DOI:
10.1039/D4RA07039A
(Paper)
RSC Adv., 2024,
14, 37697-37708
Chemical synthesis, characterization, and anticancer potential of CuO/ZrO2/TiO2/RGO nanocomposites against human breast (MCF-7) cancer cells
Received
30th September 2024
, Accepted 13th November 2024
First published on 25th November 2024
Abstract
Nanocomposites (NCs) have attractive potential applications in gas-sensing, energy, photocatalysis, and biomedicine. In the present work, the fabrication of CuO/ZrO2/TiO2/RGO nanocomposites (NCs) was done via a simple chemical route. Our aim in this work was to synthesis and investigate the selective anticancer activity of TiO2 NPs by supporting CuO, ZrO2, and RGO toward cancer and normal cells. Different analytical techniques, such as X-ray diffraction (XRD), high-resolution transmission electron microscopy (HRTEM), scanning electron microscopy (SEM) with energy dispersive X-ray (EDX), X-ray photoelectron spectroscopy (XPS), Raman spectroscopy, Fourier transform infrared (FTIR) spectroscopy, photoluminescence (PL) spectroscopy, and dynamic light scattering (DLS), were carefully applied to characterize the physicochemical properties of the produced samples. XRD results showed that the phase and crystal structure of TiO2 NPs were enhanced after adding CuO, ZrO2, and RGO. TEM and SEM images showed that CuO/ZrO2/TiO2/RGO NCs were similarly distributed on RGO sheets with high crystallinity, excellent quality of lattice fringes, and lower agglomeration compared with pure TiO2 NPs. EDX and XPS analysis confirmed the presence of elements Cu, Zr, Ti, O, and C in the obtained CuO/ZrO2/TiO2/RGO NCs. Raman and FTIR spectra verified the presence of functional groups and crystal structures in the produced samples. PL data showed that the optical properties of TiO2 improved after adding CuO, ZrO2, and RGO sheets owing to the reduction in the recombination rate between the electron–hole pair. DLS analysis showed that the prepared CuO/ZrO2/TiO2/RGO NCs had excellent colloidal stability and good distribution in the suspension of the media culture. Anticancer results for CuO/ZrO2/TiO2/RGO NCs exhibited about 2-fold higher toxicity for 24 h and 4-fold for 48 h against breast cancer (MCF-7) cells than pure TiO2 NPs, while their biocompatibility was excellent against HUVEC normal cells. Additionally, the IC50 values of CuO/ZrO2/TiO2/RGO NCs were 44.19 ± 1.2 μg mL−1 and 24.52 ± 0.8 μg mL−1 for 24 h and 48 h, respectively. These results indicate that adding CuO, ZrO2, and RGO plays a crucial role in enhancing the anticancer property of TiO2 NPs. This study suggests that CuO/ZrO2/TiO2/RGO NCs could be applied in cancer therapy applications in in vivo models.
1. Introduction
Due to their tailorable drug delivery, enhanced bioavailability, and low toxicity, nanomaterials have potential medical applications, including in cancer therapy and diagnostics.1,2 The high development cost, toxicity concerns, and targeting accuracy of some nanomaterials pose challenges in their application in medicine.3 To resolve these obstacles, common nanomaterials have excellent properties, such as low toxicity and high biocompatibility.4 Currently, titanium dioxide nanoparticles (TiO2 NPs) are important in potential applications in environmental remediation and medical fields owing to their excellent physicochemical properties.5,6 Despite these advantages, pure TiO2 NPs exhibit some limitations in cancer therapy.7 One major limitation of TiO2 NPs is their agglomeration due to their high surface energy, which reduces their colloidal stability and hampers their bioavailability in cellular environments.8 Previous studies have shown that the integration of other metal oxides, such as CeO2 NPs,9 SnO2 NPs,10 ZnO NPs,11 CuO NPs,12 ZrO2 NPs,13 Al2O3 NPs,14 and WO3 NPs,15 can improve their anticancer properties. Generally, nanocomposites (NPs) are a type of nanomaterials that are produced by the combination of two or multi-metal oxides to enhance their physicochemical properties for potential applications in photocatalytic and medical fields.16,17
To enhance the properties of metal oxide NPs, many researchers have focused on improving different approaches (chemical, physical, and biological) to synthesize novel nanocomposites (NCs), which are mixed with metal oxide NCs. Particularly, chemical approaches such as hydrothermal, sol–gel, co-precipitation, and thermal decomposition techniques have been applied to the synthesis of NCs due to their low cost and environmental friendliness.18–20 For example, Kubiak et al.21 prepared ZnO/TiO2, ZrO2/TiO2 and MoS2/TiO2 NCs using the hydrothermal method with enhanced antibacterial and photocatalytic activities compared to pure TiO2 NPs. Another study22 reported that the sol–gel method was used to synthesize SnO2/WO3 NCs with highly improved optical properties compared with WO3 NPs. Moreover, CuO/NiO/ZnO NCs have been synthesized via the co-precipitation process, and they show highly enhanced antibacterial activity compared to individual samples.23 Correspondingly, Marsooli et al.24 synthesized Fe2O4/CdWO4/PrVO2 NCs through the precipitation method, which exhibits excellent anticancer performance. Indumathi et al.25 prepared CuO/TiO2/chitosan-farnesol NCs by standard acetic acid synthesis to investigate antimicrobial and anticancer activities toward melanoma cancer cells.
Reduced graphene oxide (RGO) has attracted potential biomedical applications such as cancer therapy owing to its excellent characteristics.26 Numerous studies show that different metal oxide NPs combined with RGO can improve their synthesis and anticancer properties. For instance, Ahamed and Kadiyala et al.27,28 reported that ZrO2/RGO NCs have enhanced cytotoxicity against cancer cells while maintaining biocompatibility with normal cells. Similarly, Scotti et al.29 showed that ZnO/ZrO2/RGO NCs exhibit 3.5-fold higher anticancer efficacy against lung and breast cancer cells compared to ZnO NPs. Besides, our previous study30 investigated that Bi2O3-doped WO3/RGO NCs have enhanced anticancer activity and better biocompatibility than both NPs. The synthesized ZnO–TiO2/RGO NCs showed improved cytotoxicity towards cancer cells and better biocompatibility compared to normal cells.31,32 Askari et al.,33 investigated that Mn2O4/Co3O4/RGO NCs have a good ability to inhibit the growth of cancer cells.
The purpose of this work was to fabricate CuO/ZrO2/TiO2/RGO NCs to enhance the selective anticancer activity toward human cancer and normal cell lines. The prepared samples were further characterized by XRD, HRTEM, and SEM with EDX, XPS, Raman, FTIR, PL, and DLS techniques to envisage the enhanced physicochemical properties. In the present work, MTT assay was applied to assess the selective anticancer performance of the prepared samples against breast cancer cells and HUVEC normal cells for 24 h and 48 h.
