DOI:
10.1039/D3SC05849B
(Edge Article)
Chem. Sci., 2024,
15, 511-515
Soluble Gd6Cu24 clusters: effective molecular electrocatalysts for water oxidation†
Received
2nd November 2023
, Accepted 4th December 2023
First published on 5th December 2023
Abstract
The water oxidation half reaction in water splitting for hydrogen production is extremely rate-limiting. This study reports the synthesis of two heterometallic clusters (Gd6Cu24-IM and Gd6Cu24-AC) for application as efficient water oxidation catalysts. Interestingly, the maximum turnover frequency of Gd6Cu24-IM in an NaAc solution of a weak acid (pH 6) was 319 s−1. The trimetallic catalytic site, H2O–GdIIICuII2–H2O, underwent two consecutive two-electron two-proton coupled transfer processes to form high-valent GdIII–O–O–CuIII2 intermediates. Furthermore, the O–O bond was formed via intramolecular interactions between the CuIII and GdIII centers. The results of this study revealed that synergistic catalytic water oxidation between polymetallic sites can be an effective strategy for regulating O–O bond formation.
Introduction
Hydrogen production by water splitting is a promising solution for future energy needs;1 however, water oxidation is rate-limiting, primarily due to its four-electron transfer process with slow reaction kinetics.2 Many researchers have been inspired by natural photosynthesis to develop catalytic systems to resolve this rate-limiting issue.3 Several studies have developed molecular water oxidation catalysts.4 In particular, significant efforts have focused on mimicking the structure and catalytic properties of the natural oxygen-evolving complex (OEC), a unique heterometallic-oxide Mn4CaO5-cluster, in photosystem II (PS II) of plants, algae, and cyanobacteria.5 Although several multinuclear clusters simulating OEC structures have been reported,6 their catalytic performance is still far from that of natural OEC, with the mechanism behind the O–O bond formation still not yet conclusively known.7 Catalysts usually should easily attain four electrons and contain effective synergistic catalytic structural motifs to achieve a similar catalytic activity.8 Previous studies on structural motifs have mainly focused on the Mn–O–Mn–H2O bimetallic structure.9,10 Recent studies have shown that the redox-inert Ca2+ ion in Mn4CaO5 may play an important role in maintaining the structural integrity of clusters and supporting ligand sites for water molecules or in assisting proton transfer.11 However, the poor stability of Ca2+-containing Mn4CaO4 clusters in polar solvents has hindered their development.12 Therefore, developing efficient and stable cluster catalysts for water oxidation remains challenging.
Several studies have recently reported that replacing Ca2+ ions with lanthanide ions can stabilize the cluster structure in performance simulations.6c,13 Furthermore, lanthanide ions have high coordination numbers, rich geometries, and structural flexibility.14 This study reports the synthesis of two high-nuclearity clusters—[Gd6Cu24(IM)12(L-Al)12(μ3-OH)30(μ2-OH)6(CO3)(H2O)24]·(ClO4)16·(H2O)6 (Gd6Cu24-IM) and [Gd6Cu24(Ac)6(L-Al)12(μ3-OH)30(μ2-OH)6Cl(H2O)24]·(ClO4)11·(H2O)19 (Gd6Cu24-AC) (IM = imidazole, L-Al = L-alanine, Ac = acetate)—possessing the same metal core frames (Fig. 1 and S1†). Similar to H2O–Ca–O–Mn2–H2O in OEC, the trimetallic structural motif of H2O–Gd–O–Cu2–H2O in Gd6Cu24 may be a potentially efficient synergistic catalytic site for water oxidation.
