DOI:
10.1039/D4SC00114A
(Perspective)
Chem. Sci., 2024,
15, 6218-6228
Photoswitching the fluorescence of nanoparticles for advanced optical applications
Received
7th January 2024
, Accepted 25th March 2024
First published on 25th March 2024
Abstract
The dynamic optical response properties and the distinct features of nanomaterials make photoswitchable fluorescent nanoparticles (PF NPs) attractive candidates for advanced optical applications. Over the past few decades, the design of PF NPs by coupling photochromic and fluorescent motifs at the nanoscale has been actively pursued, and substantial efforts have been made to exploit their potential applications. In this perspective, we critically summarize various design principles for fabricating these PF NPs. Then, we discuss their distinct optical properties from different aspects by highlighting the capability of NPs in fabricating new, robust photoswitch systems. Afterwards, we introduce the pivotal role of PF NPs in advanced optical applications, including sensing, anti-counterfeiting and imaging. Finally, current challenges and future development of PF NPs are briefly discussed.
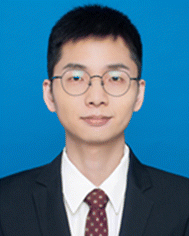 Wencheng Zhong | Wencheng Zhong received his bachelor degree from Northwestern Polytechnical University in 2017. Currently, he is pursuing a doctoral degree under the supervision of Professor Li Shang at the School of Materials Science and Engineering, Northwestern Polytechnical University. His research focuses on the development of gold nanocluster-based fluorescent probes for bio-imaging. |
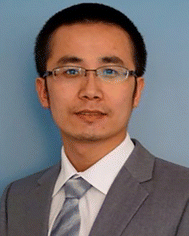 Li Shang | Li Shang received his PhD in Chemistry from the Changchun Institute of Applied Chemistry, Chinese Academy of Sciences in 2010. Then, he joined the group of Prof. G. Ulrich Nienhaus at the Karlsruhe Institute of Technology (KIT), Germany, as a Humboldt fellow and then as a Research Associate. In 2016, he became a Professor at the School of Materials Science and Engineering, Northwestern Polytechnical University, China. His research interests focus on the development of luminescent nanomaterials for biological applications. Up to now, he has published more than 100 peer-reviewed papers, with an H-index of 50. |
1. Introduction
Fluorescent nanoparticles (NPs) have garnered significant interest due to their immense potential in various applications, including optical devices, fluorescent sensing and biological imaging.1,2 Fluorescent NPs, constructed from individual organic dyes, exhibit enhanced brightness and stability compared to monomers.3 Furthermore, when inorganic NPs are reduced to specific small sizes, the quantum size effect induces discrete energy levels, which results in distinct fluorescence properties. Traditionally, it is believed that fluorescence is emitted continuously from NPs under light excitation, which is manifested as a bright state (‘1’). However, there are some interesting phenomena that do not conform to this situation. For example, the random blinking state of fluorescence was found in quantum dots (QDs).4,5 This random blinking behavior indicates that the fluorescence can switch randomly between the bright state (‘1’) and the dark state (‘0’). Obviously, the blinking feature of fluorescence from QDs provides additional information, which also enables their innovative application in super-resolution imaging.6 A phenomenon similar to the fluorescence switch was also found in fluorescent proteins, such as Dronpa.7–9 Upon irradiation with specific wavelengths of light, fluorescence of these proteins can be reversibly switched on or off. It is noteworthy that controllable manipulation of fluorescence endows these materials with an intelligent response feature, which provides the feasibility of real-time manipulation of their optical signals via a non-invasive approach. Apparently, photoswitchable modulation of luminescence in NPs will benefit their potential usage in advanced optical applications, which has attracted intensive attention in the past few years.
To date, fluorescent photoswitches can be categorized into two major classes: small-molecule photoswitches and photoswitchable fluorescent NPs (PF NPs).10–12 While small-molecule photoswitches remain rare due to the difficulty of design, PF NPs showed the advantages of easy fabrication, good designability and enhanced photophysical properties. To fabricate these PF NPs, one of the most effective ways is to introduce photochromic components,13,14 which typically feature different isomers with varied physicochemical properties (e.g., colour, emission, charges and hydrophilicity). Commonly used photochromic components can be categorized into two types, organic and inorganic ones.15–18 The broad spectral variation of inorganic photochromic materials stands out as a significant advantage in the construction of PF NPs with a wide range of tunable fluorescence.19–21 However, this advantage becomes a disadvantage when it comes to precisely tuning the fluorescence of PF NPs. In contrast, the absorption spectra of organic photochromic materials are typically much narrower than that of inorganic ones. Therefore, for the precise regulation of fluorescence, organic photochromic materials show prior advantages compared with inorganic materials. The photoisomerisation of organic photochromic materials mainly includes E–Z isomerisation (e.g., azobenzene and hydrazone) and cyclisation/retro-cyclisation (e.g., diarylethene and spiropyran).12 Among various organic photochromic components, diarylethene,22 spiropyran,23 azobenzene24 and their derivatives are widely used in the preparation of PF NPs. Upon the marriage of fluorescent components with photochromic components, fluorescence of the as-prepared PF NPs can be artificially controlled by alternating light irradiation. Importantly, the primary challenge in preparing PF NPs for advanced optical applications lies in the imperative to ensure both photoswitching properties and fluorescence stability simultaneously.
The emerging research interests in PF NPs focus on engineering their optical properties, such as multi-fluorescence response, photoswitching in an aqueous environment and the nanoconfinement behaviours.25 The poor water-solubility of most photochromic molecules, as well as their abnormal behavior in aqueous environments, hinder their efficacy in modulating the fluorescence of PF NPs. For biological applications, the development of water-soluble PF NPs also stands as a crucial imperative. Besides, single-color PF NPs are restricted by the dimension of information they can provide, proving insufficient to meet the increasing application demands.26–28 Hence, one of the feasible solutions is to augment the quantity of switchable fluorescence signals (i.e., multi-color) for PF NPs. Except for a few PF NPs composed of intrinsically fluorescent photochromic components, the photoswitching mechanism of PF NPs mainly lies in the regulation effect of the photochromic component on the optical properties of fluorophores via the inner filter effect, energy transfer and electron transfer.29,30 Therefore, it is necessary to rationally engineer the distance between the fluorescence component and photochromic component as well as the flexibility of photochromic components in the design of PF NPs. In particular, the microenvironment of photochromic components in NPs, either on the surface or embedded inside NPs, is much different from that of free molecules in the medium. Hence, the nanoconfinement effect needs to be seriously considered in the design and fabrication of PF NPs.31,32 Apparently, a clear understanding of these optical properties of PF NPs will be beneficial to further design and fabricate robust PF NPs with enhanced photo-response properties.
Due to their distinct fluorescence switching properties, PF NPs have found widespread utility in optical sensing, anti-counterfeiting, and high-resolution imaging applications. The ability of PF NPs to provide multidimensional information makes them promising candidates for advanced applications in these fields. The switching ratio, response time, and fatigue resistance are the most critical properties of PF NPs for their advanced optical applications. Therefore, for potential practical applications, researchers have made intensive efforts for augmenting the properties of PF NPs, such as the switching ratio, response time, and fatigue resistance. Given the extensive application of organic photochromic components, this perspective will mainly focus on PF NPs based on organic photochromic components. We will summarize recent advances of these PF NPs in fabrication strategies, optical properties and emerging applications. Furthermore, the future development in the field of PF NPs is discussed at the end.
2. Fabrication strategies
To obtain efficient PF NPs, it is crucial to establish a diverse set of preparation strategies. To date, researchers have developed a series of synthesis methods in the pursuit of fabricating PF NPs. Based on the interaction mode between the photochromic components and fluorescent components, the current fabrication strategies of PF NPs can be categorized into self-assembly, covalent modification, and host–guest encapsulation. In the following section, we will briefly discuss these three strategies.
