DOI:
10.1039/D3SC06222H
(Edge Article)
Chem. Sci., 2024,
15, 4019-4030
Molecular engineering of AIE-active boron clustoluminogens for enhanced boron neutron capture therapy†
Received
21st November 2023
, Accepted 1st February 2024
First published on 1st February 2024
Abstract
The development of boron delivery agents bearing an imaging capability is crucial for boron neutron capture therapy (BNCT), yet it has been rarely explored. Here we present a new type of boron delivery agent that integrates aggregation-induced emission (AIE)-active imaging and a carborane cluster for the first time. In doing so, the new boron delivery agents have been rationally designed by incorporating a high boron content unit of a carborane cluster, an erlotinib targeting unit towards lung cancer cells, and a donor–acceptor type AIE unit bearing naphthalimide. The new boron delivery agents demonstrate both excellent AIE properties for imaging purposes and highly selective accumulation in tumors. For example, at a boron delivery agent dose of 15 mg kg−1, the boron amount reaches over 20 μg g−1, and both tumor/blood (T/B) and tumor/normal cell (T/N) ratios reach 20–30 times higher than those required by BNCT. The neutron irradiation experiments demonstrate highly efficient tumor growth suppression without any observable physical tissue damage and abnormal behavior in vivo. This study not only expands the application scopes of both AIE-active molecules and boron clusters, but also provides a new molecular engineering strategy for a deep-penetrating cancer therapeutic protocol based on BNCT.
Introduction
The research and development of boron-containing functional molecules have attracted extensive attention and represent a cutting-edge frontier in chemistry owing to the unique electronic structure and properties of the boron atom.1 It is noteworthy that a 10B atom possesses a high neutron-capture cross-section (3835 barns), which renders 10B-based molecules highly desirable as neutron capture agents for boron neutron capture therapy (BNCT). The fundamental principle of BNCT is based on the nuclear reaction that upon irradiation by thermal neutrons, a 10B atom releases an alpha particle (4He2+) and recoil lithium nucleus (7Li3+), which enables the killing of a cancer cell in a mode of inside one single cell (5–9 μm). Thus BNCT could minimize damage to healthy tissues which makes it an advanced binary and cell-level radiotherapy.2,3 However, the clinical applications of BNCT were severely hampered because known boron delivery agents such as BPA (boronophenylalanine) and BSH (sodium mercaptoundecahydro-closo-dodecaborate)2f,g (Fig. 1a) have issues such as low boron content (BPA), low in vivo stability and solubility, poor biocompatibility, and lack of targeting and imaging. Currently, the key issue for BNCT is the development of a new generation of boron delivery agents that can fulfil the requirements of BNCT.
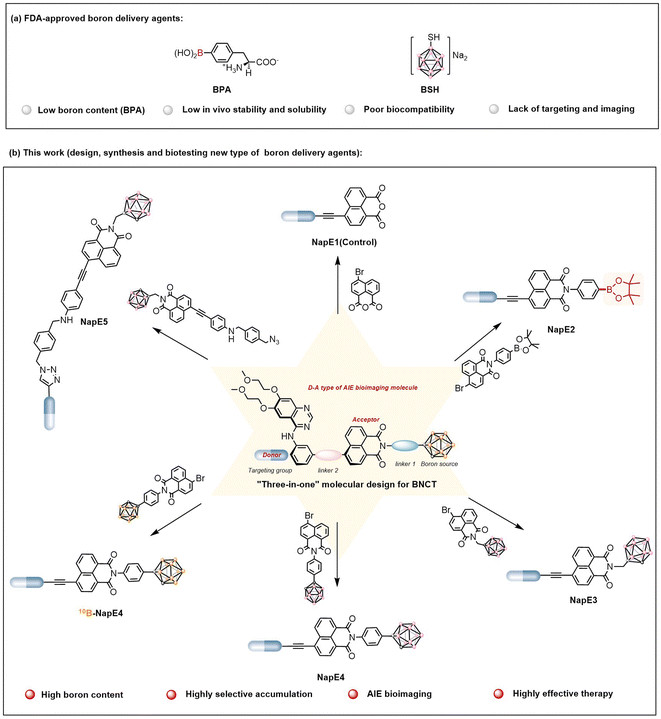 |
| Fig. 1 (a) Two FDA-approved boron delivery agents for BNCT. (b) New boron delivery agents for BNCT based on the “three-in-one” molecular design strategy (this work). | |
BNCT requires delivery agents containing a substantial boron content.2 Notably, icosahedral carboranes (i.e., C2B10H12) are a type of classical boron-enriched clusters bearing diverse properties4 such as low toxicity, biocompatibility, unique electronic properties, three-dimensional aromaticity, large size, tunable hydrophobicity and hydrophilicity, and high chemical and thermal stabilities. Thus carboranes have been extensively used for biological activity studies,5 luminescent materials,6 bioimaging7 and others.8 In particular, carboranes have also been utilized to design delivery agents for BNCT investigations, which have demonstrated different effectiveness in vitro and in vivo.9 Despite these important advances, the development of carborane-based delivery agents is sporadic and still faces challenges such as low biocompatibility, inadequate tumor accumulation, low tumor/blood (T/B) and tumor/normal cell (T/N) ratios, and lack of imaging. Undoubtedly, the development of multifunctional boron-enriched delivery agents with high biocompatibility, targeting, imaging, and therapeutic efficacy will hold great potential for high-performance applications in BNCT for both diagnosis and treatment.
Among various imaging techniques, fluorescence imaging is a useful tool for non-invasive cancer diagnosis. However, traditional luminogens used for biological imaging usually suffer from the aggregation-caused quenching effect,10a which restricts the working concentration in biological systems. In particular, BNCT requires extremely high boron content (>109 10B atoms/per cancer cell) accumulated inside tumor cells. As such, aggregation-induced emission luminogens (AIEgens) are appropriate for bioimaging in the design of multifunctional delivery agents as they can exhibit bright emission in an aggregate state, excellent photobleaching resistance, and a high signal-to-noise ratio.10 Until now, by means of the rational design of AIEgens, biomedical applications related to AIE-active imaging have been developed including real-time tracing,11 fluorescence sensing,12 tumor imaging,13 photodynamic therapy (PDT),14 photothermal therapy (PTT),15 surgical navigation,16 and immunotherapy.17 Nevertheless, to the best of our knowledge, AIEgens have not yet been reported in BNCT investigations. Based on the above considerations, AIEgens should be ideal tracking reagents for BNCT for visualization purposes.
To address the aforementioned issues in boron delivery agents for BNCT applications, in this study, a new molecular platform that integrates AIE-active imaging with a boron-enriched cluster to construct a new type of boron delivery agent was developed for the first time. As a proof of concept, boron delivery agents based on a “three-in-one” strategy were designed by incorporating a high boron content unit of carborane, an Eriotinib-based lung cancer targeting unit, and a D−A (donor−acceptor) type AIE imaging unit using naphthalimide as a strong acceptor (Fig. 1b). Such a molecular system exhibited the expected AIE properties with red emissions, negligible toxicity and excellent biocompatibility. More importantly, highly selective 10B accumulation (>20 μg g−1) inside lung cancer cells was achieved even when a low dose (15 mg kg−1) of the boron delivery agent 10B-NapE4 was applied (for comparison, the dose of BPA for the treatment of melanoma used was 600 mg kg−1 to give a 10B accumulation of 36 ± 4 μg g−1 (ref. 2b)). In particular, the T/B and T/N ratios reached up to 30
:
1, far beyond 3
:
1 as required by BNCT. Under AIE imaging, satisfactory outcomes have been obtained as evidenced by efficient tumor growth inhibition on A549 tumor-bearing mice (a model for lung cancer) with no physical tissue damage and abnormal behavior during a 10-day observation after neutron irradiation. This work presents multiple functionalized boron delivery agents for BNCT investigations and expands the type of tumors applicable to BNCT. The findings may demonstrate that BNCT is not only suitable for superficial cancers such as melanoma, and head and neck cancers, but also has great potential in deep-penetrating cancer treatment.
Results and discussion
“Three-in-one” molecular design of boron delivery agents
The two clinically used boron delivery agents as shown in Fig. 1a2a have either inadequate boron content or inadequate targeting function, and both also lack imaging capability. In this study, to address these issues, we proposed a “three-in-one” strategy for molecular design which includes a boron source as a pharmacophore, a targeting functional group to deliver boron delivery agents into the targeted tumors, and an imaging functional unit to track the pathways of a boron delivery agent (Fig. 1b). Despite valuable progress in other boron-containing carriers,18 here a carborane cluster was chosen as a boron source owing to its advantages such as high boron content (i.e., one carborane cluster contains ten boron atoms), high stability, and low toxicity.5 On the other hand, previous BNCT treatments focused on superficial cancers such as melanoma, head and neck cancers, and brain glioma. In this study, we explored the possibility of treating deep-penetrating cancers, for instance, lung tumors. In doing so, the widely used non-small cell lung cancer-targeting drug, Eriotinib, was selected as a targeting moiety to deliver boron delivery agents to obtain sufficient boron accumulation in tumors. It is noted that Eriotinib was approved as a small-molecule-targeted epidermal growth factor receptor (EGFR) inhibitor by the Food and Drug Administration (FDA) in 2004,19 and exhibits a specific affinity to tumor cells by targeting the adenosine triphosphate binding domain of tyrosine kinase in EGFR-overexpressing tumors.20 Moreover, to achieve real-time tracking of boron delivery agents, an imaging unit needs to be incorporated. As BNCT requires a very high boron amount inside cancer cells, the boron delivery agents are optimally designed as AIEgens to ensure the effectiveness of imaging. Based on the AIE working mechanism,10 luminogens with intramolecular charge transfer are capable of exhibiting AIE properties. As such, we exploited the chromophore of naphthalimide in molecular design as a strong electron acceptor since naphthalimide has been commonly used for biological imaging.21 Notably, here the carborane moiety is also a three-dimensional steric group that can enhance AIE emission, and for the first time Eriotinib acts as an electron donor to be used in the construction of luminogens. Thus the D–A type of AIE-active luminescent boron delivery agents have been drafted, denoted as NapE3-5, as depicted in Fig. 1b. Such an integration of the molecular moieties of naphthalimide, carborane and Eriotinib may lead to AIE properties for the expected boron delivery agents.