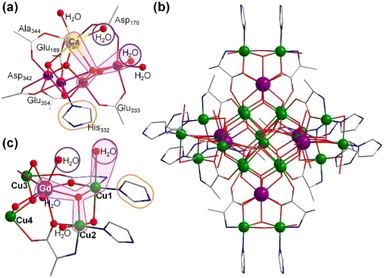 |
| Fig. 1 (a) Structure of the Mn4CaO5 cluster. (b) Core of the Gd6Cu24-IM. (c) Asymmetric unit (GdCu4) of Gd6Cu24-IM. H2O–Ca–O–Mn2–H2O and H2O–Gd–O–Cu2–H2O motifs are marked in pink and water molecules are marked by a purple circle. Color codes: Gd, purple; Cu, green; Mn, pink; Ca, yellow; C, gray; N, blue; O, red. All H atoms were omitted. | |
Results and discussion
Clusters Gd6Cu24-IM and Gd6Cu24-AC were obtained by the reaction of Gd(ClO4)3, Cu(ClO4)2·6H2O, and L-alanine with imidazole and NaAc, respectively. The asymmetric structural unit [GdCu4] of Gd6Cu24 can be considered as two corner-deficient [GdCu2O3] and [GdCu2O4] cubes linked together by sharing a Gd3+ ion (Fig. 1c). Six [GdCu4] units were connected by four μ3-O2−, resulting in an octahedral conformational arrangement. The metal core of Gd6Cu24 displayed an octahedral inner core of Gd6Cu12 connected to six outer Cu2 units. Each [GdCu4] unit contained five metal ions, in which Gd, Cu1, and Cu2 were bound to water molecules. Furthermore, the Gd6Cu24-IM cluster was protected by 12 amino acids and 12 imidazole ligands, highly resembling the peripheral ligands of the OEC. Furthermore, each cluster core unit of Gd6Cu24-IM and Gd6Cu24-AC had 16 and 11 positive charges in addition to 12 sets of water-binding sites, which can promote water oxidation catalysis.
The catalytic properties were characterized in an aqueous solution, and the stability was verified in the high-resolution electrospray ionization mass spectrometry (HRESI-MS) spectra (Fig. S6†). For Gd6Cu24-IM, the peak at 1978.73 can be attributed to [Gd6Cu24(IM)12(L-Al)12(μ3-OH)28(O)8(CO3)1(H2O)24(ClO4)5]3+ (Fig. S6a†); for Gd6Cu24-AC, these peaks are observed and can be attributed to [Gd6Cu24(Ac)x(L-Al)10(μ3-OH)28(O)8(H2O)2(ClO4)8−x]4+ (X = 0–6) (Fig. S6b†). The redox properties of Gd6Cu24-IM and Gd6Cu24-AC were analyzed by cyclic voltammetry (CV) and differential pulse voltammetry (DPV) in an NaAc/HAc buffer solution (0.5 M, pH = 6) (Fig. 2, S7 and S8†). The redox current of Gd6Cu24-IM at E1/2 (0.15 V, all potentials were compared to the normal hydrogen electrode, NHE) depended linearly on the square root of the scan rate and corresponded to diffusion control. Moreover, the difference between the cathodic and anodic peak potentials of 30 mV (ΔEp, Fig. 2a, b and S7†) indicated a two-electron quasi-reversible process.15 However, the reduction current of Gd6Cu24-AC (Fig. 2c, d and S8†) at 0.13 V was not reversible for the reaction, although it linearly depended on the square root of the scan rate owing to the dissociation of the charged acetate during the reduction process.16 By performing DPV at a low scan rate, the reduction current corresponded to the splitting of the oxidation current peak into two peaks attributed to two electron transfers as well as the relocation of the acetate ligand. As determining the anodic shoulder peak potential (Ep) of approximately 1.35 V is difficult, the half-peak potential Ep/2versus ln(ν) was fitted using the Laviron equation (Fig. 3a, b, S9 and S10†).17 The fitted slope was equal to RT/(1 − α)nF for the anodic shoulder peak, where α is the transfer coefficient (0.5) and n was calculated to be 2. These results indicated that the two peripheral Cu2+ ions in the [GdCu4] unit could be considered equivalent groups for the single-electron reaction, i.e., the quasi-reversible process near 0.15 V can be attributed to the reaction pair of two-electron reduction of
