DOI:
10.1039/D3SC06122A
(Review Article)
Chem. Sci., 2024,
15, 4709-4722
Unlocking the potential of nanoscale sulfur in sustainable agriculture
Received
15th November 2023
, Accepted 27th February 2024
First published on 1st March 2024
Abstract
The global population is growing rapidly, which poses a significant challenge to food security. Innovation in agricultural technologies is necessary to achieve sustainable development in agriculture and combat food insecurity. Nanotechnology has emerged as a promising tool in agriculture; compared to conventional agricultural chemicals, demonstrated benefits include increased efficiency of delivery and utilization of both nutrients and pesticides, as well as nanoscale-specific stimulation of stress tolerance pathways. Among the many studied nanomaterials, nano-sulfur has demonstrated superior effects at enhancing plant resilience to pathogens and abiotic stresses, as well as improving plant growth and nutritional quality of edible tissues. A number of published studies have investigated the physiological effects (growth promotion, disease resistance) of single or several sulfur and sulfide compounds on crop species. However, there is no systematic analysis of this literature, including the effects and specific mechanisms of various sulfur forms in agricultural applications. In this review, we will discuss the effects of sulfur (including nano-sulfur) on crop species, the underlying mechanisms of action for their transport and transformation in the soil-plant system, and evaluate their suitability in sustainable agricultural development. Additionally, we discuss the current challenges and knowledge gaps for nanoscale sulfur use in agriculture, and describe future research directions to advance our understanding of the sustainable use of this material at the scale of individual fields.
1 Introduction
Food insecurity has become a pressing concern due to our continuously increasing global population. Data released by the Food and Agriculture Organization of the United Nations (FAO) shows that the global per capita area of arable land declined from about 0.45 hectares per capita in 1961 to 0.21 in 2016. This demonstrates that from a global perspective, the agricultural sector is grappling with the challenge of inadequate cultivated land area for production of food, fibres and fuels. Thus, efforts to increase per area production are a critical need to combat food insecurity in the coming years.1 However, further complicating these efforts are the increasing challenges posed by a changing climate, resulting in increased environmental stress and the likely need to move agriculture onto more marginal lands.2 For example, drought stands out as one of the most severe and impactful abiotic factors impeding plant productivity worldwide.3 Additionally, biological stresses such as pests and diseases pose significant threats to food security.4,5 Both of these factors will worsen as the climate continues to change.6 The widespread and inadequate use of agricultural chemicals is another shortcoming of conventional agriculture, with excessive chemical fertilizers and pesticides contaminating soil, water bodies, and the atmosphere, posing a significant threat to environmental and public health. Consequently, there is an urgent need for sustainable and environmentally friendly solutions to address these multifaceted issues.7
Sulfur is a valuable commodity and the primary precursor of sulfuric acid, which is considered an essential chemical for the global economy.8 Besides, sulfur plays an important role in agriculture, it is an essential macronutrient for plants as it is an important component of most plant proteins.9 As nanotechnology continues to evolve, nanomaterials are demonstrating greater efficiencies in delivery and utilization than traditional agrochemicals, while reducing the environmental hazards associated with chemicals.10 Nanoparticles are particles with sizes between 1–100 nm.11 In agriculture, nano-sulfur mainly refers to elemental sulfur (S) and sulfide compounds (e.g., CuS, ZnS) with a particle size of 1–100 nm, but also includes materials after modification via coating and loading. Elemental sulfur can be synthesised to nanoscale by physical, chemical and biological methods.12 Among the physical methods, a top-down approach is often used, in which elemental sulfur is powdered and ground in a high-energy ball mill.13 In addition to this, methods and techniques such as reverse microemulsion,14 electrochemical methods,15 water-in-microemulsion systems,16 ultrasonic methods,13etc., have been used to synthesise nanoparticles of desired size and shape. Nano-sized sulfide such as ZnS can be synthesized by liquid phase synthesis methods.17 It could be also synthesized using biosynthesis. For example, Hosseini et al. treated F. oxysporum cells or their secretions with copper sulfate solution for the biosynthesis of copper sulfide nanoparticles.18
Nano-sulfur has generated interest due to its antibacterial19 and insecticidal properties.20 Cao et al. showed that sulfur nanoparticles of 30 nm and 100 nm were effective in controlling Fusarium wilt compared to the application of bulk sulfur granules and sulfate.21 Research has shown that nano-sulfur acts as a plant growth promoter, enhancing plant stress resistance, and improving the nutritional quality of plants.22 Moreover, nano-sulfur can also reduce the absorption of toxic metals,23,24 minimizing contamination of the food chain, and enhancing food safety and security.25,26 While nano-sulfur holds great promise for promoting sustainable and eco-friendly farming practices, mechanistic investigations to support its widescale application remain somewhat limited. The likely multifunctional mechanisms by which nano-sulfur uniquely impacts plants, the long-term fate of these materials in soil-plant systems, and associated environmental costs and benefits remain largely unknown. However, given highly promising data that exists, as well as the magnitude of the problem we face with regard to food security in a changing climate, research in this area is critically needed.
2 Role of sulfur in agriculture
Sulfur is an essential macroelement for plants, playing a vital role in plant growth, development, and response to environmental factors. It is involved in various plant processes, including the synthesis of amino acids, chloroplasts, cell membrane structure, enzymes, and coenzymes. Moreover, sulfur actively participates in redox processes and plays a central role in the glutathione biosynthesis pathway,27 thereby influencing plant growth, metabolism, heavy metals detoxification, disease resistance, stress resistance, and nutritional quality.28–31
Sulfur has been used for centuries to control pests and diseases in agriculture and remains the most widely used pesticide in terms of volume.32 According to the California Department of Pesticide Regulation (CDPR), sulfur continues to be the most used chemical in the top 100 chemicals by pounds in total statewide pesticide use in 2021, with a total application of approximately 18 million kg.33 Sulfur has been used as an insecticide since the Egyptians began crop cultivation, and the idea of using sulfur to control powdery mildew of fruit trees caused by a fungi was first proposed by Forsyth in 1802.34 Since then, sulfur's effectiveness in combatting agricultural threats has solidified its position as a trusted and enduring pest management solution for farmers worldwide. Sulfur has also been used as a nutritional strategy to enhance plant resistance to pathogens such as bacteria and fungi.35 Last, sulfur is also used as an insecticide and is known to disrupt the metabolism, digestive processes, and intercellular and intracellular transport of essential substances of insects (e.g. aphids, thrips, and red spiders).36
Essential plant nutrients are categorized as macronutrients and micronutrients based on the amounts required by the plant.37 Notably, research on fertilizers has historically focused on the three major nutrients essential for plant growth, nitrogen (N), phosphorus (P), and potassium (K), primarily because of the large amounts needed and the dominant role they play in providing plant nutrition. Importantly, sulfur as a macronutrient is a vital component of most proteins and plays a crucial role in supporting overall plant growth.38 Presently, sulfur fertilizers are primarily categorized as sulfur-based or sulfate-based. In addition, a variety of sulfur-containing fertilizers are commonly used, including sulfur phosphorus ammonia, gypsum ammonium nitrate, ammonium sulfate, calcium sulfate, liquid sulfur dioxide, ammonium thiosulfate, ammonium polysulfide, urea sulfuric acid, ammonium bisulfite, and urea sulfur, among others. The sulfate pathway is the main route for sulfur accumulation in plants. Therefore, any sulfur-based fertilizers require conversion into sulfate for optimal absorption and utilization.39 It is worth noting that the soil microbiome plays an important role in the sulfur cycle, participating in the processes of biological oxidation (oxidation of sulfides to sulfanes and sulfates), assimilatory reduction (reduction of sulfates to sulfur-containing organic matter), and allochthonous reduction (reduction of sulfates to sulfides).40 Therefore, soil incorporation is the most common way of applying traditional sulfur fertilisers, and readily soluble materials containing SO42−–S, such as ammonium sulfate, potassium sulfate and sodium sulfate, are considered suitable for neutral to slightly alkaline soils.41 However, SO42−–S is susceptible to leaching losses in coarse textured soils.42 In addition, there have been several studies targeting application methods such as seed dressings and seed coatings with sulfur.43
As research into the sulfur cycle continues, it is found that the burning fossil fuels led to emissions of sulfur dioxide and reactive sulfur into the atmosphere, with wide-ranging health and environmental impacts, ultimately leading to calls for the reduction of sulfur emissions.44 However, studies have shown that with the implementation of air quality regulations, there has been a reduction in atmospheric deposition of sulfur, and consequently, an increase in the use of sulfur in agriculture.45 Although there has been increasing application of various sulfur fertilizers such ammonium sulfate, gypsum, potassium sulfate, magnesium sulfate and sulfates of micronutrients, the use efficiency of sulfur is very low, as is the case with nearly all fertilizers, and this efficiency is influenced by a large number of factors, such as soil properties, soil microbial population, crop species, and environmental conditions.46 This inefficiency causes waste of an important finite natural resource and creates environmental concerns due to excessive sulfur in soil, which could acidify the soil, affect nutrient uptake, plant growth and leaching into groundwater.47 Specifically, the global use efficiency of sulfur in cereals was estimated to be only 18%, according to a report in 2019.48 This highlights the need for the development of new types of increased use efficiency of sulfur-containing fertilizers that can be tailored to sustainably address different soil and crop requirements.