2. Experimental
2.1 Chemicals and cells
Titanium butoxide Ti (OBu)4, zirconium nitrate pentahydrate (Zr(NO3)4·5H2O), Cu(NO3)2·6H2O, and sodium hydroxide (NaOH) were supplied by Sigma-Aldrich in St. Louis, MO, USA. Dulbecco's Modified Eagle Medium (DMEM), fetal bovine serum (FBS), MTT (3-(4,5-dimethylthiazol-2-yl)-2,5-diphenyltetrazolium bromide), and dimethyl sulfoxide (DMSO) were also obtained from Sigma-Aldrich in St. Louis, MO, USA. Human breast (MCF-7) cancer and Human Umbilical Vein Endothelial Cells (HUVEC) cells were bought from the American Type Culture Collection (ATTC) based in Manassas, WV, USA.
2.2 Preparation of CuO/ZrO2/TiO2 nanocomposites (NCs)
The preparation of CuO/ZrO2/TiO2 NCs (0.5
:
0.5
:
1 mol ratio) was achieved using a co-precipitation process. Firstly, 0.01 mol of Ti(OBu)4 was slowly added to 50 mL of absolute ethanol under stirring. Then, 0.005 mol of Zr(NO3)4·5H2O and 0.0025 mol of Cu(NO3)2·6H2O were further added into the above solution under stirring for 1 h at room temperature. Next, 1 M of NaOH was dropped slowly to the mixture solution with stirring at 65 °C for 4 h to obtain the precipitate. Subsequently, the obtained precipitate was filtered, washed with ethanol and water several times, and dried in an oven at 80 °C for 12 h. The calcination for the dried precipitate was carried out using an oven at 500 °C under air for 5 h to obtain CuO/ZrO2/TiO2 NCs as a nanopowder. Under the same protocols, pure TiO2 NPs were successfully prepared without using Zr(NO3)4·5H2O and Cu(NO3)2·6H2O. Similarly, ZrO2/TiO2 NCs were synthesized without using Zr(NO3)4·5H2O.
2.3 Preparation of CuO/ZrO2/TiO2/RGO nanocomposites (NCs)
The CuO/ZrO2/TiO2 NCs were successfully anchored onto RGO sheets via the sonication process. A specific amount of CuO/TiO2/ZrO3 NCs was suspended in 50 mL ethanol and distilled water (1
:
1) under ultrasonication for 1 h as the first solution. 10% of reduced graphene oxide (RGO) sheets were dissolved in 50 mL ethanol under stirring for 1 h as the second solution. Then, the two solutions were mixed and sonicated for 4 h at 80 kW. Next, the mixture solution was further dried at 60 °C for 24 h to obtain CuO/ZrO2/TiO2/RGO NCs.
2.4 Characterization methods
Different analytical techniques, such as XRD, HRTEM, SEM with EDX, XPS, Raman, FTIR, PL, and DLS, effectively characterized the physicochemical properties of the obtained NPs and NCs. Thus, the details of these techniques are mentioned in our previous works.34,35
2.5 Cell culture and maintenance
Human breast (MCF-7) cancer and HUVEC normal cells were cultured in DMEM supplemented with 10% fetal bovine serum (FBS), 1% penicillin–streptomycin, and 1% glutamine in the flask. The cultured cells were maintained at 37 °C in a humidified atmosphere with 5% CO2. Further details were done in our published studies.36,37
2.6 Anticancer and biocompatibility performance
In the present work, the evaluation of the anticancer activity of the prepared samples was estimated using the MTT assay as reported in our pervious study.34 Briefly, about 5 × 104 cells per well were seeded in a 96-well plate and incubated at 37 °C with 5% CO2. Then, the stock solution (3 mg/3 mL) of the prepared samples in DMEM was successfully prepared. A section of each sample was also diluted to different concentrations (0, 0.5, 1, 5, 10, 25, 50, and 100 μg mL−1). The next day, these different concentrations of each sample were added to the seeded cells in a 96-well plate with incubation at 37 °C with 5% CO2 for 24 h and 48 h. After the incubation period, the DMEM of each well without the control was removed. Then, 20 μL of MTT solution (5 mg mL−1) was added to each well and incubated for 4 h. Following this, 100 μL of DMSO formazan crystals formed by metabolically active cells were dissolved. The absorbance was measured at 570 nm using a microplate reader. The cell viability was calculated as a percentage relative to the untreated control cells, and the IC50 values (the concentration at which 50% of the cells are inhibited) were determined.
2.7 Data analysis
Data analysis in all experiments was done using one-way analysis of variance (ANOVA) as mean ± standard deviation. A p-value of <0.05 was considered statistically significant.
3. Results and discussion
3.1 XRD study
X-ray diffraction (XRD) analysis of the synthesized NPs and NCs was done to determine their phase and crystallinity. Fig. 1a–d illustrates the XRD spectra of the obtained pure TiO2 NPs, ZrO2/TiO2 NCs, CuO/ZrO2/TiO2 NCs, and CuO/ZrO2/TiO2/RGO NCs. The XRD spectra of pure TiO2 NPs (Fig. 1a) observed that the diffraction peaks were associated to 2θ values of 25.3°, 37.8°,48.0°, 55.1°, 62.9°, 70.0°, and 75.3° corresponding to the (101), (004), (200), (211), (204), (220), and (215) planes, respectively.38 These spectra reveal that pure TiO2 NPs have high crystallinity and purity without the presence of impurities. Hence, Fig. 1b exhibits new peaks at 30.3° and 50.4°, corresponding to the crystalline and phase of ZrO2, as reported in a preceding investigation.39 This indicates that the ZrO2/TiO2 NCs were successfully prepared with a change in their crystallinity. For CuO/ZrO2/TiO2 NCs (Fig. 1c), the XRD spectra display all peaks corresponding to CuO, ZrO2, and TiO2. Furthermore, the peaks at 35.5° and 38.7° confirmed the presence of the monoclinic phase of CuO in the synthesized CuO/ZrO2/TiO2 NCs.40 This phenomena reveals that there is a change in the crystal structure of ZrO2/TiO2 NCs after adding CuO. In addition, the XRD spectra of CuO/ZrO2/TiO2/RGO NCs (Fig. 1d) exhibit the peaks for all three metal oxides (CuO, ZrO2, and TiO2) along with a broad peak at 24°, signifying the amorphous nature of reduced graphene oxide (RGO). By the Scherrer equation,41 the average crystallite sizes of pure TiO2 NPs, ZrO2/TiO2 NPs, Cu/ZrO2/TiO2 NCs, and CuO/ZrO2/TiO2/RGO NCs were 10.1 ± 1.2 nm, 16.0 ± 1.5 nm, 50.3 ± 0.9 nm, and 52.1 ± 0.6 nm, respectively. XRD results showed that the supporting ZrO2, CuO, and RGO strongly contributed to improving the structural properties of TiO2, such as their crystallinity, phase purity, and increased crystallite size. This study highlighted that CuO/ZrO2/TiO2/RGO NCs could progress their performance in potential applications. Several earlier studies42–45 were matched with the obtained XRD results.