Preparation and characterization of the NapE compounds
To synthesize the above-designed boron delivery agents, we initially used the B10H12(CH3CN)2 precursor to react with naphthalimide-substituted alkynes to obtain carborane-conjugated naphthalimide intermediates in moderate yields. The targeted products NapE3-5 were generated by the use of either the classic Sonogashira reaction or copper(I)-catalyzed azide-alkyne cycloaddition in high yields. In addition, the control compounds NapE1 without a boron atom and NapE2 containing one boron atom were also synthesized for comparison (Fig. 1b, 2, S1 and Schemes S1–S5†). All of these compounds were thoroughly characterized by using Fourier transform infrared (FT-IR) spectra (Fig. S2†), NMR spectra (Fig. S21–S33†), and high-resolution mass spectrum (HRMS), as shown in the ESI.†
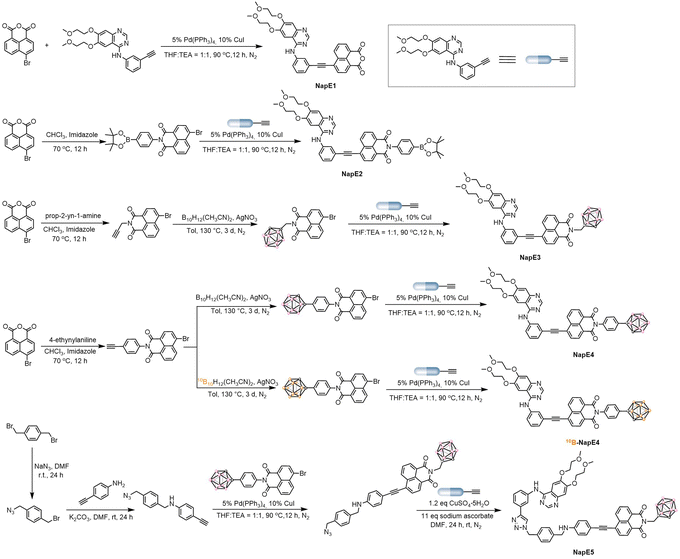 |
| Fig. 2 Synthesis of five carborane-based boron delivery agents. | |
Photophysical properties of the NapE compounds
With the carborane-based compounds in hand, the photophysical properties were investigated to examine the above molecular design (Fig. 3a, S3, Tables S1 and S2†). In solution, the absorption bands of NapE compounds were observed within the range of 300–500 nm. The long-wavelength absorption band is beneficial for the molecular imaging study. NapE1-4 exhibits a comparable absorption spectral profile while NapE5 displays a more red-shifted absorption band which could be attributed to the strong electron-donating ability of the N-alkyl phenyl group. All the absorption bands for NapE1-5 arise from charge transfer, as supported by the separate electronic distributions of HOMOs (highest occupied molecular orbitals) and LUMOs (lowest unoccupied molecular orbitals) in the ground state (Fig. 3b and S4†). The photoluminescence (PL) spectra of NapE1-5 show broad emission bands with large Stokes shifts beyond 100 nm (Fig. S3b and Table S1†). Besides, the emission peaks range from 523 to 664 nm and are red-shifted in comparison to those of the individual Eriotinib and naphthalimide.20f,21d The emissions should originate from the charge transfer state.6h In particular, NapE5 shows a deep red emission with a CIE (Commission Internationale de l'Eclairage) coordinate of (0.68, 0.32) (Fig. S3c†) due to the strong electron-donating ability of N-alkyl phenyl. To confirm CT emission, PL spectra in different solvents were investigated for NapE1-5 (Fig. S5 and Table S3†). They all show solvatochromic luminescence where upon increasing the polarity of the solvent, a more bathochromic emission appears. This indicates that the excited luminophore is in balance with the solvent, leading to a strong dependence on solvent polarity.22 Further theoretical calculations23 also reveal that the hole localizes on the Eriotinib unit, whereas the electron resides on phenyl and partial naphthalimide (Fig. S6†). All the observations demonstrate a charge transfer emission feature, consistent with the D–A type structural design for luminescence. We further evaluated the AIE properties of NapE4-5 by using DMSO and water-mixed solvents (Fig. 3c–e and S7†). In DMSO solution, weak emissions were observed for the two compounds. These should be attributed to intramolecular charge transfer emission in a polar solvent, which leads to strong non-radiative decay in the excited state in solution. The finding is in accordance with classical ICT-based molecular systems.11 Upon increasing the water fraction (fw), the PL intensity was increased, which reached the maximum at fw = 99%. To validate the enhancement of PL emission triggered by molecular aggregation, both dynamic light scattering (DLS) and transmission electron microscopy (TEM) were used to verify the formation of nanoparticles. As illustrated in Fig. S8,† at fw = 99% an average diameter of 100–160 nm was observed for NapE4-5. The zeta-potential values of NapE4 and NapE5 in aqueous solution (Fig. S8c†) were measured to be −27 and −54 mV, respectively. Next, we measured the absolute quantum yields of NapE4-5 in DMSO to be 1.0% and 2.0%, respectively, and in the mixed DMSO/water at fw = 99% to be 5.6% and 11.4%, respectively. The above results demonstrate the AIE properties for the designed molecular system. The AIE mechanism of NapE4-5 is attributed to the restriction of molecular motions.10 In solution, strong non-radiative decay occurs in the excited state due to molecular motions, particularly the rotation at the ethynyl linker, which results in weak emission. However, when aggregated, the easy molecular motions in solution are suppressed. On the other hand, a large-size carborane cluster can also disrupt non-specific intermolecular interactions that usually cause concentration quenching. Consequently, enhanced emission can be observed in aggregates. Besides, Eriotinib is also approved as a good electron donor for AIEgen design. Thereby this study provides the first example of an Eriotinib-based D–A molecular system and demonstrates multiple applications of Eriotinib.
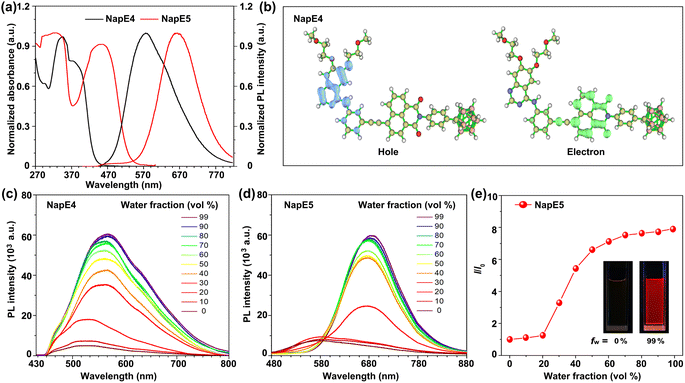 |
| Fig. 3 (a) Normalized UV-vis absorption spectra (left side) and PL spectra (right side) at room temperature in the mixed solvents for NapE4-5 (the volume ratio between PBS buffer solution and DMSO = 95 : 5, 10 μM, λex = 365 nm). (b) HOMO and LUMO distributions of NapE4 in the ground state. PL spectra of NapE4 (c) and NapE5 (d) in mixed DMSO/water with different water fractions (fw). (e) The plot of the relative emission intensity (I/I0) versus water fraction. I0 and I are the peak values of PL intensities of NapE5 in DMSO and DMSO/water mixtures, respectively. Inset: luminescence photograph. | |
Cytotoxicity, cellular uptake and localization of NapE compounds
As BNCT requires low cytotoxicity for boron delivery agents, the cytotoxicity of NapE1-5 on the A549 lung cancer cells was examined by CCK8 assay. Except for NapE2, they all exhibited negligible cytotoxic effect on A549 cells at concentrations lower than 60 μM at 24 h, 48 h and even 72 h, respectively (Fig. S9†). The cytotoxicity of carborane-based NapE3-5 is less than that of the one-boron-atom-containing compound NapE2. Specifically, no cytotoxicity was observed even at a concentration of 100 μM for NapE5. These results reveal that the carborane moiety not only provides high boron content, but also reduces the cytotoxicity of NapE compounds. Next, the stability of NapE1-5 was examined in a physiological environment, and showed no observable change in the UV-vis absorption intensity after 72 h of incubation in a PBS buffer solution (Fig. S10†). Thus, NapE1-5 was considered for further biological imaging applications. Firstly, the initial inspection of the uptake of NapE1-5 in A549 cells was performed by confocal microscopy, which showed that the fluorescence intensity of NapE4-5 was considerably higher than that of NapE2-3 at 48 h (Fig. S11†). Next NapE4-5 was selected to monitor the cellular uptake of A549 cells at a concentration of 60 μM for different time periods. The microscopy images showed that NapE4-5 could be transported to the cytoplasm of lung cancer cells and reached the maximum around 48 h culture (Fig. 4a and S12†). Besides, a good overlap of fluorescence imaging between NapE4-5 and EGFR was observed (Fig. 4a and S12†). Notably, owing to the advantage of AIE properties, both boron delivery agents exhibited bright imaging in cancer cells even at a concentration of 60 μM (Fig. S12†). Secondly, we further evaluated the cellular uptake of NapE4-5 in A549 cells by inductively coupled plasma mass spectrometry (ICP-MS). The boron content reached 68.85 ± 9.58 ng/106 cells at 24 h, 217.90 ± 5.08 ng/106 cells at 48 h and 132.54 ± 4.35 ng/106 cells at 72 h for NapE4 (Fig. 4b and Table S4†). For NapE5, the boron content reached 29.06 ± 4.03 ng/106 cells at 24 h, 46.28 ± 1.73 ng/106 cells at 48 h, and 41.83 ± 3.44 ng/106 cells at 72 h (Fig. 4b and Table S4†). It is clear that NapE4 enters A549 cells more quickly and efficiently, which might be attributed to its relatively lower molecular weight (806.4107 g mol−1) in comparison to NapE5 (1006.5148 g mol−1).
