis diffuse-reflectance spectra of 3R/S/Rac. Inset: optical bandgap derived from the Kubelka–Munk function based on diffuse-reflectance data. (d) PXRD patterns of the single crystal were recorded from the (023) and (001) planes. (e) I–V curves recorded in the dark and under 100 mW cm−2 illumination along the [100]. (f) Bulk photovoltaic effect of 3R/S. | |
The dark current and on/off ratio are the two main parameters that affect the optoelectronic properties of semiconductors. The dark current of the 3R single crystal device along the [100] direction in a dark environment is as low as 10−10 A (Fig. 3d and e), which is much lower than 10−7 A of MAPbI3.46 Under sunlight irradiation of 100 mW cm−2, the photocurrent rapidly increases to 3.6 × 10−7 A, and the on/off ratio is greater than 4 × 103, consistent with our previously reported results.41 The BPVE induced by chirality was observed in the I–V curve for the [001] parallel alignment with a zero-bias photocurrent with a short-circuit current |Isc| of ∼0.37 nA and an open-circuit voltage |Voc| of ∼0.11 V for both 3R and 3S (Fig. 3f). The Isc and Voc values are larger than those of reported quasi-2D CHMHs and (R/S-MPA)2(MA)Pb2I7.10 No BPVE phenomenon was observed in 3Rac (Fig. 3f).
Chiroptical properties and the Rashba–Dresselhaus effect
The CD spectra of 3R and 3S were measured on the crystal powders. In the wavelength range of 200–580 nm, there is a clear CD signal at the edge of the absorption band and exciton peak at 565 nm, extending to 580 nm. The enantiomers show the same signal size but an opposite sign of the absorption peaks at 565 nm, a typical Cotton effect in the CD spectrum (Fig. 4a). In contrast, 3Rac has no characteristic absorption in the CD spectrum (Fig. 4a). At the same time, the UV-vis absorption spectra in the 200–580 nm range are consistent with the characteristic peaks of the CD absorption spectra (Fig. 3b). These results confirm the effective chiral transfer from the chiral cations to the inorganic sublattices. The calculated gCD is 4.1 × 10−6 at 565 nm (Fig. 4b). Based on a 3R single crystal device (Fig. 4c), we investigated its response to 565 nm CPL. The anisotropy factor for photocurrent (gIph) is up to 0.08 at a bias of 5 V and at 0.17 mW cm−2 (Fig. 4d). This is smaller than the reported low-dimensional CHMH detector gIph,22,36,47 and we speculate that is caused by the order–disorder motion of the cations at room temperature, as this reduces the efficiency of chiral transfer from the chiral cations to the inorganic framework,48 thereby reducing the gIph. Although the chiral optical properties of our materials are still unsatisfactory due to the disorder of the cations, it can be foreseen that the introduction of room-temperature ordered chiral cations into 3D lead iodide structures will greatly improve the chiroptical properties of such materials. In addition to the CPL sensing, the device also exhibits good photoresponses. The Iph/Idark ratio at 565 nm was obtained by measuring the I–V curves in the dark and under different illumination intensities from 0–0.17 mW cm−2 (Fig. 4e). The measured responsivity and specific detectivity without frequency modulation are 0.25 A W−1 and 3.2 × 1012 jones, respectively (Fig. 4f). These merits indicate that the single-crystal device is a good candidate for CPL detection.