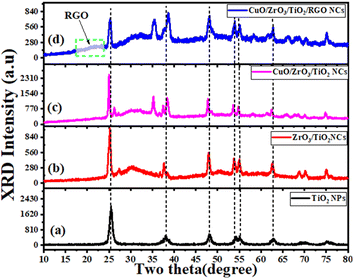 |
| Fig. 1 XRD pattern: (a) pure TiO2 NPs, (b) ZrO2/TiO2 NPs, (c) CuO/ZrO2/TiO2 NCs, and (d) CuO/ZrO2/TiO2/RGO NCs. | |
3.2 TEM study
Fig. 2A–I depicts the TEM, HRTEM images and SAED analysis of the produced pure TiO2 NPs, CuO/ZrO2/TiO2 NCs, and CuO/ZrO2/TiO2/RGO NCs. The TEM image of pure TiO2 NPs (Fig. 2A) displays that their morphology was spherical and uniformly distributed with high agglomeration, and the average particle size was 9.2 ± 1.9 nm, as reported in a recent study.46 Moreover, the HRTEM image (Fig. 2B) verifies the crystal structure of pure TiO2 NPs by calculating the d-spacing value of 0.365 nm, which matches the (101) planes.47 Likewise, the SAED pattern (Fig. 2C) exhibits distinct diffraction rings, further confirming the crystallinity of TiO2 in the anatase phase, which corresponds to the (101), (004), (200), and (211) planes. After the incorporation of CuO and ZrO2, as shown in Fig. 3D, the produced TiO2 NPs demonstrate a more heterogeneous morphology with the lowest agglomeration and particle size of about 46.9 ± 1.3 nm. The HRTEM image (Fig. 2E) reveals that the d-spacing values of TiO2 NPs, ZrO2, and CuO in CuO/ZrO2/TiO2 NCs were 0.337 nm, 0.266 nm, and 0.271 nm, corresponding to the (101), (111), and (002) planes, respectively, as matched with a previous study.48 Also, the SAED pattern (Fig. 2F) exhibits additional diffraction rings compared to that of pure TiO2(101), corresponding to CuO(111) and ZrO2(002). These phenomena indicate that the addition of CuO and ZrO2 could change the crystal structure of TiO2 NPs. Additionally, TEM images (Fig. 2G) confirmed that the synthesized CuO/ZrO2/TiO2 NCs were anchored onto RGO sheets with a slight reduction in the agglomeration compared to both pure TiO2 NPs and CuO/ZrO2/TiO2 NCs, as reported in some recent investigations. The average size of the particles was approximately 53.1 nm. However, the d-spacing for TiO2, ZrO2, and CuO was found be 0.277 nm, 0.29 nm, and 0.156 nm, respectively, as revealed in Fig. 2H. These values confirm the presence of all three components in the prepared CuO/ZrO2/TiO2/RGO NCs. The SAED pattern (Fig. 2I) shows distinct spots and rings indicative of a polycrystalline structure, confirming the presence of RGO and further supporting the homogeneity of the NCs. These TEM images suggest that supporting RGO on CuO/ZrO2/TiO2 NCs enhances the photocatalytic and anticancer performance. Similar studies49–52 were supported by the presented TEM results.
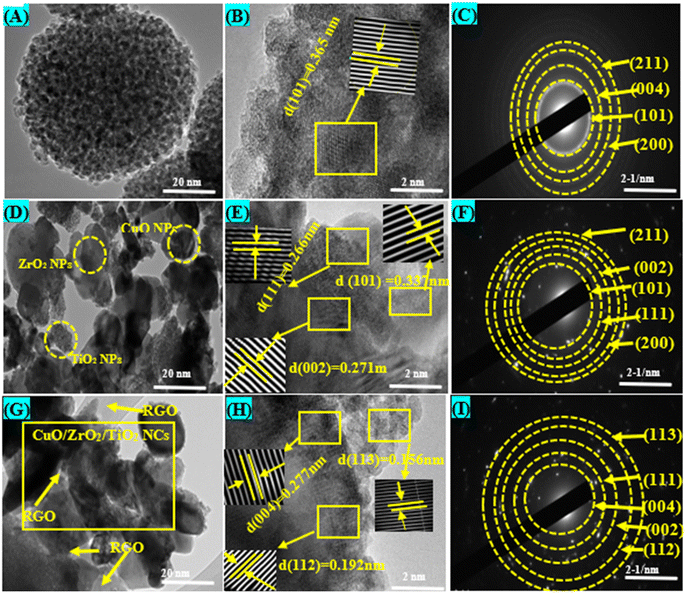 |
| Fig. 2 TEM and HR-TEM images with SAED analysis: (A–C) pure TiO2 NPs, (D–F) CuO/ZrO2/TiO2 NCs, and (G–I) CuO/ZrO2/TiO2/RGO NCs. | |
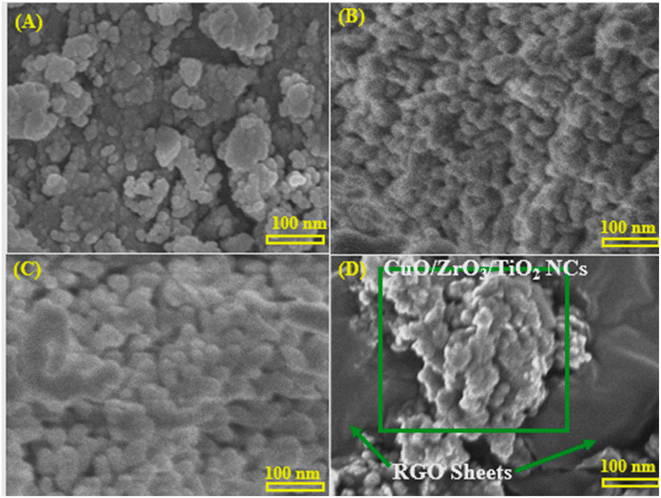 |
| Fig. 3 Scanning electron microscopy (SEM) images: (A) pure TiO2 NPs, (B) ZrO2/TiO2 NCs, (C) CuO/ZrO2/TiO2 NCs, and (D) CuO/ZrO2/TiO2/RGO NCs. | |
3.3 SEM and EDX with elemental mapping analysis
SEM analysis was achieved to investigate the morphologies of the prepared samples, as displayed in Fig. 3A–D. Pure TiO2 NPs (Fig. 3A) show an agglomerated morphology with densely packed spherical particles, in agreement with an earlier report.53 Consistently, ZrO2/TiO2 NCs (Fig. 3B) exhibit a more compact structure with more uniform distributions and increased particle aggregation. We observed that the addition of ZrO2 played a role in changing of the surface morphology and the particle sizes compared to the TEM image of pure TiO2 NPs, in agreement with an earlier study.54 This phenomenon confirms the successful reaction between ZrO2 and TiO2 NCs to produce ZrO2/TiO2 NCs. The prepared CuO/ZrO2/TiO2 NCs (Fig. 3C) show a rougher and more uniformly distributed surface, indicating enhanced particle interaction due to CuO inclusion.55 Fig. 3D confirms that the prepared CuO/ZrO2/TiO2 NPs were adhered onto RGO sheets, as reported in a previous study.56 These results suggest that CuO/ZrO2/TiO2/RGO NCs reveal well-dispersed NCs supported on RGO sheets, preventing aggregation and maximizing the effective surface area for biological applications.