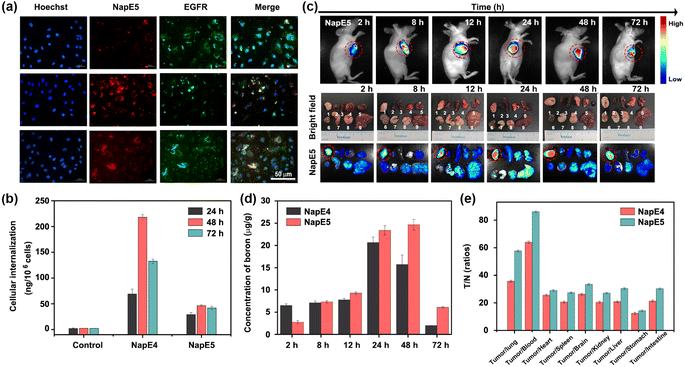 |
| Fig. 4 (a) Confocal microscopy images of A549 cells incubated with NapE5 (60 μM), Hoechst and EGFR (24 h, 48 h, 72 h; NapE5, λex = 559 nm and λem = 600–720 nm; EGFR, λex = 488 nm and λem = 500–600 nm; Hoechst: λex = 405 nm and λem = 400–500 nm), scale bar = 50 μm. (b) The boron content of A549 cells incubated with NapE4-5 (60 μM). (c) Fluorescence images of A549 tumor-bearing mice and organs at different times after intravenous injection of 15 mg kg−1 of NapE5. 1: tumor; 2: heart; 3: spleen; 4: lung; 5: kidney; 6: intestine; 7: stomach; 8: brain; 9: liver (NapE5: λex = 455 nm and λem = 580–750 nm). (d) The boron content of NapE4-5 internalized in the A549 tumor-bearing mice at different times. (e) The T/N ratio of the boron content of different organs from A549 tumor-bearing mice at 24 h for NapE4-5. | |
To demonstrate the generality of the molecular design, H2228 cancer cells (low-sensitive lung cancer cells) were also selected and incubated with NapE4-5 to test the intracellular boron content. To our delight, more fast and higher uptake (i.e.NapE4: 293.36 ± 4.35 ng/106 cells at 72 h and NapE5: 78.41 ± 4.03 ng/106 cells at 24 h) was obtained (Fig. S12, 13 and Table S4†). The ICP-MS analytical results are well consistent with the AIE fluorescence indication, demonstrating the significance of bioimaging in this research. Moreover, BEAS-2B cells, human bronchial epithelial cells, were also incubated with NapE4-5 to test cytotoxicity and intracellular boron content (Fig. S9g–h, S13c and Table S4†). The results showed that the two compounds have no cytotoxicity on normal cells and the intracellular boron contents in normal cells are much lower than those in lung cancer cells. The aforementioned findings suggest that the incorporation of an Eriotinib unit into NapE4-5 has led to high uptake in both highly sensitive and low sensitive lung cancer cells. These results have established a basis for a subsequent in vivo imaging study.
In vivo imaging and biological distribution of NapE4-5
The boron content in tumors is a crucial factor for the therapeutic efficacy of BNCT. To track the dynamic biological distribution of boron delivery agents, fluorescence navigation continued to be employed. As shown in Fig. 4c and S14,†NapE4-5 reached the maximum concentrations in the lung tumors in the period of 24–48 h of administration at a dose of 15 mg kg−1. Such a retention time period is sufficient for further experiments. To our delight, the images of other organs in mice showed that the fluorescence intensities are rather weak, demonstrating that targeting delivery has been achieved for the two compounds (Fig. 4c and S14†). Moreover, bioimaging could show a time-dependent distribution of compounds in mice (Fig. S14 and S15†), and therefore, the delivery of two boron delivery agents could be in vivo monitored.
To quantify the uptake of the boron delivery agents in vivo, the boron content in different organs at different times was measured by ICP-MS (Fig. 4d, e and Tables S5–S8†). For NapE4, the boron accumulation in tumors was measured to be 20.66 ± 1.23 μg g−1 (tumors) after 24 h of injection. In sharp contrast, the uptake in other organs such as the lung, brain, kidney, liver, spleen, stomach, small intestine, heart and blood was quite lower (<1.67 μg g−1, Table S5 and Fig. S16a†), and a higher T/N of 35.70 ± 0.73 and T/B of 64.03 ± 0.78 were obtained (Fig. 4e, S16c and Table S6†). NapE5 demonstrated better results for boron accumulation: 23.41 ± 1.02 μg g; T/N = 57.70 ± 0.63; T/B = 86.17 ± 0.65 at 24 h (Fig. 4e, S16b, d, Tables S7 and S8†). Similarly, NapE5 showed very low uptake in other organs (<1.65 μg g−1, Table S7†). These data exceed the values required by BNCT (i.e. boron accumulation of 20 μg g−1, T/N = 3 and T/B = 3), demonstrating the excellent tumor selectivity of the carborane-based boron delivery agents. Note that NapE5 showed longer retention in tumor cells than NapE4 (Fig. 4d), which might be attributed to its molecular size. Again, a strong correlation between the boron content measured by ICP-MS and the fluorescence imaging outcomes in vivo was observed. These results have proven our molecular design on both targeted delivery and imaging tracking, which lay the foundation for high efficacy and biosafety upon neutron irradiation. Further hematoxylin and eosin (H&E) staining analyses of the main organs, which were harvested on the 7th day, showed no evident pathological damage or change. This indicates that the compounds have good biocompatibility in vivo (Fig. S17†) and are suitable for further neutron irradiation experiments.
Neutron irradiation of cells
After consideration of the synthesis and biological activity, NapE4 was chosen for further neutron irradiation experiments. As such, NapE4 was replaced by 10B-enriched 10B-NapE4 (Fig. 5a), which was synthesized as shown in Fig. 1b, 2 and Scheme S5.† The photophysical properties of 10B-NapE4 and its cellular uptake at 48 h were measured again (Fig. S3d, e and Table S9†), and are similar to those of NapE4. Next, A549 cells were treated with different concentrations of 10B-NapE4 for 48 h, and then exposed to neutron irradiation with different doses (low dose: 17 min and high dose: 26 min). After this, the irradiated cells were cultured to examine cell viability. As a result, the cell proliferation was considerably inhibited with an increased concentration of 10B-NapE4 (Fig. 5b, S18a and b†). After 72 h the cell proliferation was reduced by 67.7% (for 26 min exposure) and 46.7% (for 17 min exposure) with a concentration of 60 μM of 10B-NapE4 (Table S10†). Moreover, the colony formation assay also showed excellent dose dependence. After 14 days of culture, the colony formation rate was decreased by 47.0% with a concentration of 60 μM in comparison to the control group with no boron delivery agents and irradiation applied (Fig. 5c, S18c, d and Table S11†). These results indicate that 10B-NapE4 can efficiently inhibit the proliferation and colony formation of tumor cells after neutron irradiation, demonstrating its suitability for further in vivo investigations.
