DOI:
10.1039/D4SC00034J
(Edge Article)
Chem. Sci., 2024,
15, 7789-7794
Copper-catalyzed remote double functionalization of allenynes†
Received
3rd January 2024
, Accepted 10th April 2024
First published on 20th April 2024
Abstract
Addition reactions of molecules with conjugated or non-conjugated multiple unsaturated C–C bonds are very attractive yet challenging due to the versatile issues of chemo-, regio-, and stereo-selectivities. Especially for the readily available conjugated allenyne compounds, the reactivities have not been explored. The first example of copper-catalyzed 2,5-hydrofunctionalization and 2,5-difunctionalization of allenynes, which provides a facile access to versatile conjugated vinylic allenes with a C–B or C–Si bond, has been developed. This mild protocol has a broad substrate scope tolerating many synthetically useful functional groups. Due to the highly functionalized nature of the products, they have been demonstrated as platform molecules for the efficient syntheses of monocyclic products including poly-substituted benzenes, bicyclic compounds, and highly functionalized allene molecules.
Unsaturated hydrocarbons are a class of very important compounds due to their ubiquity in organic synthesis, natural products, materials, and pharmaceutics.1–4 Of particular interest, studies on versatile reactivities of C–C multiple bonds have been drawing more and more attention from organic, medicinal, and materials chemists.5–7 The reactions of the C–C double bond and C–C triple bond, including hydrofunctionalization and difunctionalization reactions, have been extensively developed with attractive regio- and stereo-selectivity (Schemes 1A and B).8–42 Recently, the addition reactions of allenes, which deliver stereodefined olefins with decent regioselectivities, have also been established (Scheme 1C).43–60 Such reactions of molecules with multiple unsaturated C–C bonds are very attractive yet challenging due to the versatile issues of chemo-, regio-, and stereo-selectivities (Scheme 1D).61–63 Although conjugated enynes and dienes have been studied,64–66 the reaction of allenynes merging an alkyne unit and an allene unit in a conjugated manner has not been studied.67–69 It would be challenging to control the related selectivities forming different 1,2-, 2,3-, 2,5-, 4,5-addition products with different unsaturated C–C bonds (Scheme 1E). Herein, we wish to disclose our recent observation on copper-catalyzed highly regioselective 2,5-boryl- and silyl-cupration of readily available conjugated allene-ynes for efficient synthesis of conjugated vinylic allenes with a versatile C–B or C–Si bond (Scheme 1F).
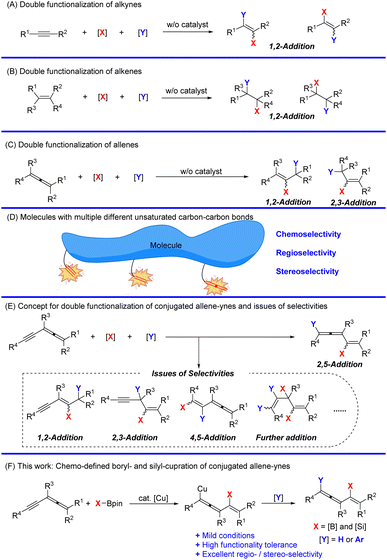 |
| Scheme 1 Double functionalization of alkynes, alkenes, allenes, and conjugated allene-ynes. | |
We initiated the study with the reaction of allenyne 1a70–89 and B2(pin)22a in the presence of MeOH with NaOtBu as the base and CuCl (5 mol%) and Biphep (L1) (6 mol%) as the catalyst at 30 °C in THF for 16 h. To our delight, the 2,5-addition product, 1,3,4-trienyl boronate 3aa, was formed in 33% yield with 3% yield of the 2,3-addition product, 1,4-enyne 3aa′ (entry 1, Table 1). Upon adjusting the ratio of 1a/2a to 1/1, a much higher yield of the desired product 3aa was achieved (entries 2–5, Table 1). When the reaction was conducted at 15 °C, the yield of 3aa was improved to 85% with 5% yield of 3aa′ (entry 6, Table 1). Interestingly, when MeOH was replaced with tBuOH, the generation of 3aa′ was completely suppressed with 43% yield of 2,5-double functionalization product 3aa (entry 9, Table 1). Further screening of the ligand and [Cu] with tBuOH led to the observation that the combination of Binap (L2) and Cu(CH3CN)4PF6 was better than that with Biphep and CuCl as the catalyst with the yield of 86% with 4% recovery of 1a (entries 10–11, Table 1). Upon adjusting the ratio of 1a/2a to 1/1.05, the reaction delivered the best results affording 3aa in 87% yield with no recovery of 1a and no formation of 3aa′ (entry 12, Table 1).