2.1 Self-assembly
Self-assembly methods are commonly utilized in the synthesis of tailored NPs loaded with functional molecules. The advantages of self-assembly methods mainly include a controllable size, a simple fabrication process and adaptability. Therefore, these methods provide an efficient approach for the spontaneous and rapid organization of PF NPs. Through alterations in the physicochemical properties of the solution, the self-assembly of encapsulated contents and skeleton materials (e.g., polymers, surfactants, and lipids) gives rise to the formation of PF NPs. Notably, certain isomeric photochromic components, such as the merocyanine isomers of spiropyran,10,33,34 exhibit exceptional fluorescence properties. Therefore, the utilization of fluorescent photochromic compounds as a basis for constructing PF NPs represents a convenient pathway for creating single-emission PF NPs by self-assembly.
As shown in Scheme 1A, fluorescent photochromic component-based PF NPs can be constructed by direct or pre-modification self-assembly strategies. Direct self-assembly refers to the passive encapsulation of fluorescent photochromic components. Pre-modification self-assembly means the formation of NPs by using photochromic component-modified skeletal materials, which might avoid possible leakage problems in the formation of NPs. Expanding on the above concept, PF NPs can be formed through the co-self-assembly of multiple components. In this situation, the fluorescence switching of PF NPs is accomplished through the interaction between the fluorescent component and the photochromic component (either fluorescent or non-fluorescent), such as fluorescence resonance energy transfer (FRET, Scheme 1B). In various self-assembly methods, the nanoprecipitation method has been widely employed in the preparation of self-assembled PF NPs.35–38 For example, photochromic diarylethene was passively encapsulated in poly[2-methoxy-5-(2-ethylhexyloxy)-1,4-(1-cyanovinylene-1,4-phenylene)] (CN-PPV)-based fluorescent polymer dots by nanoprecipitation, which yielded polymer dot-based PF NPs with modifiable surfaces (Fig. 1A). Moreover, PF NPs can be prepared by pre-covalently linking photochromic components with a polymer, followed by nanoprecipitation.39,40 As an example, photochromic diarylethene-conjugated polymers were further transformed into PF NPs through nanoprecipitation (Fig. 1B). Due to the rapid synthetic process, nanoprecipitation has been demonstrated as a convenient strategy for the preparation of PF NPs. Nevertheless, in the nanoprecipitation method, controlling the encapsulation rate and modification extent of photochromic components remains challenging. This may result in a suboptimal fluorescence switching effect, which limits the universality and efficacy of nanoprecipitation for the preparation of PF NPs.
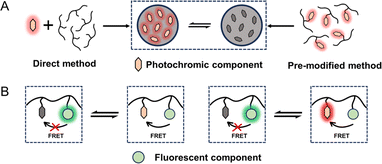 |
| Scheme 1 (A) Illustration of the self-assembly strategy of preparing PF NPs based on fluorescent photochromic components. (B) Schematic diagram of the interaction, such as FRET, between individual fluorescent and photochromic components in self-assembled PF NPs. | |
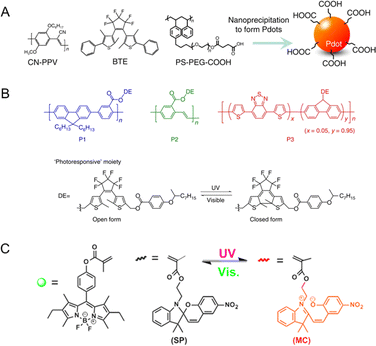 |
| Fig. 1 (A) Preparation of photoswitchable polymer dots by passive encapsulation of photochromic BTE in CN-PPV/PS-PEG-COOH-based polymer NPs.35 Adapted with permission from ref. 32. Copyright 2016 Springer Nature. (B) Photochromic diarylethene-conjugated polymers were synthesized into PF NPs by nanoprecipitation.40 Adapted with permission from ref. 37. Copyright 2014 Springer Nature. (C) Miniemulsion polymerization for the preparation of spiropyran-based PF NPs.41 Adapted with permission from ref. 41. Copyright 2015 American Chemical Society. | |
To address the above challenges, a polymerization reaction-based method was proposed to construct self-assembled PF NPs. In this approach, the integration of multiple independent components is achieved through the polymerization reaction. In the early stages, Li and colleagues developed a series of self-assembly PF NPs based on this approach, which significantly expanded their application in various fields.42–44 Furthermore, the Chen group expanded the development of intricately designed self-assembly PF NPs based on miniemulsion copolymerization of photochromic component-linked and fluorescent component-linked methacrylate (Fig. 1C).41,45,46 In the polymerization-based self-assembly method, high photoswitching ratios could be easily achieved due to sufficient amounts of photochromic molecules in PF NPs. Meanwhile, the photochromic components are well protected by polymer NPs, which show excellent anti-fatigue properties for photoswitching.
In the synthesis of PF NPs, inorganic fluorescent NPs are also regarded as crucial candidates for fluorescence components. As reported in the literature, most luminescent NPs possess surface ligand layers that envelop their surfaces, commonly referred to as ligand-protected fluorescent NPs.49,50 The retained catalytic activity in ligand-protected NPs suggests that the ligand coverage on the surface of luminescent NPs may not be complete, which leads to the formation of insertable regions on the NP surface. These insertable regions will facilitate the self-assembly between photochromic components and NPs. Indeed, self-assembly of photochromic components on the surface of inorganic fluorescent NPs has been proved as an effective method for preparing PF NPs. As depicted in Fig. 2A, the direct surface assembly method involves the anchoring of the photochromic component onto the NP surface.47,51 As an example, Akaishi et al. coupled fluorescent perovskite nanocrystals with photochromic diarylethene for achieving a photoswitching of fluorescence from perovskite nanocrystals (Fig. 2B).47 In this work, it has been demonstrated that alterations in the moieties of diarylethene affect the interactions with nanocrystals, thus influencing the photoswitching ratio of PF NPs. In addition to the direct surface-assembly method, a coating layer on the surface of NPs can also facilitate the self-assembly of photochromic components (Fig. 2C). For example, Díaz et al. first synthesized amphiphilic comb-like polymers modified with diarylethene molecules with alkyl chains of different lengths.48,52 As displayed in Fig. 2D, the diarylethene-modified polymer layer was coated on the surface of fluorescent QDs to realize the self-assembly between two components. By controlling the chain length of diarylethene, the distance between photochromic components and QDs can be managed, which ultimately alters the fluorescence photoswitching ratio of QDs. In summary, the self-assembly strategy for the preparation of PF NPs offers the advantages of efficient and controllable preparation. Additionally, the interior of the self-assembly structure provides a flexible isomeric space for the photochromic components.
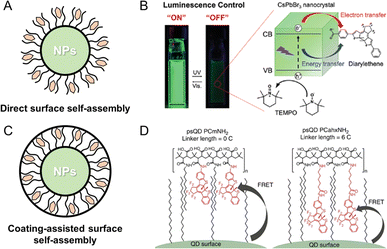 |
| Fig. 2 (A) Schematic illustration of the direct surface self-assembly method. (B) Photoswitchable fluorescent perovskite nanocrystals coupled with photochromic diarylethene.47 Adapted with permission from ref. 47. Copyright 2022 Wiley. (C) Schematic illustration of the coating-assisted surface self-assembly method. (D) Photoswitchable fluorescent QDs by surface polymer-assisted modification of diarylethene.48 Adapted with permission from ref. 48. Copyright 2015 Springer Nature. | |
2.2 Covalent modification
Rationally engineering the distance between the fluorophore and the photochromic component is important to regulate the interactions as well as optical properties of PF NPs. Covalent modification of the photochromic components on the surface of NPs represents an efficient method owing to their high efficiency and good stability. In the covalent modification methods, both components were connected by a rigid covalent linkage. Due to the fluorescence stability and the tunability of surface ligands, inorganic NPs are frequently chosen as the donors in PF NPs.53 For instance, PbS QDs with near-infrared fluorescence were covalently coupled with diarylethene to synthesize PF NPs, revealing a novel fluorescence photoswitching mechanism of triplet energy transfer (Fig. 3A).54 Bonacchi et al. reported the design of gold NPs with near-infrared emission by the covalent linking of azobenzene, which exhibited photoswitchable fluorescence by excitation energy transfer from azobenzene to gold NPs.55 Typically, photochromic molecules can be covalently anchored to the surface of fluorescent NPs via the well-established conjugation strategy.54,56,57 For example, Cong et al. synthesized thiolated spiropyran as the surface ligands to fabricate photoswitchable gold nanoclusters (AuNCs) with the assistance of another co-ligand, 11-mercaptoundecanoic acid.58 Then, photoswitchable AuNC-based NPs were employed for intracellular photoswitchable fluorescence imaging. The results demonstrated that spiropyran molecules, covalently attached to AuNCs, preserved their photochromic properties in aqueous environments. However, this work also highlights that covalently linked photochromic components on the surface of NPs may exhibit poor anti-fatigue properties. This issue may be attributed to the direct exposure of the photochromic components to the external environment, leading to an increased photodegradation rate of photochromic components.59
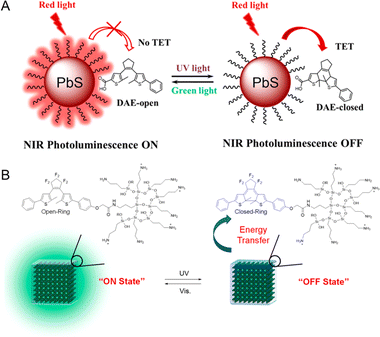 |
| Fig. 3 (A) Photoswitchable photoluminescence of diarylethene-bound PbS QDs.54 Adapted with permission from ref. 50. Copyright 2022 American Chemical Society. (B) Photoswitchable perovskite lead halide QDs coated by triethoxysilane-modified diarylethene.62 Adapted with permission from ref. 57. Copyright 2020 American Chemical Society. | |
Alternatively, one can coat the surface of NPs with a shell loaded with photochromic molecules.60,61 For instance, perovskite lead halide QDs were coated with a silicon shell of triethoxysilane-modified diarylethene.62 In this system, the shell coating method ensures sufficient modification of diarylethene, which results in a photoswitching ratio up to 90% for fluorescent QDs. This coating method not only achieved the efficient photoswitching of green fluorescence of QDs, but also stabilized the QDs (Fig. 3B). However, it should be noted that the shell coating methods necessitate pre-modification of photochromic components, which can be cumbersome and time-consuming.