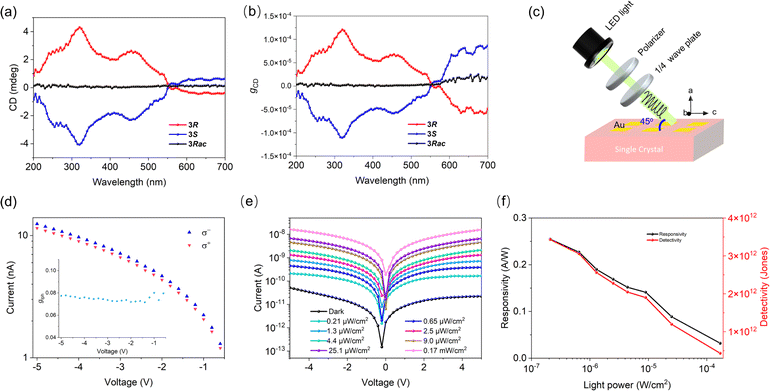 |
| Fig. 4 (a) CD and (b) gCD spectra of 3R/S and 3Rac. (c) Device architecture for the CPL detection of 3R. (d) I–V curves of the 3R photodetector under R-CPL and L-CPL illumination at 565 nm with an intensity of 0.17 mW cm−2, as well as the calculated gIph. (e) I–V curves under different illumination intensities at 565 nm; (f) power intensity dependence of R and D* at 565 nm. | |
The combination of heavy element Pb and non-centrosymmetric structures (chirality) leads to the Rashba–Dresselhaus (RD) spin splitting effect. The possible existence of RD spin splitting is verified by first-principles calculation. As shown in Fig. 5a and b, band splitting occurs at the Y point at the base of the conduction band (Fig. 5b). The RD splitting coefficient is defined as αRD = 2ERD/Δk, where ERD is the energy splitting and Δk is the momentum shift. Fig. 5b shows that the ERD along the Γ–Y path is 3 meV, the Δk is 0.0285 Å−1, and the αRD of the CBM is 0.21 eV Å. The ERD along the Y–C0 path is 0.5 meV, the Δk is 0.00424 Å−1, and the αRD of the CBM is 0.23 eV Å, which is smaller than that of 2D chiral ferroelectric (R-3AMP)PbBr4
22 and comparable to that of the polar quasi-2D lead iodide hybrid (IBA)2(EA)2Pb3I10 (IBA = 4-isopropylbenzylammonium, EA = ethylammonium).49 Due to the different contributions of Pb and I elements to the CBM and VBM, the αRD of the VBM is much less obvious than that of the CBM (about 0.04 eV Å). The difference in αRD values along the Γ–Y and Y–C0 paths indicates the anisotropy of the band splitting (Fig. 5b). The calculated RD effect was also verified by CPL excited photoluminescence measurements.50 The opposite fluorescence intensity difference between 3R and 3S under the excitation of CPL with different helicity further indicates that the existence of RD splitting causes them to produce spin splitting bands with opposite optical helicity (Fig. S11†).
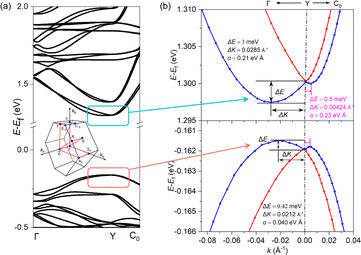 |
| Fig. 5 (a) DFT-PBE + SOC band structure of 3R along selected k paths (inset is the Brillouin zone with high symmetry k points). (b) Rashba–Dresselhaus splitting. | |
Conclusions
In conclusion, we have constructed two pairs of 3D chiral lead-iodide hybrids (R/S-3AEP)Pb2I6 and (R/S-2AEP)Pb2I6 through chiral introduction and ortho substitution strategies and obtained bulk single crystals of 3R/S. These 3D CHMHs exhibit good circular dichroism, CPL detection performance, and the RD effect. Meanwhile, the crystal device shows excellent photodetection performances in terms of the on/off ratio, responsivity, detectivity, and bulk photovoltaic effect for self-power detection. This provides new research ideas and materials for the research and development of chiroptics and spintronics.
Data availability
The data supporting this article have been included as part of the ESI.†
Author contributions
Chang-Chun Fan: conceptualization, data curation, formal analysis, investigation, methodology, validation and writing-original draft; Cheng-Dong Liu: data curation and methodology; Bei-Dou Liang: visualization and formal analysis; Tong-Yu Ju supervision; Wei Wang: investigation; Ming-Liang Jin: formal analysis; Chao-Yang Chai: supervision; Wen Zhang: conceptualization, data curation, funding acquisition, resources, supervision, software and writing-review & editing.
Conflicts of interest
There are no conflicts to declare.
Acknowledgements
This research was made possible as a result of a generous grant from the National Natural Science Foundation of China (Grant 21991144). We thank the Big Data Center of Southeast University for providing the facility support on the numerical calculations.
References
- M. Liu, L. Zhang and T. Wang, Chem. Rev., 2015, 115, 7304–7397 CrossRef CAS PubMed.