while the anodic shoulder peak around 1.35 V can be attributed to the two-electron oxidation of
6e
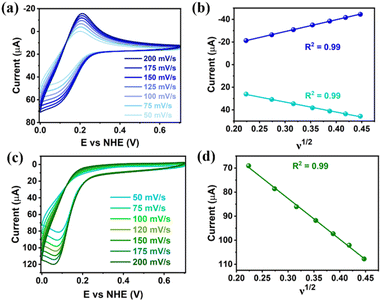 |
| Fig. 2 (a) CV of 0.25 mM Gd6Cu24-IM in 0.5 M NaAc/HAc (pH = 6) buffer solution using a GC electrode with different scan rates (0.05–0.2 V s−1) at 0–0.7 V. (b) Plots of ip (μA) vs. ν1/2 (V1/2 s−1/2) for Gd6Cu24-IM. (c) CV of 0.25 mM Gd6Cu24-AC in 0.5 M NaAc/HAc (pH = 6) buffer solution using the GC electrode with different scan rates (0.05–0.2 V s−1) at 0–0.7 V. (d) Plots of ip (μA) vs. ν1/2 (V1/2 s−1/2) for Gd6Cu24-AC. | |
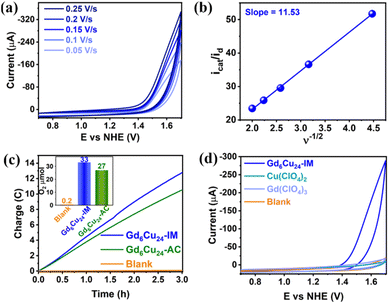 |
| Fig. 3 (a) CVs of Gd6Cu24-IM (0.25 mM) in NaAc/HAc (0.5 M, pH = 6) buffer solution at different scan rates: 50–250 mV s−1 at 0.7–1.7 V. (b) Plot of icat/idversus ν−1/2 at 1.7 V. (c) CPE data showing charge versus time for a 20 mL solution containing NaAc/HAc buffer solution (0.5 M, pH = 6) and 0.25 mM Gd6Cu24-IM and Gd6Cu24-AC. (d) CVs of Gd6Cu24-IM (0.25 mM), Gd(ClO4)3 (1.5 mM), and Cu(ClO4)2 (6 mM). | |
The DPVs of Gd6Cu24-IM and Gd6Cu24-AC transformed significantly by changing the solvent from superdry acetonitrile to an aqueous NaAc/HAc buffer solution (pH = 6; Fig. S11 and S12†).6e,13d In acetonitrile, the oxidation peak at 1.72 V can be attributed to the two-electron oxidation of the
reaction pair. In the NaAc/HAc buffer, the anodic current was significantly enhanced after 1.35 V, thus indicating the occurrence of aqueous oxidation. A linear relationship was observed in the plot of the catalytic peak current versusGd6Cu24-IM concentration (Fig. S13†), thus indicating that the rate law for Gd6Cu24-IM catalyzed water oxidation can be expressed in terms of a pseudo-first-order rate reaction (eqn (1)–(3), ESI†).18 The catalytic peak currents were directly proportional to the square root of the scan rate, thus indicating a diffusion-controlled catalytic redox process.19 Linear scan voltammograms (LSV) of Gd6Cu24-IM and Gd6Cu24-AC were recorded on an indium tin oxide (ITO) electrode. The significantly enhanced catalytic currents indicated water oxidation (Fig. S14†). The overpotentials for Gd6Cu24-IM and Gd6Cu24-AC required to reach 1 mA cm−2 were 598 and 689 mV, respectively. In comparison to previous reports (Table S2†), the overpotentials represent a relatively lower level. The difference in the overpotentials may be attributed to the different peripheral coordination groups between Gd6Cu24-IM and Gd6Cu24-AC (imidazole and acetate, respectively), and the conjugation effect of imidazole ligands may help to reduce the reaction energy barrier.20 The apparent rate constants (kcat), called turnover frequency (TOF), for Gd6Cu24-IM and Gd6Cu24-AC at 1.7 V were 319 and 169 s−1, respectively (Fig. 3b and S9†),21 much higher than those of previously reported mono- and di-nuclear catalysts (0.4–100 s−1).22
A gas-tight double-compartment cell with the cathode and anode separated by a Nafion membrane was utilized to verify the release of oxygen. Gd6Cu24-IM (0.25 mM) was subjected to controlled potential electrolysis (CPE) at 1.70 V in an NaAc/HAc buffer solution (0.5 M, pH = 6) using an ITO electrode (1.00 cm2; Fig. 3c). Electrolysis was performed for 3 h and oxygen production was determined by gas chromatography, where approximately 33 μmol of O2 were formed with a faradaic efficiency of 94%. The ratio of H2 to O2 was measured (2