2.3 Host–guest encapsulation
In nature, the structure of photoswitchable fluorescent proteins consists of fluorescent proteins and photoisomeric molecules that are interconnected in a host–guest configuration.63,64 The inherent host–guest structure in photoswitchable fluorescent proteins enhances the stability of photochromic components, thereby facilitating the preservation of their photoswitching properties. Inspired by the remarkable properties of photoswitchable fluorescent proteins, researchers have embarked on developing PF NPs via host–guest interactions.65–68 For example, by mimicking photoswitching fluorescent proteins, the Tian group designed a kind of carbohydrate modified naphthalimide-spiropyran dyad (Gal-NSp), which was further encapsulated in human serum albumin (HSA).69,70 The host–guest interaction between Gal-NSp and HSA is achieved by the insertion of Gal-NSp into the hydrophobic pocket of HSA. The incorporation of Gal-NSp with HSA activates the fluorescence from the merocyanine state of spiropyran, resulting in a dual-fluorescence photoswitch (Fig. 4A).
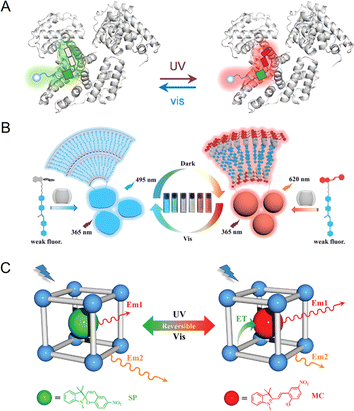 |
| Fig. 4 (A) Dual-fluorescence photoswitchable artificial Gal-NSp–HSA hybrid.69 Adapted with permission from ref. 64. Copyright 2018 American Chemical Society. (B) CB[8]/spiropyran-modified cyanostilbene derivative-based multicolor luminescent supramolecular shuttle.75 Adapted with permission from ref. 70. Copyright 2023 Wiley. (C) Dual-emission PF NPs by the insertion of spiropyran in the pores of a MOF.78 Adapted with permission from ref. 73. Copyright 2023 Wiley. | |
Additionally, synthetic supramolecules frequently serve as versatile hosts for incorporating photochromic components, thus enhancing the photoswitching properties of photochromic components.71–74 For example, Zhang et al. designed a spiropyran-modified cyanostilbene(BCNMC)/cucurbit[8]uril (CB[8]) complex, which exhibited multi-fluorescence photoswitching behaviors.75 As shown in Fig. 4B, BCNMC and CB[8] form a stable host–guest structure, leading to reversible changes in topological morphology and multi-color fluorescence through light irradiation. Besides, the incorporation of supramolecular and fluorescent photochromic components has been observed to enhance the fatigue resistance of photoswitching fluorescence.76,77 Kim et al. discovered that the number of photoswitching cycles of the cucurbit[7]uril/diarylethene complex (2560 times) is higher than that of the free diarylethene (80 times) in an aqueous solution. The enhancement of the photofatigue resistance may be due to the protective effect of the supramolecular structure of cucurbit[7]uril on diarylethene molecules, which further underscores the great potential of the host–guest strategy in designing robust PF NPs.76
Additionally, the method of embedding within the porous structure is commonly employed for porous NPs.78,79 For example, as shown in Fig. 4C, Zheng et al. designed a metal–organic framework (MOF) loaded with spiropyran (ZJU-128⊃SP).78 Due to the FRET between spiropyran and ZJU-128, the fluorescence of ZJU-128⊃SP changes from blue fluorescence (from ZJU-128) to red fluorescence (from the merocyanine state of spiropyran) under the irradiation of UV light. In this method, the pores of NPs ensure the necessary spatial flexibility for the isomerization of photochromic molecules, which maintains the photoswitching ability of PF NPs.
3. Optical properties
3.1 From a non-aqueous environment to an aqueous environment
For many applications, PF NPs need to exhibit their photo-responsive properties in an aqueous environment, e.g., biological systems.12,80,81 However, most of the reported organic photochromic molecules possess limited water-solubility, which poses the challenge of regulating their photoswitchable properties from a non-aqueous environment to an aqueous environment. For example, hydrophobic photochromic components tend to aggregate or decompose in aqueous environments, resulting in their diminished photochromic properties.82–84 One common solution is the modification of hydrophilic groups to photochromic dyes that can greatly enhance their water-solubility.85,86 For instance, spiropyran with sulfonate group modification exhibits greater water solubility compared to native spiropyran.87 Also, the modification of hydrophobic components into hydrophilic nanostructures can endow the resultant PF NPs with much improved water-dispersity, which has been adopted in many previous research studies.23 As an illustration, Torii et al. covalently modified furylfulgimide-based photoswitchable fluorescent molecules onto proteins, resulting in a significant improvement in the water solubility.88 Remarkably, the system exhibited good anti-fatigue properties of photoswitching in aqueous solution.
Meanwhile, it is noteworthy that photochromic components might exhibit an unfavorable photo-isomerisation process in NPs owing to the change in local hydrophobicity,89,90 which then compromises their photo-isomerism efficiency as well as the fluorescence switching performance. In addition, the photochromic properties of PF NPs are also influenced by the microenvironment within the fabricated hydrophilic NPs. For instance, the aqueous microenvironment leads to the protonation of the MC state of spiropyran into MCH+.91 The different photochromic properties of MC and MCH+ could potentially cause a lack of spectral overlap in the FRET pair within PF NPs.92 Hence, preserving the characteristics of non-aqueous photochromic components is crucial when designing PF NPs. Undoubtedly, the development of hydrophilic PF NPs will be necessary and beneficial for their potential applications in biological environments.
3.2 From a single emission color to multiple emission colors
PF NPs with single emission can only provide dark (fluorescence-off state) and bright (fluorescence-on state) signals at one specific wavelength. The limitation of a single signal not only restricts information storage capacity but also makes it susceptible to decryption, significantly hindering the realm of its application. In contrast, multi-emission fluorescence photoswitching would be an ideal and compelling choice. A straightforward approach is to synthesize several single-emission PF NPs, which are then mixed together for further application.40,45 As depicted in Fig. 5A, the Akagi group successfully developed three types of polymer-based single-emission PF NPs, which were mixed together for the reversible photoswitching of white fluorescence. By controlling the quantity of PF NP components in the mixed solution, three distinct fluorescence colors could be reversibly switched.