Table 1 Optimization of the conditionsa
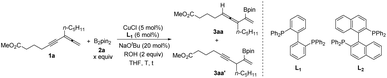
|
Entry |
ROH |
X
|
T (oC) |
t (h) |
1a (%)b |
3aa/3aa′ (%)b |
Reaction conditions: 1a (0.2 mmol), 2a (X equiv.), CuCl (5 mol%), Biphep (L1) (6 mol%), NaOtBu (20 mol%), and ROH (2 equiv.) in THF (1 mL).
Determined by 1H NMR analysis of the crude product using mesitylene as the internal standard.
Binap (L2) was applied instead of Biphep (L1).
Cu(CH3CN)4PF6 was applied instead of CuCl.
|
1 |
MeOH |
2.0 |
30 |
16 |
0 |
33/3 |
2 |
MeOH |
1.5 |
30 |
16 |
0 |
51/6 |
3 |
MeOH |
1.2 |
30 |
15.5 |
0 |
62/7 |
4 |
MeOH |
1.1 |
30 |
15.5 |
0 |
73/6 |
5 |
MeOH |
1.0 |
30 |
15.5 |
0 |
80/6 |
6 |
MeOH |
1.0 |
15 |
5.5 |
5 |
85/5 |
7 |
MeOH |
1.0 |
40 |
5.5 |
4 |
80/4 |
8 |
MeOH |
1.0 |
50 |
5 |
4 |
71/3 |
9 |
t
BuOH |
1.0 |
15 |
16 |
35 |
43/0 |
10c |
t
BuOH |
1.0 |
15 |
13 |
10 |
83/0 |
11c,d |
t
BuOH |
1.0 |
15 |
1 |
4 |
86/0 |
12c,d |
t
BuOH |
1.05 |
15 |
1 |
0 |
87/0 |
With the optimized reaction conditions in hand (entry 12, Table 1), we next investigated the reactivity of various allenynes (Table 2). A series of allenynes bearing different R1 substituents incorporating functional groups, such as ester (3aa), highly strained cyclopropyl (3ca), benzyl (3da), halide (3ea), and nitrile (3ga) were all tolerated with the yields of 77–97%. In addition, the substrate with the OH group being protected as TBS ether also could undergo the reaction smoothly with the yield of 80% (3fa). Even the glucose-derived allenyne also afforded the boryl-substituted 1,3,4-triene 3ha with the yield of 65%. R1 may also be aryl groups; a variety of different substituents on the aryl units including electron-donating (3ja and 3ka) and electron-withdrawing groups as well as biologically or synthetically useful groups such as F, Cl, Br, CF3, CN, and COOMe at the para-, ortho-, or meta-position (3la–3sa) were accommodated to afford the 1,3,4-trien-2-yl boronates in moderate to good yields (64–87%). p-NO2 on the benzene ring also survived with 48% yield of 3ta. Different R2 groups bearing halide (3ua, 76%) and benzyl (3va, 53%) were tolerated.
Table 2 Scope of 2,5-hydrofunctionalization
Reaction conditions: 1, 2a (1.05 equiv.), tBuOH (2.0 equiv.), Cu(CH3CN)4PF6 (5 mol%), Binap (L2) (6 mol%), and NaOtBu in THF (5 mL) at 15 °C on a 1.0 mmol scale.
1.1 equiv. 2a was used.
Reaction conditions: 1 (1 equiv.), 4a (1.5 equiv.), tBuOH (2.0 equiv.), Cu(CH3CN)4PF6 (5 mol%), Binap (6 mol%) and NaOtBu in THF (5 mL) at 15 °C on a 1.0 mmol scale.
3ua′ is 2-(3-(3-chloropropyl)-9-methoxy-9-oxonon-1-en-4-yn-2-yl)-4,4,5,5-tetramethyl-1,3,2-dioxaborolane.