The EDX and elemental mapping analysis of the synthesized CuO/ZrO2/TiO2/RGO NCs are presented in Fig. 4A–J. Hence, the EDX spectra (Fig. 4A) verified that the elemental compositions of the prepared sample are in agreement with their percentage in the synthesis process. Fig. 4B displays the electron image of the sample, which displays the surface morphology and distribution of NCs. The elemental mapping analysis (Fig. 4C–J) confirmed that the elements (Cu, Zr, Ti, O, and C) were present with highly uniform distribution in the obtained CuO/ZrO2/TiO2/RGO NCs. As shown in these results, the anchoring of CuO/ZrO2/TiO2 NCs onto the RGO sheet plays a role in enhancing their surface morphologies and structure for potential applications such as environmental remediation and therapy. These results are in excellent agreement with a previous study.57–59
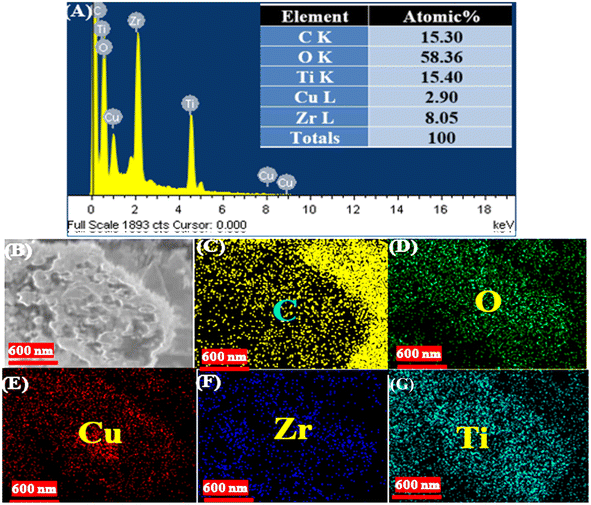 |
| Fig. 4 EDX with elemental mapping of the synthesized CuO/ZrO2/TiO2/RGO NCs: (A) EDX spectra, (B) electron image, (C) carbon (C), (D) oxygen (O), (E) copper (Cu), (F) zirconium (Zr), and (G) titanium (Ti). | |
3.4 XPS analysis
The evaluation of the chemical composition and the oxidation states of all elements in the prepared samples were further analyzed by XPS technique. Fig. 5A–F depicts the XPS spectra of the survey and the high-resolution spectra of Cu 2p, Zr 3d, Ti 2p, O 1s, and C 1s. Thus, the XPS spectra (Fig. 5A) of the survey exhibited the presence of all elements Cu, Zr, Ti, O, and C in the produced CuO/ZrO2/TiO2/RGO NCs with high purity, consistent with similar studies.60,61 In Fig. 5B, the high-resolution spectra of Cu CuO/ZrO2/TiO2/RGO NCs 2p (Fig. 5B) reveals two characteristic peaks at binding energies (B.E) of 935.5 eV and 955.8 eV corresponding to Cu 2p3/2 and Cu 2p1/2, respectively, in agreement with earlier studies.62,63 These peaks are related to the presence of Cu2+ in CuO, as shown through the energy difference of ∼20.3 eV between the two peaks. Also, the 940–949 eV range of B.E represents satellite peaks, which confirm the existence of Cu2+ species. From the XPS spectra of Zr 3d (Fig. 5C), the B.E of Zr 3d5/2 and Zr 3d3/2 was located at 183.3 eV and 185.7 eV, respectively. It can be shown that the Zr 3d doublet splitting of roughly 2.4 eV verifies the stability of Zr in its oxidation state.64 The XPS spectra of Ti 2p (Fig. 5D) displays two peaks, which are located at 459.6 eV and 465 eV, respectively. These peaks indicate that the oxidized Ti4+ species was present in TiO2 within the CuO/ZrO2/TiO2/RGO NCs.65 Furthermore, three distinct XPS peaks of O 1s were further assigned at 529.8 eV, 531.3 eV, and 532.5 eV, respectively. Besides, the peak at 529.8 eV is produced due to the lattice oxygen in the structure of CuO, ZrO2, and TiO2 NPs. The peaks at 531.3 eV and 532.5 eV were attributed to oxygen defect or hydroxyl group and chemisorbed water on the surface of the prepared NCs, respectively.66 On the other hand, the three main peaks at B.E of 283.8 eV, 285.3 eV, and 289.5 eV corresponded to the XPS spectra of C 1s (Fig. 5F). It can be observed that the sp2-hybridized carbon atoms (C
C) present in the RGO framework are assigned at 283.8 eV. The peaks at 285.2 eV and 289.5 eV are due to C–C and C–O bonds, respectively.67 Our XPS results indicate that the addition of CuO, ZrO2, and TiO2 NPs into RGO sheets exhibited distinct binding energies corresponding to the Cu2+, Zr4+, Ti4+, and O2− species in the presence of sp2-hybridized carbon in RGO. These binding energies of elements have a contribution in the enhanced catalytic or electronic properties of these NCs.