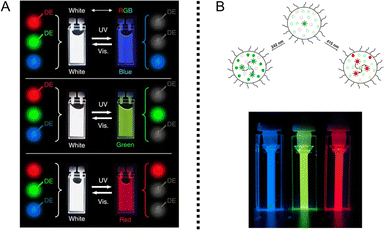 |
| Fig. 5 (A) The photoswitching fluorescence of a mixed solution based on the combination of three distinct types of PF NPs.40 Adapted with permission from ref. 37. Copyright 2014 Springer Nature. (B) Scheme of multiple-emission in single PF NPs.97 Adapted with permission from ref. 92. Copyright 2019 Springer Nature. | |
However, the above “mixing strategy” suffers from complicated synthesis procedures and limited switching efficiency, which is not favorable for practical application. Correspondingly, individual NPs possessing multi-emission photoswitching capabilities will be appealing.27 The development of such multi-emission PF NPs is expected to store more information at the individual particle level than single-emission PF NPs.93–96 As displayed in Fig. 5B, Naren et al. reported the design of tri-emission PF NPs, which exhibit tuneable emission in the RGB system.97 Besides, the property of multi-emission photoswitching prevents potential information loss due to dark states in the photoswitching process.34 Therefore, the development of integrated multi-emission PF NPs is particularly attractive for advanced applications in the future.
3.3 From a free molecule to a nano-confined surface
The photochromic behavior observed in NPs can be viewed as a manifestation within a nano-confined microenvironment.98–100 Thus, understanding the photochromic behavior of PF NPs within the nano-confined microenvironment is a crucial prerequisite for understanding their photoswitching behaviors. The properties of photochromic components, such as the quantum yield and the photoswitching rate, can be influenced by the nano-confined microenvironment. Indeed, the isomerization of photochromic dyes is impeded by too narrow nano-confined microenvironments, and it has been demonstrated that a certain spatial flexibility is necessary for photochromic components.101
This nanoconfinement effect can be harnessed to regulate the photochromic performance of PF NPs. For instance, the Shustova group reported the coordinated integration of photochromic moieties within MOFs.102–104 By adjusting the structure of MOFs, the photoisomerism and photoisomerization rates can be finely tuned, which results in comprehensive photoisomerism control. As shown in Fig. 6A, spiropyran in solvent-free UiO-67 exhibits a faster photoisomerization rate with ∼1000-fold enhancement than that in the solution.102 Moreover, photochromic dyes in coordination cages have also been thoroughly studied by the Klajn group.105–107 When being encapsulated in Td and D2h-symmetric coordination cages, the photoisomerism of the encapsulated spiropyran can be terminated and maintained, respectively (Fig. 6B).108 Meanwhile, the substitution group of encapsulated spiropyran also plays an important role in their photochromic behaviors in coordination cages. Thus, the physicochemical properties of photochromic components can be finely controlled within the nano-confined microenvironment, which paves the way for the development of highly efficient PF NPs.
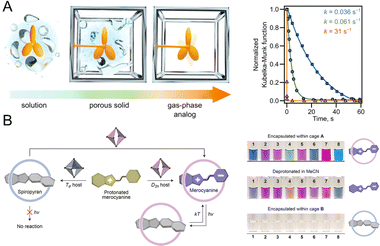 |
| Fig. 6 (A) Scheme of spiropyran in a MOF in different situations and photoisomerization kinetics studies of spiropyran in solution (blue line), UiO-67 (green line) containing DMF, and UiO-67 (solvent-free, orange line).102 Adapted with permission from ref. 97. Copyright 2023 Springer Nature. (B) Controllable photochromism of spiropyran in different coordination cages with different symmetries.108 Adapted with permission from ref. 103. Copyright 2022 American Chemical Society. | |
4. Emerging applications
4.1 Multiplexed optical sensing
Recently, PF NPs are gaining increasing attention in the field of optical sensing.109 First, the initiation and termination of sensing by PF NPs can be artificially and remotely controlled, thereby enhancing the accuracy of sensing.110 In addition, PF NPs can be utilized to acquire additional information during the sensing process, potentially improving both sensitivity and selectivity. For example, early in 2011, Tian et al. proposed a PF NP-based sensing method for target analytes in complex environments.111 In their work, the frequency information of the FRET efficiency was introduced by dual-fluorescence photoswitching. Thus, frequency-modulated signals of the target analyte can be easily identified from noise in the environments. Therefore, multi-emission PF NPs hold great potential as competitors in multiplexed optical sensing applications. Moreover, novel photoswitch-mediated FRET methods have been found to significantly reduce the detection limit of the optical sensors.112 As shown in Fig. 7A, photoswitchable nucleic acid detection provided a limit of detection down to 0.5 nM, which is at least 6 times lower than that of traditional FRET-based assays. In addition to fluorescence intensity information, the photoswitching dynamics of PF NPs can also be utilized to increase the encoding capacity for multiplexed detection.113 For instance, photoswitch-based fluorescence encoding microspheres were developed by the Xie group for detecting DNA sequences of COVID-19.114 In this system, three dimensional information, including fluorescence intensity (before and after UV irradiation) and photoswitching dynamics, was recorded for a multiplexed DNA mixture, which improves the selectivity of the target analyte (Fig. 7B). Such analyte-specific responsive PF NPs are believed to be attractive for simultaneously and efficiently detecting multiple analytes via multiplexed optical sensing.
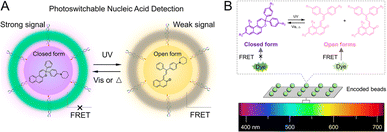 |
| Fig. 7 (A) Photoswitch-mediated FRET-based detection of nucleic acids.112 Adapted with permission from ref. 107. Copyright 2021 American Chemical Society. (B) Photoswitch-based fluorescence encoding particles for multiplexed detection.114 Adapted with permission from ref. 109. Copyright 2022 American Chemical Society. | |
4.2 Advanced optical anti-counterfeiting
Compared to NPs with static fluorescence, PF NPs with dynamic fluorescent properties have the advantage of displaying multi-dimensional encoded information. This advantage enhances the suitability of PF NPs for advanced anti-counterfeiting scenarios. At first, the encryption application of PF NPs primarily focused on basic anti-counterfeiting measures by utilizing single-emission PF NPs. As shown in Fig. 8A, a spiropyran-loaded MOF was prepared into a paper-based anti-counterfeiting device.115 Fluorescent patterns emerge or vanish under light excitation, illustrating a simple anti-counterfeiting mode. However, single-emission photoswitching anti-counterfeiting lacks the capability to address the intricate security and encryption requirements. Hence, employing multi-emission photoswitching imparts advanced anti-counterfeiting potential to PF NPs. Indeed, the dual-emission photoswitching properties enable dynamic anti-counterfeiting (Fig. 8B) and complex programming encryption (Fig. 8C). As seen in Fig. 8C, dual-emission PF NPs can provide up to twelve different signal instructions.116 Clearly, utilizing multi-emission PF NPs for anti-counterfeiting proves effective in elevating the security level. Furthermore, with the aid of artificial masks, diverse encryption patterns can be achieved using multi-emission PF NPs. Moreover, the dynamic multi-fluorescence changes during the photoswitching process can serve as the basis of time-dependent encryption (Fig. 8D), where the evolution of fluorescent color over time also yields additional encryption information.75 Conclusively, harnessing the coding capability and advancing the development of novel PF NPs (such as multi-fluorescence photoswitches) are pivotal for expanding the anti-counterfeiting applications of PF NPs.