3va′ is 2-(3-benzyl-9-methoxy-9-oxonon-1-en-4-yn-2-yl)-4,4,5,5-tetramethyl-1,3,2-dioxaborolane.
On a 0.5 mmol scale.
|
|
Furthermore, B2(pin)22a may be replaced with Me2PhSiBpin 4a. The corresponding 1,3,4-trien-2-yl silanes bearing different R1 functional groups, such as ester (5aa), halide (5ea), and nitrile (5ga), were obtained in yields of 70–78%. In addition, synthetically versatile p-OMe (5ka), -Cl (5ma), -Br (5pa), and -CN (5ra) on the benzene ring also survived with the yields of 66–79%. As for different substitutions at the R2 position, 1u (R2 = ClC3H6–) and 1y (R2 = PhC2H4–) were tested to afford the corresponding silane products 5ua in 61% yield and 5ya in 71% yield, respectively.
In addition, trisubstituted allenyne (1w) and tetrasubstituted allenyne (1x) are also suitable for affording the corresponding Z- and E-isomers of 1,3,4-trienyl boronates 3wa in 68% yield and 3xa in 68% yield. Furthermore, the reaction of 1w also works with Me2PhSiBpin 4a affording 1,3,4-trien-2-yl silanes E-5wa in 75% yield (Scheme 2). When we tried iodobenzene as the electrophile under standard conditions, the target arylborylation product 7aa was not produced. Interestingly, 7aa was formed when an extra catalytic amount of Pd(dba)2 was introduced (Table 3). Further screening of the temperature and ligand led to the formation of 7aa in 53% yield. Aryl iodides with different electron-donating or -withdrawing groups afforded 7ab, 7ac, 7ad, 7ae, and 7af in 42–51% yields. Allenynes with different alkyl substituents (R2 = n-C6H13–, AcOC4H8–) also afforded the desired products 7ya and 7za (Table 3). 2,5-Hydrofunctionalization byproducts 3 were observed in all the cases, indicating that the Cu-catalyzed borylation reaction occurred before the Pd cross-coupling reaction with ArI.
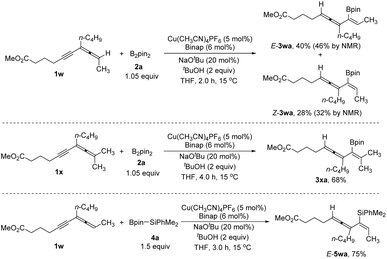 |
| Scheme 2 Scope of trisubstituted and tetrasubstituted allenynes | |
Table 3 Scope of 2,5-arylborationa
Reaction conditions: 1 (0.1 mmol), 2a (1.4 equiv.), 6 (1.4 equiv.), Cu(CH3CN)4PF6 (7.5 mol%), Pd(dba)2 (5 mol%), dppf (12.5 mol%), and NaOtBu (2 equiv.) in THF (1 mL) at 70 °C on a 0.1 mmol scale.
1H NMR yield of 2,5-hydroboration by-products 3aa, 3ya, and 3za in parentheses.
|
|
To gain insight into the mechanism, a deuterium-labelling experiment was conducted. When the reaction was performed with a stoichiometric amount of tBuOD, 70% of [D]-3aa with 75% deuterium incorporation at the allenic position was observed (Scheme 3A). When the R1 group in 1z′ is a highly sterically hindered TMS group, the reaction failed to afford the 1,3,4-trienes. Instead, 1,4-enyne [D]-3za′ with 66% deuterium incorporation was formed, indicating that the steric hindrance of TMS plays a critical role in determining the regioselectivity (Scheme 3B). On the basis of these experimental data, we proposed a possible mechanism (Scheme 3C). Initially, the copper–boryl complex I is generated in situ via the reaction of B2(pin)2 with the Cu precursor. Then, the C
C bond in the allene next to the C–C triple bond highly regioselectively inserted into the copper–boryl bond in I with the boron connected to the middle carbon atom to afford the propargylic copper species II, which would rapidly isomerize to allenyl copper species III and subsequently get trapped with tBuOD to produce the final product [D]-3, regenerating LCuOtBu to complete this catalytic cycle. Meanwhile, protonation of another isomeric form II at the γ position would also afford product [D]-3. When the R group is the sterically bulky TMS group, II would directly undergo protonolysis to form 1,4-enyne [D]-3za′. In the first step, the allene was inserted into the copper–boryl bond in intermediate I since the allene unit is more reactive than the alkyne unit. The regioselectivity for the protonolysis depended on the steric hindrance of the R1 group in copper species II or III.
