DOI:
10.1039/D3SC05014A
(Edge Article)
Chem. Sci., 2024,
15, 720-725
Primary activation of para-quinone methides by chiral phosphoric acid for enantioselective construction of tetraarylmethanes†
Received
24th September 2023
, Accepted 21st November 2023
First published on 2nd December 2023
Abstract
Demonstrated here is an asymmetric nucleophilic addition via primary activation of para-quinone methides (p-QMs) based on a chiral phosphoric acid catalytic system. In sharp contrast to previous CPA-based bifunctional activation processes that all required the nucleophiles to have an effective hydrogen bond donor unit (e.g., OH, NH), here no such unit is required in the nucleophile. N-protected indole nucleophiles were successfully utilized for the synthesis of chiral tetraarylmethanes with high efficiency and enantioselectivity under mild conditions. Therefore, this protocol significantly expanded the scope of asymmetric transformations of p-QMs.
Introduction
Para-Quinone methides (p-QMs) are versatile intermediates in organic synthesis and biological processes.1 Recently, they have been demonstrated to be particularly powerful in asymmetric synthesis,2 especially for the construction of quaternary stereogenic centers.3 Their strong tendency for aromatization permits the catalytic asymmetric 1,6-conjugate nucleophilic addition to the remote methide position, enabling rapid construction of a range of a benzylic stereogenic centers.4,5 While a range of catalytic asymmetric systems have been reported for these reactions, many of them required the presynthesis of those generally unstable QMs and thus limited the utility of such reactions.4 In 2015, we reported the first example of using chiral phosphoric acids (CPAs) as catalysts for the asymmetric nucleophilic addition to p-QMs (Scheme 1a).5a Notably, this catalytic system does not require the presynthesis of p-QMs, as it allows the in situ generation of QMs from the corresponding stable, racemic benzylic alcohols and subsequent asymmetric addition to take place in a one-pot fashion. Consequently, this protocol has been widely utilized since then.6
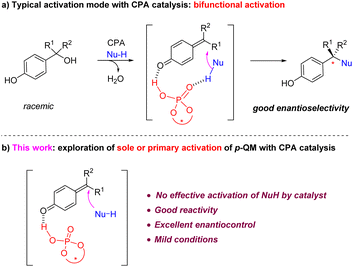 |
| Scheme 1 Modes of activation in CPA-catalyzed asymmetric addition to p-QMs. | |
However, despite the broad utility of CPA catalysts7 in p-QM chemistry,4–6 it is worth noting that, due to the typically long distance between the carbonyl activation site and the nucleophilic addition site, so far all the CPA-catalyzed p-QM addition reactions took advantage of the bifunctional activation mode, i.e., the CPA catalyst not only activates the QM carbonyl, but also has an effective hydrogen bonding interaction with the nucleophile partner (Scheme 1a).4h,5,6a–k This hydrogen bond interaction with the nucleophile not only helps achieve a tight enantiodetermining transition state, thereby facilitating enantiocontrol, but also increases the nucleophilicity of the nucleophile and reduces the reaction barrier. However, to the best of our knowledge, an efficient example of sole or primary activation of p-QM but without effective activation of the nucleophile by the CPA catalyst has not been demonstrated.8 Herein we report a highly efficient example of this type (Scheme 1b).
Nucleophiles currently widely used CPA-catalyzed asymmetric p-QM addition reactions include those with an N–H functionality (e.g., amines, indoles, pyrroles)5,6h–k or an O–H functionality (e.g., naphthols, carboxylic acids, 1,3-dicarbonyl compounds with enol tautomer).6a,b,d,e These functionalities are well-known hydrogen bond donors. In many of these reactions, control experiments by protecting these free N–H or O–H functionalities normally led to either dramatically low reactivity or low enantioselectivity, which confirmed the key role of their involvement in the secondary hydrogen bond interaction.