:
1). For Gd6Cu24-IM, neither the blank nor the inorganic salt catalytic tests showed a significant catalytic current. The reproducibility of the 50 times cycle scan, the almost identical UV-Vis spectra, and the CV curves before and after 3 h of controlled potential electrolysis (Fig. 3d and S15–S18†) indicated that water oxidation occurred under homogeneous catalytic conditions in addition to the high activity and stability of Gd6Cu24-IM.23
The DPVs of Gd6Cu24 clusters in a mixture of superdry CH3CN and H2O at different concentrations were recorded to elucidate the water oxidation mechanism. The results (Fig. 4a) revealed that the DPV curves of Gd6Cu24-AC changed significantly with the addition of water, where the oxidation peak current increased dramatically and the oxidation onset potential shifted positively, indicating that the coordination of water can reduce the oxidation onset potential. The DPV curves of Gd6Cu24-IM changed in a pattern similar to that of Gd6Cu24-AC, although its two oxidation peaks did not show significant splitting (Fig. S19†). Changes in the oxidation onset potential were observed by changing the pH of the NaAc/HAc buffer solution, indicating that the oxidation process involved a proton-coupled electron transfer (PCET). The Pourbaix diagram revealed that (Fig. 4b) in an NaAc/HAc buffered aqueous solution (0.5 M, pH 4.98–7), two consecutive oxidation peaks of Gd6Cu24-AC exhibited Nernstian responses at approximately −55 and −50 mV pH−1, consistent with the 2e−/2H+ PCET (−59 mV pH−1, Nernstian ideal),24 while the responses of Gd6Cu24-IM were −54 and −57 mV pH−1 (Fig. S22†). In addition, the catalytic processes of Gd6Cu24-IM and Gd6Cu24-AC showed low solvent kinetic isotope effects of 1.23 and 1.25, respectively, (KIE = kH2O/kD2O, Fig. S23†),25 significantly different from the hydrophilic nucleophilic attack mechanism of mononuclear catalysts but consistent with the intramolecular O–O bond formation route for Cu3 catalysts.26
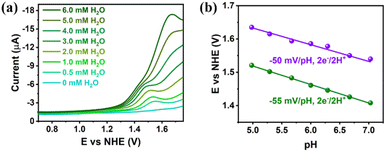 |
| Fig. 4 (a) DPVs of Gd6Cu24-AC (0.25 mM) in CH3CN with Et4NClO4 (0.1 M) before and after addition of different amounts of H2O. (b) Pourbaix diagram of Gd6Cu24-AC (0.25 mM) in NaAc/HAc (0.5 M, pH 4.98–7.03) buffer solution. Ep values are cited rather than E1/2 due to chemical irreversibility. | |
Based on the typical mechanisms reported for natural OEC-catalyzed water oxidation,12b,27 it was inferred that the two molecules of water (W1 and W2) that coordinated on the heterometallic centers CuII and GdIII can be used as the oxygen source for O–O bond formation (Fig. S24†). W1 and W2 had a distance of 2.81 Å, close to the distance between the two water molecules coordinated on CaII and MnIII in the OEC (3.26 Å).28 Based on the experimental results of previous studies and previously reported catalytic mechanisms for water oxidation by multinuclear molecular catalysts,6e,13d a plausible and reasonable mechanism for water oxidation catalyzed by Gd6Cu24 was proposed (Fig. 5). Under electrolytic catalytic conditions, the trimetallic reaction site is oxidized from the initial GdIII(OH2)CuIII2(OH2) species 1 through a 2e−/2H+ PCET process to give GdIII(OH)CuIII2(OH) species 2, which can