3 Nano-sulfur: diverse uptake pathways in plants
While nano-sulfur has been demonstrated to enhance plant growth compared with bulk and salt forms sulfur (e.g., sulfate), the underlying mechanisms of these nanoscale phenomena remain largely unknown. A distinct advantage of nanoscale agrochemicals is the utilization of diverse uptake and distribution pathways by plants. Plants acquire sulfur primarily from soil with sulfate as the source, although they also absorb gaseous SO2 and H2S through leaf stomata. Hinckley et al. studied the fate, transformation and effects of elemental sulfur in viticulture in California, USA, and found that elemental sulfur oxidises rapidly to sulfate (SO42−) at the soil surface, which then accumulates during the growing season.49 Sulfate is taken up by plant roots through specific sulfur transporters (SULTRs) present in the root cell membranes. This uptake process is energy-dependent and regulated by various factors, including sulfur availability in the soil and the soil microbiome. Specifically, microbial species in the soil can break down sulfur-containing organic matter into hydrogen sulfide, which can be further converted to elemental sulfur or sulfate by sulfur and sulfide bacteria.50 After being absorbed by the root system, sulfate ions are transported to various parts of the plant to support growth and physiological function as demostrated in Fig. 1. Within plant cells, sulfate is reduced to sulfide (S2−) during the assimilation process and is incorporated into various biomolecules, including for the synthesis of essential sulfur-containing compounds such as cysteine, methionine, and glutathione. Cysteine plays essential roles in enzyme catalysis, transcriptional regulation, and protein folding.51 Methionine affects the synthesis of phytohormones such as cytokinins and auxins, which further affects plant growth and development.52,53 Glutathione, as a significant antioxidant, affecting plant stress tolerance by forming a redox system through its oxidation and reduction states, thereby eliminating reactive oxygen species (ROS) generated during oxidative damage and effectively enhancing plant stress tolerance.54 Glutathione and its derivatives phytochelatins (PCs) also detoxify heavy metals and metalloids via chelation and sequester them into vacuoles and thus enhanced tolerance to meta(loid)s.31,55
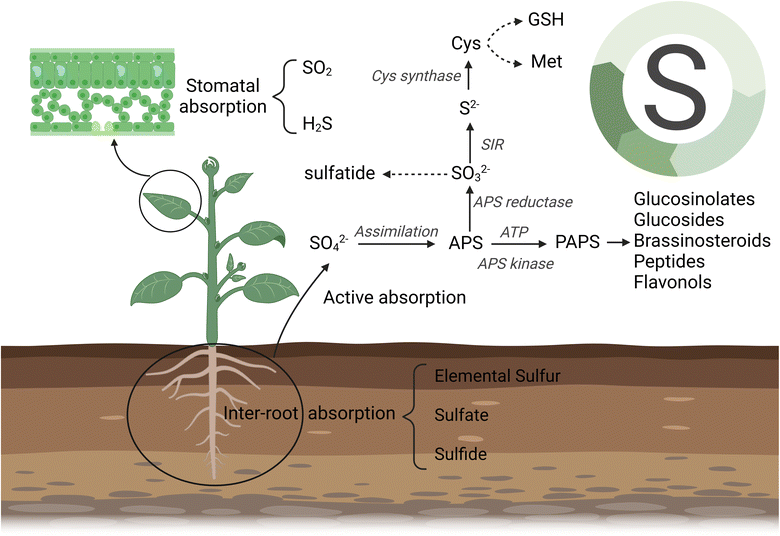 |
| Fig. 1 Absorption and transformation of different source of sulfur in plants (Created with https://www.biorender.com/. With permission.). | |
While sulfate ions need specific transporters for accumulation, nano-sulfur accesses more diverse pathways for plant uptake. Due to unique particle properties and small size, nano-sulfur may enter the root cells through additional transport pathways, including diffusion and endocytosis. Importantly, these adsorption and translocation processes are energy-independent. Once inside the plant, nano-sulfur can be transported via both the xylem and phloem. The xylem vessels carry water and nutrients from the roots to other parts of the plant, allowing nano-sulfur to move with the transpiration stream.56 The phloem vessels, responsible for transporting sugars and other compounds systemically, can facilitate the movement of nano-sulfur to different plant tissues. In addition, intracellular transport of nano-sulfur to different organelles can occur. The in planta nano-sulfur can be either directly assimilated to organic sulfur catalyzed by enzymes such as thiocyanase or thiotransferase/thiosulfate transferase (TST) in various cellular compartments or can transform and release sulfate from its surface upon reacting with cellular components to participate in various assimilation processes. The possible pathways for uptake, transport and assimilation pathways of nano-sulfur in plants are demonstrated in Fig. 2. Such diverse uptake and assimilation pathways enable significantly more efficient use of sulfur by the plant. In addition, the direct uptake and assimilation of elemental nano-sulfur was described by Wang et al.57 Importantly, the conventional sulfate ion transporter uptake and assimilation pathway leads to the formation of potentially cytotoxic SO32− and HS−/S2−,58,59 whereas the direct uptake of nano-sulfur can reduce the generation of these toxic sulfur species. Furthermore, nanoparticles are susceptible to transformations in biofluids by the attachment of biomolecules, and these transformations are often associated with the formation of a biomolecular-corona surrounding the surface of the nanomaterials.60 This biomolecular corona alters the particle size and surface properties, which in turn affects their nature, distribution, and fate in the plant. In medicine and biology, targeted drug transport is achieved by coupling antibodies, ligands, or other targeting functional groups to the surface of the nanocarrier to avoid biomolecular-corona coverage.61 In fact, in plants nano-sulfur activity is known to be similarly influenced; for example, Wang et al. demonstrated that soil application of 200 mg L−1 of stearic acid coated (1–2 wt%) nano-sulfur significantly reduced Fusarium infested tomato plants compared to the uncoated nanomaterial.57
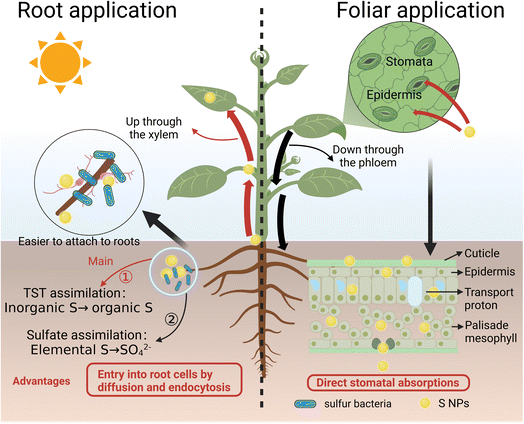 |
| Fig. 2 Diverse uptake, transport and assimilation pathways of nano-sulfur in plants (Created with https://www.biorender.com/. With permission.). | |
Another advantage of nano-sulfur is its ability to avoid immobilization in the soil and to attach to the root surface while reacting with soil microorganisms and inter-root secretions. This allows for direct and sustained release of sulfur into the root system, where it is converted to sulfate by microbes for uptake by the plant.62 Studies on the effects of elemental sulfur and nano-sulfur bio-organic phosphate composites on maize growth demonstrated that nano-sulfur distributed evenly in the soil and around the roots, reducing soil pH and increasing nutrient bioavailability, thereby promoting plant growth.63 Subramanian et al. reported that the total dry matter production (DMP) of sunflower treated with 40 kg per ha nano-sulfur (86.1 and 88.7 g per plant) was significantly higher than that of sunflower treated with 20 kg per ha nano-sulfur (76.3 and 78.1 g per plant) and gypsum (S 40 kg ha−1) (61.3 g per plant) in the absence and presence of sulfur oxidizing bacterial (SOB) inoculum.12 The significant increase in DMP was attributed to the slow release of sulfur. In addition, the sulfate release time of the soil applied with a low dose of 20 kg ha−1 of nano-sulfur was still 7 days longer than that of gypsum fertilized soil.12
In short, the diverse uptake and assimilation pathways of nano-sulfur in plants can significantly enhance the use efficiency, subsequently improving plant growth. However, the full picture of the transport of nano-sulfur from soil to root and adsorption, uptake, transport and assimilation remains unclear, as does the potential tuning of synthesis and surface chemistry that could be performed to optimize benefit. This highlights the need for further studies to elucidate these processes and the underlying mechanisms, which is the key to understanding and fully taking advantage of the biological effects of nano-sulfur.
4 Mechanisms of nano-sulfur beneficial impact on plants
The positive effects of nano-sulfur on plants have been reported for both as fertilizer and pesticide (Table 1). The mechanisms underlying these impacts include enhancement of essential biological processes in plants such as photosynthesis and nutrient absorption, as well as increased resilience to stress by modulating antioxidative pathways or phytohormones.63–65 Such impacts are likely driven by altered activity at the genetic, protein or metabolite level. In terms of application as a pesticide, nano-sulfur can damage bacteria and fungi through multiple antimicrobial mechanisms. For example, the ions dissolved by NPs enter into the bacteria after direct contact with them to destroy their structure and induce apoptosis. Alternatively, NPs can induce the production of ROS, which can damage the internal structure of bacteria, such as DNA damage, DNA replication inhibition, protein denaturation and enzyme destruction.66 Some of these mechanisms have been reported while others require further investigation.