For example, in 2020, we reported the first catalytic asymmetric synthesis of chiral tetraarylmethanes via conjugate addition of pyrroles to in situ generated p-QMs bearing two aryl groups at the methide position.6h The unique three-dimensional structure together with their interesting biological activities promoted us to further develop new reactions for the synthesis of their analogues.6j,9 In particular, indoles are extremely versatile pharmacophores in drugs.10 However, indoles with a free N–H motif were not generally effective nucleophiles in this process. Therefore, we employed this reaction as an example to examine the possibility of sole or primary activation of p-QMs, which means the use of N-protected indoles to achieve high enantioselectivity in the absence of the secondary hydrogen bond interaction.
Results and discussion
The initial study was carried out with racemic triarylmethanol 1a as the model substrate and N-methylindole 2a as the nucleophile. In the presence of 10 mol% of CPA (R)-A1, the desired tetraarylmethane 3aa was smoothly obtained, with encouraging conversion and enantioselectivity (56% ee). It is worth noting that the reaction of 1a with free indole (without substitution in the N−1 position) only resulted in 12% ee. It is also notable that this reaction was clean and the remaining substrate accounted for the remainder of mass balance. Encouraged by the initial results, we performed further optimization aiming to improve the enantiocontrol for this challenging situation. Various CPAs were evaluated (Table 1, entries 2–6). The steric hindrance of the 3,3′-substituents seemed to be crucial for enantiocontrol, as catalysts A1 (ref. 11) and A2,12 bearing the bulky 2,4,6-triisopropylphenyl or 2,4,6-tricyclohexylphenyl groups, respectively, could give similar enantioselectivity, but the less hindered A3 and A4 could only give moderate enantiocontrol. Along these lines, we utilized a more hindered CPA A5 bearing 2,6-diisopropyl-4-adamantylphenyl groups in the 3,3′-positions,13 which proved superior and gave the best enantiocontrol (80% ee, Table 1, entry 5), though with moderate conversion. Extending the reaction time did not significantly enhance the reaction conversion. Then, our attention was turned to solvent screening (Table 1, entries 6–10). Toluene proved to be the optimal choice (97% conversion, 92% ee). After systematic optimization of reaction parameters, including concentration, nucleophile equivalence, and temperature, we were able to achieve 99% conversion and 92% ee, which were determined to be the standard reaction conditions (Table 1, entry 11).
Table 1 Evaluation of reaction conditionsa
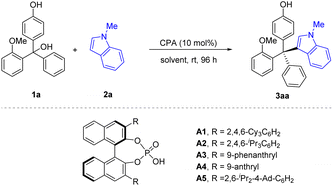
|
Entry |
CPA |
Solvent |
Conv.b (%) |
eec (%) |
Reaction conditions: 1a (0.1 mmol), N-methylindole (0.2 mmol), CPA (10 mol%), solvent (1 mL), rt, 96 h.
Conversion was determined by 1H NMR.
The ee value was determined by chiral HPLC.
N-methylindole (1.5 equiv.), toluene (0.5 mL), 72 h. NR = no reaction.
|
1 |
(R)-A1 |
DCE |
48 |
56 |
2 |
(R)-A2 |
DCE |
41 |
55 |
3 |
(R)-A3 |
DCE |
92 |
16 |
4 |
(R)-A4 |
DCE |
55 |
27 |
5 |
(R)-A5 |
DCE |
58 |
80 |
6 |
(R)-A5 |
DCM |
41 |
60 |
7 |
(R)-A5 |
Toluene |
97 |
92 |
8 |
(R)-A5 |
CHCl3 |
65 |
91 |
9 |
(R)-A5 |
1,4-Dioxane |
NR |
— |
10 |
(R)-A5 |
CH3CN |
90 |
25 |
11d |
(R)-A5 |
Toluene |
99 |
92 |
Next, we proceeded to examine the nucleophile scope regarding N-protected indoles under the standard conditions (Scheme 2). Substitution with various electron-withdrawing or electron-donating substitutes at the 5 or 6 position on the N-methyl indole skeleton successfully reacted to form the corresponding tetraarylmethane products in essentially quantitative yields and good to excellent enantioselectivities (80–94% ee, Scheme 2, 3ab – 3ah). In addition to the methyl group in the N−1 position, we also examined the PMB group, which maintained a high ee value, but at a reduced reaction rate.