be further oxidized by the 2e−/2H+ PCET process to GdIII(O˙)CuIII2(O˙) species 3. The redox potentials of these two steps were 1.35 and 1.55 V, respectively. Species 3 forms species 4, containing O–O bonds through intramolecular interactions, while species 4 releases O2 and completes the cycle by coordinating with two water molecules to form starting cluster 1. The M3–O2 intermediate is stabilized by the trimetallic site of GdIIICuIII2, which may facilitate the formation of O–O bonds. This pathway differs from the common mechanism of O–O bond formation by the nucleophilic water attack on the highly oxidized state of Mn+
O. The formation of the highly oxidized state of CuIV
O and ligand hydrocarbon oxidation are also avoided.29
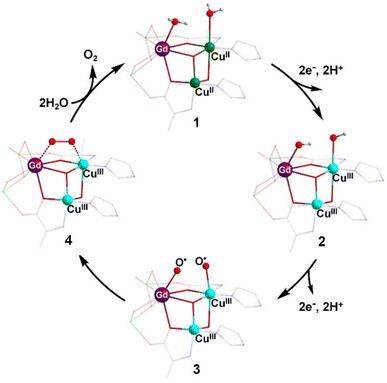 |
| Fig. 5 Proposed catalytic mechanism for the water oxidation based on Gd6Cu24-IM. Color codes: Gd, purple; CuII, green; CuIII, turquoise; H, white; C, gray; N, blue; O, red. | |
Conclusions
In conclusion, this study reported an efficient catalytic water oxidation using water-soluble Gd6Cu24 clusters in aqueous solutions of weak acids. The trimetallic catalytic site synergistically catalyzed water oxidation by promoting O–O bond formation, while simple amino acid ligands provided both water solubility and stability, which assisted the catalytic process. Interestingly, the TOFs of Gd6Cu24-IM and Gd6Cu24-AC at 1.7 V reached 319 and 169 s−1, respectively. This work offers a possibility to understand the synergistic effect of multiple metals in the water oxidation mechanism. Furthermore, Gd6Cu24 has a tunable molecular structure and metal sites, which is beneficial for designing highly active and stable catalysts in the future.
Data availability
The crystal data and structure of the clusters, experimental details and experimental data for this article are available in the ESI.†
Author contributions
J.-N. C., Z.-H. P. and X.-J. K. conceived and designed the research; J.-N. C. and Z.-H. P. synthesized and characterized the compounds. X.-J. K., J.-N. C., Z.-H. P., Q.-H. Q., C. W., L.-S. L., and L.-S. Z. analyzed the data. J.-N. C., Z.-H. P. and X.-J. K. wrote the manuscript with contributions from all authors. All authors analyzed the data and commented on the manuscript. J.-N. C. and Z.-H. P. contributed equally to this work.
Conflicts of interest
The authors declare no competing financial interest.
Acknowledgements
We gratefully acknowledge the financial support from the National Natural Science Foundation of China (Grant No. 92161104, 92161203, and 21721001).
Notes and references
-
(a) T. J. Meyer, Nature, 2008, 451, 778–779 CrossRef CAS PubMed;
(b) A. Cho, Science, 2010, 329, 786–787 CrossRef CAS PubMed;
(c) N. S. Lewis, Science, 2016, 351, aad1920 CrossRef PubMed.
-
(a) M. D. Kärkäs, O. Verho, E. V. Johnston and B. Åkermark, Chem. Rev., 2014, 114, 11863–12001 CrossRef PubMed;
(b) X.-P. Zhang, A. Chandra, Y.-M. Lee, R. Cao, K. Ray and W. Nam, Chem. Soc. Rev., 2021, 50, 4804–4811 RSC;
(c) B. Zhang and L. Sun, Chem. Soc. Rev., 2019, 48, 2216–2264 RSC;
(d) X.-P. Zhang, H.-Y. Wang, H. Zheng, W. Zhang and R. Cao, Chin. J. Catal., 2021, 42, 1253–1268 CrossRef CAS.
-
(a) N. Nelson and A. Ben-Shem, Nat. Rev. Mol. Cell Biol., 2004, 5, 971–982 CrossRef CAS PubMed;
(b) Y. Umena, K. Kawakami, J.-R. Shen and N. Kamiya, Nature, 2011, 473, 55–60 CrossRef CAS PubMed.