Table 1 Select publications on nano-sulfur application in agriculture
Crop type |
Concentration |
Application methods |
Size (nm) |
Results |
References |
Sunflower |
20,40 kg ha−1 |
Soil application |
35–45 |
The sulfur release of nano-sulfur is extended by 7 days compared to normal sulfur fertilizer (gypsum) |
12
|
Bread wheat |
1 mg L−1 |
Soil application |
20 |
Nano-sulfur applications in bread wheat have had positive effects on all the examined traits. SA results in the highest field germination rate (100%) and the fastest emergence rate. In most of the traits, the SA has the highest trait values; especially grain yield and protein ratio properties |
64
|
Tomato |
100–600 mg L−1 |
Soil application |
5–80 |
Increasing the concentration of nano-sulfur from 100 ppm to 300 ppm caused an increase in root and shoot length, while higher concentrations of 400 ppm to 600 ppm caused an inhibitory effect |
65
|
Cucumber |
100–600 mg L−1 |
Seed germination |
5–80 |
The increase in nano-sulfur concentration (100–600 ppm) significantly enhanced cucumber seed germination 15–25% |
67
|
Tomato |
200 mg L−1 |
Soil application |
38–65 |
Disease reduced the plant biomass by up to 87%, but nano-sulfur significantly reduced disease as determined by area-under-the-disease-progress curve by 54% |
68
|
Tomato |
200 mg L−1 |
Soil application |
65; stearic acid coated |
By day 16, the benefit of bulk sulfur was lost, but nano-sulfur increased shoot biomass by 264% and root biomass by 200% |
57
|
Wheat |
50, 100, 200 mg L−1 |
Foliar applications |
— |
The nano-sulfur (NS) and nano-sulfur coated with stearic acid (NS SA) and sulfate showed a significant increase in chlorophyll 6.3–10.7%), dry biomass (7.9–14.6%), Fv/FM (2.8–3.0%) whereas the bulk sulfur was less effective |
69
|
Maize |
12, 24 and 36 kg ha−1 |
Soil application |
20 |
The treatment of nano-sulfur 15 kg/fed increased the available contents of N, P, K, Fe, Mn and Zn in the soil by 17%, 29%, 20%, 24%, 27% and 29% respectively, compared to the control group |
70
|
Tomato |
30–100 mg L−1 |
Foliar applications |
30, 100 |
Foliar application of 1 mg/plant of 30 nm nano-sulfur for 10 weeks reduced disease incidence by 47.6% and increased aboveground biomass of tomato by 55.6%, while upregulating the expression of pathogenesis-related and antioxidant enzyme-related genes (11–352%) and enhancing the activity and content of disease-related biomolecules (5–49%) |
21
|
4.1. Enhanced photosynthesis
Nano-sulfur has been found to enhance photosynthesis by increasing the activity of photosynthetic enzymes and improving the efficiency of light absorption by chloroplasts.71 Nano-sulfur can also increase the transfer rate of electrons in the electron transport chain, promoting the formation of ATP and NADPH. For instance, Smith et al. investigated the effect of nano-sulfur on wheat growth and showed that photosynthetic parameters, including SPAD (chlorophyll content index, 6.3–10.7%), and Fv/Fm (maximum quantum efficiency of photosystem II, 2.8–3.0%), were significantly increased in plants foliar sprayed with three concentrations (50, 100, and 200 ppm) of nano-sulfur compared to the bulk sulfur control group.69 This enhancement was attributed to the large specific surface area and quantum size effect of nano-sulfur, allowing for effective light absorption in visible and ultraviolet wavelength regions.72 Nano-sulfur can be effectively adsorbed by plant cells by diffusion or endocytosis, and importantly, direct entry of nanoparticles in chloroplast is possible.73 Once in cell, the expanded spectrum of plant photosynthesis enables the collection of more electrons that can drive photosynthesis, leading to improved activation of photosynthetic pigments. Additionally, nanoparticles can convert ultraviolet and near-infrared light into visible light.74 For example, Yb, Nd, and Er-doped up-conversion nanoparticles (UCNPs) can convert near-infrared light excited at the wavelengths of 808 and 980 nm into visible light at 510–570 nm and 640–700 nm.75 Similarly, polyethyleneimine (PEI)-capped down-converting nanophosphors β-NaYF4:Gd3+,Tb3+@PEI can convert ultraviolet light (273 nm) to visible light (480–630 nm).76 Thus, introducing these nanoparticles into plants could potentially expand the light spectrum available for photosynthesis. In addition, sulfur containing nanomaterials able to capture and donate electrons could be intentionally loaded into chloroplasts to increase flow through the electron transport chain. Both approaches have the potential to improve the efficiency of photosynthesis, especially under light-insufficient conditions.77 Consequently, this enhances the light energy conversion efficiency and the overall generation of photosynthetic energy.77
Nano-sulfur may also enhance photosynthesis by promoting the activity of key enzymes involved in the Calvin cycle and carbon assimilation. Specifically, enzymes involved in carboxylation or oxygenation, such as Rubisco nuclease and Rubisco activase, play a crucial role in catalyzing Calvin cycle reactions and fixing CO2 during the light independent reactions.78 Other intrinsic photocatalytic nanomaterials such as nano-anatase TiO2 can enhance the activity of Rubisco,.79 Also, nano-sulfur may enhance photosynthetic enzymes through different mechanisms. For example, Giordano et al.80 observed a strong reduction of photosynthesis in green algae under sulfur-limited growth conditions and correlated this with a substantial decrease of Rubisco and chlorophyll a/b-binding proteins. Sulfur is a critical component of Rubisco and at the onset of sulfur limitation, Rubisco may be metabolized as an intracellular source of bioavailable S. Thus, direct involvement of supplemented sulfur in the synthesis of Rubisco will enhance photosynthetic activity. In addition, nano-sulfur can release sulfur ions in a controlled and sustained manner that ensures a steady supply of the nutrient over an extended period, optimizing its availability during critical stages of growth and photosynthesis. Maderi et al. evaluated the release patterns of nutrients from pure ammonium sulfate and surface-modified sulfur nano-zeolites, and showed that pure ammonium sulfate depleted all available sulfate within 384 h, whereas sulfate-loaded surface-modified nano-zeolites continued to release SO42− at concentrations ranging from 47.56 to 8.27 μg g−1 after 912 h. The release of SO42− from surface-modified nano-zeolites was also shown to be a major factor in the release of other nutrients from the surface-modified sulfur nano-zeolites.81 These findings clearly demonstrate that nano-sulfur can provide plants with nutrients for a significantly longer period of time than traditional sulfur fertilizers. Additionally, nano-sulfur possess inherent antioxidant properties,21 facilitating the quenching or capture of reactive oxygen species (ROS), which are not only the natural by-products of photosynthesis but also the physiological response to biotic and abiotic stress; this antioxidant activity offers direct protection to sensitive cellular components, including chloroplasts and photosynthetic pigments. Importantly, much of this activity could likely be enhanced by tuning the chemistry and properties of nano-sulfur, enabling targeted spatial and temporal activity that benefits the plant at a tissue, cellular, or organelle level. Importantly, understanding the mechanisms underlying this potential enhanced activity need to be further elucidated.
4.2. Enhance inorganic nutrient absorption
Nano-sulfur has been shown to indirectly enhance the absorption of inorganic nutrients from soil by plants through several mechanisms. First, it positively impacts root growth; for example, tomato roots grown in soil with 300 ppm nano-sulfur application were more robust, with root length and dry weight being increased by 126.8% and 133.3%, respectively, compared to the untreated control group.65 This treatment significantly increased the surface area and number of root hairs, expanding the absorption capacity for other nutrients. Nano-sulfur's small size facilitates greater penetration of the soil matrix and this increased mobility ensures better distribution and availability of nutrients, making them more accessible to plant roots. Subramanian et al. determined the fractions of water-soluble sulfur (WS), exchangeable sulfur (ES), occluded sulfur (Occ. S), organic sulfur (Org. S), and total sulfur (TS) in nano-sulfur-fertilized and conventionally sulfur-fertilized soils. The results showed that WS (8.3 ppm), ES (4.4 ppm) and TS (249.4 ppm) were greater in the nano-sulfur fertilized soil (sulfate-loaded surface-modified nano zeolite) at 40 kg S ha−1; values in the conventional treatment were WS (7.4 ppm), ES (3.9 ppm), and TS (248.6).82 These data clearly demonstrate that nano-sulfur facilitates greater availability (WS and ES), while conventional-S contributes to increased occluded S. Last, nano-sulfur may increase the cation exchange capacity of the soil, creating additional binding sites for cations, and facilitate the retention and uptake of essential inorganic nutrients by plant roots.57
Secondly, sulfur enhances the metabolic activity of soil microorganisms and stabilizes the microbial community structure, leading to an increased decomposition rate of organic matter and nutrient release in the soil, and nano-sulfur has more capability than conventional sulfur in these areas. Specific bacterial groups, including Thiobacillus83 and β-proteobacteria,84 are responsible for the oxidation of elemental sulfur in soil. Sulfur cycling bacteria play a crucial role in the absorption and transformation of sulfur by plants. Karimi et al.85 demonstrated a negative correlation between the amount of applied sulfur and soil pH, indicating successful oxidation of elemental sulfur. El-Hamdi et al.86 found that the decrease in soil pH following the application of elemental sulfur was attributed to microorganisms in the soil, particularly sulfide bacteria, which oxidize elemental sulfur into sulfuric acid. Similarly, Esmaeil et al.70 proposed that nano-sulfur, being an economical, effective, and readily available soil amendment, can be oxidized by various soil microorganisms to form sulfuric acid. This acid reacts with calcium carbonate (CaCO3) in the soil, forming calcium sulfate (CaSO4) that can ionize into Ca2+ and SO42−. The presence of Ca2+ enhances soil aggregation and permeability, reduces soil pH, promotes the release of available nutrients, and increases nutrient solubility and bioavailability for plant uptake. Etesami et al. showed that sulfur oxidation by sulfur bacillus lowers soil pH and promotes phosphate solubilization in the soil, resulting in an increase in plant-available phosphorus.87 Nadeem et al. also showed that elemental sulfur application produces soil acidification which can improve Fe and Mn availability and also facilitate the conversion of more organic-P to inorganic-P.88 Similarly, studies on sugarcane in Florida, USA by Orem et al. have shown that phosphorus release can be modulated by the application of elemental sulfur.89,90 The authors reported that due to the increase in plant available nutrients, plant growth was improved.91 In addition, soil pH significantly influences the availability of micronutrients to plants,92 thereby impacting the absorption of inorganic nutrients from the soil. In addition, decreased pH will also increase the availability of toxic heavy metals but that the greater precision and tunability offered by nano-sulfur can be used to maximize the benefit (increased availability of nutrients) and minimize the risk (increased toxic metal availability).