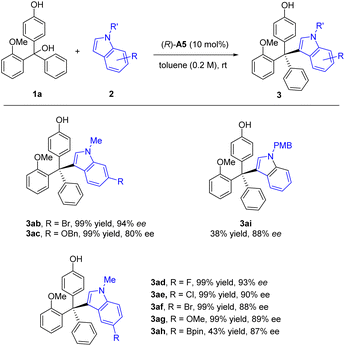 |
| Scheme 2 Scope of N-protected indoles 2. Standard conditions: 1a (0.3 mmol), N-protected indole 2 (0.45 mmol), CPA (10 mol%), solvent (1.5 mL), rt, 1–7 days. Isolated yield. The ee value was determined by chiral HPLC. | |
We then investigated the reaction scope with various substituted triarylmethanols (Scheme 3). Different ortho-alkoxyl groups, including the methoxylmethoxyl (MOMO) group, in one of the aryl groups could serve as the hydrogen bond acceptor for the differentiation between the two aryl substituents on the methide carbon of the in situ generated QM intermediates. The corresponding tetraarylmethane products were all obtained with high ee values (3aa–3da). Electron-donating substituents on the aryl group resulted in diminished enantioselectivity (3ea–3ha). However, electron-withdrawing groups were found to be beneficial to enantiocontrol (3ia–3la). The effect of substitutes on the 2-methoxy-substituted phenyl ring was also explored. The corresponding products 3ma–3oa could be synthesized smoothly with good enantioselectivities (86–91% ee). Moreover, 5-methoxyl-1-methyl-1H-indole 2g could also serve as a good nucleophile, leading to a range of substrates with diverse substituents in different positions (3dg–3tg). The low yields of 3ga and 3na were due to relatively slow conversion, but the reactions were clean themselves. Notably, in all these cases, the indole nucleophiles lack an obvious hydrogen bond donor motif, which means the CPA catalyst primarily activates the p-QM for the reactivity.
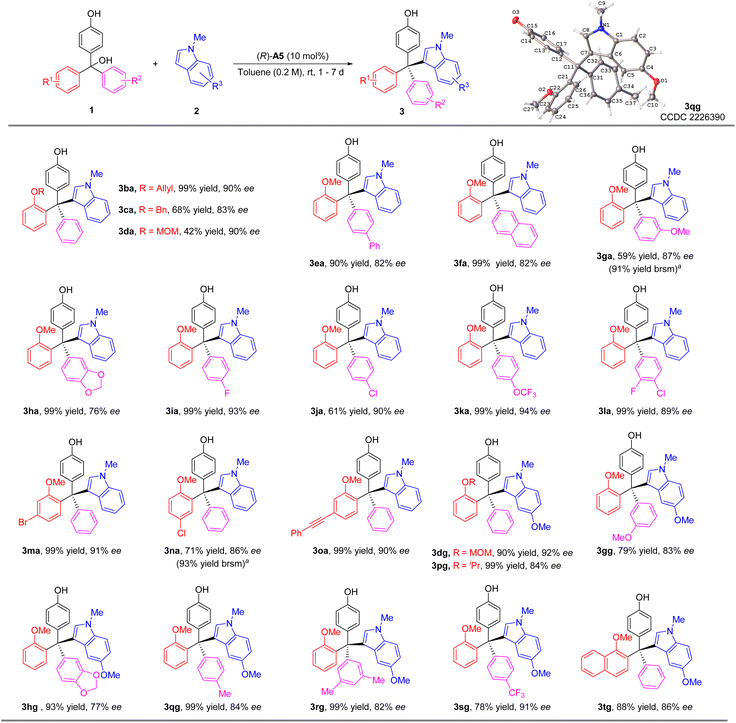 |
| Scheme 3 Scope of triarylmethanols 1. Standard conditions: 1a (0.3 mmol), N-protected indole 2 (0.45 mmol), CPA (10 mol%), solvent (1.5 mL), rt, 1–7 days. Isolated yield. The ee value was determined by chiral HPLC. aYield in parentheses was based on recovered starting material. | |
To gain insight into the mechanism, we monitored the ee value of the substrate and product in the standard reaction of 1a and 2a. The ee value of 3aa was essentially constant throughout the reaction. The remained starting material 1a was kept racemic (Fig. 1). These results are consistent with the involvement of a p-QM intermediate and no kinetic resolution of the substrate.