-
(a) M. Kondo, H. Tatewaki and S. Masaoka, Chem. Soc. Rev., 2021, 50, 6790–6831 RSC;
(b) J. Li, C. A. Triana, W. Wan, D. P. A. Saseendran, Y. Zhao, S. E. Balaghi, S. Heidari and G. R. Patzke, Chem. Soc. Rev., 2021, 50, 2444–2485 RSC;
(c) R. Matheu, P. Garrido-Barros, M. Gil-Sepulcre, M. Z. Ertem, X. Sala, C. Gimbert-Suriñach and A. Llobet, Nat. Rev. Chem., 2019, 3, 331–341 CrossRef CAS.
-
(a) S. Paul, F. Neese and D. A. Pantazis, Green Chem., 2017, 19, 2309–2325 RSC;
(b) Q.-F. Chen, Y.-H. Guo, Y.-H. Yu and M.-T. Zhang, Coord. Chem. Rev., 2021, 448, 214164 CrossRef CAS;
(c) M. Okamura, M. Kondo, R. Kuga, Y. Kurashige, T. Yanai, S. Hayami, V. K. K. Praneeth, M. Yoshida, K. Yoneda, S. Kawata and S. Masaoka, Nature, 2016, 530, 465–468 CrossRef CAS PubMed.
-
(a) L. Sun, Science, 2015, 348, 635–636 CrossRef CAS PubMed;
(b) B. Gerey, E. Gouré, J. Fortage, J. Pécaut and M.-N. Collomb, Coord. Chem. Rev., 2016, 319, 1–24 CrossRef CAS;
(c) R. Yao, Y. Li, Y. Chen, B. Xu, C. Chen and C. Zhang, J. Am. Chem. Soc., 2021, 143, 17360–17365 CrossRef CAS PubMed;
(d) F. Evangelisti, R. Güttinger, R. Moré, S. Luber and G. R. Patzke, J. Am. Chem. Soc., 2013, 135, 18734–18737 CrossRef CAS PubMed;
(e) X. Jiang, J. Li, B. Yang, X.-Z. Wei, B.-W. Dong, Y. Kao, M.-Y. Huang, C.-H. Tung and L.-Z. Wu, Angew. Chem. Int. Ed., 2018, 57, 7850–7854 (
Angew. Chem.
, 2018
, 130
, 7976–7980
) CrossRef CAS PubMed;
(f) C. Chen, Y. Chen, R. Yao, Y. Li and C. Zhang, Angew. Chem. Int. Ed., 2019, 58, 3939–3942 (
Angew. Chem.
, 2019
, 131
, 3979–3982
) CrossRef CAS PubMed;
(g) W.-S. Gao, J.-M. Wang, N.-N. Shi, C.-N. Chen, Y.-H. Fan and M. Wang, New J. Chem., 2019, 43, 4640–4647 RSC.
-
(a) J. Wang, M. Feng, M. N. Akhtar and M.-L. Tong, Coord. Chem. Rev., 2019, 387, 129–153 CrossRef CAS;
(b) R. Chen, Z.-H. Yan and X.-J. Kong, ChemPhotoChem, 2020, 4, 157–167 CrossRef CAS.
-
(a) M. Gil-Sepulcre and A. Llobet, Nat. Catal., 2022, 5, 79–82 CrossRef CAS;
(b) G. Maayan, N. Gluz and G. Christou, Nat. Catal., 2018, 1, 48–54 CrossRef CAS.
-
(a) T. Ghosh and G. Maayan, Angew. Chem. Int. Ed., 2019, 58, 2785–2790 (
Angew. Chem.
, 2019
, 131
, 2811–2816
) CrossRef CAS PubMed;
(b) A. K. Poulsen, A. Rompel and C. J. McKenzie, Angew. Chem. Int. Ed., 2005, 44, 6916–6920 (
Angew. Chem.
, 2005
, 117
, 7076–7080
) CrossRef CAS PubMed;
(c) M. D. Kärkäs and B. Åkermark, Dalton Trans., 2016, 45, 14421–14461 RSC.
-
(a) X.-J. Su, M. Gao, L. Jiao, R.-Z. Liao, P. E. M. Siegbahn, J.-P. Cheng and M.-T. Zhang, Angew. Chem. Int. Ed., 2015, 54, 4909–4914 (
Angew. Chem.