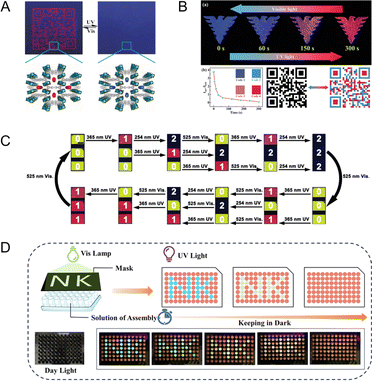 |
| Fig. 8 (A) Single-emission photoswitchable fluorescent pattern in a paper-based anti-counterfeiting device.115 Adapted with permission from ref. 110. Copyright 2019 Wiley. (B) Dual-emission photoswitchable fluorescent pattern in a hydrogel-based security label.78 Adapted with permission from ref. 73. Copyright 2023 Wiley. (C) Various signal instructions by dual-emission PF NPs.116 Adapted with permission from ref. 111. Copyright 2021 Wiley. (D) Time-dependent fluorescence evolution for advanced encryption by dual-emission PF NPs.75 Adapted with permission from ref. 70. Copyright 2023 Wiley. | |
4.3 High-resolution fluorescence imaging
In the fluorescence imaging application, the principal objectives entail precise tracking and the attainment of high resolution. In this regard, PF NPs possess significant advantages as robust probes for advanced fluorescence imaging. In particular, PF NPs possess dynamic fluorescence characteristics that can easily distinguish them from static fluorescence signals. As shown in Fig. 9A, the fluorescence of PF NPs can be cyclically photoswitched for generating a dynamic bright/dark signal.117 The dynamic fluorescence properties of PF NPs allow precise artificial signal control, which enables them to serve as specific tracking probes during the imaging process. However, the absence of a fluorescence signal in single-emission PF NPs during the dark state can compromise the integrity of the tracking process. Therefore, multi-emission PF NPs have been developed to solve this issue. For example, our groups recently utilized dual-emission PF NPs based on ligand-protected AuNCs for enhanced cell imaging.92 Dual-emission photoswitching ensures the presence of at least one bright signal, which provides a more reliable tracking process with enhanced imaging resolution compared to single-emission probes. As shown in Fig. 9B, the utilization of dual-fluorescence photoswitching showed the ability of distinguishing between dynamic and static fluorescence signals at the subcellular level.
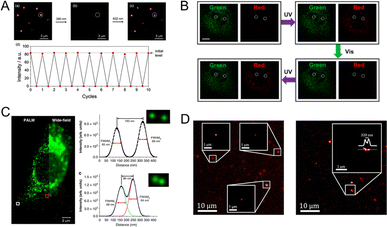 |
| Fig. 9 (A) Near-infrared fluorescence photoswitching of single-emission PF NPs.117 Adapted with permission from ref. 112. Copyright 2021 Royal Society of Chemistry. (B) Cellular dynamic and static fluorescence differentiation by dual-emission PF NPs.92 Adapted with permission from ref. 87. Copyright 2023 Royal Society of Chemistry. (C) Cellular super-resolution imaging by PF NPs.37 Adapted with permission from ref. 34. Copyright 2019 Springer Nature. (D) Super-resolution imaging of spiropyran-modified CNTs.129 Adapted with permission from ref. 123. Copyright 2019 American Association for the Advancement of Science. | |
Besides in vitro imaging, PF NPs have also been recently used for in vivo imaging.118–122 In an interesting study, the Kim group fabricated photoswitchable fluorescent diarylethene-based dendritic nanoclusters based on FRET between diarylethene and Cy5.5 dyes, which was used in bio-imaging on living zebrafish.120 These NPs maintain excellent fluorescence switching properties in the living zebrafish system. This work demonstrated the potential application of PF NPs for precise and effective real-time tracking in vivo, contributing to the development of high-resolution imaging techniques within living organisms.
Single-molecule localization microscopy (SMLM) is a type of super-resolution imaging method that requires fluorescent probes with the ability of reversible bright-to-dark conversion under light stimulation.123,124 Therefore, the photoswitchable properties of PF NPs make them attractive fluorophores for the SMLM technique.125–128 Recently, the Park group reported the synthesis of polymer NPs with dual-fluorescence photoswitching properties, based on which super-resolution imaging with about 70 nm resolution was achieved using SMLM (Fig. 9C).37 Interestingly, the distinct feature of these PF NPs also enables one to investigate their structure and properties by the super-resolution imaging techniques. For example, the morphology and internal distribution of photochromic dye-coupled carbon nanotubes (CNTs) can be visualized by means of SMLM (Fig. 9D).129 In another study, Siemes et al. successfully employed stochastic optical reconstruction microscopy to reveal the spatial distribution of cross-linkers in polymer microgels through photoswitching fluorescence of diarylethene.130
5. Conclusions
Owing to the photocontrollable and dynamic fluorescence properties, PF NPs have garnered increasing attention in recent years. In this perspective, we present the preparation method of PF NPs, their optical properties and advanced optical applications in various fields. In particular, the reasonable integration of photochromic components and fluorescent components at the nanoscale is important for constructing robust PF NPs. While several approaches have proved to be efficient for fabricating PF NPs with good performance, two key issues need to be emphasized in the design of robust PF NPs. One involves effectively reducing the distance between the fluorescent component and the photochromic component to enhance the photoswitching ratio. The other involves preserving the properties of the photochromic component, especially in biological environments. Consequently, many studies on the photoswitching behaviors of photochromic components in a nanoconfined microenvironment are conducted to understand their photoswitching behaviors. The relevant knowledge will be beneficial for the enhancement of photoswitching properties of PF NPs, such as the photoresponse rate, photoswitching ratio and anti-fatigue properties. In order to meet the demands of versatile advanced applications, the development of FP NPs with multi-emission properties at the single particle level represents an emerging and attractive direction. In the future, it is of utmost importance to achieve individual photoswitching of each fluorescent component at the single particle level. Meanwhile, we also note that the lack of standardized criteria for evaluating the performance of PF NPs poses a hindrance to their potential application and possible translation, which needs to be addressed by the community. Finally, we anticipate that this perspective will contribute to a deeper understanding of these emerging PF NPs regarding their design and properties, ultimately advancing their practical applications in diverse fields.
Author contributions
All authors contributed to the writing of the manuscript.
Conflicts of interest
There are no conflicts to declare.
Acknowledgements
This work was supported by the National Natural Science Foundation of China (22274131), the Shenzhen Science and Technology Program (Grant no. JCYJ20220530161800001), the Natural Science Foundation of Chongqing (cstc2021jcyj-msxmX0980), and the Innovation Foundation for Doctor Dissertation of Northwestern Polytechnical University (CX2021053).
Notes and references
- W. Li, G. S. Kaminski Schierle, B. Lei, Y. Liu and C. F. Kaminski, Chem. Rev., 2022, 122, 12495 CrossRef CAS PubMed.
- G. R. Tan, M. H. Wang, C. Y. Hsu, N. G. Chen and Y. Zhang, Adv. Opt. Mater., 2016, 4, 984 CrossRef CAS.
- M. J. Ruedas-Rama, J. D. Walters, A. Orte and E. A. H. Hall, Anal. Chim. Acta, 2012, 751, 1 CrossRef CAS PubMed.
- K. T. Shimizu, R. G. Neuhauser, C. A. Leatherdale, S. A. Empedocles, W. K. Woo and M. G. Bawendi, Phys. Rev. B, 2001, 63, 205316 CrossRef.
- A. L. Efros and D. J. Nesbitt, Nat. Nanotechnol., 2016, 11, 661 CrossRef CAS.
- Y. Wang, G. Fruhwirth, E. Cai, T. Ng and P. R. Selvin, Nano Lett., 2013, 13, 5233 CrossRef CAS PubMed.
- N. Coquelle, M. Sliwa, J. Woodhouse, G. Schirò, V. Adam, A. Aquila, T. R. M. Barends, S. Boutet, M. Byrdin, S. Carbajo, E. De la Mora, R. B. Doak, M. Feliks, F. Fieschi, L. Foucar, V. Guillon, M. Hilpert, M. S. Hunter, S. Jakobs, J. E. Koglin, G. Kovacsova, T. J. Lane, B. Lévy, M. N. Liang, K. Nass, J. Ridard, J. S. Robinson, C. M. Roome, C. Ruckebusch, M. Seaberg, M. Thepaut, M. Cammarata, I. Demachy, M. Field, R. L. Shoeman, D. Bourgeois, J. P. Colletier, I. Schlichting and M. Weik, Nat. Chem., 2018, 10, 31 CrossRef CAS PubMed.
- D. M. Chudakov, V. V. Verkhusha, D. B. Staroverov, E. A. Souslova, S. Lukyanov and K. A. Lukyanov, Nat. Biotechnol., 2004, 22, 1435 CrossRef CAS.