4.3. Regulation of gene expression
Nano-sulfur regulates the expression of a number of genes and gene pathways in plants, leading to changes in growth and stress tolerance. Nano-sulfur may promote activation of the plant immune system and improve plant resistance to pathogenic and pest species. Cao et al. used hierarchical clustering and PCA analysis to study the expression of antioxidant and pathogenesis-related genes in tomato shoots and roots after nano-sulfur treatment. Proteins with antifungal activity are regulated by PR1, PR-P2, STH2, alkaline β-1,3-glucanase (GluB), alkaline chitinase (CHI9) and PR5-like genes, while genes related to phytotoxin accelerator and PR1 protein biosynthesis include WRKY33, GAME1, GAME4 and NPR1.93 The authors report that while conventional sulfate treatment did not significantly differ from infected controls, nano-sulfur significantly upregulated the expression of PR1, GluB, CHI9, WRKY33, NPR1, GAME1 and GAME4 in tomato buds and roots. Nano-sulfur affects the synthesis of antifungal proteins by regulating gene expression and enhancing the antibacterial activity of plants.21 Similarly, Wang et al.68 reported that after 8 days of pristine nano-sulfur (nS) and stearic acid coated nano-sulfur (cS) treatment, the expression of WRKY6 in infected tomatoes was significantly up-regulated by 280–365% and 212–282% compared with the healthy control and bulk sulfur treatment. WRKY6 encodes a plant resistance protein that provides immunity by recognizing F. oxysporum f.sp. lycopersici effectors,94 indicating that both nS and cS enhanced plant immunity by regulating gene transcription. These findings suggest that nano-sulfur can regulate the expression of genes related to antifungal activity and pathogen-related proteins, sulfur as well as metabolic pathways for sulfur, chlorophyll and other key genes in plants,95 all of which can significantly increase plant growth, stress response and immune system activation.
Importantly, this observed regulation may also be caused by epigenetic modifications,96 such as alteration of DNA methylation and histone modifications, thereby affecting how genes are turned on or off, subsequently modulating the expression of genes involved in plant growth and development. For example, it has been shown that cadmium telluride quantum dots and silica NPs affect the global DNA methylation pattern, modulate DNA methyltransferase activity, methyl-CpG-binding domain (MBD) protein expression, and/or alter posttranslational modifications of histone proteins;97 similar activity with nano-sulfur may be possible. The altered genetic activity may be also caused by transcription factor regulation, with nano-sulfur directly binding to these proteins to affect their activity. During transcription, regulation is primarily achieved by suppressing the selective and obligatory affinity of RNA polymerases for the template DNA. Regulation can also occur through increasing the number of DNA supercoils and thus, decreasing the amount of exposed template DNA.98 Further studies are required to fully explore these potential mechanisms.
4.4. Antimicrobial activity
Elemental sulfur (bulk sulfur) has a long history of use as an antimicrobial agent. The main direct mechanism of action for the antimicrobial effect of elemental sulfur is absorption into the cell by the pathogen, with enzymatic reduction to H2S.99 This subsequently affects the synthesis of many essential enzymes in the cell and important intermediate metabolic processes occurring in the mitochondria, effectively inhibiting pathogen activity.100,101
Similarly, nano-sulfur has been shown to exhibit antimicrobial activity against a range of plant pathogens, such as fungi and bacteria, which can help to reduce the need for synthetic pesticides. Importantly, several studies have shown that nano-sulfur exhibits greater antimicrobial efficacy than the bulk equivalent. Roy Choudhury et al. showed that serially diluted (2-fold) concentrations of nano-sulfur exhibited antibacterial activity against select Gram-positive and Gram-negative bacteria, whereas analogous serially diluted concentrations of elemental sulfur failed to exhibit any inhibitory effect on bacterial growth.102 Roy Choudhury et al. showed that compared with elemental sulfur, nano-sulfur has a greater bactericidal effect on the facultative fungal food pathogen Aspergillus niger.103 Moreover, colloidal sulfur did not exhibit anti-bacterial and anti-fungal activity compared to nano-sulfur (10 nm).15 This finding that the antimicrobial activity of nano-sulfur is highly related to size is not surprising and has been observed with other materials, such as Ag and Cu.104,105 Nano-sulfur particles have a significantly greater surface area compared to bulk sulfur, providing more contact points for interactions with pest and pathogenic species. The enhanced surface area allows for better coverage and adhesion to the plant surface, improving the efficacy of pesticidal activity. Choudhury et al. investigated the reasons for the difference in antimicrobial effects between nano-sulfur and elemental sulfur, and showed that elemental sulfur only retarded fungal growth, whereas nano-sulfur inhibited the formation of spores, the propagules of fungi.106 Subsequently, it was shown that nano-sulfur (50 and 100 ppm) changed the phospholipid content in A. niger and F. oxysporum, thereby affecting the synthesis of nucleotides, sugars and other morphogens involved in many developmental processes. However, the contact toxicity of sulfur to fungi is not always related to its direct effect on the phospholipid content, which is a highly species-specific.103 Rao et al. also found that nano-sulfur anti-fungal activity was a function of the deposition of nanoparticles on the cell wall, subsequently compromising structural integrity.107 In addition, the antimicrobial effect of nano-sulfur is closely related to dose. Deshpande et al. evaluated the antimicrobial activity of nano-sulfur and colloidal sulfur against A. niger and A. flavus and demonstrated that nano-sulfur at higher concentrations (1500–3000 μg ml−1) inhibited the normal growth of fungal cells, while at lower concentrations (30–150 μg ml−1) fungicidal activity was lost.108
At a molecular level, the inhibitory effect of nano-sulfur on bacteria is mainly through strong interaction with target molecules such as enzymes and proteins present on the cell surface, disrupting the cell structure and possibly also binding to DNA to cause cell death. Previous studies have shown that nano-sulfur has antibacterial activity against a number of Gram-positive and Gram-negative bacteria,109 although only limited activity against Escherichia coli.110 Conversely, Shankar et al.111 prepared nano-sulfur using sodium thiosulfate and hydrochloric acid, and reported that chitosan-terminated nano-sulfur had strong antibacterial activity against E. coli and Staphylococcus aureus, effectively inhibiting the proliferation and migration of cancer cells, and had reduced cytotoxicity against healthy cells as compared to sodium thiosulfate (STS). The authors cited a potential mechanism involving nano-sulfur binding to the bacterial cell wall, leading to cell membrane cracking and cell death. Importantly, nanoscale sulfur effects will vary with cell wall type. Compared with Gram-negative bacteria, nano-sulfur will more effectively penetrate Gram-positive cells (Fig. 3). Separately, Paralikar et al.112 explored the possible mechanism of antibacterial effects of nano-sulfur. The authors reported that nano-sulfur enters bacterial cells through the lysosomal membrane and causes cell leakage. At the same time, nano-sulfur can bind to ribosomes and inhibit the translation process, as well as bind to and inactivate DNA, eventually leading to cell death.
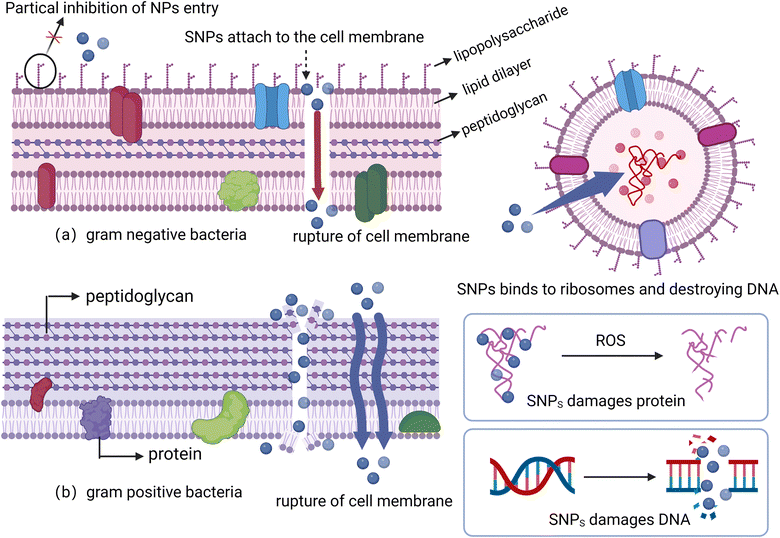 |
| Fig. 3 Antibacterial activity of sulfur nanoparticles against Gram-negative (a) and Gram-positive (b) bacteria (Created with https://www.biorender.com/. With permission.). | |
For the indirect mechanism of antimicrobial activity, nano-sulfur interacts with the plant antioxidant system and triggers plant disease resistance pathways, providing another mechanism of action to minimize the impact of plant diseases and insect pests. Pathogens, upon infecting plants, induce oxidative stress. Sulfur-containing antioxidants such as cysteine and glutathione play a crucial role in maintaining reactive oxygen species (ROS) balance during disease. Pathogen presence, as well as the application of elicitors, can activate Systemic Acquired Resistance (SAR) in plant tissues. In an experiment involving tomato infected with F. oxysporum,21 a reduction in tomato disease incidence following treatment with nano-sulfur was linked to a decrease in plant ROS accumulation and an increase in the content of the plant hormone SA (salicylic acid). Additionally, the balance between SA and ABA (abscisic acid) was altered. The anti-Fusarium activity of nano-sulfur also involves the activation of SA-dependent SAR, leading to the up-regulation of disease-related and antioxidant-related genes. This, in turn, enhances the activity of disease-related enzymes and promotes the synthesis of phytotoxins and antioxidants.