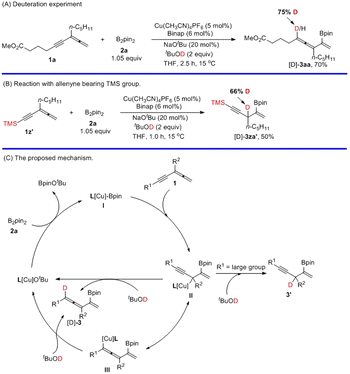 |
| Scheme 3 Deuteration experiment and the proposed mechanism. | |
In order to showcase the synthetic utility of the products, further transformations of the 1,3,4-trien-2-yl boronates/silanes were demonstrated (Scheme 4): The one-pot sequential 2,5-borylcurpration of allenyne 8 and a Diels–Alder reaction with maleic anhydride afforded stereodefined bicyclic lactonyl borate 9 (Scheme 4A).90 Similarly, allenene 3aa could also be readily converted to corresponding bicyclic lactone product 10, 1,4-cyclohexadiene with a stereodefined exo-cyclic C
C double bond 12, and penta-substituted benzene 13via a Diels–Alder reaction with dimethyl but-2-ynedioate under different conditions. Such differently polysubstituted benzenes are difficult to prepare yet very useful.91 In order to show the reactivity of the C–B bond, the Diels–Alder product 13 was employed for further transformation. Firstly, 13 was successfully coupled with methyl 4-iodobenzoate to afford 14 in 89% yield.92 Secondly, the iodination of 13 with KI afforded the iodination product 15 in 49% yield catalyzed by copper iodide under air.93 Thirdly, bromination of the C–B bond in 13 has been realized to afford 16 in an excellent yield by its treatment with copper bromide.93 The intramolecular cycloisomerization of 3aa also proceeded smoothly to give the corresponding cyclopentadienyl boronate 11 (Scheme 4B).94 Moreover, 3aa could be readily converted into 2-alkynyl-1,3,4-triene 17via alkynylation with alkynyl bromide79 and allenyl ketone 18via oxidation with H2O2.95 In addition, the C–B bond in 3aa was successfully coupled with methyl 4-iodobenzoate to afford 19 in 38% yield even in the presence of a highly reactive allene unit (Scheme 4C). Meanwhile, other dienophiles, such as 1,4-naphthoquinone, maleic anhydride, and N-phenylmaleimide, could all undergo the Diels–Alder reaction with silane 5aa to produce the corresponding products 20, 21, and 22 in decent yields (Scheme 4D).90
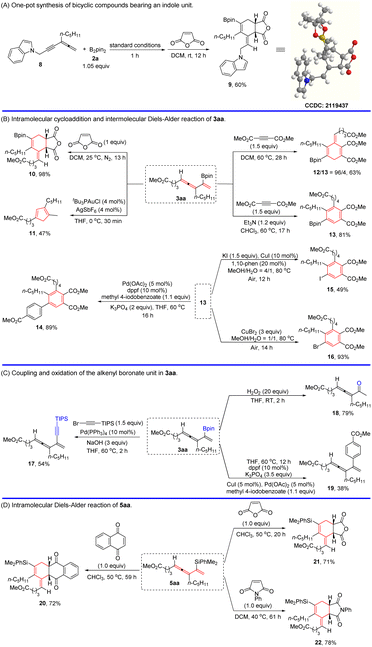 |
| Scheme 4 Synthetic applications. | |
Conclusions
In summary, we have developed the first example of copper-catalyzed 2,5-boryl- or silyl-cupration of allenynes, providing an efficient protocol for substituted 1,3,4-trien-2-yl boronates or silanes with excellent regioselectivity. This method has advantages of a readily available catalyst and starting materials, high catalytic activity, mild conditions, a broad substrate scope tolerating many synthetically useful functional groups, and synthetically useful functionalities. The synthetic utility of this protocol has been demonstrated via the versatile transformations of the resulting products to furnish valuable functionalized molecules. Further studies are being actively pursued in our laboratory.