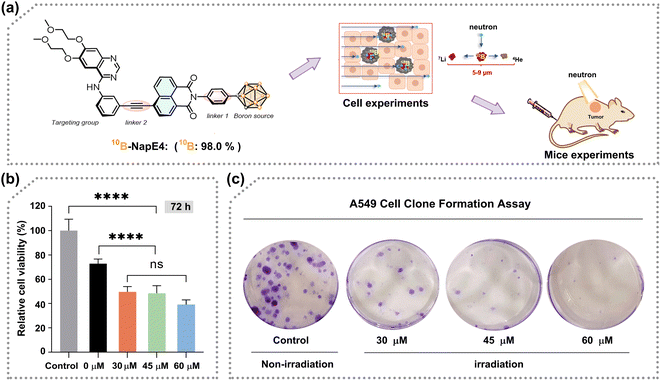 |
| Fig. 5 (a) 10B-NapE4 instead of NapE4 for neutron irradiation experiments. (b) A549 cell viability observed after 72 hours post-26 minute neutron irradiation exposure. P values = ****p < 0.0001; ***p < 0.001; ns: no significance. (c) The clone formation assay of irradiated A549 cells observed at the 14th day. Note: The control group was not exposed to neutron irradiation with no boron delivery agents applied. | |
Neutron irradiation of tumor-bearing mice
Similarly, the boron content of the selected organs after 24 h injection of 10B-NapE4 (15 mg kg−1) in the tumor-bearing mice (n = 3) needs to be tested again to ensure the consistent boron content accumulation in vivo after replacement of NapE4. The 10B accumulation in tumors was 22.66 ± 3.28 μg g−1, and the T/N and T/B ratios were 35.42 ± 1.84 and 28.93 ± 2.29, respectively, similar to those in NapE4 (Table S12†). Then 10B-NapE4 was intravenously administered at a dose of 15 mg kg−1 body weight in subcutaneous A549 tumor-bearing mice. After 24 h the mice were exposed to different doses of neutron beams (L BNCT; M BNCT; H BNCT; Fig. S19 and Table S13†). For comparison, three other groups were also set up as the control, 10B-NapE4 injection only (or drug only), and neutron irradiation only (2.0 Gy) (or neutron only), respectively. Firstly, the biosafety of the irradiation was assessed by measuring the body weight of the mice. No obvious adverse effects or abnormalities were observed, indicating that 10B-NapE4 is well tolerated (Fig. 6a). Secondly, no evident effect on tumor growth was observed in the three comparison groups. In sharp contrast, in the three 10B-NapE4 + irradiation groups, obvious suppression of tumor growth was observed after 10 days of irradiation (Fig. 6b and c). In particular, in the H BNCT group, the tumor growth was significantly inhibited at such a low dose of boron delivery agent (15 mg kg−1). Thus, the in vivo experimental results demonstrate that 10B-NapE4 is an efficient boron delivery agent. This should be attributed to three factors: (1) 10B-NapE4 is a 10B-enriched species; (2) the Eriotinib targeting plays a crucial role in the selective accumulation of 10B-NapE4 in tumor cells; (3) the real-time imaging detection of 10B-NapE4 accumulation in tumor tissue allows for choosing the best irradiation time.
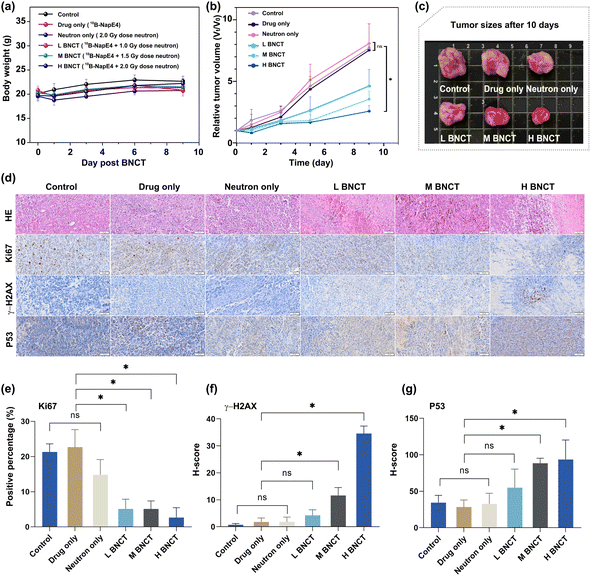 |
| Fig. 6 (a) Weight growth curves of mice after irradiation (0–9 days, L BNCT: 10B-NapE4 + neutron irradiation for 7 minutes = radioactive dose of 1.0 Gy; M BNCT: 10B-NapE4 + neutron irradiation for 10 minutes = radioactive dose of 1.5 Gy; H BNCT: 10B-NapE4 + neutron irradiation for 14 minutes = radioactive dose of 2.0 Gy). (b) Tumor growth curves (n = 6) after irradiation (0–9 days). Statistical analysis was conducted by using the one-way ANOVA multiple comparison test with Tukey's method for tumor volumes 9 days post treatment, *p < 0.05. (c) Comparison of the explanted tumor size after 10 days of irradiation. (d) Pathological and immunohistochemical results. Representative pathological images of apoptosis and proliferation in tumor tissues. Statistical analysis of (e) Ki67, (f) γ-H2AX, and (g) P53. P values = *p < 0.05; ns: no significance. Note: the control group was not exposed to neutron irradiation with no boron delivery agents applied. | |
Mechanistic investigations
We further performed histological and immunohistochemical analyses on the irradiated tumor tissues by H&E, Ki67, γ-H2AX, and P53 staining (Fig. 6d and S20†). H&E staining revealed that the cell nuclei were blurred and fragmented in the 10B-NapE4 + irradiation groups. Ki67 staining showed a low percentage of proliferating cells in the 10B-NapE4 + irradiation groups, in particular in the H BNCT group. In sharp contrast, the three comparison groups had intact cell nuclei and high percentages of Ki67-positive cells (Fig. 6d and e). γ-H2AX is a marker of DNA double-strand breakage induced by radiation. P53 protein is usually activated when DNA is damaged, which then triggers cell apoptosis.24 Here the results showed that γ-H2AX and P53 expression was significantly increased in M BNCT and H BNCT groups compared to the other groups (Fig. 6f and g). These demonstrate that 10B-NapE4 has apparent antitumor activity by inducing DNA double-strand damage upon neutron irradiation.
Conclusions
In this contribution, we demonstrate the successful molecular engineering of a new type of boron delivery agent for BNCT investigations by the “three-in-one” strategy. The three functional groups of carborane, Eriotinib and naphthalimide as the boron source, targeting unit and chromophore exhibit perfect interplay to lead to the expected AIEgens which match the high boron content of a boron delivery agent required by BNCT. AIE imaging has played an important role in finding the optimal neutron irradiation timing for achieving the best treatment and biosafety. This series of boron delivery agents show excellent targeting properties to give rise to a 10B accumulation of over 20 μg g−1 and T/N and T/B ratios 20–30 times higher than those (3
:
1) required by BNCT even at a low dose of boron delivery agents of 15 mg kg−1. For comparison, the dose of clinically used BPA for the treatment of melanoma was 600 mg kg−1 to give a 10B accumulation of 36 ± 4 μg g−1.2b Both in vitro and in vivo neutron irradiation assessments exhibit highly efficient inhibition on tumor cell growth, and no physical tissue damage and abnormal behavior in vivo. The histological and immunohistochemical analyses have verified DNA damage and the following cancer cell apoptosis. The preliminary results have offered an effective strategy for constructing multi-functional boron delivery agents, which may hold significant potential for advanced BNCT platforms.
Data availability
Data supporting this study is available in the ESI† and further details are available from the authors on reasonable request.
Author contributions
Wenli Ma designed and synthesized all the target compounds. Deshuang Tu, Changsheng Lu and Hong Yan supervised the research. Mengmeng Wang performed theoretical calculations. Qinfeng Xu, Yanyang Wang, Yilin Xue, Guoqiang Shao and Wanhua Guo completed the biological tests. Yuan-Hao Liu and Diyun Shu designed and completed neutron irradiation experiments. All authors discussed the results and revised the manuscript.
Conflicts of interest
There are no conflicts to declare.
Acknowledgements
This work was supported by the National Natural Science Foundation of China (92261202, 91961104 and 21820102004), the Ministry of Science and Technology (2021YFE0114800), the Natural Science Foundation of Jiangsu Province (BZ2022007 and SBZ2022080031), the Nanjing health science and technology development special fund project (YKK21086 and YKK21254), the High-Performance Computational Center of Nanjing University, and Henan Normal University. We are grateful to the Experimental Technologies Center of the Nanjing Institute of Geology and Palaeontology, Chinese Academy of Sciences, for ICP-MS technical assistance. All in vivo experiments were approved by the Animal Care and Use Committee and Ethics Committee of Nanjing First Hospital (DWSY-23058362). All animals were acclimatized to the environment for at least one week to reduce stress before all animal experiments.
Notes and references
-
(a)
C. Lovatt and W. Dugger, Boron, Springer, 1984, pp. 389–421, ISBN: 978-1-4684-4777-4 Search PubMed;
(b) S. Hermánek, Chem. Rev., 1992, 92, 175 CrossRef;
(c)
N. S. Hosmane, Boron Science: New Technologies and Applications, CRC, Boca Raton, 2011, ISBN: 978-1-4398-2662-1 Search PubMed;
(d)
N. S. Hosmane and R. Eagling, Handbook of Boron Chemistry in Organometallics, Catalysis, Materials and Medicine, Imperial College Press/World Scientific Publishing (UK) Ltd., 2018, ISBN: 978-1-78634-441-0 Search PubMed.