4.5. Enhance plant stress tolerance
Nano-sulfur has demonstrated the potential to improve the ability of plants to tolerate various environmental stresses, such as drought, salinity, or heavy metal toxicity, by enhancing antioxidant enzyme activity and reducing oxidative stress. For example, in a pot experiment, sunflower seeds were immersed in different concentrations (12.5, 25, 50, 100, and 200 μM) of nano-sulfur, and 100 mM MnSO4 was subsequently used to irrigate the sunflower seeds for 14 days. The authors reported that the application of nano-sulfur reduced Mn absorption and enhanced S metabolism by increasing both cysteine levels and the water content of the seedlings, minimizing drought damage by increasing in planta osmotic agents such as amino acids and proline. The authors also reported that nano-sulfur has the potential to alleviate Mn stress.113 Nano-sulfur has also been shown to promote plant nutrient accumulation and alleviate the toxicity of copper stress in Brassica napus L.; specifically, increases in superoxide dismutase (SOD), peroxidase (POD), catalase (CAT), ascorbate peroxidase (APX), glutathione reductase (GR) and glutathione S-transferase (GST) enzyme activities caused by Cu stress were mitigated in shoots (10.9–37.1%) and roots (14.6–35.3%) with nano-sulfur addition.114 Meselhy et al.26 showed that nano-sulfur can not only improve the productivity of rice, but also offset toxic metal damage by reducing As accumulation in roots, shoots, and grains. More specifically, nano-sulfur effectively reduced the bioavailability and accumulation of As in rice and promoted AsIII detoxification by regulating the expression of genes mediating As transport, S assimilation and glutathione synthesis pathways. Similarly, it has been shown that nano-sulfur application decreased Hg accumulation and increased growth of oilseed rape seedlings (Brassica napus L.) via modulation. Of antioxidant enzymes pathway and affecting the soil microbial communities when grown on mercury-contaminated soil.
Nano-sulfur has also been shown to alleviate salinity stress in plants. For example, Esmaeil et al.70 assessed the impact of nano-sulfur on soil properties and maize productivity in saline soil. The authors reported significant improvements compared to the mineral sulfur control group; the nano-sulfur treated plots exhibited increased hydraulic conductivity and total porosity, along with a notable decrease in bulk density. These positive changes in physical properties were likely attributed to the oxidation of elemental sulfur, forming sulfuric acid that reacted with soil lime to produce soluble calcium. Consequently, the removal of Na+ from the soil adsorption complex led to enhanced soil aggregation and drainage, resulting in reduced bulk density. Moreover, the application of nano-sulfur increased the availability of essential macronutrients (nitrogen, phosphorus, and potassium) and micronutrients (iron, manganese, and zinc) in the soil. This enrichment was further reflected in maize productivity, as both grain and straw yields increased. Elevated macro and micronutrient content in maize seeds were also observed. The mechanism underlying these effects was attributed to reduced soil pH, facilitating the conversion of insoluble phosphorus into a more accessible form. This conversion releases plant nutrients from previously unusable pools into the soil solution, alleviating stress effects and promoting better plant nutrition as a function of nano-sulfur amendment. Another key mechanism that was not explored in this study is the antioxidant mimic activity of nano-sulfur, which mediates the capture of excessive ROS and enhances plant tolerance to saline stress.
5 Conclusion and future outlook
Nano-sulfur holds great promise as an important nanomaterial to alleviate the current food security challenges faced by agriculture and to simultaneously reduce the input of synthetic pesticides. Its beneficial effects are attributed to its ability to improve plant growth and development, enhance nutrient absorption through soil improvement, stimulate significant defence activity against plant pathogens, and regulate oxidative stress. However, it is important to note that the current understanding of the mechanism of nano-sulfur uptake by plants is incomplete. Nano-sulfur has great potential for application in sustainable agricultural development, but the mechanism of nano-sulfur uptake and transport in plants, the effect of nano-sulfur on soil-plant-microbial system still need to be explored in depth, and the environmental and safety factors in nano-sulfur application need to be considered more comprehensively. Future research work in this area should focus on the following knowledge gaps:
• The rapid development of nanotechnology provides an opportunity to gain significant mechanistic insight into the absorption and transport of nanoparticles (specifically nano-sulfur) in plants. This involves delving into the intricate mechanisms at play on multiple levels:
Current studies on the role of nano-sulfur at the gene, proteome, and metabolome scales are insufficiently in-depth, and in order to reveal the effects of nano-sulfur more comprehensively, it is necessary to explore how it affects gene expression, protein profiles, and metabolic pathways. By identifying specific genes, proteins and metabolites affected by nano-sulfur, we can reveal the molecular basis of nano-sulfur's effects on plant growth and defense mechanisms.
Targeted delivery of the right amount of nano-sulfur at the right growth stage to achieve the best results is an important aspect of future research. Researchers should investigate how plants react to nano-sulfur treatment over time, from initial exposure to long-term effects. Understanding the temporal response of plants to nano-sulfur can inform the optimal timing and dosage of applications, ensuring that the benefits of nano-sulfur are utilized at the right stage of plant growth. In addition, the efficacy of nano-sulfur can be maximized by achieving precisely targeted delivery of nano-sulfur to specific plant tissues, cells and organelles, and scientists should explore innovative delivery mechanisms, such as nanocarriers or smart release systems, to ensure that nano-sulfur reaches its intended destination in the plant.
Investigating the role of material properties, such as particle charge, charge density, morphology, coatings, and dissolution profiles, is essential for understanding how these factors influence the action of nano-sulfur on plants. Researchers should aim to establish a clear correlation between material properties and the efficacy of nano-sulfur in enhancing plant growth and defense mechanisms.
• Environmental and safety considerations are of utmost importance when utilizing nanomaterials. Future research should focus on assessing the environmental and safety aspects associated with nano-sulfur application:
The application of nano-sulfur in agriculture requires a thorough evaluation of potential toxicity and safety risks, both to environmental and public health. Researchers should investigate how factors like dosage, treatment routes, and particle characteristics affect toxicity, both in terms of its impact on plants and its potential to accumulate in the environment, is crucial. In addition, in practical applications, nano-sulfur may be used in combination with other agricultural inputs. Researchers should explore how nano-sulfur interacts with pesticides, fertilizers, or other nanomaterials. These interactions may influence both the effectiveness and safety of agricultural practices. This knowledge will guide the establishment of safe application guidelines. Establishing robust regulations and guidelines for the use of nano-sulfur in agriculture is a priority. Future research should contribute to the development of these regulatory frameworks, ensuring that nano-sulfur is integrated into farming practices responsibly and in accordance with environmental and public health standards.
• Taking a holistic view of the interaction between nano-sulfur and plants, it is imperative to consider the soil-plant-microorganism system. Researchers should investigate how the application of nano-sulfur influences soil properties, including nutrient availability and soil structure, contributing to the optimization of soil health. At the same time, Researchers should study how nano-sulfur alters the composition and function of beneficial and pathogenic microorganisms associated with plants. This knowledge can help ensure that nano-sulfur applications do not disrupt the delicate balance of the plant microbiome. Investigating the formation and dynamics of the biomolecular corona (molecules that adhere to nanoparticles) in various environmental compartments, including soil, plants, and within plant tissues, is important. Understanding how the biomolecular corona influences the fate and behavior of nano-sulfur will provide insights into its environmental and biological interactions.
By addressing these knowledge gaps, future research can advance our understanding of nano-sulfur's role in agriculture and pave the way for more efficient, sustainable, and responsible use of this nanomaterial in addressing food security challenges.
Author contributions
Yi sun and Yaqi Jiang: writing – original draft, conceptualization, visualization. Yuanbo Li, Qibin Wan, Guikai Zhu, Tianjing Yi, Quanlong Wang, Yi Wang, Om Parkash Dhankher, Zhiqiang Tan and Iseult Lynch: review & editing. Jason White, Yukui Rui and Peng Zhang: writing – review & editing, supervision.
Conflicts of interest
The authors declare that they have no known competing financial interests or personal relationships that could have appeared to influence the work reported in this work.
Acknowledgements
Funding support from the National Key R&D Program of China (2017YFD0801103, 2017YFD0801300), and the 111 project of the Education Ministry of China (No. B18053), and the National Natural Science Foundation (32130081) ,and the National Key Research and Development Program of China (No. 2023YFC3711500) and the Fundamental Research Funds for the Central Universities are acknowledged. JCW and OPD acknowledge USDA NIFA AFRI Award 2020-67022-32416.
References
- X. Y. Zheng, W. P. Liu, Z. G. Xu, R. Y. Ying and C. H. Ye, Restructuring grain production in China: regional heterogeneity and its causality, China Agric. Econ. Rev., 2018, 10, 647–665 CrossRef.
- S. M. Howden, J.-F. Soussana, F. N. Tubiello, N. Chhetri, M. Dunlop and H. Meinke, Adapting agriculture to climate change, Proc. Natl. Acad. Sci. U. S. A., 2007, 104, 19691–19696 CrossRef CAS PubMed.
- Z. Tang, Y. Yang and L. Zhang, Molecular Mechanism of Responsing to Drought Stress in Plants, J. Maize Sci., 2006, 14, 96–100 Search PubMed.
- L. I. Ming, Z. Rensen and L. U. O. Shiming, Secondary Metabolites Related with Plant Resistance against Pathogenic Microorganisms and Insect Pests, Chin. J. Biol. Control, 2007, 23, 269–273 Search PubMed.
-
A. E. Douglas, in Annual Review of Plant Biology, ed. S. S. Merchant, 2018, vol. 69, pp. 637–660 Search PubMed.
- A. Iglesias, S. Quiroga and A. Diz, Looking into the future of agriculture in a changing climate, Eur. Rev. Agric. Econ., 2011, 38, 427–447 CrossRef.
- D. Qian, Problem and Countermasure of the Sustainable Utilization of Agricultural Resource in China, J. Anhui Agric. Sci., 2006, 34, 122–123 Search PubMed.
- J.-G. Wagenfeld, K. Al-Ali, S. Almheiri, A. F. Slavens and N. Calvet, Sustainable applications utilizing sulfur, a by-product from oil and gas industry: A state-of-the-art review, Waste Manage., 2019, 95, 78–89 CrossRef PubMed.