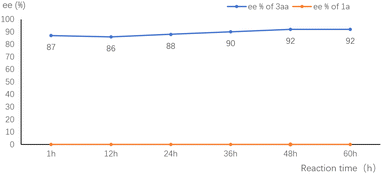 |
| Fig. 1 ee value–time plot of 2a and 3aa in the model reaction. | |
To obtain more information about the key intermediate, we carried out several control experiments (Scheme 4). Firstly, upon protection of the free OH group in the substrate, the reaction of 4a was completely shut down, suggesting that p-QM is likely the key intermediate. The importance of the hydrogen bond acceptor at the ortho-position was also investigated by employing substrates 4b and 4c bearing a methoxylmethyl group and an ethyl group on the ortho-position, respectively. These substrates also didn't show reactivity, highlighting the role of the appropriate directing group in this catalytic system. We also evaluated the substrates 4d and 4e, in which the methoxy group was installed on the meta and para position, respectively. Although both transformations proceeded efficiently, the enantiocontrol was poor in both cases. Interestingly, the ortho-fluorine group could induce enantiocontrol effectively. The desired product 4fa was obtained in 99% yield and 82% ee, demonstrating the same role of fluorine as that of the alkoxyl group.
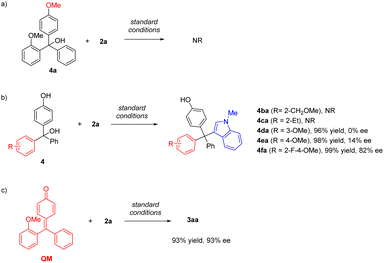 |
| Scheme 4 (a) Control experiment with a methyl-protected substrate. (b) Control experiments with different hydrogen bond acceptors. | |
DFT studies were performed to gain more insights into the enantiodetermining transition states (Fig. 2). For the sake of computational cost, we employed (R)-A2 (Table 1) as the model catalyst. Based on the computed transition state structures (TS-S and TS-R), the CPA activation on the p-QM intermediate is so strong that almost complete protonation of the carbonyl group can be observed. In contrast, there is no effective activation of the indole nucleophile by the CPA catalyst, which is consistent with our initial proposal. This is also in contrast to previous asymmetric nucleophilic additions to p-QMs by CPA catalysis, where strong nucleophile-CPA hydrogen bonding was typically observed. Moreover, during C–C bond formation, the hydrogen atom in the indole 3-position has interaction with the ortho-OMe directing group, and this interaction is more effective in TS-S than that in TS-R. Indeed, TS-R suffers great steric repulsion between the methoxy, phenyl group of 1a and the CPA catalyst, but there is no such steric clash in TS-S. These factors are probably responsible for the higher computed energy of TS-R than TS-S (by 0.8 kcal mol−1), which is in qualitative agreement with the experimental outcome.