- L. Guankui, Z. Yecheng, Z. Mingtao, S. Randy, R. Abdullah, H. Li, L. Girish and G. Weibo, Adv. Mater., 2019, 31, 1807628 CrossRef PubMed.
- P. Duan, H. Cao, L. Zhang and M. Liu, Soft Matter, 2014, 10, 5428–5448 RSC.
- S. Ma, J. Ahn and J. Moon, Adv. Mater., 2021, 33, 2005760 CrossRef CAS PubMed.
- G. Long, R. Sabatini, M. I. Saidaminov, G. Lakhwani, A. Rasmita, X. Liu, E. H. Sargent and W. Gao, Nat. Rev. Mater., 2020, 5, 423–439 CrossRef.
- H.-Y. Liu, H.-Y. Zhang, X.-G. Chen and R.-G. Xiong, J. Am. Chem. Soc., 2020, 142, 15205–15218 CrossRef CAS PubMed.
- X. B. Han, C. Y. Chai, M. L. Jin, C. C. Fan and W. Zhang, Adv. Opt. Mater., 2023, 11, 2300580 CrossRef CAS.
- J. Ahn, E. Lee, J. Tan, W. Yang, B. Kim and J. Moon, Mater. Horiz., 2017, 4, 851–856 RSC.
- C. Y. Chai, X. B. Han, C. D. Liu, C. C. Fan, B. D. Liang and W. Zhang, J. Phys. Chem. Lett., 2023, 14, 4063–4070 CrossRef CAS PubMed.
- P. J. Huang, K. Taniguchi and H. Miyasaka, J. Am. Chem. Soc., 2019, 141, 14520–14523 CrossRef CAS PubMed.
- B.-D. Liang, C.-C. Fan, C.-D. Liu, T.-Y. Ju, C.-Y. Chai, X.-B. Han and W. Zhang, Inorg. Chem. Front., 2023, 10, 5035–5043 RSC.
- P.-J. Huang, K. Taniguchi, M. Shigefuji, T. Kobayashi, M. Matsubara, T. Sasagawa, H. Sato and H. Miyasaka, Adv. Mater., 2021, 33, 2008611 CrossRef CAS PubMed.
- C. Yuan, X. Li, S. Semin, Y. Feng, T. Rasing and J. Xu, Nano Lett., 2018, 18, 5411–5417 CrossRef CAS PubMed.
- D. Fu, J. Xin, Y. He, S. Wu, X. Zhang, X. M. Zhang and J. Luo, Angew. Chem., Int. Ed., 2021, 60, 20021–20026 CrossRef CAS PubMed.
- C.-C. Fan, C.-D. Liu, B.-D. Liang, W. Wang, M.-L. Jin, C.-Y. Chai, C.-Q. Jing, T.-Y. Ju, X.-B. Han and W. Zhang, Nat. Commun., 2024, 15, 1464 CrossRef CAS PubMed.
- C.-K. Yang, W.-N. Chen, Y.-T. Ding, J. Wang, Y. Rao, W.-Q. Liao, Y.-Y. Tang, P.-F. Li, Z.-X. Wang and R.-G. Xiong, Adv. Mater., 2019, 31, 1808088 CrossRef PubMed.
- J. Naciri, D. K. Shenoy, P. Keller, S. Gray, K. Crandall and R. Shashidhar, Chem. Mater., 2002, 14, 5134–5139 CrossRef CAS.
- H. Y. Zhang, Y. Y. Tang, P. P. Shi and R. G. Xiong, Acc. Chem. Res., 2019, 52, 1928–1938 CrossRef CAS PubMed.
- S. Jiao, H. Jiang, C. Fan, C. Xu, J. Jiang, Y. Xu, Z. Tang, X. Sun, P. Ji, X. Yang, K. Ye, L. Xu, Q. You, S. Chen, H.-L. Cai and X. Wu, Chem. Eng. J., 2023, 477, 146805 CrossRef CAS.
- T. Y. Ju, C. C. Fan, B. D. Liang, C. D. Liu, M. L. Jin, C. Y. Chai and W. Zhang, Adv. Funct. Mater., 2024, 34, 2316747 CrossRef.