- O. P. Narayan, P. Kumar, B. Yadav, M. Dua and A. K. Johri, Sulfur nutrition and its role in plant growth and development, Plant Signaling Behav., 2023, 18, 2030082 CrossRef.
- D. J. Wang, N. B. Saleh, A. Byro, R. Zepp, E. Sahle-Demessie, T. P. Luxton, K. T. Ho, R. M. Burgess, M. Flury, J. C. White and C. M. Su, Nano-enabled pesticides for sustainable agriculture and global food security, Nat. Nanotechnol., 2022, 17, 347–360 CrossRef CAS PubMed.
- M. D. Schulz, O. Khullar, J. V. Frangioni, M. W. Grinstaff and Y. L. Colson, Nanotechnology in Thoracic Surgery, Ann. Thorac. Surg., 2010, 89, S2188–S2190 CrossRef PubMed.
- K. S. Subramanian, R. Rajeswari, M. Yuvaraj, D. Pradeep, M. Guna and G. Yoganathan, Synthesis and Characterization of Nano-Sulfur and Its Impact on Growth, Yield, and Quality of Sunflower (Helianthus annuus L.), Commun. Soil Sci. Plant Anal., 2022, 53, 2700–2709 CrossRef CAS.
-
M. H. Siddiqui, M. H. Al-Whaibi, M. Firoz and M. Y. Al-Khaishany, in Nanotechnology and Plant Sciences: Nanoparticles and Their Impact on Plants, ed. M. H. Siddiqui, M. H. Al-Whaibi and F. Mohammad, Springer International Publishing, Cham, 2015, pp. 19–35, DOI:10.1007/978-3-319-14502-0_2.
-
P. K. Mani and S. Mondal, in Plant Nanotechnology: Principles and Practices, ed. C. Kole, D. S. Kumar and M. V. Khodakovskaya, Springer International Publishing, Cham, 2016, pp. 263–303, DOI:10.1007/978-3-319-42154-4_11.
- A. S. Deshpande, R. B. Khomane, B. K. Vaidya, R. M. Joshi, A. S. Harle and B. D. Kulkarni, Sulfur Nanoparticles Synthesis and Characterization from H2S Gas, Using Novel Biodegradable Iron Chelates in W/O Microemulsion, Nanoscale Res. Lett., 2008, 3, 221 CrossRef CAS.
- Y. Guo, J. Zhao, S. Yang, K. Yu, Z. Wang and H. Zhang, Preparation and characterization of monoclinic sulfur nanoparticles by water-in-oil microemulsions technique, Powder Technol., 2006, 162, 83–86 CrossRef CAS.
- X. Wang, H. Huang, B. Liang, Z. Liu, D. Chen and G. Shen, ZnS Nanostructures: Synthesis, Properties, and Applications, Crit. Rev. Solid State Mater. Sci., 2013, 38, 57–90 CrossRef CAS.
- M. R. Hosseini, M. Schaffie, M. Pazouki, E. Darezereshki and M. Ranjbar, Biologically synthesized copper sulfide nanoparticles: Production and characterization, Mater. Sci. Semicond. Process., 2012, 15, 222–225 CrossRef CAS.
- R. Priyadarshi, Z. Riahi, J. W. Rhim and J. T. Kim, Comparison of physicochemical and functional properties of elemental sulfur, sulfur nanoparticles, and sulfur quantum dots for sustainable biological applications, Mater. Today Sustain., 2022, 20, 100243 CrossRef.
- M. Awad, E. D. S. Ibrahim, E. I. Osman, W. H. Elmenofy, A. W. M. Mahmoud, M. A. M. Atia and M. A. M. Moustafa, Nano-insecticides against the black cutworm Agrotis ipsilon (Lepidoptera: Noctuidae): Toxicity, development, enzyme activity, and DNA mutagenicity, PLoS One, 2022, 17(2), e0254285 CrossRef CAS.
- X. Cao, C. Wang, X. Luo, L. Yue, J. C. White, W. Elmer, O. P. Dhankher, Z. Wang and B. Xing, Elemental Sulfur Nanoparticles Enhance Disease Resistance in Tomatoes, ACS Nano, 2021, 15, 11817–11827 CrossRef CAS PubMed.
- G. A. Ragab and K. M. Saad-Allah, Green synthesis of sulfur nanoparticles using Ocimum basilicum leaves and its prospective effect on manganese-stressed Helianthus annuus (L.) seedlings, Ecotoxicol. Environ. Saf., 2020, 191 CrossRef CAS PubMed.
- H. Yuan, Q. Liu, Z. Guo, J. Fu, Y. Sun, C. Gu, B. Xing and O. P. Dhankher, Sulfur nanoparticles improved plant growth and reduced mercury toxicity via mitigating the oxidative stress in Brassica napus L, J. Cleaner Prod., 2021, 318, 128589 CrossRef CAS.
- Q. Zhuang, Q. Liu, Y. Sun, J. Fu, S. Tang, S. Sharma, O. P. Dhankher and H. Yuan, Influence of nanoscale sulfur on mercury accumulation and plant growth in oilseed rape seedlings (Brassica napus L.) grown on mercury-contaminated soil, Int. J. Phytorem., 2024, 26(4), 524–534 CrossRef CAS PubMed.
- S. Sharma, G. Singh, Y. Wang, J. C. White, B. Xing and O. P. Dhankher, Nanoscale sulfur alleviates silver nanoparticle toxicity and improves seed and oil yield in Soybean (Glycine max), Environ. Pollut., 2023, 336, 122423 CrossRef CAS PubMed.
- A. G. Meselhy, S. Sharma, Z. Guo, G. Singh, H. Yuan, R. D. Tripathi, B. Xing, C. Musante, J. C. White and O. P. Dhankher, Nanoscale Sulfur Improves Plant Growth and Reduces Arsenic Toxicity and Accumulation in Rice (Oryza sativa L.), Environ. Sci. Technol., 2021, 55, 13490–13503 CrossRef.
- J. M. Jez, Structural biology of plant sulfur metabolism: from sulfate to glutathione, J. Exp. Bot., 2019, 70, 4089–4103 CrossRef CAS.
- S. Kopriva, M. Malagoli and H. Takahashi, Sulfur nutrition: impacts on plant development, metabolism, and stress responses, J. Exp. Bot., 2019, 70, 4069–4073 CrossRef CAS PubMed.
- M. Lewandowska and A. Sirko, Recent advances in understanding plant response to sulfur-deficiency stress, Acta Biochim. Pol., 2008, 55, 457–471 CrossRef CAS.
- I. D. Nwachukwu, A. J. Slusarenko and M. C. H. Gruhlke, Sulfur and Sulfur Compounds in Plant Defence, Nat. Prod. Commun., 2012, 7, 395–400 CrossRef CAS PubMed.
- B. Paulose, S. Chhikara, J. Coomey, H. I. Jung, O. Vatamaniuk and O. P. Dhankher, A γ-glutamyl cyclotransferase protects Arabidopsis plants from heavy metal toxicity by recycling glutamate to maintain glutathione homeostasis, Plant cell, 2013, 25, 4580–4595 CrossRef CAS PubMed.
- C. M. Griffith, J. E. Woodrow and J. N. Seiber, Environmental behavior and analysis of agricultural sulfur, Pest Manage. Sci., 2015, 71, 1486–1496 CrossRef CAS PubMed.
-
The Top 100 Chemicals by Pounds in Total Statewide Pesticide Use in 2021.[Online], 2021 Search PubMed.
-
W. Forsyth, A Treatise on the Culture and Management of Fruit-Trees: In Which a New Method of Pruning and Training is Fully Described, Cambridge University Press, Cambridge, 2011 Search PubMed.
- J. S. Williams and R. M. Cooper, The oldest fungicide and newest phytoalexin – a reappraisal of the fungitoxicity of elemental sulphur, Plant Pathol., 2004, 53, 263–279 CrossRef CAS.
- M. Afrousheh and h. hasheminasab, Sulfur Application as Pesticide in Pistachio Orchard: Health and Safety, Pistachio Health J., 2018, 1, 52–63 Search PubMed.
- P. J. White and P. H. Brown, Plant nutrition for sustainable development and global health, Ann. Bot., 2010, 105, 1073–1080 CrossRef CAS.
- F. A. Gilbert, The place of sulfur in plant nutrition, Bot. Rev., 1951, 17, 671–691 CrossRef CAS.
- E. D. Solberg, S. S. Malhi, M. Nyborg and K. S. Gill, Fertilizer type, tillage, and application time effects on recovery of sulfate-S from elemental sulfur fertilizers in fallow field soils, Commun. Soil Sci. Plant Anal., 2003, 34, 815–830 CrossRef CAS.
- H. Zhang, Y. Li, W. Wang and L. Wang, Research progress of the microbial sulfur-cycling network, Acta Microbiol. Sin., 2021, 61, 1567–1581 CAS.
- B. V. Subbiah and N. Singh, Efficiency of gypsum as a source of sulphur to oilseed crops studied with radioactive sulphur and radioactive calcium, Indian J. Agric. Sci., 1970, 40, 227–234 CAS.
-
M. S. Aulakh, in Sulphur in Plants, ed. Y. P. Abrol and A. Ahmad, Springer Netherlands, Dordrecht, 2003, pp. 341–358, DOI:10.1007/978-94-017-0289-8_19.
- D. Scott and W. J. Archie, Sulphur, phosphate, and molybdenum coating of legume seed, N. Z. J. Agric. Res., 1978, 21, 643–649 CrossRef.
- J. R. Gerson and E.-L. S. Hinckley, It Is Time to Develop Sustainable Management of Agricultural Sulfur, Earth's Future, 2023, 11, e2023EF003723 CrossRef CAS.