Data availability
The data supporting this article have been uploaded as the ESI.†
Author contributions
S. M. and J. Z. conceived and designed the experiments. Y. S., J. Z. performed the experiments. Y. S., C. F., J. Z., and S. M. wrote the manuscript. S. M. and J. Z. directed the research.
Conflicts of interest
There are no conflicts to declare.
Acknowledgements
Financial support from the National Key R&D Program of China (2022YFA1503200), the National Natural Science Foundation of China (21988101), and the Fundamental Research Funds for the Central Universities (226-2022-00224) is greatly appreciated. We thank Mr Wenxiang He in this group for reproducing the preparation of 3ga, 3ta and 5ka. Shengming Ma is a Qiu Shi Adjunct Professor at Zhejiang University.
Notes and references
- R. Aloise Pilli and M. da Conceição Ferreira de Oliveira, Nat. Prod. Rep., 2000, 17, 117–127 RSC.
- G. R. Bedford and D. N. Richardson, Nature, 1966, 212, 733–734 CrossRef CAS.
- M. Irie, Chem. Rev., 2000, 100, 1685–1716 CrossRef CAS PubMed.
-
J. Wang, Stereoselective Alkene Synthesis, Springer, 2012 Search PubMed.
- J. L. Kennemur, R. Maji, M. J. Scharf and B. List, Chem. Rev., 2021, 121, 14649–14681 CrossRef CAS PubMed.
- H. Pan, K. Liu, A. Caracciolo and P. Casavecchia, Chem. Soc. Rev., 2017, 46, 7517–7547 RSC.
- H. Olivier-Bourbigou, P. A. R. Breuil, L. Magna, T. Michel, M. F. Espada Pastor and D. Delcroix, Chem. Rev., 2020, 120, 7919–7983 CrossRef CAS PubMed.
- J. Chen and Z. Lu, Org. Chem. Front., 2018, 5, 260–272 RSC.
- S. Zhang, H. Neumann and M. Beller, Chem. Soc. Rev., 2020, 49, 3187–3210 RSC.
- D. Mandal, S. Roychowdhury, J. P. Biswas, S. Maiti and D. Maiti, Chem. Soc. Rev., 2022, 51, 7358–7426 RSC.
- V. W. Bhoyare, A. G. Tathe, A. Das, C. C. Chintawar and N. T. Patil, Chem. Soc. Rev., 2021, 50, 10422–10450 RSC.
- H. Jiang and A. Studer, Chem. Soc. Rev., 2020, 49, 1790–1811 RSC.
- Z.-L. Li, G.-C. Fang, Q.-S. Gu and X.-Y. Liu, Chem. Soc. Rev., 2020, 49, 32–48 RSC.
- L.-J. Cheng and N. P. Mankad, Chem. Soc. Rev., 2020, 49, 8036–8064 RSC.
- J. R. Wilkinson, C. E. Nuyen, T. S. Carpenter, S. R. Harruff and R. Van Hoveln, ACS Catal., 2019, 9, 8961–8979 CrossRef CAS.
- K. Semba, T. Fujihara, J. Terao and Y. Tsuji, Tetrahedron, 2015, 71, 2183–2197 CrossRef CAS.
- D. Hemming, R. Fritzemeier, S. A. Westcott, W. L. Santos and P. G. Steel, Chem. Soc. Rev., 2018, 47, 7477–7494 RSC.
- Y. Tsuji and T. Fujihara, Chem. Rec., 2016, 16, 2294–2313 CrossRef CAS PubMed.
- J. Yun, Asian J. Org. Chem., 2013, 2, 1016–1025 CrossRef CAS.
- T. Fujihara, K. Semba, J. Terao and Y. Tsuji, Catal. Sci. Technol., 2014, 4, 1699–1709 RSC.
- H. Mei, Z. Yin, J. Liu, H. Sun and J. Han, Chin. J. Chem., 2019, 37, 292–301 CrossRef CAS.
- Q. Wang, X. Yu, J. Jin, Y. Wu and Y. Liang, Chin. J. Chem., 2018, 36, 223–226 CrossRef CAS.
- J. Guo, Z. Cheng, J. Chen, X. Chen and Z. Lu, Acc. Chem. Res., 2021, 54, 2701–2716 CrossRef CAS PubMed.