-
(a)
N. S. Hosmane, J. A. Maguire, Y. Zhu and M. Takagaki, Boron and Gadolinium Neutron Capture Therapy for Cancer Treatment, World Scientific Publishers, Singapore, 2011, Ch. 9, pp. 165–170, ISBN: 978-981-4338-67-7 Search PubMed;
(b)
W. Sauerwein, A. Wittig, R. Moss and Y. Nakagawa, Neutron Capture Therapy, Springer Heidelberg, New York Dordrecht London, 2012, ISBN: 978-3-642-31333-2 CrossRef;
(c) M. F. Hawthorne, Angew. Chem., Int. Ed., 1993, 32, 950–984 CrossRef;
(d) K. Nedunchezhian, N. Aswath, M. Thiruppathy and S. Thirugnanamurthy, J. Clin. Diagn. Res., 2016, 10, ZE01−ZE04 Search PubMed;
(e) R. Barth, Z. Zhang and T. Liu, Cancer Commun., 2018, 38, 36 Search PubMed;
(f) L. E. Farr, W. H. Sweet, J. S. Robertson, C. G. Foster, H. B. Locksley, D. L. Sutherland, M. L. Mendelsohn and E. E. Stickley, Am. J. Roentgenol., 1954, 71, 279–293 CAS;
(g) H. R. Snyder, A. J. Reedy and W. J. Lennarz, J. Am. Chem. Soc., 1958, 80, 835–838 CrossRef CAS;
(h) C. Perks, A. Mill, G. Constantine, K. Harrison and J. Gibson, Br. J. Radiol., 1988, 61, 1115–1126 CrossRef CAS PubMed;
(i) J. Goodman, J. McGregor, N. Clendenon, R. Gahbauer, R. Barth, A. Soloway and R. Fairchild, Neurosurgery, 1990, 27, 383–388 CrossRef CAS.
-
(a) M. Suzuki, Int. J. Clin. Oncol., 2020, 25, 43–50 CrossRef PubMed;
(b) R. F. Barth and J. C. Grecula, Appl. Radiat. Isot., 2020, 160, 109029 CrossRef CAS PubMed;
(c) J. Bahng, B.-S. Lee, E.-S. Kim, S. Park and H.-K. Park, Rev. Sci. Instrum., 2020, 91, 023323 CrossRef CAS PubMed.
-
R. N. Grimes, Carboranes, Elsevier, Oxford, 3rd edn, 2016, pp. 283–502, ISBN: 978-0-12-801894-1 Search PubMed.
-
(a) P. Stockmann, M. Gozzi, R. Kuhnert, M. B. Sarosi and E. Hey-Hawkins, Chem. Soc. Rev., 2019, 48, 3497 RSC;
(b) F. Issa, M. Kassiou and L. M. Rendina, Chem. Rev., 2011, 111, 5701–5722 CrossRef CAS PubMed;
(c) J. Brynda, P. Mader, V. Šícha, M. Fábry, K. Poncová, M. Bakardiev, B. Grüner, P. Cígler and P. Řezáčová, Angew. Chem., Int. Ed., 2013, 52, 13760–13763 CrossRef CAS PubMed;
(d) Y. Yin, N. Ochi, T. W. Craven, D. Baker, N. Takigawa and H. Suga, J. Am. Chem. Soc., 2019, 141, 19193–19197 CrossRef CAS PubMed;
(e) Y. Zhu, A. Peng, K. Carpenter, J. A. Maguire, N. S. Hosmane and M. Takagaki, J. Am. Chem. Soc., 2005, 127, 9875–9880 CrossRef PubMed;
(f) S. Li, Z. Wang, Y. Wei, C. Wu, S. Gao, H. Jiang, X. Zhao, H. Yan and X. Wang, Biomaterials, 2013, 34, 902–911 CrossRef CAS PubMed;
(g) R. Varkhedkar, F. Yang, R. Dontha, J. Zhang, J. Liu, B. Spingler, S. van der Veen and S. Duttwyler, ACS Cent. Sci., 2022, 8, 322–331 CrossRef CAS PubMed;
(h) C. Wu, H. Ye, W. Bai, Q. Li, D. Guo, G. Lv, H. Yan and X. M. Wang, Bioconjugate Chem., 2011, 22, 16–25 CrossRef CAS PubMed;
(i) N. Meher, K. Seo, S. Wang, A. P. Bidkar, M. Fogarty, S. Dhrona, X. Huang, R. Tang, C. Blaha, M. J. Evans, D. R. Raleigh, Y.-W. Jun, H. F. VanBrocklin, T. A. Desai, D. M. Wilson, T. Ozawa and R. R. Flavell, ACS Appl. Mater. Interfaces, 2021, 13, 54739–54752 CrossRef CAS PubMed;
(j) S. Li, C. Wu, X. Zhao, H. Jiang, H. Yan and X. M. Wang, J. Biomed. Nanotechnol., 2013, 9, 393–402 CrossRef CAS PubMed;
(k) R. Li, J. Zhang, J. Guo, Y. Xu, K. Duan, J. Zheng, H. Wan, Z. Yuan and H. Chen, Mol. Pharm., 2020, 17, 202–211 CrossRef CAS PubMed;
(l) H. Dou, W. Zhong, Y. Hou and H. Yan, Bioorg. Med. Chem., 2012, 20, 4693–4700 CrossRef CAS PubMed;
(m) P. Cígler, M. Kožíšek, P. Řezáčová, J. Brynda, Z. Otwinowski, J. Pokorná, J. Plešek, B. Grüner, L. Marešová, M. Máša, J. Sedláček, J. Bodem, H.-G. Kräusslich, V. Král and J. Konvalinka, Proc. Natl. Acad. Sci. U. S. A., 2005, 102, 15394–15399 CrossRef PubMed;
(n) C. Wu, L. Shi, Q. Li, H. Jiang, M. Selke, H. Yan and X. M. Wang, Nanomedicine, 2012, 8, 860–869 CrossRef CAS PubMed;
(o) S. Wang, C. Blaha, R. Santos, T. Huynh, T. R. Hayes, D. R. Beckford-Vera, J. E. Blecha, A. S. Hong, M. Fogarty, T. A. Hope, D. R. Raleigh, D. M. Wilson, M. J. Evans, H. F. VanBrocklin, T. Ozawa and R. R. Flavell, Mol. Pharm., 2019, 16, 3831–3841 CrossRef CAS PubMed;
(p) G. Zheng, C. Wu, H. Ye, H. Yan and X. M. Wang, J. Nanobiotechnol., 2011, 9, 1–8 CrossRef PubMed;
(q) S. Fujii, H. Masuno, Y. Taoda, A. Kano, A. Wongmayura, M. Nakabayashi, N. Ito, M. Shimizu, E. Kawachi, T. Hirano, Y. Endo, A. Tanatani and H. Kagechika, J. Am. Chem. Soc., 2011, 133, 20933–20941 CrossRef CAS PubMed.
-
(a) R. Núñez, M. Tarres, A. Ferrer-Ugalde, F. F. de Biani and F. Teixidor, Chem. Rev., 2016, 116, 14307–14378 CrossRef PubMed;
(b) J. F. Valliant, K. J. Guenther, A. S. King, P. Morel, P. Schaffer, O. O. Sogbein and K. A. Stephenson, Coord. Chem. Rev., 2002, 232, 173–230 CrossRef CAS;
(c) A. Marfavi, P. Kavianpour and L. M. Rendina, Nat. Rev. Chem., 2022, 6, 486–504 CrossRef PubMed;
(d) A. Saha, E. Oleshkevich, C. Viňas and F. Teixidor, Adv. Mater., 2017, 29, 1704238 CrossRef PubMed;
(e) J. Ochi, K. Tanaka and Y. Chujo, Angew. Chem., Int. Ed., 2023, 62, e202214397 CrossRef CAS PubMed;
(f) D. Tu, S. Cai, C. Fernandez, H. Ma, X. Wang, H. Wang, C. Ma, H. Yan, C. Lu and Z. An, Angew. Chem., Int. Ed., 2019, 58, 9129–9133 CrossRef CAS PubMed;
(g) R. Furue, T. Nishimoto, I. S. Park, J. Lee and T. Yasuda, Angew. Chem., Int. Ed., 2016, 55, 7171–7175 CrossRef CAS PubMed;
(h) D. Tu, P. Leong, S. Guo, H. Yan, C. Lu and Q. Zhao, Angew. Chem., Int. Ed., 2017, 56, 11370–11374 CrossRef CAS PubMed;
(i) J. Tong, Y. Cao, Y.-W. Zhang, P. Wang, P. Wang, X.-J. Liao, W. Zhang, Y. Wang, Y.-X. Zheng, J.-J. Zhu and Y. Pan, Angew. Chem., Int. Ed., 2022, 61, e202209438 CrossRef CAS PubMed;
(j) X. Wei, M. Zhu, Z. Cheng, M. Lee, H. Yan and C. J. Xu, Angew. Chem., Int. Ed., 2019, 58, 3162–3166 CrossRef CAS PubMed;
(k) J. Ochi, K. Tanaka and Y. Chujo, Angew. Chem., Int. Ed., 2020, 59, 9841–9855 CrossRef CAS PubMed;
(l) Y. H. Lee, J. Park, J. Lee, S. U. Lee and M. H. Lee, J. Am. Chem. Soc., 2015, 137, 8018–8021 CrossRef CAS PubMed;
(m) L. Ji, S. Riese, A. Schmiedel, M. Holzapfel, M. Fest, J. Nitsch, B. F. E. Curchod, A. Friedrich, L. Wu, H. H. Al Mamari, S. Hammer, J. Pflaum, M. A. Fox, D. J. Tozer, F. Maik, C. Lambert and T. B. Marder, Chem. Sci., 2022, 13, 5205–5219 RSC;
(n) S. Sinha, Z. Kelemen, E. Humpfner, I. Ratera, J. P. Malval, J. P. Jurado, C. Viňas, F. Teixidor and R. Núñez, Chem. Commun., 2022, 58, 4016–4019 RSC;
(o) K.-R. Wee, W.-S. Han, D. Cho, S. Kwon, C. Pac and S. O. Kang, Angew. Chem., Int. Ed., 2012, 124, 2731–2734 CrossRef;
(p) J. C. Axtell, K. O. Kirlikovali, P. I. Djurovich, D. Jung, V. T. Nguyen, B. Munekiyo, A. T. Royappa, A. L. Rheingold and A. M. Spokoyny, J. Am. Chem. Soc., 2016, 138, 15758–15765 CrossRef CAS PubMed.