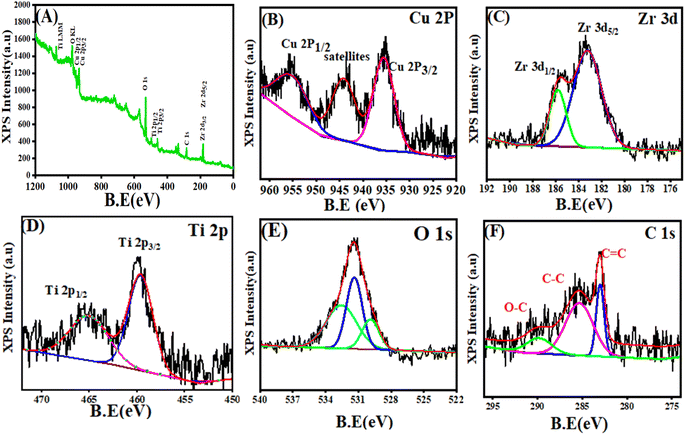 |
| Fig. 5 XPS spectra of CuO/ZrO2/TiO2/RGO NCs: (A) survey spectra, (B) Cu 2p, (C) Zr 3d, (D) Ti 2p, (E) O 1s, and (F) C 1s. | |
3.5 Raman analysis
The Raman spectra (Fig. 6A and B) display vibrational modes of the produced pure TiO2 NPs, ZrO2/TiO2 NCs, CuO/ZrO2/TiO2 NCs, and CuO/ZrO2/TiO2/RGO NCs. The Raman band of pure TiO2 (Fig. 6A(I)) were exhibited at 148.7 cm−1, 403.2 cm−1, 520.1 cm−1, and 644.8 cm−1 with high intensity.68 For ZrO2/TiO2 NCs (Fig. 6A(II)), the shift of the Raman bands at 146.1 cm−1, 400.6 cm−1, 520.1 cm−1, and 639.6 cm−1, respectively, was observed as agreed with previous studies.69,70 We observed that the Raman bands shift to a lower wavenumber. This shift indicates a change in the crystal structure of pure TiO2 NPs due to the addition of ZrO2. Upon the addition of CuO in Fig. 6A(III), new peaks appear at about 213 cm−1, characteristic of the CuO phase in a similar study.71 These results confirmed the successful integration of CuO into the ZrO2/TiO2 NCs. As shown in the above results, supporting TiO2 with CuO or ZrO2 can introduce defects and surface oxygen vacancies, causing shifts in the Raman peak positions. Fig. 6A and B(IV) display that the introduction of the RGO sheet modifies the Raman bands compared to that of pure TiO2. Furthermore, Fig. 6B(IV) shows the ratio (ID/IG = 0.97) between the Raman bands of the RGO sheet.72 In addition, a decrease in the intensity of the CuO/ZrO2/TiO2/RGO NCs peaks is observed, which may be attributed to the interaction between the CuO, ZrO2, and TiO2/RGO matrix and RGO. Raman results illustrate that introducing CuO, ZrO2, and RGO sheets enhanced the crystal structure properties of TiO2. It suggests that CuO/ZrO2/TiO2/RGO NCs are suitable for potential applications such as catalysis and medical and environmental remediation. The XRD and FTIR results (Fig. 1 and 7) were also in good agreement with the Raman results.
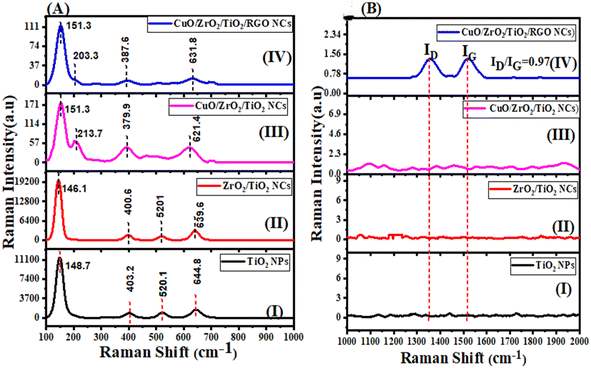 |
| Fig. 6 Raman spectra of pure TiO2 NPs, ZrO2/TiO2 NCs, CuO/ZrO2/TiO2 NCs, and CuO/ZrO2/TiO2/RGO NCs. | |
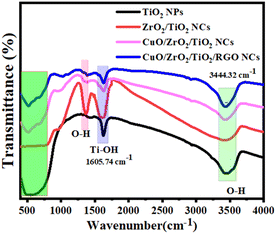 |
| Fig. 7 FT-IR spectra of pure TiO2 NPs, ZrO2/TiO2 NCs, CuO/ZrO2/TiO2 NCs, and CuO/ZrO2/TiO2/RGO NCs. | |
3.6 FTIR analysis
The composition and structure of NPs and NCs were further determined through Fourier transform infrared (FT-IR) spectroscopy. Fig. 7 illustrates the FTIR spectra of the synthesized TiO2 NPs, ZrO2/TiO2 NCs, CuO/ZrO2/TiO2 NCs, and CuO/ZrO2/TiO2/RGO NCs. The FTIR spectra demonstrate important absorption bands between 400 and 800 cm−1, which could be attributable to the stretching vibration of Ti–O, Zr–O, and Cu–O.73,74 Furthermore, the Ti–OH stretching vibrations in all the samples appeared at 1605.74 cm−1. This indicates hydrated molecules due to the hydroxyl group band, as shown in a previous study.75 We observed that the O–H stretching band of all the samples was assigned at 344.32 cm−1, signifying the successful reduction of graphene oxide and the existence of hydroxyl groups. Significantly, the shifts and intensity changes in the characteristic bands reflect strong interactions between the components, which can improve the physicochemical properties of CuO/ZrO2/TiO2/RGO NCs. This indicates the effect on the crystal structure of TiO2 NCs after the addition of CuO, ZrO2, and TiO2, as supported by the XRD and TEM results (Fig. 1 and 2). FTIR results showed that the presence of surface hydroxyls and metal–oxygen bonds highlight the potential of these materials for improved catalytic and anticancer performance.
3.7 Photoluminescence (PL) analysis
The charge recombination behavior of the prepared samples (Fig. 8) was investigated by PL analysis at an excitation wavelength of 325 nm. It can be observed that the PL spectra of pure TiO2 NPs displays a strong emission peak at about 360–480 nm, corresponding to the near-band-edge emission of the anatase phase of TiO2. The emission peaks of all the samples appeared at 372.3 nm, 416.5 nm, and 461.1 nm. Upon the incorporation of ZrO2, it can be observed that the PL intensity of pure TiO2 NPs was reduced. This reduction indicates improved charge separation due to the formation of heterojunctions between ZrO2 and TiO2, which suppresses recombination.76,77 With the addition of CuO, the PL intensity of pure TiO2 NPs is further reduced due to the effective charge transfer between CuO and the ZrO2/TiO2 NCs, as reported in a previous study.78 Furthermore, incorporating reduced graphene oxide (RGO) into CuO/ZrO2/TiO2 NCs improves the PL intensity by creating oxygen vacancies and defects, in agreement with some investigations.79,80 The PL results emphasized that the prepared CuO/ZrO2/TiO2/RGO NCs have potential applications, including environmental remediation and therapy.