- X. Cong and X. Zeng, Acc. Chem. Res., 2021, 54, 2014–2026 CrossRef CAS PubMed.
- R. Y. Liu and S. L. Buchwald, Acc. Chem. Res., 2020, 53, 1229–1243 CrossRef CAS PubMed.
- L. M. Wickham and R. Giri, Acc. Chem. Res., 2021, 54, 3415–3437 CrossRef CAS PubMed.
- W. Zhao, H.-X. Lu, W.-W. Zhang and B.-J. Li, Acc. Chem. Res., 2023, 56, 308–321 CrossRef CAS PubMed.
- S. Ma and J. F. Hartwig, Acc. Chem. Res., 2023, 56, 1565–1577 CrossRef CAS PubMed.
- R. Alfaro, A. Parra, J. Alemán, J. L. García Ruano and M. Tortosa, J. Am. Chem. Soc., 2012, 134, 15165–15168 CrossRef CAS PubMed.
- L. Zhang, J. Cheng, B. Carry and Z. Hou, J. Am. Chem. Soc., 2012, 134, 14314–14317 CrossRef CAS PubMed.
- H. Yoshida, S. Kawashima, Y. Takemoto, K. Okada, J. Ohshita and K. Takaki, Angew. Chem., Int. Ed., 2012, 51, 235–238 CrossRef CAS PubMed.
- H. Yoshida, I. Kageyuki and K. Takaki, Org. Lett., 2013, 15, 952–955 CrossRef CAS PubMed.
- P. Liu, Y. Fukui, P. Tian, Z.-T. He, C.-Y. Sun, N.-Y. Wu and G.-Q. Lin, J. Am. Chem. Soc., 2013, 135, 11700–11703 CrossRef CAS PubMed.
- Y. Zhou, W. You, K. B. Smith and M. K. Brown, Angew. Chem., Int. Ed., 2014, 53, 3475–3479 CrossRef CAS PubMed.
- Y. D. Bidal, F. Lazreg and C. S. J. Cazin, ACS Catal., 2014, 4, 1564–1569 CrossRef CAS.
- T. Itoh, Y. Shimizu and M. Kanai, J. Am. Chem. Soc., 2016, 138, 7528–7531 CrossRef CAS PubMed.
- W. Su, T.-J. Gong, Q. Zhang, Q. Zhang, B. Xiao and Y. Fu, ACS Catal., 2016, 6, 6417–6421 CrossRef CAS.
- S.-H. Kim-Lee, I. Alonso, P. Mauleón, R. G. Arrayás and J. C. Carretero, ACS Catal., 2018, 8, 8993–9005 CrossRef CAS.
- L.-J. Cheng and N. P. Mankad, Angew. Chem., Int. Ed., 2018, 57, 10328–10332 CrossRef CAS PubMed.
- Z.-L. Wang, Y. Wang, J.-L. Xu, M. Zhao, K.-Y. Dai, C.-C. Shan and Y.-H. Xu, Org. Lett., 2021, 23, 4736–4742 CrossRef CAS PubMed.
- W.-D. Chu, C.-M. Wang, J. Zhan, Y.-L. Zeng, Y. Liu, B. Chen, K. Zhang, C.-Y. He and Q.-Z. Liu, Eur. J. Org Chem., 2023, 26, e202300210 CrossRef CAS.
- Y. Gao, S. Yazdani, A. Kendrick IV, G. P. Junor, T. Kang, D. B. Grotjahn, G. Bertrand, R. Jazzar and K. M. Engle, Angew. Chem., Int. Ed., 2021, 60, 19871–19878 CrossRef CAS PubMed.
- R. Blieck, M. Taillefer and F. Monnier, Chem. Rev., 2020, 120, 13545–13598 CrossRef CAS PubMed.
- E. Soriano and I. Fernández, Chem. Soc. Rev., 2014, 43, 3041–3105 RSC.
- X. Huang and S. Ma, Acc. Chem. Res., 2019, 52, 1301–1312 CrossRef CAS PubMed.
- J. Ye and S. Ma, Acc. Chem. Res., 2014, 47, 989–1000 CrossRef CAS PubMed.
- R. Zimmer, C. U. Dinesh, E. Nandanan and F. A. Khan, Chem. Rev., 2000, 100, 3067–3126 CrossRef CAS PubMed.