- M. Andresen, A. C. Stiel, J. Fölling, D. Wenzel, A. Schönle, A. Egner, C. Eggeling, S. W. Hell and S. Jakobs, Nat. Biotechnol., 2008, 26, 1035 CrossRef CAS PubMed.
- Y. Wu, Y. Zhu, C. Yao, J. Zhan, P. Wu, Z. Han, J. Zuo, H. Feng and Z. Qian, J. Mater. Chem. C, 2023, 11, 15393 RSC.
- X. Ping, J. Pan, X. Peng, C. Yao, T. Li, H. Feng and Z. Qian, J. Mater. Chem. C, 2023, 11, 7510 RSC.
- M. Olesińska-Mönch and C. Deo, Chem. Commun., 2023, 59, 660 RSC.
- Z. Tian, W. Wu and A. D. Q. Li, ChemPhysChem, 2009, 10, 2577 CrossRef CAS PubMed.
- L. Wang and Q. Li, Chem. Soc. Rev., 2018, 47, 1044 RSC.
- R. Pardo, M. Zayat and D. Levy, Chem. Soc. Rev., 2011, 40, 672 RSC.
- I. Yildiz, E. Deniz and F. M. Raymo, Chem. Soc. Rev., 2009, 38, 1859 RSC.
- J. J. Zhang, Q. Zou and H. Tian, Adv. Mater., 2013, 25, 378 CrossRef CAS.
- Z. Li, X. Gao, H. Zhang, X. Ma, Y. Liu, H. Guo and J. Yin, Chin. Chem. Lett., 2023, 34, 107645 CrossRef CAS.
- T. He and J. Yao, J. Mater. Chem., 2007, 17, 4547 RSC.
- A. B. A. Kayani, S. Kuriakose, M. Monshipouri, F. A. Khalid, S. Walia, S. Sriram and M. Bhaskaran, Small, 2021, 17, 2100621 CrossRef CAS PubMed.
- X. Yan, W. Zhong, S. Qu, Z. Li and L. Shang, J. Colloid Interface Sci., 2023, 646, 855 CrossRef CAS PubMed.
- M. Irie, T. Fulcaminato, K. Matsuda and S. Kobatake, Chem. Rev., 2014, 114, 12174 CrossRef CAS PubMed.
- L. Kortekaas and W. R. Browne, Chem. Soc. Rev., 2019, 48, 3406 RSC.
- H. M. D. Bandara and S. C. Burdette, Chem. Soc. Rev., 2012, 41, 1809 RSC.
- J. Volarić, W. Szymanski, N. A. Simeth and B. L. Feringa, Chem. Soc. Rev., 2021, 50, 12377 RSC.
- R. Yang, X. Ren, L. Mei, G. Pan, X.-Z. Li, Z. Wu, S. Zhang, W. Ma, W. Yu, H.-H. Fang, C. Li, M.-Q. Zhu, Z. Hu, T. Sun, X. Bin and W. Tian, Angew. Chem., Int. Ed., 2022, 61, e202117158 CrossRef CAS.
- D. Kim and S. Y. Park, Adv. Opt. Mater., 2018, 6, 1800678 CrossRef.
- M. M. Russew and S. Hecht, Adv. Mater., 2010, 22, 3348 CrossRef CAS.
- R. Klajn, J. F. Stoddart and B. A. Grzybowski, Chem. Soc. Rev., 2010, 39, 2203 RSC.
- R. D. Harris, S. Bettis Homan, M. Kodaimati, C. He, A. B. Nepomnyashchii, N. K. Swenson, S. Lian, R. Calzada and E. A. Weiss, Chem. Rev., 2016, 116, 12865 CrossRef CAS PubMed.
- M. A. Petersen, B. Rasmussen, N. N. Andersen, S. P. A. Sauer, M. B. Nielsen, S. R. Beeren and M. Pittelkow, Chem.–Eur. J., 2017, 23, 17010 CrossRef CAS PubMed.
- A. B. Grommet, L. M. Lee and R. Klajn, Acc. Chem. Res., 2020, 53, 2600 CrossRef CAS.
- T. Fukaminato, S. Ishida and R. Metivier, NPG Asia Mater., 2018, 10, 859 CrossRef CAS.
- L. Wang, W. Zhong, W. Gao, W. Liu and L. Shang, Chem. Eng. J., 2024, 479, 147490 CrossRef CAS.
- C. T. Kuo, A. M. Thompson, M. E. Gallina, F. Ye, E. S. Johnson, W. Sun, M. Zhao, J. Yu, I. C. Wu, B. Fujimoto, C. C. DuFort, M. A. Carlson, S. R. Hingorani, A. L. Paguirigan, J. P. Radich and D. T. Chiu, Nat. Commun., 2016, 7, 11468 CrossRef CAS PubMed.
- G. X. Feng, D. Ding, K. Li, J. Liu and B. Liu, Nanoscale, 2014, 6, 4141 RSC.
- D. Kim, K. Jeong, J. E. Kwon, H. Park, S. Lee, S. Kim and S. Y. Park, Nat. Commun., 2019, 10, 3089 CrossRef PubMed.
- M. Balter, S. M. Li, M. Morimoto, S. C. Tang, J. Hernando, G. Guirado, M. Irie, F. M. Raymo and J. Andréasson, Chem. Sci., 2016, 7, 5867 RSC.
- D. H. Hu, Z. Y. Tian, W. W. Wu, W. Wan and A. D. Q. Li, J. Am. Chem. Soc., 2008, 130, 15279 CrossRef CAS PubMed.
- J. Bu, K. Watanabe, H. Hayasaka and K. Akagi, Nat. Commun., 2014, 5, 3799 CrossRef CAS PubMed.
- J. Chen, W. B. Zhong, Y. Tang, Z. Wu, Y. Li, P. G. Yi and J. H. Jiang, Macromolecules, 2015, 48, 3500 CrossRef CAS.
- Y. Zhang, K. Zhang, J. Wang, Z. Tian and A. D. Q. Li, Nanoscale, 2015, 7, 19342 RSC.
- L. Y. Zhu, W. W. Wu, M. Q. Zhu, J. J. Han, J. K. Hurst and A. D. Q. Li, J. Am. Chem. Soc., 2007, 129, 3524 CrossRef CAS.
- M. Q. Zhu, G. F. Zhang, C. Li, M. P. Aldred, E. Chang, R. A. Drezek and A. D. Q. Li, J. Am. Chem. Soc., 2011, 133, 365 CrossRef CAS PubMed.
- M. L. Yu, P. S. Zhang, B. P. Krishnan, H. Wang, Y. Gao, S. Chen, R. J. Zeng, J. X. Cui and J. Chen, Adv. Funct. Mater., 2018, 28, 11 Search PubMed.
- P. Zhang, M. Xue, Z. Lin, H. Yang, C. Zhang, J. Cui and J. Chen, Sens. Actuators, B, 2022, 367, 132049 CrossRef CAS.
- Y. Akaishi, A. Mokhtar, M. Shimoyoshi, T. Nohara, Y. Inomata, D. Kosumi, T. Fukaminato and T. Kida, Small, 2022, 18, e2205046 CrossRef PubMed.
- S. A. Diaz, F. Gillanders, E. A. Jares-Erijman and T. M. Jovin, Nat. Commun., 2015, 6, 6036 CrossRef CAS PubMed.
- K. M. Greskovich, K. M. Powderly, M. M. Kincanon, N. B. Forney, C. A. Jalomo, A. Wo and C. J. Murphy, Acc. Chem. Res., 2023, 56, 1553 CrossRef CAS.
- K. Bhattacharjee and B. L. V. Prasad, Chem. Soc. Rev., 2023, 52, 2573 RSC.
- S. Padgaonkar, C. T. Eckdahl, J. K. Sowa, R. Lopez-Arteaga, D. E. Westmoreland, E. F. Woods, S. Irgen-Gioro, B. Nagasing, T. Seideman, M. C. Hersam, J. A. Kalow and E. A. Weiss, Nano Lett., 2021, 21, 854 CrossRef CAS PubMed.
- S. A. Diaz, G. O. Menendez, M. H. Etchehon, L. Giordano, T. M. Jovin and E. A. Jares-Erijman, ACS Nano, 2011, 5, 2795 CrossRef CAS PubMed.