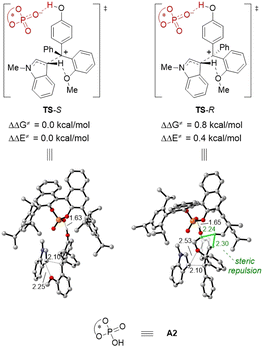 |
| Fig. 2 DFT-optimized enantiodetermining transition states for the reaction of 1a and 2a using A2 as a model catalyst. ΔΔG≠ and ΔΔE≠ are relative activation energies. The key values of the geometry structure are given in Å. | |
To further explore the versatility of our method, we selected the chiral product 3ka as an example for derivatization. The phenolic hydroxyl group was readily transformed to a triflate group, which could be further modified into various functional groups. Under mild conditions, the triflate group of 3ka′ could be removed using Pd/C catalyzed reduction conditions, yielding a new tetraarylmethane 3ka′-1 in quantitative yield and 93% ee. Additionally, with nickel as catalyst, the triflate 3ka′ reacted with a Grignard reagent, PMPMgBr, resulting in the coupling product 3ka′-2 with a high retention of enantiomeric purity (Scheme 5).
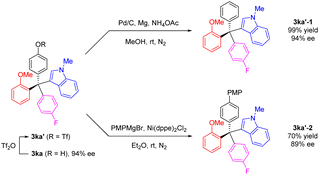 |
| Scheme 5 Transformations of indole-based tetraarylmethane 3ka. | |
Conclusions
In conclusion, we have demonstrated an example of primary activation of para-quinone methides for their asymmetric nucleophilic addition with the CPA catalytic system. This is in sharp contrast to those previous CPA-based bifunctional activation processes that all required the nucleophiles to have an effective hydrogen bond donor unit (e.g., OH, NH). In the present reaction, N-protected indole nucleophiles were proved to be capable of such reactions. Thus, a range of previously inaccessible tetraarylmethanes were synthesized with high efficiency and enantioselectivity under mild conditions. Therefore, this protocol also expanded the power of CPA catalysis as well as the versatility of p-QMs in asymmetric synthesis. Further expansion of p-QM chemistry by means of sole/primary activation is underway in our laboratory.
Data availability
Experimental procedures, spectral data and DFT data can be found in the ESI.†
Author contributions
Jianwei Sun and Zhengyu Han supervised the project and wrote the manuscript. Jianwei Sun conceived the project. Biao Zhu and Yu Zang performed condition optimization, scope study and product derivatizations. Chaoshen Zhang performed DFT studies. Xiu-Qin Dong and Hai Huang conducted scientific discussions. All the authors proofread and commented on the manuscript.
Conflicts of interest
There are no conflicts to declare.
Acknowledgements
This work was supported by the National Natural Science Foundation of China (NSFC, 22071210, 22101033, 22201023, and 22271242), the Jiangsu Key Laboratory of Advanced Catalytic Materials and Technology (BM2012110), the Natural Science Foundation of Jiangsu Province (BK20210849), the Jiangsu Province Key Laboratory of Fine Petrochemical Engineering (KF2102), the Innovation & Entrepreneurship Talents Plan of Jiangsu Province (JSSCRC2021536), and the Science, Technology and Innovation Committee of Shenzhen Municipality (JCYJ20200109141408054).
Notes and references
-
(a)
S. E. Rokita, Quinone Methides, John Wiley & Sons, Inc., New York, 2009, p. 456 CrossRef;
(b) N. J. Willis and C. D. Bray, Chem.–Eur. J., 2012, 18, 9160–9173 CrossRef CAS PubMed;
(c) S. Gnaim and D. Shabat, Acc. Chem. Res., 2014, 47, 2970–2984 CrossRef CAS PubMed;
(d) Q. Li, T. Dong, X. Liu, X. Zhang, X. Yang and X. Lei, Curr. Org. Chem., 2014, 18, 86–92 CrossRef CAS;
(e) M. S. Singh, A. Nagaraju, N. Anand and S. Chowdhury, RSC Adv., 2014, 4, 55924–55959 RSC.