- J. M. Alonso, M. T. Quirós and M. P. Muñoz, Org. Chem. Front., 2016, 3, 1186–1204 RSC.
- G. Qiu, J. Zhang, K. Zhou and J. Wu, Tetrahedron, 2018, 74, 7290–7301 CrossRef CAS.
- C. Aubert, L. Fensterbank, P. Garcia, M. Malacria and A. Simonneau, Chem. Rev., 2011, 111, 1954–1993 CrossRef CAS PubMed.
- L. Liu, R. M. Ward and J. M. Schomaker, Chem. Rev., 2019, 119, 12422–12490 CrossRef CAS PubMed.
- W. Yuan and S. Ma, Adv. Synth. Catal., 2012, 354, 1867–1872 CrossRef CAS.
- K. Semba, M. Shinomiya, T. Fujihara, J. Terao and Y. Tsuji, Chem.–Eur. J., 2013, 19, 7125–7132 CrossRef CAS PubMed.
- H. Jang, B. Jung and A. H. Hoveyda, Org. Lett., 2014, 16, 4658–4661 CrossRef CAS PubMed.
- J. Rae, K. Yeung, J. J. W. McDouall and D. J. Procter, Angew. Chem., Int. Ed., 2016, 55, 1102–1107 CrossRef CAS PubMed.
- W. Zhao and J. Montgomery, J. Am. Chem. Soc., 2016, 138, 9763–9766 CrossRef CAS PubMed.
- A. Boreux, K. Indukuri, F. Gagosz and O. Riant, ACS Catal., 2017, 7, 8200–8204 CrossRef CAS.
- K. Yeung, F. J. T. Talbot, G. P. Howell, A. P. Pulis and D. J. Procter, ACS Catal., 2019, 9, 1655–1661 CrossRef CAS.
- J. Han, W. Zhou, P.-C. Zhang, H. Wang, R. Zhang, H.-H. Wu and J. Zhang, ACS Catal., 2019, 9, 6890–6895 CrossRef CAS.
- C.-C. Shan, K.-Y. Dai, M. Zhao and Y.-H. Xu, Eur. J. Org Chem., 2021, 2021, 4054–4058 CrossRef CAS.
- J. Walkowiak, J. Szyling, A. Franczyk and R. L. Melen, Chem. Soc. Rev., 2022, 51, 869–994 RSC.
- C. Nájera, I. P. Beletskaya and M. Yus, Chem. Soc. Rev., 2019, 48, 4515–4618 RSC.
- A. M. Asiri and A. S. K. Hashmi, Chem. Soc. Rev., 2016, 45, 4471–4503 RSC.
- J. Xuan and A. Studer, Chem. Soc. Rev., 2017, 46, 4329–4346 RSC.
- A. G. Csákÿ, G. d. l. Herrán and M. C. Murcia, Chem. Soc. Rev., 2010, 39, 4080–4102 RSC.
- L. Fu, S. Greßies, P. Chen and G. Liu, Chin. J. Chem., 2020, 38, 91–100 CrossRef CAS.
- For a report on Ag- or Au-catalyzed cyclization of allenols with an alkynyl substituent, see: S. Kim and P. H. Lee, Adv. Synth. Catal., 2008, 350, 547–551 CrossRef CAS.
- For a report on the reaction of allenols bearing an alkynyl substituent with allylic halides in the presence of In, the reaction afforded 1,2-products, see: S. Kim and P. H. Lee, Eur. J. Org Chem., 2008, 2008, 2262–2266 CrossRef.
- For a report on the Cu-catalyzed reaction of 3-pentyldeca-1,2-diene-3-yne with PhB(OH)2, the reaction only afforded the 1,2-product, see: T. Itoh, Y. Shimizu and M. Kanai, Org. Lett., 2014, 16, 2736–2739 CrossRef CAS PubMed.
- T. Mandai, M. Ogawa, H. Yamaoki, T. Nakata, H. Murayama, M. Kawada and J. Tsuji, Tetrahedron Lett., 1991, 32, 3397–3398 CrossRef CAS.
- G. A. Molander, E. M. Sommers and S. R. Neufeldt, J. Org. Chem., 2006, 71, 1563–1568 CrossRef CAS PubMed.
- S. Ma, Z. Gu and Y. Deng, Chem. Commun., 2006, 94–96 RSC.