-
(a) A. Barba-Bon, G. Salluce, I. Lostalé-Seijo, K. I. Assaf, A. Hennig, J. Montenegro and W. M. Nau, Nature, 2022, 603, 637–642 CrossRef CAS PubMed;
(b) C. Shi, H. Sun, X. Tang, W. Lv, H. Yan, Q. Zhao, J. Wang and W. Huang, Angew. Chem., Int. Ed., 2013, 52, 13434–13438 CrossRef CAS PubMed;
(c) M. Couto, M. F. Garcia, C. Alamon, M. Cabrera, P. Cabral, A. Merlino, F. Teixidor, H. Cerecetto and C. Viňas, Chem.–Eur. J., 2018, 24, 3122–3126 CrossRef CAS PubMed;
(d) M. Couto, I. Mastandrea, M. Cabrera, P. Cabral, F. Teixidor, H. Cerecetto and C. Viňas, Chem.–Eur. J., 2017, 23, 9233–9238 CrossRef CAS PubMed;
(e) M. Scholz, A. L. Blobaum, L. J. Marnett and E. Hey-Hawkins, Bioorg. Med. Chem., 2011, 19, 3242–3248 CrossRef CAS PubMed;
(f) C. Shi, D. Tu, Q. Yu, H. Liang, Y. Liu, Z. Li, H. Yan, Q. Zhao and W. Huang, Chem.–Eur. J., 2014, 20, 16550–16557 CrossRef CAS PubMed;
(g) D. J. Worm, S. Els-Heindl, M. Kellert, R. Kuhnert, S. Saretz, J. Koebberling, B. Riedl, E. Hey-Hawkins and A. G. Beck-Sickinger, J. Pept. Sci., 2018, 24, e3119 CrossRef PubMed;
(h) L. Zhu, X. Tang, Q. Yu, W. Lv, H. Yan, Q. Zhao and W. Huang, Chem.–Eur. J., 2015, 21, 4721–4730 CrossRef CAS PubMed;
(i) X. Li, X. Tong, Y. Yin, H. Yan, C. Lu, W. Huang and Q. Zhao, Chem. Sci., 2017, 8, 5930–5940 RSC;
(j) L. Zhu, W. Lv, S. Liu, S. Li, H. Yan, Q. Zhao and W. Huang, Chem. Commun., 2013, 49, 10638–10640 RSC;
(k) J. Wang, L. Chen, J. Ye, Z. Li, H. Jiang, H. Yan, M. Y. Stogniy, I. B. Sivaev, V. I. Bregadze and X. Wang, Biomacromolecules, 2017, 18, 1466–1472 CrossRef CAS PubMed;
(l) C. Shi, H. Sun, Q. Jiang, Q. Zhao, J. Wang, W. Huang and H. Yan, Chem. Commun., 2013, 49, 4746–4748 RSC.
-
(a) M. F. Hawthorne, J. I. Zink, J. M. Skelton, M. J. Bayer, C. Liu, E. Livshits, R. Baer and D. Neuhauser, Science, 2004, 303, 1849–1851 CrossRef CAS PubMed;
(b) A. M. Spokoyny, C. W. Machan, D. J. Clingerman, M. S. Rosen, M. J. Wiester, R. D. Kennedy, C. L. Stern, A. A. Sarjeant and C. A. Mirkin, Nat. Chem., 2011, 3, 590–596 CrossRef CAS PubMed;
(c) H. S. Ban and H. Nakamura, Chem. Rev., 2015, 15, 616–635 CAS;
(d) M. Scholz and E. Hey-Hawkins, Chem. Rev., 2011, 111, 7035–7062 CrossRef CAS PubMed;
(e) Y. Liu, W. Dong, Z. H. Li and H. Wang, Chem, 2021, 7, 1843–1851 CrossRef CAS;
(f) A. M. Spokoyny, T. C. Li, O. K. Farha, C. W. Machan, C. She, C. L. Stern, T. J. Marks, J. T. Hupp and C. A. Mirkin, Angew. Chem., Int. Ed., 2010, 49, 5339–5343 CrossRef CAS PubMed;
(g) Z. Wang, X. Gou, Q. Shi, K. Liu, X. Chang, G. Wang, W. Xu, S. Lin, T. Liu and Y. Fang, Angew. Chem., Int. Ed., 2022, 61, e202207619 CrossRef CAS PubMed;
(h) N. V. Nghia, S. Jana, S. Sujith, J. Y. Ryu, J. Lee, S. U. Lee and M. H. Lee, Angew. Chem., Int. Ed., 2018, 57, 12483–12488 CrossRef CAS PubMed;
(i) X. Tan, X. Wang, Z. H. Li and H. Wang, J. Am. Chem. Soc., 2022, 144, 23286–23291 CrossRef CAS PubMed;
(j) K.-R. Wee, Y.-J. Cho, S. Jeong, S. Kwon, J.-D. Lee, I.-H. Suh and S. O. Kang, J. Am. Chem. Soc., 2012, 134, 17982–17990 CrossRef CAS PubMed;
(k) J. J. Schwartz, A. M. Mendoza, N. Wattanatorn, Y. Zhao, V. T. Nguyen, A. M. Spokoyny, C. A. Mirkin, T. Baše and P. S. Weiss, J. Am. Chem. Soc., 2016, 138, 5957–5967 CrossRef CAS PubMed;
(l) M. P. Grzelczak, S. P. Danks, R. C. Klipp, D. Belic, A. Zaulet, C. Kunstmann-Olsen, D. F. Bradley, T. Tsukuda, C. Viňas, F. Teixidor, J. J. Abramson and M. Brust, ACS Nano, 2017, 11, 12492–12499 CrossRef CAS PubMed;
(m) W. Li, H. Chen, M. Shen, Z. Yang, Z. Fan, J. Xiao, J. Chen, H. Zhang, Z. Wang and X. Wang, Small, 2022, 18, 2108055 CrossRef CAS PubMed;
(n) Y. Xu, Y. Yang, Y. Liu, Z. H. Li and H. Wang, Nat. Catal., 2023, 6, 16–22 CrossRef CAS.
-
(a) A. G. Beck-Sickinger, D. P. Becker, O. Chepurna, B. Das, S. Flieger, E. Hey-Hawkins, N. S. Hosmane, S. S. Jalisatgi, H. Nakamura, R. Patil, M. d. G. H. Vicente and C. Viñas, Cancer Biother. Radiopharm., 2023, 38, 160–172 CrossRef PubMed;
(b) K. Hu, Z. Yang, L. Zhang, L. Xie, L. Wang, H. Xu, L. Josephson, S. H. Liang and M.-R. Zhang, Coord. Chem. Rev., 2020, 405, 213139 CrossRef CAS;
(c) Y. Shi, Q. Fu, J. Li, H. Liu, Z. Zhang, T. Liu and Z. Liu, ACS Appl. Mater. Interfaces, 2020, 12, 55564–55573 CrossRef CAS PubMed;
(d) Y. Shi, J. Li, Z. Zhang, D. Duan, Z. Zhang, H. Liu, T. Liu and Z. Liu, ACS Appl. Mater. Interfaces, 2018, 10, 43387–43395 CrossRef CAS PubMed;
(e) J. Chen, Q. Dai, Q. Yang, X. Bao, Y. Zhou, H. Zhong, L. Wu, T. Wang, Z. Zhang, Y. Lu, Z. Zhang, M. Lin, M. Han and Q. Wei, J. Nanobiotechnol., 2022, 20, 102 CrossRef CAS PubMed.
-
(a) Y. Wang, J. Nie, W. Fang, L. Yang, Q. Hu, Z. Wang, J. Z. Sun and B. Z. Tang, Chem. Rev., 2020, 120, 4534–4577 CrossRef CAS PubMed;
(b) J. Mei, N. L. C. Leung, R. T. K. Kwok, J. W. Y. Lam and B. Z. Tang, Chem. Rev., 2015, 115, 11718–11940 CrossRef CAS PubMed;
(c) Z. Zhao, H. Zhang, J. Lam and B. Z. Tang, Angew. Chem., Int. Ed., 2020, 59, 9888–9907 CrossRef CAS PubMed;
(d) X. Cai and B. Liu, Angew. Chem., Int. Ed., 2020, 59, 9868–9886 CrossRef CAS PubMed;
(e) B. Liu and B. Z. Tang, Angew. Chem., Int. Ed., 2020, 59, 9788–9789 CrossRef CAS PubMed;
(f) S. Xu, Y. Duan and B. Liu, Adv. Mater., 2020, 32, 1903530 CrossRef CAS PubMed;
(g) B. Li, C. Liu, W. Pan, J. She, J. Guo, T. Lun, J. Feng, B. Situ, T. An, Y. Zhang and L. Zheng, Biosens. Bioelectron., 2020, 168, 112520 CrossRef CAS PubMed.