- E.-L. S. Hinckley and C. T. Driscoll, Sulfur fertiliser use in the Midwestern US increases as atmospheric sulfur deposition declines with improved air quality, Commun. Earth Environ., 2022, 3, 324 CrossRef.
- J. J. Germida and H. H. Janzen, Factors affecting the oxidation of elemental sulfur in soils, Fert. Res., 1993, 35, 101–114 CrossRef CAS.
- C. Fn and M. Mf, Factors Affecting Water Pollution: A Review, J. Ecosyst. Ecography, 2017, 2017, 1–3 Search PubMed.
- L. Aula, J. S. Dhillon, P. Omara, G. B. Wehmeyer, K. W. Freeman and W. R. Raun, World Sulfur Use Efficiency for Cereal Crops, Agron. J., 2019, 111, 2485–2492 CrossRef CAS.
- E.-L. S. Hinckley and P. A. Matson, Transformations, transport, and potential unintended consequences of high sulfur inputs to Napa Valley vineyards, Proc. Natl. Acad. Sci. U. S. A., 2011, 108, 14005–14010 CrossRef CAS PubMed.
- P. Ranadev, A. Revanna, D. J. Bagyaraj and A. H. Shinde, Sulfur oxidizing bacteria in agro ecosystem and its role in plant productivity—a review, J. Appl. Microbiol., 2023, 134, lxad161 CrossRef PubMed.
- Y.-M. Go, J. D. Chandler and D. P. Jones, The cysteine proteome, Free Radicals Biol. Med., 2015, 84, 227–245 CrossRef CAS PubMed.
- S. Ravanel, B. Gakiere, D. Job and R. Douce, The specific features of methionine biosynthesis and metabolism in plants, Proc. Natl. Acad. Sci. U. S. A., 1998, 95, 7805–7812 CrossRef CAS PubMed.
- A. Wawrzynska, G. Moniuszko and A. Sirko, Links Between Ethylene and Sulfur Nutrition—A Regulatory Interplay or Just Metabolite Association?, Front. Plant Sci., 2015, 6, 1053 Search PubMed.
- Q. Li, Y. Gao and A. Yang, Sulfur Homeostasis in Plants, Int. J. Mol. Sci., 2020, 21(23), 8926 CrossRef CAS.
- O. P. Dhankher, Y. Li, B. P. Rosen, J. Shi, D. Salt, J. F. Senecoff, N. A. Sashti and R. B. Meagher, Engineering tolerance and hyperaccumulation of arsenic in plants by combining arsenate reductase and γ-glutamylcysteine synthetase expression, Nat. Biotechnol., 2002, 20, 1140–1145 CrossRef CAS PubMed.
- Z. Zhang, X. He, H. Zhang, Y. Ma, P. Zhang, Y. Ding and Y. Zhao, Uptake and distribution of ceria nanoparticles in cucumber plants, Metallomics, 2011, 3, 816–822 CrossRef CAS PubMed.
- Y. Wang, C. Deng, Y. Shen, J. Borgatta, C. O. Dimkpa, B. Xing, O. P. Dhankher, Z. Wang, J. C. White and W. H. Elmer, Surface Coated Sulfur Nanoparticles Suppress Fusarium Disease in Field Grown Tomato: Increased Yield and Nutrient Biofortification, J. Agric. Food Chem., 2022, 70, 14377–14385 CrossRef CAS PubMed.
- M. Agrawal and M. Verma, Amelioration of Sulphur Dioxide Phytotoxicity in Wheat Cultivars by Modifying NPK Nutrients, J. Environ. Manage., 1997, 49, 231–244 CrossRef.
- J. N. Cape, D. Fowler and A. Davison, Ecological effects of sulfur dioxide, fluorides, and minor air pollutants: recent trends and research needs, Environ. Int., 2003, 29, 201–211 CrossRef CAS.
- X. He, P. Fu, W. G. Aker and H.-M. Hwang, Toxicity of engineered nanomaterials mediated by nano–bio–eco interactions, J. Environ. Sci. Health, Part C, 2018, 36, 21–42 CrossRef CAS PubMed.
- M. Tonigold, J. Simon, D. Estupiñán, M. Kokkinopoulou, J. Reinholz, U. Kintzel, A. Kaltbeitzel, P. Renz, M. P. Domogalla, K. Steinbrink, I. Lieberwirth, D. Crespy, K. Landfester and V. Mailänder, Pre-adsorption of antibodies enables targeting of nanocarriers despite a biomolecular corona, Nat. Nanotechnol., 2018, 13, 862–869 CrossRef CAS PubMed.
-
C. Dong, C. Jiao and Z. Zhang, in Nano-Enabled Sustainable and Precision Agriculture, ed. P. Zhang, I. Lynch, J. C. White and R. D. Handy, Academic Press, 2023, pp. 355–375, DOI:10.1016/B978-0-323-91233-4.00005-3.
- A. Shakoor, G. Jilani, T. Iqbal, I. Mahmood, T. Alam, M. A. Ali, S. S. H. Shah and R. Ahmad, Synthesis of Elemental- and Nano-sulfur-enriched Bio-organic Phosphate Composites, and Their Impact on Nutrients Bioavailability and Maize Growth, J. Soil Sci. Plant Nutr., 2023, 23, 3281–3289 CrossRef CAS.
- M. Kaya, R. Karaman and A. Sener, Effects of nano sulfur (s) applications on yield and some yield properties of bread wheat, Agronomy, 2018, 61, 274–279 Search PubMed.
- N. M. Salem, L. Al-Banna, A. O. Abdeen, Q. I. Ibrahim and A. M. Awwad, Sulfur Nanoparticles Improves Root and Shoot Growth of Tomato, J. Agric. Sci., 2016, 8, 179–185 Search PubMed.
- Y. Jiang, P. Zhou, P. Zhang, M. Adeel, N. Shakoor, Y. Li, M. Li, M. Guo, W. Zhao, B. Lou, L. Wang, I. Lynch and Y. Rui, Green synthesis of metal-based nanoparticles for sustainable agriculture, Environ. Pollut., 2022, 309, 119755 CrossRef CAS PubMed.
-
L. Al-Banna, N. M. Salem and A. M. Awwad, Seed Germination and Growth of Cucumber (Cucumis sativus): Effect of Nano-Crystalline Sulfur, J. Agric. Sci., 2016, 8, 219 Search PubMed.
- Y. Wang, C. Deng, W. H. Elmer, C. O. Dimkpa, S. Sharma, G. Navarro, Z. Wang, J. LaReau, B. T. Steven, Z. Wang, L. Zhao, C. Li, O. P. Dhankher, J. L. Gardea-Torresdey, B. Xing and J. C. White, Therapeutic Delivery of Nanoscale Sulfur to Suppress Disease in Tomatoes: In Vitro Imaging and Orthogonal Mechanistic Investigation, ACS Nano, 2022, 16, 11204–11217 CrossRef CAS PubMed.
-
J. J. Smith, Nanoscale Sulfur as a Novel Fertilizer for Promoting Wheat (Triticum aestivum L.) Growth and Yield, Masters Theses, 2023, p. 1275.
- S. Elghany, A. Abdel Fattah, A. Arafat and M. Esmaeil, Assessment of the Effect of Nano Sulfur on Some Soil Properties and Maize Productivity in Saline Soil,
Curr. Sci. Int.
, 2020, 9, 656–665 Search PubMed.
- N. M. Salem, L. S. Albanna and A. M. Awwad, Green synthesis of sulfur nanoparticles using Punica granatum peels and the effects on the growth of tomato by foliar spray applications, Environ. Nanotechnol., Monit. Manage., 2016, 6, 83–87 Search PubMed.
- Y. Wang, L. Chen, L. Scudiero and W. H. Zhong, The beauty of frost: nano-sulfur assembly via low pressure vapour deposition, Chem. Commun., 2015, 51, 15967–15970 RSC.
- J. Yong, M. Wu, R. Zhang, S. Bi, C. W. G. Mann, N. Mitter, B. J. Carroll and Z. P. Xu, Clay nanoparticles efficiently deliver small interfering RNA to intact plant leaf cells, Plant Physiol., 2022, 190, 2187–2202 CrossRef PubMed.
- B. Hou, B. Zheng, W. Yang, C. Dong, H. Wang and J. Chang, Construction of near infrared light triggered nanodumbbell for cancer photodynamic therapy, J. Colloid Interface Sci., 2017, 494, 363–372 CrossRef CAS PubMed.
- L. M. Wiesholler, F. Frenzel, B. Grauel, C. Würth, U. Resch-Genger and T. Hirsch, Yb,Nd,Er-doped upconversion nanoparticles: 980 nm versus 808 nm excitation, Nanoscale, 2019, 11, 13440–13449 RSC.
- M. Malik, P. Padhye and P. Poddar, Downconversion Luminescence-Based Nanosensor for Label-Free Detection of Explosives, ACS Omega, 2019, 4, 4259–4268 CrossRef CAS.
- H. Wu and Z. Li, Recent advances in nano-enabled agriculture for improving plant performance, Crop J., 2022, 10, 1–12 CrossRef CAS.
- M. Ghorbanpour, A. Movahedi, M. Hatami, K. Kariman, F. Bovand and M. A. J. P. Shahid, Insights into nanoparticle-induced changes in plant photosynthesis, Photosynthetica, 2021, 59, 570–586 CrossRef CAS.
- F. Gao, F. Hong, C. Liu, L. Zheng, M. Su, X. Wu, F. Yang, C. Wu and P. Yang, Mechanism of nano-anatase TiO2 on promoting photosynthetic carbon reaction of spinach, Biol. Trace Elem. Res., 2006, 111, 239–253 CrossRef CAS.
- M. Giordano, V. Pezzoni and R. d. Hell, Strategies for the Allocation of Resources under Sulfur Limitation in the Green Alga Dunaliella salina, Plant Physiol., 2000, 124, 857–864 CrossRef CAS PubMed.