- For selected reviews, see:
(a) L. Caruana, M. Fochi and L. Bernardi, Molecules, 2015, 20, 11733–11764 CrossRef CAS PubMed;
(b) A. Parra and M. Tortosa, ChemCatChem, 2015, 7, 1524–1526 CrossRef CAS;
(c) W. Li, X. Xu, P. Zhang and P. Li, Chem.–Asian J., 2018, 13, 2350–2359 CrossRef CAS PubMed;
(d) C. G. S. Lima, F. P. Pauli, D. C. S. Costa, A. S. de Souza, L. S. M. Forezi, V. F. Ferreira and F. de Carvalho da Silva, Eur. J. Org Chem., 2020, 2650–2692 CrossRef CAS;
(e) X. Li, Z. Li and J. Sun, Nat. Synth., 2020, 1, 426–438 CrossRef.
- For selected reviews and examples to construct quaternary stereogenic centers, see:
(a) B. Wang and Y.-Q. Tu, Acc. Chem. Res., 2011, 44, 1207–1222 CrossRef CAS PubMed;
(b) K. W. Quasdorf and L. E. Overman, Nature, 2014, 516, 181–191 CrossRef CAS PubMed;
(c) M. Buschleb, S. Dorich, S. Hanessian, D. Tao, K. B. Schenthal and L. E. Overman, Angew. Chem., Int. Ed., 2016, 55, 4156–4186 CrossRef PubMed;
(d) X. P. Zeng, Z. Y. Cao, Y. H. Wang, F. Zhou and J. Zhou, Chem. Rev., 2016, 116, 7330–7396 CrossRef CAS PubMed;
(e) P. Chen, M. J. Lv, J. K. Cheng, S. H. Xiang, X. Z. Ren, J. Zhang and B. Tan, Chem. Sci., 2023, 14, 2330–2335 RSC.
- For examples with pre-synthesized p-QMs, see:
(a) W. D. Chu, L. F. Zhang, X. Bao, X. H. Zhao, C. Zeng, J. Y. Du, G. B. Zhang, F. X. Wang, X. Y. Ma and C. A. Fan, Angew. Chem., Int. Ed., 2013, 52, 9229–9233 CrossRef CAS PubMed;
(b) L. Caruana, F. Kniep, T. K. Johansen, P. H. Poulsen and K. A. Jørgensen, J. Am. Chem. Soc., 2014, 136, 15929–15932 CrossRef CAS PubMed;
(c) Y. Lou, P. Cao, T. Jia, Y. Zhang, M. Wang and J. Liao, Angew. Chem., Int. Ed., 2015, 54, 12134–12138 CrossRef CAS PubMed;
(d) K. Zhao, Y. Zhi, T. Shu, A. Valkonen, K. Rissanen and D. Enders, Angew. Chem., Int. Ed., 2016, 55, 12104–12108 CrossRef CAS PubMed;
(e) K. Zhao, Y. Zhi, A. Wang and D. Enders, ACS Catal., 2016, 6, 657–660 CrossRef CAS;
(f) Y. H. Deng, X. Z. Zhang, K. Y. Yu, X. Yan, J. Y. Du, H. Huang and C. A. Fan, Chem. Commun., 2016, 52, 4183–4186 RSC;
(g) C. Ma, Y. Huang and Y. Zhao, ACS Catal., 2016, 6, 6408–6412 CrossRef CAS;
(h) N. Dong, Z. P. Zhang, X. S. Xue, X. Li and J. P. Cheng, Angew. Chem., Int. Ed., 2016, 55, 1460–1464 CrossRef CAS PubMed;
(i) S. Li, Y. Liu, B. Huang, T. Zhou, H. Tao, Y. Xiao, L. Liu and J. Zhang, ACS Catal., 2017, 7, 2805–2809 CrossRef CAS;
(j) S. Santra, A. Porey, B. Jana and J. Guin, Chem. Sci., 2018, 9, 6446–6450 RSC;
(k) Y. Lu, N. He, X. Miao and D. Wang, Org. Chem. Front., 2022, 9, 4840–4845 RSC;
(l) H. H. Li, Y. N. Meng, C. M. Chen, Y. Q. Wang, Z. X. Zhang, Z. Xu, B. Zhou and L. W. Ye, Sci. China: Chem., 2023, 66, 1467–1473 CrossRef CAS.