- Z. Li, Q. Sun, Y. Zhu, B. Tan, Z. P. Xu and S. X. Dou, J. Mater. Chem. B, 2014, 2, 2793 RSC.
- L. Hou, R. Ringstrom, A. B. Maurer, M. Abrahamsson, J. Andreasson and B. Albinsson, J. Am. Chem. Soc., 2022, 144, 17758 CrossRef CAS.
- S. Bonacchi, A. Cantelli, G. Battistelli, G. Guidetti, M. Calvaresi, J. Manzi, L. Gabrielli, F. Ramadori, A. Gambarin, F. Mancin and M. Montalti, Angew. Chem., Int. Ed., 2016, 55, 11064 CrossRef CAS PubMed.
- Y. Nakagawa, B. D. Yu, Y. Niidome, K. Hayashi, A. Staykov, M. Yamada, T. Nakashima, T. Kawai, T. Fujigaya and T. Shiraki, J. Phys. Chem. C, 2022, 126, 10478 CrossRef CAS.
- B. Liao, W. Wang, P. Long, X. Deng, B. He, Q. Liu and S. Yi, Carbon, 2015, 91, 30 CrossRef CAS.
- Y. Cong, X. Wang, S. Zhu, L. Liu and L. Li, ACS Appl. Bio Mater., 2021, 4, 2790 CrossRef CAS PubMed.
- L. Zhu, M.-Q. Zhu, J. K. Hurst and A. D. Q. Li, J. Am. Chem. Soc., 2005, 127, 8968 CrossRef CAS PubMed.
- A. D. Pramata, Y. Akaishi, N. Kodama, Y. Mokuge, S. Kawashima, M. Shimoyoshi, C. Sairot, P. Nuket, P. Vas-Umnuay and T. Kida, ACS Appl. Nano Mater., 2021, 4, 4103 CrossRef CAS.
- J. Jeong, E. Yun, Y. Choi, H. Y. Jung, S. J. Chung, N. W. Song and B. H. Chung, Chem. Commun., 2011, 47, 10668 RSC.
- A. Mokhtar, R. Morinaga, Y. Akaishi, M. Shimoyoshi, S. Kim, S. Kurihara, T. Kida and T. Fukaminato, ACS Mater. Lett., 2020, 2, 727 CrossRef CAS.
- M. G. Romei, C. Y. Lin, I. I. Mathews and S. G. Boxer, Science, 2020, 367, 76 CrossRef CAS PubMed.
- J. Kwon, J. S. Park, M. Kang, S. Choi, J. Park, G. T. Kim, C. Lee, S. Cha, H. W. Rhee and S. H. Shim, Nat. Commun., 2020, 11, 273 CrossRef CAS PubMed.
- H. G. Fu, Y. Chen, X. Y. Dai and Y. Liu, Adv. Opt. Mater., 2020, 8, 5 Search PubMed.
- J. R. Nilsson, C. P. Carvalho, S. M. Li, J. P. Da Silva, J. Andreasson and U. Pischel, ChemPhysChem, 2012, 13, 3691 CrossRef CAS PubMed.
- H. J. Yu, H. Wang, F. F. Shen, F. Q. Li, Y. M. Zhang, X. Xu and Y. Liu, Small, 2022, 18, 2201737 CrossRef CAS PubMed.
- W. Zhong, X. Yan, S. Qu and L. Shang, Aggregate, 2023, 4, e245 CrossRef CAS.
- Y. Fu, H.-H. Han, J. Zhang, X.-P. He, B. L. Feringa and H. Tian, J. Am. Chem. Soc., 2018, 140, 8671 CrossRef CAS.
- X. Chai, H.-H. Han, A. C. Sedgwick, N. Li, Y. Zang, T. D. James, J. Zhang, X.-L. Hu, Y. Yu, Y. Li, Y. Wang, J. Li, X.-P. He and H. Tian, J. Am. Chem. Soc., 2020, 142, 18005 CrossRef CAS PubMed.
- J. Andréasson and U. Pischel, Coord. Chem. Rev., 2021, 429, 213695 CrossRef.
- Y. M. Zhang, Y. H. Liu and Y. Liu, Adv. Mater., 2020, 32, e1806158 CrossRef PubMed.
- I. Yildiz, S. Impellizzeri, E. Deniz, B. McCaughan, J.
F. Callan and F. M. Raymo, J. Am. Chem. Soc., 2011, 133, 871 CrossRef CAS PubMed.
- L. Yang, Y. Li, H. Zhang, C. Tian, Q. Cao, Y. Xiao, L. Yuan and G. Liu, Chin. Chem. Lett., 2023, 34, 108108 CrossRef CAS.
- R. Zhang, Y. Chen and Y. Liu, Angew. Chem., Int. Ed., 2023, 62, e202315749 CrossRef CAS PubMed.
- D. Kim, A. Aktalay, N. Jensen, K. Uno, M. L. Bossi, V. N. Belov and S. W. Hell, J. Am. Chem. Soc., 2022, 144, 14235 CrossRef CAS PubMed.
- M. Canton, A. B. Grommet, L. Pesce, J. Gemen, S. Li, Y. Diskin-Posner, A. Credi, G. M. Pavan, J. Andreasson and R. Klajn, J. Am. Chem. Soc., 2020, 142, 14557 CrossRef CAS PubMed.
- H. Q. Zheng, Y. Yang, Z. Wang, D. Yang, G. Qian and Y. Cui, Adv. Mater., 2023, 35, e2300177 CrossRef.
- T. Zhao, J. Han, Y. Shi, J. Zhou and P. Duan, Adv. Mater., 2021, 33, e2101797 CrossRef PubMed.
- L. Shang and G. U. Nienhaus, Mater. Today, 2013, 16, 58 CrossRef CAS.
- B. Hao, J. Wang, C. Wang, K. Xue, M. Xiao, S. Lv and C. Zhu, Chem. Sci., 2022, 13, 4139 RSC.
- T. Stafforst and D. Hilvert, Chem. Commun., 2009, 3, 287 RSC.
- M. Shen, C. Huang, Y. Xiao, R. Huang, V. K.-M. Au and T. Yu, J. Mater. Chem. C, 2023, 11, 11486 RSC.
- S. J. Chen, W. L. Li, X. Li and W. H. Zhu, J. Mater. Chem. C, 2017, 5, 2717 RSC.
- C. Özçoban, T. Halbritter, S. Steinwand, L.-M. Herzig, J. Kohl-Landgraf, N. Askari, F. Groher, B. Fürtig, C. Richter, H. Schwalbe, B. Suess, J. Wachtveitl and A. Heckel, Org. Lett., 2015, 17, 1517 CrossRef PubMed.
- K. Uno, M. L. Bossi, M. Irie, V. N. Belov and S. W. Hell, J. Am. Chem. Soc., 2019, 141, 16471 CrossRef CAS PubMed.
- D. Moldenhauer and F. Gröhn, Chem.–Eur. J., 2017, 23, 3966 CrossRef CAS PubMed.
- K. Torii, Y. Hori and K. Kikuchi, Anal. Chem., 2023, 95, 8834 CrossRef CAS PubMed.
- M. Tomasulo, I. Yildiz and F. M. Raymo, Inorg. Chim. Acta, 2007, 360, 938 CrossRef CAS.
- S. Aiken, R. J. L. Edgar, C. D. Gabbutt, B. M. Heron and P. A. Hobson, Dyes Pigm., 2018, 149, 92 CrossRef CAS.
- P. K. Kundu, S. Das, J. Ahrens and R. Klajn, Nanoscale, 2016, 8, 19280 RSC.
- W. Zhong, K. Liang, W. Liu and L. Shang, Chem. Sci., 2023, 14, 8823 RSC.
- X. Zhang, B. Wang, Z. Zheng, G. Yang, C. Zhang and L. Liao, Dyes Pigm., 2023, 208, 110800 CrossRef.
- M. Yu, W. Zhao, F. Ni, Q. Zhao and C. Yang, Adv. Opt. Mater., 2022, 10, 9 Search PubMed.
- D. S. Wang, T. T. Zhang, B. Wu, C. X. Ye, Z. Y. Wei, Z. Q. Cao and G. J. Wang, Macromolecules, 2019, 52, 7130 CrossRef CAS.