, 2015
, 127
, 4991–4996
) CrossRef CAS PubMed;
(b) S. J. Koepke, K. M. Light, P. E. VanNatta, K. M. Wiley and M. T. Kieber-Emmons, J. Am. Chem. Soc., 2017, 139, 8586–8600 CrossRef CAS PubMed;
(c) Q.-Q. Hu, X.-J. Su and M.-T. Zhang, Inorg. Chem., 2018, 57, 10481–10484 CrossRef CAS PubMed.
-
(a) C. F. Yocum, Coord. Chem. Rev., 2008, 252, 296–305 CrossRef CAS;
(b) V. Krewald, F. Neese and D. A. Pantazis, Phys. Chem., 2016, 18, 10739–10750 CAS;
(c) K. Saito, M. Nakagawa, M. Mandal and H. Ishikita, Photosynth. Res., 2021, 148, 153–159 CrossRef CAS PubMed.
-
(a) C. Chen, Y. Li, G. Zhao, R. Yao and C. Zhang, ChemSusChem, 2017, 10, 4403–4408 CrossRef CAS PubMed;
(b) Y. Li, R. Yao, Y. Chen, B. Xu, C. Chen and C. Zhang, Catalysts, 2020, 10, 185 CrossRef CAS.
-
(a) F. Evangelisti, R. Moré, F. Hodel, S. Luber and G. R. Patzke, J. Am. Chem. Soc., 2015, 137, 11076–11084 CrossRef CAS PubMed;
(b) D.-F. Lu, X.-J. Kong, T.-B. Lu, L.-S. Long and L.-S. Zheng, Inorg. Chem., 2017, 56, 1057–1060 CrossRef CAS PubMed;
(c) R. Chen, G.-L. Zhuang, Z.-Y. Wang, Y.-J. Gao, Z. Li, C. Wang, Y. Zhou, M.-H. Du, S. Zeng, L.-S. Long, X.-J. Kong and L.-S. Zheng, Natl. Sci. Rev., 2021, 8, nwaa234 CrossRef CAS PubMed;
(d) R. Chen, C.-L. Chen, M.-H. Du, X. Wang, C. Wang, L.-S. Long, X.-J. Kong and L.-S. Zheng, Chem. Commun., 2021, 57, 3611–3614 RSC.
-
(a) X.-Y. Zheng, X.-J. Kong, Z. Zheng, L.-S. Long and L.-S. Zheng, Acc. Chem. Res., 2018, 51, 517–525 CrossRef CAS PubMed;
(b) X.-Y. Zheng, J. Xie, X.-J. Kong, L.-S. Long and L.-S. Zheng, Coord. Chem. Rev., 2019, 378, 222–236 CrossRef CAS;
(c) Z.-H. Pan, Z.-Z. Weng, X.-J. Kong, L.-S. Long and L.-S. Zheng, Coord. Chem. Rev., 2022, 457, 214419 CrossRef CAS.
-
P. Ceroni, A. Credi and M. Venturi, in Analytical Methods in Supramolecular Chemistry, 2012, pp. 371–457 Search PubMed.
- C. E. Castillo, T. Stoll, M. Sandroni, R. Gueret, J. Fortage, M. Kayanuma, C. Daniel, F. Odobel, A. Deronzier and M.-N. Collomb, Inorg. Chem., 2018, 57, 11225–11239 CrossRef CAS PubMed.
- E. Laviron, J. Electroanal. Chem., 1979, 101, 19–28 CrossRef CAS.
-
M. McKinnon and J. Rochford, in Green Chem., ed. B. Török and T. Dransfield, Elsevier, 2018, p. 695–727 Search PubMed.
- Z.-H. Pan, Y.-W. Tao, Q.-F. He, Q.-Y. Wu, L.-P. Cheng, Z.-H. Wei, J.-H. Wu, J.-Q. Lin, D. Sun, Q.-C. Zhang, D. Tian and G.-G. Luo, Chem.–Eur. J., 2018, 24, 8275–8280 CrossRef CAS PubMed.