-
(a) K. Wang, H. Gao, Y. Zhang, H. Yan, J. Si, X. Mi, S. Xia, X. Feng, D. Liu, D. Kong, T. Wang and D. Ding, Adv. Mater., 2021, 34, 2106994 CrossRef PubMed;
(b) J. Qi, C. Chen, D. Ding and B. Z. Tang, Adv. Healthcare Mater., 2018, 7, 1800477 CrossRef PubMed;
(c) Y. Wu, J. Li, Z. Shen, D. Wang, R. Dong, J. Zhang, Y. Pan, Y. Li, D. Wang and B. Z. Tang, Angew. Chem., Int. Ed., 2022, 61, e202212386 CrossRef CAS PubMed;
(d) J. Dai, X. Dong, Q. Wang, X. Lou, F. Xia and S. Wang, Adv. Healthcare Mater., 2021, 10, 2101036 CrossRef CAS PubMed;
(e) Y. Li, R. Tang, X. Liu, J. Gong, Z. Zhao, Z. Sheng, J. Zhang, X. Li, G. Niu, R. T. K. Kwok, W. Zheng, X. Jiang and B. Z. Tang, ACS Nano, 2020, 14, 16840–16853 CrossRef CAS PubMed;
(f) Y. Cheng, A. Clark, J. Zhou, T. He, Y. Li, R. Borum, M. Creyer, M. Xu, Z. Jin, J. Zhou, W. Yim, Z. Wu, P. Fajtová, A. J. O'Donoghue, A. Carlin and J. Jokerst, ACS Nano, 2022, 16, 12305–12317 CrossRef CAS PubMed.
-
(a) Y. Dong, B. Liu and Y. Yuan, J. Controlled Release, 2018, 290, 129–137 CrossRef CAS PubMed;
(b) D. Zhang, Y. Fan, H. Chen, S. Trépout and M.-H. Li, Angew. Chem., Int. Ed., 2019, 58, 10260–10265 CrossRef CAS PubMed;
(c) H. Jin, H. Li, Z. Zhu, J. Huang, Y. Xiao and Y. Yan, Angew, 2020, 59, 10081–11008 CrossRef CAS PubMed;
(d) M.-M. Zhang, X.-Y. Dong, Z.-Y. Wang, H.-Y. Li, S.-J. Li, X. Zhao and S.-Q. Zang, Angew. Chem., Int. Ed., 2020, 59, 10052–10058 CrossRef CAS PubMed;
(e) P. Sun, B. Han, H. Li, C. Zhang, X. Xin, J. Dou, Z. Gao and D. Sun, Angew. Chem., Int. Ed., 2022, 61, e20220018 Search PubMed;
(f) Y. Jin, Q. Peng, J. Xie, K. Li and S.-Q. Zang, Angew. Chem.,
Int. Ed., 2023, 62, e202301 Search PubMed;
(g) Y. Wang, Y. Zhang, J. Wang and X. Liang, Adv. Drug Delivery Rev., 2019, 143, 161–176 CrossRef CAS PubMed.
-
(a) C. Chen, X. Ni, H. Tian, Q. Liu, D. Guo and D. Ding, Angew. Chem., Int. Ed., 2020, 59, 10008–10012 CrossRef CAS PubMed;
(b) H. Li, Q. Yao, F. Xu, Y. Li, D. Kim, J. Chung, G. Baek, X. Wu, P. F. Hillman, E. Y. Lee, H. Ge, J. Fan, J. Wang, S. Nam, X. Peng and J. Yoon, Angew. Chem., Int. Ed., 2020, 59, 10186–10195 CrossRef CAS PubMed;
(c) L. Liu, C. Li, J. Gong, Y. Zhang, W. Ji, L. Feng, G. Jiang, J. Wang and B. Z. Tang, Angew. Chem., Int. Ed., 2023, e202307776 CAS;
(d) X. Ni, X. Zhang, X. Duan, H. Zheng, X. Xue and D. Ding, Nano Lett., 2019, 19, 318–330 CrossRef CAS PubMed;
(e) W. Wu, Y.-Q. Yang, Y. Yang, Y.-M. Yang, H. Wang, K. Zhang, L. Guo, H. Ge, J. Liu and H. Feng, Int. J. Nanomed., 2019, 14, 3571–3582 CrossRef CAS PubMed.
-
(a) M. Zhang, W. Wang, M. Mohammadniaei, T. Zheng, Q. Zhang, J. Ashley, S. Liu, Y. Sun and B. Z. Tang, Adv. Mater., 2021, 3, 2008802 CrossRef PubMed;
(b) K.-N. Wang, L.-Y. Liu, D. Mao, M.-X. Hou, C.-P. Tan, Z.-W. Mao and B. Liu, Angew. Chem., Int. Ed., 2022, 61, e20211460 Search PubMed;
(c) M. Wu, X. Liu, H. Chen, Y. Duan, J. Liu, Y. Pan and B. Liu, Angew. Chem., Int. Ed., 2021, 60, 9093–9098 CrossRef CAS PubMed;
(d) D. Zhu, Y. Duo, M. Suo, Y. Zhao, L. Xia, Z. Zheng, Y. Li and B. Z. Tang, Angew. Chem., Int. Ed., 2020, 59, 13836–13843 CrossRef CAS PubMed;
(e) M. Lee, E. Yu, D. Yan, J. Chau, Q. Wu, J. Lam, D. Ding, R. Kwok, D. Wang and B. Z. Tang, ACS Nano, 2023, 17, 17004–17020, DOI:10.1021/acsnano.3c04266;
(f) R. Xu, W. Chi, Y. Zhao, Y. Tang, X. Jing, Z. Wang, Y. Zhou, Q. Shen, J. Zhang, Z. Yang, D. Dang and L. Meng, ACS Nano, 2022, 16, 20151–20162 CrossRef CAS PubMed;
(g) X. He, B. Situ, M. Gao, S. Guan, B. He, X. Ge, S. Li, M. Tao, H. Zou, B. Z. Tang and L. Zheng, Small, 2019, 15, 1905080 CrossRef CAS PubMed;
(h) Y. Yang, L. Wang, H. Cao, Q. Li, Y. Li, M. Han, H. Wang and J. Li, Nano Lett., 2019, 19, 1821–1826 CrossRef CAS PubMed.
-
(a) C. Chen, X. Ni, S. Jia, Y. Liang, X. Wu, D. Kong and D. Ding, Adv. Mater., 2019, 31, 1904914 CrossRef CAS PubMed;
(b) D. Yan, M. Wang, Q. Wu, N. Niu, M. Li, R. Song, J. Rao, M. Kang, Z. Zhang, F. Zhou, D. Wang and B. Z. Tang, Angew. Chem., Int. Ed., 2022, 61, e202202614 CrossRef CAS PubMed;
(c) G. Ma, Z. Liu, C. Zhu, H. Chen, R. Kwok, P. Zhang, B. Z. Tang, L. Cai and P. Gong, Angew. Chem., Int. Ed., 2022, 61, e202207 Search PubMed;
(d) D. Mao, F. Hu, Z. Yi, Kenry, S. Xu, S. Yan, Z. Luo, W. Wu, Z. Wang, D. Kong, X. Liu and B. Liu, Sci. Adv., 2020, 26, eabb2712 CrossRef PubMed;
(e) S. Wang, W. Wu, P. Manghnani, S. Xu, Y. Wang, C. Goh, L. G. Ng and B. Liu, ACS Nano, 2019, 13, 3095–3105 CrossRef CAS PubMed.
-
(a) Y. Hong, W. Geng, T. Zhang, G. Gong, C. Li, C. Zheng, F. Liu, J. Qian, M. Chen and B. Z. Tang, Angew. Chem., Int. Ed., 2022, 61, e202209590 CrossRef CAS PubMed;
(b) S. Song, Y. Wang, Y. Zhao, W. Huang, F. Zhang, S. Zhu, Q. Wu, S. Fu, B. Z. Tang and D. Wang, Matter, 2022, 5, 2847–2863 CrossRef CAS;
(c) X. Fan, Q. Xia, S. Liu, Z. Zheng, Y. Zhang, T. Wu, Y. Li, G. Tang, B. Z. Tang, J. Qian and H. Lin, Mater. Today Bio, 2022, 16, 100399 CrossRef CAS PubMed.