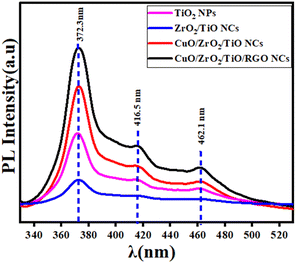 |
| Fig. 8 PL spectra of pure TiO2 NPs, ZrO2/TiO2 NCs, CuO/ZrO2/TiO2 NCs, and CuO/ZrO2/TiO2/RGO NCs. | |
3.8 Dynamic light scattering (DLS) analysis
The behaviour of the prepared samples and their stability in the culture media were assessed by measuring the particle size distribution and zeta potential, as illustrated in Fig. 9A–D. However, the distribution of particle size of pure TiO2 NPs, ZrO2/TiO2 NPs, CuO/ZrO2/TiO2 NCs, and CuO/ZrO2/TiO2/RGO NC was found to be 365.8 ± 122.6 nm, 320.8 ± 101.0 nm, 256.9 ± 4936 nm, and 250.8 ± 43.7 nm, respectively.81,82 These values indicate that the distribution of CuO–ZrO2–TiO2/RGO NCs was better than each of the prepared sample due to its excellent polydispersity index (PDI = 0.036), while the particles of pure TiO2 NPs showed high agglomeration, as supported by the TEM results (Fig. 3A, D, and G). The zeta potential values of the prepared samples to evaluate the behaviour of these samples and their stability in the culture media are shown in Table 1. Nevertheless, the zeta potential values of pure TiO2 NPs, ZrO2/TiO2 NPs, CuO/ZrO2/TiO2 NCs, and CuO/ZrO2/TiO2/RGO NC were −30.1 ± 4.56 mV, −33.9 ± 6.82 mV, −34.8 ± 6.77 mV, and −35.6 ± 6.92 mV, as matched with similar studies.83,84 These values demonstrate that the colloidal stability and distribution of CuO/ZrO2/TiO2/RGO NC were greater than those of individual samples in the suspension of the media culture. On the other hand, the negative charges on the surface of NPs and NCs could impact their interaction with cell membranes through electrostatic repulsion. DLS results suggest that CuO/ZrO2/TiO2/RGO NC could be applied in potential medical applications.
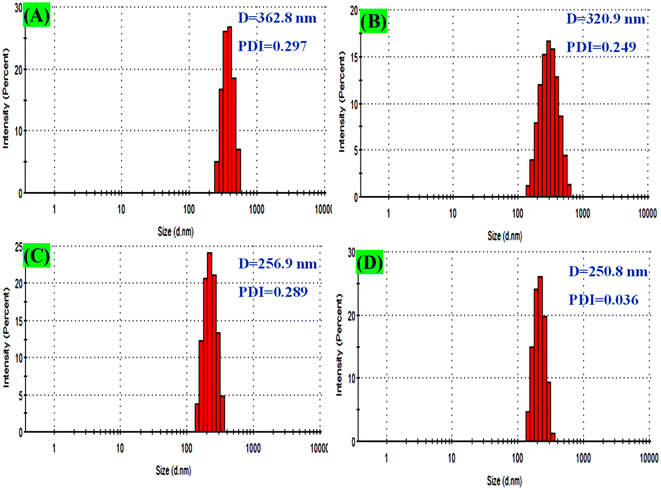 |
| Fig. 9 DLS analysis for particle size distribution: (A) pure TiO2 NPs, (B) ZrO2–TiO2 NCs, (C) CuO–ZrO2–TiO2 NCs, and (D) CuO–ZrO2–TiO2/RGO NCs. | |
Table 1 Zeta potential of the prepared samples in the culture media
Sample |
Zeta potential (mV ± SD) |
TiO2 NPs |
−30.1 ± 4.56 |
ZrO2/TiO2 NCs |
−33.9 ± 6.82 |
CuO/ZrO2/TiO2 NCs |
−34.8 ± 6.77 |
CuO/ZrO2/TiO2/RGO NCs |
−35.6 ± 6.92 |
3.9 Cytotoxicity and biocompatibility evaluations
Certain nanocomposites (NCs) have attractive potential application in medicine such as cancer therapy, biosensor, and drug delivery, as reported in previous studies.85,86 Fig. 10A–D shows the anticancer results of the prepared samples for an exposure time of 24 h and 48 h toward MCF-7 cancer cells. It can be observed in Fig. 10A that the TiO2 NPs exhibited moderate cytotoxicity against MCF-7 cells, with the cell viability decreasing as the concentration increased after 24 h. Under the same experimental conditions, after 48 hours, the cytotoxicity of these NPs was higher than that after 24 h through oxidative stress. These results were similar and supported with previous results.87,88 Fig. 10B demonstrated that the ZrO2/TiO2 NCs show improved cytotoxicity compared to pure TiO2 NPs, with a significant reduction in the cell viability after both 24 h and 48 h due to its stability. Similarly, CuO/ZrO2/TiO2 NCs (Fig. 10C) exhibited the highest cytotoxicity compared to TiO2 and ZrO2/TiO2 NCs at lower concentrations. At higher concentrations, CuO/ZrO2/TiO2/RGO NCs (Fig. 10C) induced higher cytotoxicity compared to CuO/ZrO2/TiO2 NCs owing to the ability of the RGO sheet to improve the distribution of NCs and reduce their aggregation. We observed that the CuO, ZrO2, and RGO sheets improved the cytotoxicity compared with each prepared sample. Table 2 shows the half maximal inhibitory concentration (IC50) values. These values exhibited that CuO/ZrO2/TiO2/RGO NCs have the highest cytotoxicity activity toward MCF-7 cells, with the lowest IC50 value (24.52 μg mL−1) at 48 h. These results suggest a synergistic effect from the structure of the prepared NPs and NCs and improved cell uptake. Additionally, this study highlighted that the combined CuO/ZrO2/TiO2 NC with RGO could hold potential as an anticancer agent due to the increased damage to cancer cells. Additionally, all samples show greater cytotoxicity with longer exposure, as seen from the lower IC50 values at 48 h compared to those at 24 h. In this study, we did not study the possible mechanisms of selective cytotoxicity of the present nanocomposites. However, earlier studies suggest that such types of nanocomposites exhibit anticancer activity through induction of oxidative stress.89,90 The outcome of this work paves the way for future research to delineate the plausible mechanisms of anticancer potential of CuO/ZrO2/TiO2/RGO NCs.