-
(a) Z. Wang, Y. F. Wong and J. Sun, Angew. Chem., Int. Ed., 2015, 54, 13711–13714 CrossRef CAS PubMed;
(b) Z. Wang, F. Ai, Z. Wang, W. Zhao, G. Zhu, Z. Lin and J. Sun, J. Am. Chem. Soc., 2015, 137, 383–389 CrossRef CAS PubMed.
- For selected examples of CPA-catalyzed in situ generated p-QMs, see:
(a) Y. F. Wong, Z. Wang and J. Sun, Org. Biomol. Chem., 2016, 14, 5751–5754 RSC;
(b) D. Qian, L. Wu, Z. Lin and J. Sun, Nat. Commun., 2017, 8, 567 CrossRef PubMed;
(c) M. Chen and J. Sun, Angew. Chem., Int. Ed., 2017, 56, 11966–11970 CrossRef CAS PubMed;
(d) W. Li, X. Xu, Y. Liu, H. Gao, Y. Cheng and P. Li, Org. Lett., 2018, 20, 1142–1145 CrossRef CAS PubMed;
(e) J. Yan, M. Chen, H. H. Y. Sung, I. D. Williams and J. Sun, Chem.–Asian J., 2018, 13, 2440–2444 CrossRef CAS PubMed;
(f) D. Ma, C. B. Miao and J. Sun, J. Am. Chem. Soc., 2019, 141, 13783–13787 CrossRef CAS PubMed;
(g) H. Wu, Q. Wang and J. Zhu, J. Am. Chem. Soc., 2019, 141, 11372–11377 CrossRef CAS PubMed;
(h) X. Li, M. Duan, Z. Deng, Q. Shao, M. Chen, G. Zhu, K. N. Houk and J. Sun, Nat. Catal., 2020, 3, 1010–1019 CrossRef CAS;
(i) Z. Wang, Y. Zhu, X. Pan, G. Wang and L. Liu, Angew. Chem., Int. Ed., 2020, 59, 3053–3057 CrossRef CAS PubMed;
(j) Z. Li, Y. Li, X. Li, M. Wu, M. L. He and J. Sun, Chem. Sci., 2021, 12, 11793–11798 RSC;
(k) Z. Han, Y. Zang, C. Liu, W. Guo, H. Huang and J. Sun, Chem. Commun., 2022, 58, 7128–7131 RSC ; For an example of PTC-catalyzed in situ generated p-QMs, see:;
(l) Y. J. Fan, L. Zhou and S. Li, Org. Chem. Front., 2018, 5, 1820–1824 RSC.