-
(a) Z. Sun, J. Liu, Y. Li, X. Lin, Y. Chu, W. Wang, S. Huang, W. Li, J. Peng, C. Liu, L. Cai, W. Deng, C. Sun and G. Deng, Adv. Mater., 2023, 35, 2208555 CrossRef CAS PubMed;
(b) G. Wang, L. Zhou, P. Zhang, E. Zhao, L. Zhou, D. Chen, J. Sun, X. Gu, W. Yang and B. Z. Tang, Angew. Chem., Int. Ed., 2020, 59, 10122–10128 CrossRef CAS PubMed;
(c) Z. Wang, L. Yu, Y. Wang, C. Wang, Q. Mu, X. Liu, M. Yu, K.-N. Wang, G. Yao and Z. Yu, Adv. Sci., 2022, 9, 2104793 CrossRef CAS PubMed;
(d) H. Cao, H. Gao, L. Wang, Y. Cheng, X. Wu, X. Shen, H. Wang, Z. Wang, P. Zhan, J. Liu, Z. Li, D. Kong, Y. Shi, D. Ding and Y. Wang, ACS Nano, 2022, 16, 13992–14006 CrossRef CAS PubMed;
(e) J. Dai, M. Wu, Q. Wang, S. Ding, X. Dong, L. Xue, Q. Zhu, J. Zhou, F. Xia, S. Wang and Y. Hong, Natl. Sci. Rev., 2021, 8, nwab039 CrossRef CAS PubMed.
-
(a) N. Kuthala, R. Vankayala, Y.-N. Li, C.-S. Chiang and K. C. Hwang, Adv. Mater., 2017, 29, 1700850 CrossRef PubMed;
(b) L. Li, J. Li, Y. Shi, P. Du, Z. Zhang, T. Liu, R. Zhang and Z. Liu, ACS Nano, 2019, 13, 13843–13852 CrossRef CAS PubMed;
(c) J. Li, Q. Sun, C. Lu, H. Xiao, Z. Guo, D. Duan, Z. Zhang, T. Liu and Z. Liu, Nat. Commun., 2022, 13, 2143 CrossRef CAS PubMed;
(d) J. Li, J. Kong, S. Ma, J. Li, M. Mao, K. Chen, Z. Chen, J. Zhang, Y. Chang, H. Yuan, T. Liu, Z. Zhang and G. Xing, Adv. Funct. Mater., 2021, 31, 2100969 CrossRef CAS;
(e) Z. Wang, Z. Chen, Z. Zhang, J. Li, K. Chen, H. Liang, L. Lv, Y. Chang, S. Liu, W. Yang, Z. Yang, H. Yuan, X. Meng, T. Liu, F. Wang, J. Li and G. Xing, Nano Today, 2022, 45, 101558 CrossRef CAS;
(f) Y. Wang, G. Reina, H. G. Kang, X. Chen, Y. Zou, Y. Ishikawa, M. Suzuki and N. Komatsu, Small, 2022, 18, 2204044 CrossRef CAS PubMed;
(g) L. Dai, J. Liu, X. Zhao, Y. Li, S. Zhou, L. Yuan, D. Shu, L. Pan, Y.-H. Liu and Z. Qian, Adv. Funct. Mater., 2023, 2214145 CrossRef CAS;
(h) S. Scaramuzza, C. Faria, V. Coviello, D. Forrer, L. Artiglia, D. Badocco, P. Pastore, P. Ghigna, I. Postuma, L. Cansolino, C. Ferrari, S. Bortolussi, R. Vago, A. E. Spinelli, M. Bekić, M. Čolić and V. Amendola, Adv. Funct. Mater., 2023, 2303366 CrossRef CAS;
(i) Y. Zhang, H. Kang, H. Xu, H. Luo, M. Suzuki, Q. Lan, X. Chen, N. Komatsu and L. Zhao, Adv. Funct. Mater., 2023, 2301479 CrossRef CAS PubMed;
(j) T. Nomoto, Y. Inoue, Y. Yao, M. Suzuki, K. Kanamori, H. Takemoto, M. Matsui, K. Tomoda and N. Nishiyama, Sci. Adv., 2020, 6, eaaz1722 CrossRef CAS PubMed;
(k) A. Kim, M. Suzuki, Y. Matsumoto, N. Fukumitsu and Y. Nagasaki, Biomaterials, 2021, 268, 120551 CrossRef CAS PubMed;
(l) Q. Dai, Q. Yang, X. Bao, J. Chen, M. Han and Q. Wei, Mol. Pharmaceutics, 2022, 19, 363–377 CrossRef CAS PubMed;
(m) P. Coghi, J. Li, N. S. Hosmane and Y. Zhu, Med. Res. Rev., 2023, 1–22 Search PubMed;
(n) Y. Shi, Z. Guo, Q. Fu, X. Shen, Z. Zhang, W. Sun, J. Wang, J. Sun, Z. Zhang, T. Liu, Z. Gu and Z. Liu, Nat. Commun., 2023, 14, 4 CrossRef PubMed;
(o) K. Chen, S. Liu, L. Lv, J. Tong, J. Chen, H. Liang, Y. Wang, F. Hu, Q. Liu, H. Li, Z. Chen, J. Li, Z. Wang, Y. Chang, J. Li, H. Yuan, S. Fu, T. Liang and G. Xing, Nano Today, 2023, 52, 101995 CrossRef CAS;
(p) Z. Wang, R. Lei, Z. Zhang, Z. Chen, J. Zhang, M. Mao, J. Li, H. Tang, M. Li, X. Luo, J. Yang, R. Yan, Q. Liu, L. Lv, K. Chen, Y. Chang, H. Yuan, T. Liu, J. Tong, L. Zhu, T. Liang, W. Zhang, J. Li and G. Xing, ACS Appl. Mater. Interfaces, 2024, 16, 3232–3242 CrossRef CAS PubMed.
-
(a) S. M. Thomasa and J. R. Grandis, Cancer Treat. Rev., 2004, 30, 255–268 CrossRef PubMed;
(b) O. Chahrour, D. Cairns and Z. Omran, Mini-Rev. Med. Chem., 2012, 12, 399–411 CrossRef CAS PubMed.
-
(a) M. Couto, C. Alamón, S. Nievas, M. Perona, M. A. Dagrosa, F. Teixidor, P. Cabral, C. Viñas and H. Cerecetto, Chem.–Eur. J., 2020, 26, 14335–14340 CrossRef CAS PubMed;
(b) R. R. Cheruku, J. Cacaccio, F. A. Durrani, W. A. Tabaczynski, R. Watson, K. Siters, J. R. Missert, E. C. Tracy, M. Dukh, K. Guru, R. C. Koya, P. Kalinski, H. Baumann and R. K. Pandey, J. Med. Chem., 2021, 64, 741–767 CrossRef CAS PubMed;
(c) C. Alamón, B. Dávila, M. F. García, S. Nievas, M. A. Dagrosa, S. Thorp, M. Kovacs, E. Trias, R. Faccio, M. Gabay, N. Zeineh, A. Weizman, F. Teixidor, C. Viñas, M. Gavish, H. Cerecetto and M. Couto, Mol. Pharmaceutics, 2023, 20, 2702–2713 CrossRef PubMed;
(d) C. Yewale, D. Baradia, I. Vhora, S. Patil and A. Misra, Biomaterials, 2013, 34, 8690–8707 CrossRef CAS PubMed;
(e) P. Sidaway, Nat. Rev. Clin. Oncol., 2018, 15, 654 Search PubMed;
(f) B. Mandal, P. Balabathula, N. Mittal, G. C. Wood and H. Bhattacharjee, J. Fluoresc., 2012, 22, 1425–1429 CrossRef CAS PubMed.
-
(a) S. Banerjee, E. B. Veale, C. M. Phelan, S. A. Murphy, G. M. Tocci, L. J. Gillespie, D. O. Frimannsson, J. M. Kelly and T. Gunnlaugsson, Chem. Soc. Rev., 2013, 42, 1601–1618 RSC;
(b) Z. Zhu, D. Tian, P. Gao, K. Wang, Y. Li, X. Shu, J. Zhu and Q. Zhao, J. Am. Chem. Soc., 2018, 140, 17484–17491 CrossRef CAS PubMed;
(c) B. Lozano-Torres, I. Galiana, M. Rovira, E. Garrido, S. Chaib, A. Bernardos, D. Muñoz-Espín, M. Serrano, R. Martínez-Máñez and F. Sancenón, J. Am. Chem. Soc., 2017, 139, 8808–8811 CrossRef CAS PubMed;
(d) N. I. Georgiev and V. B. Bojinov, J. Lumin., 2012, 132, 2235–2241 CrossRef CAS.
-
(a) N. Nakashima, M. Murakawa and N. Mataga, Bull. Chem. Soc. Jpn., 1976, 49, 854–858 CrossRef CAS;
(b) D. Tu, P. Leong, Z. Li, R. Hu, C. Shi, K. Y. Zhang, H. Yan and Q. Zhao, Chem. Commun., 2016, 52, 12494–12497 RSC.
-
(a) T. Lu and F. Chen, J. Comput. Chem., 2012, 33, 580–592 CrossRef CAS PubMed;
(b) T. Lu and F. Chen, J. Phys. Chem. A, 2013, 117, 3100–3108 CrossRef CAS PubMed.
-
(a) L. A.-O. Widjaja, R. A. Werner, E. Krischke, H. Christiansen, F. M. Bengel, N. Bogdanova and T. Derlin, Eur. J. Nucl. Med. Mol. Imaging, 2023, 50, 602–612 CrossRef CAS PubMed;
(b) P. L. Vaddavalli and B. Schumacher, Trends Genet., 2022, 38, 598–612 CrossRef CAS PubMed.
|
This journal is © The Royal Society of Chemistry 2024 |