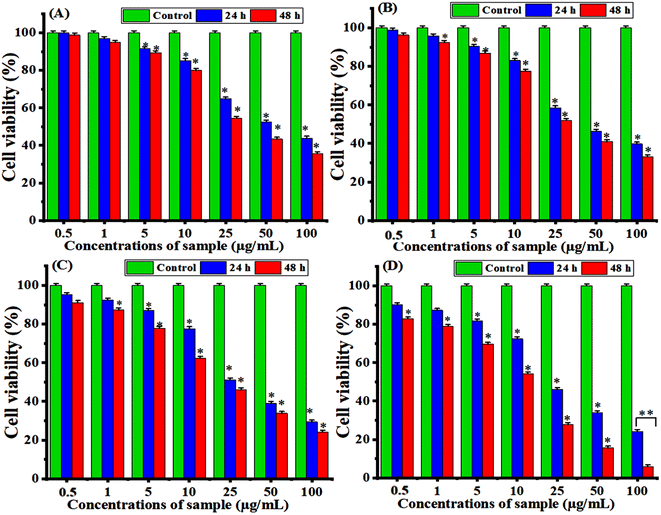 |
| Fig. 10 Cytotoxicity of the synthesized samples toward the breast (MCF-7) cancer cells for 24 h and 48 h using MTT assay: (A) pure TiO2 NPs, (B) ZrO2/TiO2 NCs, (C) CuO/ZrO2/TiO2 NCs, and (D) CuO/ZrO2/TiO2/RGO NCs. Stars (*) indicate significant differences (p < 0.05) between the treated cells and controls. | |
Table 2 IC50 values of the prepared samples for MCF-7 cells and HUVEC normal cells
Prepared samples |
MCF-7 cell lines |
HUVEC normal cells |
IC50 (μg mL−1 ± SD) |
IC50 (μg mL−1 ± SD) |
24 h |
48 h |
24 h |
48 h |
Pure TiO2 NPs |
73.98 ± 0.9 |
50.64 ± 1.1 |
1613.11 ± 10.1 |
1201.20 ± 9.6 |
ZrO2/TiO2 NCs |
53.17 ± 0.7 |
43.39 ± 1.3 |
1021 ± 5.4 |
1836.03 ± 7.8 |
CuO/ZrO2/TiO2 NC |
47.41 ± 1.0 |
34.03 ± 1.5 |
9251 ± 4.9 |
850.398 ± 6.2 |
CuO/ZrO2/TiO2/RGO NCs |
44.19 ± 1.2 |
24.52 ± 0.8 |
1923.21 ± 9.3 |
723.07 ± 5.1 |
The cell viability of HUVEC normal cells after two exposure times of 24 h and 48 h at concentrations (0.5, 1, 5, 10, 25, 50 and 100 μg mL−1) was measured, as presented in Fig. 11A and B. They observed that the cell viability remains nearby 100–85% for all the concentrations. This result indicates that the synthesized NPs and NCs are highly biocompatible with HUVEC cells. However, these samples are slightly cytotoxic at a high concentration of 100 μg mL−1 for 48 h (Fig. 11B) against HUVEC normal cells since the cell viability does not drop near or below 50%. In the present work, the IC50 of HUVEC normal cells is not feasible within the selected concentrations, as shown in Table 2. This result shows that these samples likely have an IC50 much higher than 100 μg mL−1 for HUVEC cells, which supports their biocompatibility.
 |
| Fig. 11 Biocompatibilities of the synthesized TiO2 NPs, ZrO2/TiO2 NCs, CuO/ZrO2/TiO2 NCs, and CuO/ZrO2/TiO2/RGO NCs toward the HUVEC normal cells using MTT assay: (A) 24 h and (B) 48 h. | |
4. Conclusion
In summary, the chemical co-precipitation process was successfully applied to fabricate CuO/ZrO2/TiO2/RGO NCs to improve their selective anticancer activity. XRD, HRTEM, SEM, XPS, Raman, FTIR, PL, and DLS were carefully used to examine the properties of the produced samples. XRD data showed that the supporting CuO, ZrO2, and RGO sheets for pure TiO2 NPs impact their phase and crystalline size. Surface spherical morphology and increased particle size of the prepared samples were confirmed through TEM and HRTM images. The percentage of elemental compositions (Cu, Zr, Ti, O, and C) and their distribution in the fabricated CuO/ZrO2/TiO2/RGO NCs were determined from EDX analysis. XPS results showed the chemical compositions and chemical state of the prepared NCs. Raman and FTIR spectra determined the functional groups and crystal structure. PL analysis revealed that the reduction of PL intensities of the obtained samples was due to reduced electron–hole recombination rates. DLS demonstrated that the colloidal stability and distribution of CuO/ZrO2/TiO2/RGO NC were greater than those of the individual samples in the suspension of the media culture. It can be exhibited that the negative charges on the surface of NPs and NCs could impact their interaction with cell membranes through electrostatic repulsion. In vitro study showed that the CuO/ZrO2/TiO2/RGO NCs have the highest anticancer activity (cytotoxicity) against breast cancer (MCF-7) cells compared to individual samples at high concentrations for 48 h. For the biocompatibility test, this result indicates that the synthesized NPs and NCs are highly biocompatible with HUVEC cells. Additionally, IC50 values of CuO/ZrO2/TiO2/RGO NCs (44.19 ± 1.2 μg mL−1 and 24.52 ± 0.8 μg mL−1 for 24 h and 48 h) were greater than those of each of the prepared samples. These results highlight that CuO/ZrO2/TiO2/RGO NCs have the ability to kill cancer cells for the enhanced anticancer performance of TiO2 NPs. This study should investigate their therapeutic performance on various cancer cell lines and in vivo model.
Data availability
The data supporting this work can be obtained from the corresponding author upon request.
Author contributions
Z. M. A. conceptualized the study, and the investigations and methods were carried out by Z. M. A., H. A. A., M. A. and S. A. The original draft was prepared by Z. M. A. Review and editing were conducted by Z. M. A., H. A. A., M. A. and S. A. All the authors reviewed and approved the final version of the text for publication.
Conflicts of interest
This work is original research and has not been submitted for publication elsewhere.
Acknowledgements
The authors extend their sincere appreciation to researchers supporting project number (RSPD2024R813), King Saud University, Riyadh, Saudi Arabia for funding this research.
References
- S. Quader and K. Kataoka, Mol. Ther., 2017, 25(7), 1501–1513 CrossRef CAS PubMed.
- Q. Zhou, L. Zhang and H. Wu, Nanotechnol. Rev., 2017, 6, 473–496 CAS.
- Z. Jing, Q. Du, X. Zhang and Y. Zhang, Chem. Eng. J., 2022, 446, 137–147 Search PubMed.
- J. Jeevanandam, J. K. U. Ling, A. Barhoum, Y. S. Chan and M. K. Danquah, Fundamentals of Bionanomaterials, 2022, pp. 1–29 Search PubMed.