- W. Shu, G. Jia and S. Ma, Org. Lett., 2009, 11, 117–120 CrossRef CAS PubMed.
- W. Shu and S. Ma, Tetrahedron, 2010, 66, 2869–2874 CrossRef CAS.
- G. Chen, Y. Zhang, C. Fu and S. Ma, Tetrahedron, 2011, 67, 2332–2337 CrossRef CAS.
- J. Ye, S. Li and S. Ma, Org. Biomol. Chem., 2013, 11, 5370–5373 RSC.
- J. Ye, S. Li and S. Ma, Org. Lett., 2012, 14, 2312–2315 CrossRef CAS PubMed.
- H. Wang, H. Luo, Z.-M. Zhang, W.-F. Zheng, Y. Yin, H. Qian, J. Zhang and S. Ma, J. Am. Chem. Soc., 2020, 142, 9763–9771 CrossRef CAS PubMed.
- S.-H. Yu, T.-J. Gong and Y. Fu, Org. Lett., 2020, 22, 2941–2945 CrossRef CAS PubMed.
- J. A. Dabrowski, F. Haeffner and A. H. Hoveyda, Angew. Chem., Int. Ed., 2013, 52, 7694–7699 CrossRef CAS PubMed.
- X.-F. Wei, T. Wakaki, T. Itoh, H.-L. Li, T. Yoshimura, A. Miyazaki, K. Oisaki, M. Hatanaka, Y. Shimizu and M. Kanai, Chem, 2019, 5, 585–599 CAS.
- G. E. Akpınar, M. Kuş, M. Üçüncü, E. Karakuş and L. Artok, Org. Lett., 2011, 13, 748–751 CrossRef PubMed.
- E. Ş. Karagöz, M. Kuş, G. E. Akpınar and L. Artok, J. Org. Chem., 2014, 79, 9222–9230 CrossRef PubMed.
- M. Kuş, L. Artok and M. Aygün, J. Org. Chem., 2015, 80, 5494–5506 CrossRef PubMed.
- N. Cadran, K. Cariou, G. Hervé, C. Aubert, L. Fensterbank, M. Malacria and J. Marco-Contelles, J. Am. Chem. Soc., 2004, 126, 3408–3409 CrossRef CAS PubMed.
- S. I. Lee, S. H. Sim, S. M. Kim, K. Kim and Y. K. Chung, J. Org. Chem., 2006, 71, 7120–7123 CrossRef CAS PubMed.
- S. H. Sim, S. I. Lee, J. Seo and Y. K. Chung, J. Org. Chem., 2007, 72, 9818–9821 CrossRef CAS PubMed.
- M. Murakami, S. Kadowaki and T. Matsuda, Org. Lett., 2005, 7, 3953–3956 CrossRef CAS PubMed.
- B. S. Schreib, M. Son, F. A. Aouane, M.-H. Baik and E. M. Carreira, J. Am. Chem. Soc., 2021, 143, 21705–21712 CrossRef CAS PubMed.
- H. Luo, Y. Yu and S. Ma, Org. Chem. Front., 2016, 3, 1705–1710 RSC.
- S. Saito and Y. Yamamoto, Chem. Rev., 2000, 100, 2901–2916 CrossRef CAS PubMed.
- V. Němec, M. Hylsová, L. Maier, J. Flegel, S. Sievers, S. Ziegler, M. Schröder, B.-T. Berger, A. Chaikuad, B. Valčíková, S. Uldrijan, S. Drápela, K. Souček, H. Waldmann, S. Knapp and K. Paruch, Angew. Chem., Int. Ed., 2019, 58, 1062–1066 CrossRef PubMed.
- G. Wang, L. Liu, H. Wang, Y.-S. Ding, J. Zhou, S. Mao and P. Li, J. Am. Chem. Soc., 2017, 139, 91–94 CrossRef CAS PubMed.
- J. H. Lee and F. D. Toste, Angew. Chem., Int. Ed., 2007, 46, 912–914 CrossRef CAS PubMed.
- C. Yang, Z. L. Liu, D. T. Dai, Q. Li, W. W. Ma, M. Zhao and Y. H. Xu, Org. Lett., 2020, 22, 1360–1367 CrossRef CAS PubMed.
|
This journal is © The Royal Society of Chemistry 2024 |