- M. R. Naderi and A. Danesh-Shahraki, Nanofertilizers and their roles in sustainable agriculture, Int. J. Agric. Crop Sci., 2013, 5, 2229–2232 Search PubMed.
-
K. S. Subramanian and M. Thirunavukkarasu, in Nanoscience and Plant–Soil Systems, ed. M. Ghorbanpour, K. Manika and A. Varma, Springer International Publishing, Cham, 2017, pp. 305–319, DOI:10.1007/978-3-319-46835-8_11.
- J. J. Germida and H. H. Janzen, Factors affecting the oxidation of elemental sulfur in soils, Fert. Res., 1993, 35, 101–114 CrossRef CAS.
- M. Tourna, P. Maclean, L. Condron, M. O'Callaghan and S. A. Wakelin, Links between sulphur oxidation and sulphur-oxidising bacteria abundance and diversity in soil microcosms based on soxB functional gene analysis, FEMS Microbiol. Ecol., 2014, 88, 538–549 CrossRef CAS PubMed.
- M. Karimi, H. Aminuddin, M. Yusop and R. Othman, Elemental sulphur application effects on nutrient availability and sweet maize (Zea mays L.) response in a high pH soil of Malaysia, Malays. J. Soil Sci., 2014, 18, 75–86 Search PubMed.
- K. H. El-Hamdi, M. A. El-Soud and G. M. A. El-Sanat, Effect of some soil amendments application on the productivity of wheat and soybean, mobility and availability of nitrogen, J. Soil Sci. Agric. Eng., 2007, 32, 7953–7965 Search PubMed.
- H. Etesami, B. R. Jeong and B. R. Glick, Contribution of Arbuscular Mycorrhizal Fungi, Phosphate–Solubilizing Bacteria, and Silicon to P Uptake by Plant, Front. Plant Sci., 2021, 12, 699618 CrossRef.
- S. M. Nadeem, A. Hanif, M. Y. Khan, M. R. Waqas, Z. Ahmad, M. R. Ashraf and M. Naveed, Elemental sulphur with sulphur oxidizing bacteria enhances phosphorus availability and improves growth and yield of wheat in calcareous soil, Arch. Agron. Soil Sci., 2023, 69, 1494–1502 CrossRef CAS.
- W. Orem, C. Gilmour, D. Axelrad, D. Krabbenhoft, D. Scheidt, P. Kalla, P. McCormick, M. Gabriel and G. Aiken, Sulfur in the South Florida Ecosystem: Distribution, Sources, Biogeochemistry, Impacts, and Management for Restoration, Crit. Rev. Environ. Sci. Technol., 2011, 41, 249–288 CrossRef CAS.
- A. L. Bates, W. H. Orem, J. W. Harvey and E. C. Spiker, Tracing Sources of Sulfur in the Florida Everglades, J. Environ. Qual., 2002, 31, 287–299 CrossRef CAS PubMed.
- M. Anisuzzaman, M. Y. Rafii, N. M. Jaafar, S. Izan Ramlee, M. F. Ikbal and M. A. Haque, Effect of Organic and Inorganic Fertilizer on the Growth and Yield Components of Traditional and Improved Rice (Oryza sativa L.) Genotypes in Malaysia, Agronomy, 2021, 11, 1830 CrossRef CAS.
-
T. Masunaga and J. D. Marques Fong, in Plant Micronutrient Use Efficiency, ed. M. A. Hossain, T. Kamiya, D. J. Burritt, L.-S. Phan Tran and T. Fujiwara, Academic Press, 2018, pp. 195–208, DOI:10.1016/B978-0-12-812104-7.00013-7.
- J. Lai, X. Cao, T. Yu, Q. Wang, Y. W. Zhang, X. D. Zheng and H. P. Lu, Effect of Cryptococcus laurentii on inducing disease resistance in cherry tomato fruit with focus on the expression of defense-related genes, Food Chem., 2018, 254, 208–216 CrossRef CAS PubMed.
- A. M. Catanzariti, G. T. T. Lim and D. A. Jones, The tomato I-3 gene: a novel gene for resistance to Fusarium wilt disease, New Phytol., 2015, 207, 106–118 CrossRef CAS PubMed.
- G. Huang, N. Zuverza-Mena, J. C. White, H. Hu, B. Xing and O. P. Dhankher, Simultaneous exposure of wheat (Triticum aestivum L.) to CuO and S nanoparticles alleviates toxicity by reducing Cu accumulation and modulating antioxidant response, Sci. Total Environ., 2022, 839, 156285 CrossRef CAS PubMed.
- S. H. Chun, J. S. Yuk and S. H. Um, Regulation of cellular gene expression by nanomaterials, Nano Convergence, 2018, 5, 34 CrossRef PubMed.
- A. O. Choi, S. E. Brown, M. Szyf and D. Maysinger, Quantum dot-induced epigenetic and genotoxic changes in human breast cancer cells, J. Mol. Med., 2008, 86, 291–302 CrossRef CAS PubMed.
- C. J. Dorman and M. J. Dorman, DNA supercoiling is a fundamental regulatory principle in the control of bacterial gene expression, Biophys. Rev., 2016, 8, 89–100 CrossRef CAS PubMed.
- L. Libenson, F. P. Hadley, A. P. McIlroy, V. M. Wetzel and R. R. Mellon, Antibacterial Effect of Elemental Sulfur, J. Infect. Dis., 1953, 93, 28–35 CrossRef CAS PubMed.
- S. E. A. McCallan, The nature of the fungicidal action of copper and sulfur, Biophys. Rev., 1949, 15, 629–643 CAS.
- L. Libenson, F. P. Hadley, A. P. McIlroy, V. M. Wetzel and R. R. Mellon, Antibacterial effect of elemental sulfur, J. Infect. Dis., 1953, 93, 28–35 CrossRef CAS PubMed.
- S. Roy Choudhury, A. Mandal, M. Ghosh, S. Basu, D. Chakravorty and A. Goswami, Investigation of antimicrobial physiology of orthorhombic and monoclinic nanoallotropes of sulfur at the interface of transcriptome and metabolome, Appl. Microbiol. Biotechnol., 2013, 97, 5965–5978 CrossRef CAS PubMed.
- S. Roy Choudhury, M. Ghosh, A. Mandal, D. Chakravorty, M. Pal, S. Pradhan and A. Goswami, Surface-modified sulfur nanoparticles: an effective antifungal agent against Aspergillus niger and Fusarium oxysporum, Appl. Microbiol. Biotechnol., 2011, 90, 733–743 CrossRef CAS PubMed.
- M. Valodkar, S. Modi, A. Pal and S. Thakore, Synthesis and anti-bacterial activity of Cu, Ag and Cu–Ag alloy nanoparticles: A green approach, Mater. Res. Bull., 2011, 46, 384–389 CrossRef CAS.
- X. Fan, L. H. Yahia and E. Sacher, Antimicrobial Properties of the Ag, Cu Nanoparticle System, Biology, 2021, 10, 137 CrossRef CAS PubMed.
-
S. R. Choudhury, K. K. Nair, R. Kumar, R. Gogoi, C. Srivastava, M. Gopal, B. S. Subhramanyam, C. devakumar and A. Goswami, Nanosulfur: A Potent Fungicide Against Food Pathogen, Aspergillus niger, AIP Conference Proceedings, 2010, vol. 1276, pp. 154–157 Search PubMed.
- K. J. Rao and S. Paria, Use of sulfur nanoparticles as a green pesticide on Fusarium solani and Venturia inaequalis phytopathogens, RSC Adv., 2013, 3, 10471–10478 RSC.
- A. S. Deshpande, R. B. Khomane, B. K. Vaidya, R. M. Joshi, A. S. Harle and B. D. Kulkarni, Sulfur Nanoparticles Synthesis and Characterization from H2S Gas, Using Novel Biodegradable Iron Chelates in Aqueous Surfactant Systems, MRS Online Proc. Libr., 2011, 1103, 1 Search PubMed.
- S. R. Choudhury, A. Basu, T. Nag, K. Sengupta, M. Bhowmik and A. Goswami, Expedition of in vitro dissolution and in vivo pharmacokinetic profiling of sulfur nanoparticles based antimicrobials, Environ. Toxicol. Pharmacol., 2013, 36, 675–679 CrossRef CAS PubMed.
- M. Suleiman, M. Almasri, A. Al Ali, D. Aref, A. Hussein, I. Saadeddin and I. Warad, Synthesis of Nano-sized Sulfur Nanoparticles and their Antibacterial Activities, J. Mater. Environ. Sci., 2015, 6, 513–518 CAS.
- S. Shankar, R. Pangeni, J. W. Park and J.-W. Rhim, Preparation of sulfur nanoparticles and their antibacterial activity and cytotoxic effect, Mater. Sci. Eng. C, 2018, 92, 508–517 CrossRef CAS PubMed.
- P. Paralikar and M. Rai, Bio-inspired synthesis of sulphur nanoparticles using leaf extract of four medicinal plants with special reference to their antibacterial activity, IET Nanobiotechnol., 2018, 12, 25–31 CrossRef.
- G. A. Ragab and K. M. Saad-Allah, Green synthesis of sulfur nanoparticles using Ocimum basilicum leaves and its prospective effect on manganese-stressed Helianthus annuus (L.) seedlings, Ecotoxicol. Environ. Saf., 2020, 191, 110242 CrossRef CAS PubMed.
- H. Yuan, Q. Liu, J. Fu, Y. Wang, Y. Zhang, Y. Sun, H. Tong and O. P. Dhankher, Co-exposure of sulfur nanoparticles and Cu alleviate Cu stress and toxicity to oilseed rape Brassica napus L, J. Environ. Sci., 2023, 124, 319–329 CrossRef CAS PubMed.
Footnote |
† The two authors contributed equally to the manuscript. |
|
This journal is © The Royal Society of Chemistry 2024 |