- J. Kim, H. Yun, Y. J. Lee, J. Lee, S. H. Kim, K. H. Ku and B. J. Kim, J. Am. Chem. Soc., 2021, 143, 13333 CrossRef CAS PubMed.
- G. Naren, C. W. Hsu, S. Li, M. Morimoto, S. Tang, J. Hernando, G. Guirado, M. Irie, F. M. Raymo, H. Sunden and J. Andreasson, Nat. Commun., 2019, 10, 3996 CrossRef CAS PubMed.
- D. Samanta, D. Galaktionova, J. Gemen, L. J. W. Shimon, Y. Diskin-Posner, L. Avram, P. Kral and R. Klajn, Nat. Commun., 2018, 9, 641 CrossRef PubMed.
- H.-Q. Zheng, Y. Cui and G. Qian, Acc. Mater. Res., 2023, 4, 982 CrossRef CAS.
- A. M. Rice, C. R. Martin, V. A. Galitskiy, A. A. Berseneva, G. A. Leith and N. B. Shustova, Chem. Rev., 2020, 120, 8790 CrossRef CAS.
- A. B. Grommet, M. Feller and R. Klajn, Nat. Nanotechnol., 2020, 15, 256 CrossRef CAS PubMed.
- G. C. Thaggard, K. C. Park, J. Lim, B. K. P. Maldeni Kankanamalage, J. Haimerl, G. R. Wilson, M. K. McBride, K. L. Forrester, E. R. Adelson, V. S. Arnold, S. T. Wetthasinghe, V. A. Rassolov, M. D. Smith, D. Sosnin, I. Aprahamian, M. Karmakar, S. K. Bag, A. Thakur, M. Zhang, B. Z. Tang, J. A. Castano, M. N. Chaur, M. M. Lerch, R. A. Fischer, J. Aizenberg, R. Herges, J. M. Lehn and N. B. Shustova, Nat. Commun., 2023, 14, 7556 CrossRef CAS PubMed.
- G. R. Wilson, K. C. Park, G. C. Thaggard, C. R. Martin, A. R. Hill, J. Haimerl, J. Lim, B. K. P. Maldeni Kankanamalage, B. J. Yarbrough, K. L. Forrester, R. A. Fischer, P. J. Pellechia, M. D. Smith, S. Garashchuk and N. B. Shustova, Angew. Chem., Int. Ed., 2023, 62, e202308715 CrossRef CAS PubMed.
- D. E. Williams, C. R. Martin, E. A. Dolgopolova, A. Swifton, D. C. Godfrey, O. A. Ejegbavwo, P. J. Pellechia, M. D. Smith and N. B. Shustova, J. Am. Chem. Soc., 2018, 140, 7611 CrossRef CAS PubMed.
- K. Hema, A. B. Grommet, M. J. Bialek, J. Wang, L. Schneider, C. Drechsler, O. Yanshyna, Y. Diskin-Posner, G. H. Clever and R. Klajn, J. Am. Chem. Soc., 2023, 145, 24755 CAS.
- J. Gemen, J. R. Church, T.-P. Ruoko, N. Durandin, M. J. Bialek, M. WeiSsenfels, M. Feller, M. Kazes, M. Odaybat, V. A. Borin, R. Kalepu, Y. Diskin-Posner, D. Oron, M. J. Fuchter, A. Priimagi, I. Schapiro and R. Klajn, Science, 2023, 381, 1357 CrossRef CAS PubMed.
- Z. Chu, Y. Han, T. Bian, S. De, P. Kral and R. Klajn, J. Am. Chem. Soc., 2019, 141, 1949 CrossRef CAS PubMed.
- J. Wang, L. Avram, Y. Diskin-Posner, M. J. Białek, W. Stawski, M. Feller and R. Klajn, J. Am. Chem. Soc., 2022, 144, 21244 CrossRef CAS PubMed.
- M. Li, J. Zhao, H. Chu, Y. Mi, Z. Zhou, Z. Di, M. Zhao and L. Li, Adv. Mater., 2019, 31, 1804745 CrossRef CAS.
- A. A. Ali, R. Kharbash and Y. Kim, Anal. Chim. Acta, 2020, 1110, 199 CrossRef CAS PubMed.
- Z. Y. Tian, W. W. Wu, W. Wan and A. D. Q. Li, J. Am. Chem. Soc., 2011, 133, 16092 CrossRef CAS PubMed.
- C. Guo, J. Y. Zhai, Y. F. Wang, W. Yang and X. J. Xie, Anal. Chem., 2021, 93, 8128 CrossRef CAS PubMed.
- Y. Xie, M. C. Arno, J. T. Husband, M. Torrent-Sucarrat and R. K. O'Reilly, Nat. Commun., 2020, 11, 2460 CrossRef CAS PubMed.
- C. Guo, J. Y. Zhai, Y. F. Wang, X. F. Du, Z. G. Wang and X. J. Xie, Anal. Chem., 2022, 94, 1531 CrossRef CAS PubMed.
- Z. Li, G. Wang, Y. Ye, B. Li, H. Li and B. Chen, Angew. Chem., Int. Ed., 2019, 131, 18193 CrossRef.
- M. L. Yu, P. S. Zhang, L. Liu, H. Wang, H. Wang, C. H. Zhang, Y. Gao, C. L. Yang, J. X. Cui and J. Chen, Adv. Opt. Mater., 2021, 9, 8 Search PubMed.
- S. Yamasaki, S. Ishida, S. Kim, M. Yamada, T. Nakashima, T. Kawai, S. Kurihara and T. Fukaminato, Chem. Commun., 2021, 57, 5422 RSC.
- J. K. Ji, X. Li, T. T. Wu and F. D. Feng, Chem. Sci., 2018, 9, 5816 RSC.
- K. Jeong, S. Park, Y. D. Lee, C. K. Lim, J. Kim, B. H. Chung, I. C. Kwon, C. R. Park and S. Kim, Adv. Mater., 2013, 25, 5574 CrossRef CAS.
- H. Y. Jung, B. Kim, M. H. Jeon and Y. Kim, Small, 2022, 18, 10 Search PubMed.
- Y. Kim, H. Y. Jung, Y. H. Choe, C. Lee, S. K. Ko, S. Koun, Y. Choi, B. H. Chung, B. C. Park, T. L. Huh, I. Shin and E. Kim, Angew. Chem., Int. Ed., 2012, 51, 2878 CrossRef CAS.
- J. Qi, C. Chen, X. Zhang, X. Hu, S. Ji, R. T. K. Kwok, J. W. Y. Lam, D. Ding and B. Z. Tang, Nat. Commun., 2018, 9, 1848 CrossRef.
- H. L. Li and J. C. Vaughan, Chem. Rev., 2018, 118, 9412 CrossRef CAS PubMed.
- F. M. Jradi and L. D. Lavis, ACS Chem. Biol., 2019, 14, 1077 CrossRef CAS PubMed.
- W. L. Gong, J. Yan, L. X. Zhao, C. Li, Z. L. Huang, B. Z. Tang and M. Q. Zhu, Photochem. Photobiol. Sci., 2016, 15, 1433 CrossRef CAS.
- Q. X. Hua, B. Xin, Z. J. Xiong, W. L. Gong, C. Li, Z. L. Huang and M. Q. Zhu, Chem. Commun., 2017, 53, 2669 RSC.
- J. X. Liu, B. Xin, C. Li, N. H. Xie, W. L. Gong, Z. L. Huang and M. Q. Zhu, ACS Appl. Mater. Interfaces, 2017, 9, 10338 CrossRef CAS PubMed.
- Z. Y. Tian, A. D. Q. Li and D. H. Hu, Chem. Commun., 2011, 47, 1258 RSC.
- A. G. Godin, A. Setaro, M. Gandil, R. Haag, M. Adeli, S. Reich and L. Cognet, Sci. Adv., 2019, 5, eaax1166 CrossRef CAS PubMed.
- E. Siemes, O. Nevskyi, D. Sysoiev, S. K. Turnhoff, A. Oppermann, T. Huhn, W. Richtering and D. Woll, Angew. Chem., Int. Ed., 2018, 57, 12280 CrossRef CAS.
|
This journal is © The Royal Society of Chemistry 2024 |