- K. Ishizaka, M. S. Bahramy, H. Murakawa, M. Sakano, T. Shimojima, T. Sonobe, K. Koizumi, S. Shin, H. Miyahara, A. Kimura, K. Miyamoto, T. Okuda, H. Namatame, M. Taniguchi, R. Arita, N. Nagaosa, K. Kobayashi, Y. Murakami, R. Kumai, Y. Kaneko, Y. Onose and Y. Tokura, Nat. Mater., 2011, 10, 521–526 CrossRef CAS PubMed.
- C. C. Fan, X. B. Han, B. D. Liang, C. Shi, L. P. Miao, C. Y. Chai, C. D. Liu, Q. Ye and W. Zhang, Adv. Mater., 2022, 34, 2204119 CrossRef CAS PubMed.
- D. S. Sanchez, I. Belopolski, T. A. Cochran, X. Xu, J.-X. Yin, G. Chang, W. Xie, K. Manna, V. Süβ, C.-Y. Huang, N. Alidoust, D. Multer, S. S. Zhang, N. Shumiya, X. Wang, G.-Q. Wang, T.-R. Chang, C. Felser, S.-Y. Xu, S. Jia, H. Lin and M. Z. Hasan, Nature, 2019, 567, 500–505 CrossRef CAS PubMed.
- S. D. Stranks, G. E. Eperon, G. Grancini, C. Menelaou, M. J. P. Alcocer, T. Leijtens, L. M. Herz, A. Petrozza and H. J. Snaith, Science, 2013, 342, 341–344 CrossRef CAS PubMed.
- G. Xing, N. Mathews, S. Sun, S. S. Lim, Y. M. Lam, M. Grätzel, S. Mhaisalkar and T. C. Sum, Science, 2013, 342, 344–347 CrossRef CAS PubMed.
- Q. Dong, Y. Fang, Y. Shao, P. Mulligan, J. Qiu, L. Cao and J. Huang, Science, 2015, 347, 967–970 CrossRef CAS PubMed.
- W. Wang, C.-D. Liu, C.-C. Fan, X.-B. Fu, C.-Q. Jing, M.-L. Jin, Y.-M. You and W. Zhang, J. Am. Chem. Soc., 2024, 146, 9272–9284 CrossRef CAS PubMed.
- L. M. Herz, ACS Energy Lett., 2017, 2, 1539–1548 CrossRef CAS.
- J. Lim, M. T. Hörantner, N. Sakai, J. M. Ball, S. Mahesh, N. K. Noel, Y.-H. Lin, J. B. Patel, D. P. McMeekin, M. B. Johnston, B. Wenger and H. J. Snaith, Energy Environ. Sci., 2019, 12, 169–176 RSC.
- H. Zhu, Y. Fu, F. Meng, X. Wu, Z. Gong, Q. Ding, M. V. Gustafsson, M. T. Trinh, S. Jin and X. Y. Zhu, Nat. Mater., 2015, 14, 636–642 CrossRef CAS PubMed.
- D. Shi, V. Adinolfi, R. Comin, M. Yuan, E. Alarousu, A. Buin, Y. Chen, S. Hoogland, A. Rothenberger, K. Katsiev, Y. Losovyj, X. Zhang, P. A. Dowben, O. F. Mohammed, E. H. Sargent and O. M. Bakr, Science, 2015, 347, 519–522 CrossRef CAS PubMed.
-
NREL, 2023, https://www.nrel.gov/pv/cell-efficiency.html.
- G. Long, C. Jiang, R. Sabatini, Z. Yang, M. Wei, L. N. Quan, Q. Liang, A. Rasmita, M. Askerka, G. Walters, X. Gong, J. Xing, X. Wen, R. Quintero-Bermudez, H. Yuan, G. Xing, X. R. Wang, D. Song, O. Voznyy, M. Zhang, S. Hoogland, W. Gao, Q. Xiong and E. H. Sargent, Nat. Photonics, 2018, 12, 528–533 CrossRef CAS.
- X. Wang, Y. Wang, W. Gao, L. Song, C. Ran, Y. Chen and W. Huang, Adv. Mater., 2021, 33, 2003615 CrossRef CAS PubMed.
- A. Abherve, N. Mercier, A. Kumar, T. K. Das, J. Even, C. Katan and M. Kepenekian, Adv. Mater., 2023, 35, 2305784 CrossRef CAS PubMed.