-
(a) T. Akiyama, J. Itoh, K. Yokota and K. Fuchibe, Angew. Chem., Int. Ed., 2004, 43, 1566–1568 CrossRef CAS PubMed;
(b) D. Uraguchi and M. Terada, J. Am. Chem. Soc., 2004, 126, 5356–5357 CrossRef CAS PubMed;
(c) G. Adair, S. Mukherjee and B. List, Aldrichimica Acta, 2008, 41, 31–39 CAS;
(d) S.-L. You, Q. Cai and M. Zeng, Chem. Soc. Rev., 2009, 38, 2190–2201 RSC;
(e) D. Kampen, C. M. Reisinger and B. List, Top. Curr. Chem., 2010, 291, 395–456 CrossRef CAS PubMed;
(f) M. Terada, Synthesis, 2010, 1929–1982 CrossRef CAS;
(g) J. Yu, F. Shi and L. Z. Gong, Acc. Chem. Res., 2011, 44, 1156–1171 CrossRef CAS PubMed;
(h) M. Rueping, A. Kuenkel and I. Atodiresei, Chem. Soc. Rev., 2011, 40, 4539–4549 RSC;
(i) M. Terada, Curr. Org. Chem., 2011, 15, 2227–2256 CrossRef CAS;
(j) I. Čorić, S. Vellalath, S. Müller, X. Cheng and B. List, Top. Organomet. Chem., 2013, 44, 165–194 CrossRef;
(k) D. Parmar, E. Sugiono, S. Raja and M. Rueping, Chem. Rev., 2014, 114, 9047–9153 CrossRef CAS PubMed;
(l) C. Zhu, K. Saito, M. Yamanaka and T. Akiyama, Acc. Chem. Res., 2015, 48, 388–398 CrossRef CAS PubMed;
(m) T. Akiyama and K. Mori, Chem. Rev., 2015, 115, 9277–9306 CrossRef CAS PubMed;
(n) T. James, M. van Gemmeren and B. List, Chem. Rev., 2015, 115, 9388–9409 CrossRef CAS PubMed.
- For examples of sole activation of other types of electrophiles by CPA, see:
(a) T. Akiyama, Y. Honma, J. Itoh and K. Fuchibe, Adv. Synth. Catal., 2008, 350, 399–402 CrossRef CAS;
(b) Y. Xie and B. List, Angew. Chem., Int. Ed., 2017, 56, 4936–4940 CrossRef CAS PubMed;
(c) Z. Wang and J. Sun, Org. Lett., 2017, 19, 2334–2337 CrossRef CAS PubMed , For examples of CPA activation of both nucleophile and electrophile, see Ref. 7..
-
(a) X. Tan, Z. Deng, Q. Wang, S. Chen, G. Zhu and J. Sun, Nat. Synth., 2023, 2, 275–285 Search PubMed , For another recent work by others on chiral tetraarylmethane synthesis, see:;
(b) M. Liu, B. Shen, C. Liu, P. Yu and P. Li, J. Am. Chem. Soc., 2023, 145, 14562–14569 CrossRef CAS PubMed.
-
(a) S. Schierle, C. Flauaus, P. Heitel, S. Willems, J. Schmidt, A. Kaiser, L. Weizel, T. Goebel, A. S. Kahnt, G. Geisslinger, D. Steinhilber, M. Wurglics, G. E. Rovati, A. Schmidtko, E. Proschak and D. Merk, J. Med. Chem., 2018, 61, 5758–5764 CrossRef CAS PubMed;
(b) V. Praveen Kumar, J. Renjitha, C. T. Fathimath Salfeena, K. T. Ashitha, R. S. Keri, S. Varughese and S. B. Somappa, Chem. Biol. Drug Des., 2017, 90, 703–708 CrossRef CAS PubMed;
(c) C. Zhang, Y. Qu and B. Niu, Bioorg. Med. Chem., 2016, 24, 5781–5786 CrossRef CAS PubMed;
(d) T. V. Sravanthi and S. L. Manju, Eur. J. Pharm. Sci., 2016, 91, 1–10 CrossRef CAS PubMed.
- S. Hoffmann, A. M. Seayad and B. List, Angew. Chem., Int. Ed., 2005, 44, 7424–7427 CrossRef CAS PubMed.
- W. Yang, Z. Wang and J. Sun, Angew. Chem., Int. Ed., 2016, 55, 6954–6958 CrossRef CAS PubMed.
- X. Cheng, S. Vellalath, R. Goddard and B. List, J. Am. Chem. Soc., 2008, 130, 15786–15787 CrossRef CAS PubMed.
Footnote |
† Electronic supplementary information (ESI) available: Experimental procedures and characterization for all new compounds. CCDC 2226390. For ESI and crystallographic data in CIF or other electronic format see DOI: https://doi.org/10.1039/d3sc05014a |
|
This journal is © The Royal Society of Chemistry 2024 |