- J. S. Pap, Ł. Szyrwiel, D. Srankó, Z. Kerner, B. Setner, Z. Szewczuk and W. Malinka, Chem. Commun., 2015, 51, 6322–6324 RSC.
- A. M. Geer, C. Musgrave III, C. Webber, R. J. Nielsen, B. A. McKeown, C. Liu, P. P. M. Schleker, P. Jakes, X. Jia, D. A. Dickie, J. Granwehr, S. Zhang, C. W. Machan, W. A. Goddard III and T. B. Gunnoe, ACS Catal., 2021, 11, 7223–7240 CrossRef CAS.
-
(a) S. M. Barnett, K. I. Goldberg and J. M. Mayer, Nat. Chem., 2012, 4, 498–502 CrossRef CAS PubMed;
(b) M. K. Coggins, M.-T. Zhang, Z. Chen, N. Song and T. J. Meyer, Angew. Chem. Int. Ed., 2014, 53, 12226–12230 (
Angew. Chem.
, 2014
, 126
, 12422–12426
) CrossRef CAS PubMed;
(c) H. Kuilya, N. Alam, D. Sarma, D. Choudhury and A. Kalita, Chem. Commun., 2019, 55, 5483–5486 RSC;
(d) K. J. Fisher, K. L. Materna, B. Q. Mercado, R. H. Crabtree and G. W. Brudvig, ACS Catal., 2017, 7, 3384–3387 CrossRef CAS;
(e) P. Garrido-Barros, D. Moonshiram, M. Gil-Sepulcre, P. Pelosin, C. Gimbert-Suriñach, J. Benet-Buchholz and A. Llobet, J. Am. Chem. Soc., 2020, 142, 17434–17446 CrossRef CAS PubMed;
(f) L.-L. Zhou, T. Fang, J.-P. Cao, Z.-H. Zhu, X.-T. Su and S.-Z. Zhan, J. Power Sources, 2015, 273, 298–304 CrossRef CAS.
- K. J. Lee, B. D. McCarthy and J. L. Dempsey, Chem. Soc. Rev., 2019, 48, 2927–2945 RSC.
- M. Schulze, V. Kunz, P. D. Frischmann and F. Würthner, Nat. Chem., 2016, 8, 576–583 CrossRef CAS PubMed.
- S. J. Edwards, A. V. Soudackov and S. Hammes-Schiffer, J. Phys. Chem. A, 2009, 113, 2117–2126 CrossRef CAS PubMed.
- Q.-F. Chen, Z.-Y. Cheng, R.-Z. Liao and M.-T. Zhang, J. Am. Chem. Soc., 2021, 143, 19761–19768 CrossRef CAS PubMed.
-
(a) J. Barber, Nat. Plants, 2017, 3, 17041 CrossRef CAS PubMed;
(b) K. Kawashima, T. Takaoka, H. Kimura, K. Saito and H. Ishikita, Nat. Commun., 2018, 9, 1247 CrossRef PubMed;
(c) P. E. M. Siegbahn, Biochim. Biophys. Acta, Bioenerg., 2013, 1827, 1003–1019 CrossRef CAS PubMed;
(d) B. Zhang and L. Sun, ChemSusChem, 2019, 12, 3401–3404 CrossRef CAS PubMed.
- M. Suga, F. Akita, K. Hirata, G. Ueno, H. Murakami, Y. Nakajima, T. Shimizu, K. Yamashita, M. Yamamoto, H. Ago and J.-R. Shen, Nature, 2015, 517, 99–103 CrossRef CAS PubMed.
- A. Conde, L. Vilella, D. Balcells, M. M. Díaz-Requejo, A. Lledós and P. J. Pérez, J. Am. Chem. Soc., 2013, 135, 3887–3896 CrossRef CAS PubMed.
Footnotes |
† Electronic supplementary information (ESI) available: The crystal data and structure of the clusters, experimental details and experimental data. CCDC 2244617 and 2244618. For ESI and crystallographic data in CIF or other electronic format see DOI: https://doi.org/10.1039/d3sc05849b |
‡ These authors contributed equally to this work. |
|
This journal is © The Royal Society of Chemistry 2024 |