- C. Chen, L. Gao, W. Gao, C. Ge, X. Du, Z. Li, Y. Yang, G. Niu and J. Tang, Nat. Commun., 2019, 10, 1927 CrossRef PubMed.
- J. X. Gao, W. Y. Zhang, Z. G. Wu, Y. X. Zheng and D. W. Fu, J. Am. Chem. Soc., 2020, 142, 4756–4761 CrossRef CAS PubMed.
- Q. Guan, T. Zhu, Z. K. Zhu, H. Ye, S. You, P. Xu, J. Wu, X. Niu, C. Zhang, X. Liu and J. Luo, Angew. Chem., Int. Ed., 2023, 62, e202307034 CrossRef CAS PubMed.
- X. Li, Y. He, M. Kepenekian, P. Guo, W. Ke, J. Even, C. Katan, C. C. Stoumpos, R. D. Schaller and M. G. Kanatzidis, J. Am. Chem. Soc., 2020, 142, 6625–6637 CrossRef CAS PubMed.
- D. Umeyama, L. Leppert, B. A. Connor, M. A. Manumpil, J. B. Neaton and H. I. Karunadasa, Angew. Chem., Int. Ed., 2020, 59, 19087–19094 CrossRef CAS PubMed.
- C.-C. Fan, B.-D. Liang, C.-D. Liu, C.-Y. Chai, X.-B. Han and W. Zhang, Inorg. Chem. Front., 2022, 9, 6404–6411 RSC.
- J. Even, L. Pedesseau, J.-M. Jancu and C. Katan, J. Phys. Chem. Lett., 2013, 4, 2999–3005 CrossRef CAS.
- H.-Y. Zu, C.-C. Fan, C.-D. Liu, C.-Q. Jing, C.-Y. Chai, B.-D. Liang, X.-B. Han and W. Zhang, Chem. Mater., 2023, 35, 5854–5863 CrossRef CAS.
- C.-C. Fan, C.-D. Liu, B.-D. Liang, M.-L. Jin, T.-Y. Ju, C.-Y. Chai, X.-B. Han and W. Zhang, Inorg. Chem., 2023, 62, 12634–12638 CrossRef CAS PubMed.
- I. Spanopoulos, I. Hadar, W. Ke, Q. Tu, M. Chen, H. Tsai, Y. He, G. Shekhawat, V. P. Dravid, M. R. Wasielewski, A. D. Mohite, C. C. Stoumpos and M. G. Kanatzidis, J. Am. Chem. Soc., 2019, 141, 5518–5534 CrossRef CAS PubMed.
- Y. Liu, Z. Yang, D. Cui, X. Ren, J. Sun, X. Liu, J. Zhang, Q. Wei, H. Fan, F. Yu, X. Zhang, C. Zhao and S. F. Liu, Adv. Mater., 2015, 27, 5176–5183 CrossRef CAS PubMed.
- Y. Peng, X. Liu, L. Li, Y. Yao, H. Ye, X. Shang, X. Chen and J. Luo, J. Am. Chem. Soc., 2021, 143, 14077–14082 CrossRef CAS PubMed.
- M. K. Jana, R. Song, H. Liu, D. R. Khanal, S. M. Janke, R. Zhao, C. Liu, Z. V. Vardeny, V. Blum and D. B. Mitzi, Nat. Commun., 2020, 11, 4699 CrossRef CAS PubMed.
- Y. Chen, L. Tang, X. Zeng, W. Guo, T. Yang, H. Xu, Y. Liu, G. Gou, Y. Zhao, J. Luo and Z. Sun, Adv. Funct. Mater., 2024, 34, 2311726 CrossRef CAS.
- E. Lafalce, E. Amerling, Z. G. Yu, P. C. Sercel, L. Whittaker-Brooks and Z. V. Vardeny, Nat. Commun., 2022, 13, 483 CrossRef CAS PubMed.
Footnote |
† Electronic supplementary information (ESI) available: Experimental details, Fig. S1–S15 and Tables S1–S5. CCDC 2323588–2323597. For ESI and crystallographic data in CIF or other electronic format see DOI: https://doi.org/10.1039/d4sc00954a |
|
This journal is © The Royal Society of Chemistry 2024 |
Click here to see how this site uses Cookies. View our privacy policy here.