- Z. Yin, L. Wu, H. G. Yang and Y. Su, Phys. Chem. Chem. Phys., 2013, 15(14), 4844–4858 RSC.
- A. Mansoor, Z. Khurshid, M. T. Khan, E. Mansoor, F. B. Butt, A. Jamal and P. J. Palma, Nanomaterials, 2022, 12, 3670 CrossRef CAS PubMed.
- S. Sargazi, S. Er, S. S. Gelen, A. Rahdar, M. Bilal, R. Arshad, N. Ajalli, M. F. A. Khan and S. Pandey, J. Drug Delivery Sci. Technol., 2022, 75, 103605 CrossRef CAS.
- Z. E. Allouni, M. R. Cimpan, P. J. Høl, T. Skodvin and N. R. Gjerdet, Colloids Surf., B, 2009, 68(1), 83–87 CrossRef CAS PubMed.
- A. Y. Elderdery, B. Alzahrani, A. Alabdulsalam, S. M. A. Hamza, A. M. E. Elkhalifa, A. H. Alhamidi, F. Alanazi, A. Mohamedain, S. K. Subbiah and P. L. Mok, Bioinorg. Chem. Appl., 2022, 1, 6835625 CrossRef PubMed.
- S. f. Alanazi, Z. M. Alaizeri, R. Lateef, N. Madkhali, A. Alharbi and M. Ahamed, Appl. Sci., 2023, 13, 12456 CrossRef CAS.
- P. Sivakumar, M. Lee, Y.-S. Kim and M. S. Shim, J. Mater. Chem. B, 2018, 6(30), 4852–4871 RSC.
- Y. T. Gebreslassie and F. G. Gebremeskel, Biotechnol. Rep., 2024, 44, e00828 CrossRef PubMed.
- Y. Shang, Q. Wang, J. Li, H. Liu, Q. Zhao, X. Huang, H. Dong, W. Chen, R. Gui and X. Nie, Front. Chem., 2021, 9, 522708 CrossRef CAS PubMed.
- A. J. Talaei, N. Zarei, A. Hasan, S. H. Bloukh, Z. Edis, N. A. Gamasaee, M. Heidarzadeh, M. M. N. Babadaei, K. Shahpas and, M. Sharifi, K. Akhatri, S. Khan, M. Xue and M. Falahati, Arabian J. Chem., 2021, 14, 102923 CrossRef.
- A. T. Mohamed, R. A. Hameed, S. H. EL-Moslamy, M. Fareid, M. Othman, S. A. Loutfy, E. A. Kamoun and M. S. Elnouby, Sci. Rep., 2024, 14, 6081 CrossRef CAS PubMed.
- Z. Lin, N. Goswami, T. Xue, O. J. H. Chai, H. Xu, Y. Liu, Y. Su and J. Xie, Adv. Funct. Mater., 2021, 31, 2105662 CrossRef CAS.
- X. Liu, J. Iocozzia, Y. Wang, X. Cui, Y. Chen, S. Zhao, Z. Li and Z. Lin, Energy Environ. Sci., 2017, 10, 402–434 RSC.
- D. Y. Nadargi, M. S. Tamboli, S. S. Patil, R.
B. Dateer, I. Mulla, H. Choi and S. S. Suryavanshi, ACS Omega, 2020, 5, 8587–8595 CrossRef CAS PubMed.
- P. Sable, N. Thabet, J. Yaseen and G. Dharne, Trends Sci., 2022, 19, 3092 CrossRef.
- S. C. Yadav, N. Rani and K. Saini, IOP Conf. Ser.:Mater. Sci. Eng., 2022, 012004 CrossRef CAS.
- A. Kubiak, K. Siwińska-Ciesielczyk and T. Jesionowski, Materials, 2018, 11, 2295 CrossRef PubMed.
- V. Isahi, C. Maghanga, M. Mwamburi, O. Munyati, S. Hatwaambo, E. Akoto, W. Isoe and M. W. Alam, Tanzan. J. Sci., 2023, 49, 590–602 Search PubMed.
- D. Paul, S. Mangla and S. Neogi, Mater. Lett., 2020, 271, 127740 CrossRef CAS.
- M. A. Marsooli, M. Fasihi-Ramandi, K. Adib, S. Pourmasoud, F. Ahmadi, M. R. Ganjali, A. S. Nasab, M. R. Nasrabadi and M. E. Plonska-Brzezinska, Materials, 2019, 12, 3274 CrossRef CAS PubMed.
- T. Indumathi, J. Suriyaprakash, A. A. Alarfaj, A. H. Hirad, R. Jaganathan and M. Mathanmohun, J. Basic Microbiol., 2024, 64, 2300505 CrossRef CAS PubMed.
- N. Thiyagarajulu, P. Deepak, C. Kamaraj, K. A. Al-Ghanim, A. Lakshminarayanan, M. Nicoletti, S. Arumugam and M. Govindarajan, Biomass Convers. Biorefin., 2023, 1–15 Search PubMed.
- N. K. Kadiyala, B. K. Mandal, L. V. K. Reddy, C. H. W. Barnes, L. D. L. S. Valladares and D. Sen, ACS Omega, 2023, 8, 2406–2420 CrossRef PubMed.
- M. Ahamed, M. J. Akhtar and M. Khan, Mater. Today Commun., 2023, 36, 106756 CrossRef CAS.
- L. Scotti, A. Aceto, B. B. Manshian, M. Ahamed, R. Lateef, M. A. M. Khan, P. Rajanahalli and M. J. Akhtar, J. Funct. Biomater., 2023, 14, 1–38 Search PubMed.
- Z. M. Alaizeri, H. A. Alhadlaq, S. Aldawood, M. J. Akhtar and M. Ahamed, ACS Omega, 2023, 8, 25020–25033 CrossRef CAS PubMed.
- Z. M. Alaizeri, H. A. Alhadlaq, S. Aldawood and N. A. Y. Abduh, RSC Adv., 2024, 14, 16685–16695 RSC.
- N. Askari, M. Askari and A. Di Bartolomeo, J. Electrochem. Soc., 2022, 169, 106511 CrossRef CAS.
- N. Askari, M. Askari and A. Di Bartolomeo, J. Electrochem. Soc., 2022, 169, 106511 CrossRef CAS.
- Z. A. M. Alaizeri, H. A. Alhadlaq, S. Aldawood and N. A. Y. Abduh, RSC Adv., 2024, 14, 16685–16695 RSC.
- Z. M. Alaizeri, H. A. Alhadlaq, S. Aldawood, M. J. Akhtar and M. Ahamed, ACS Omega, 2023, 8, 25020–25033 CrossRef CAS PubMed.
- Z. A. M. Alaizeri, H. A. Alhadlaq, S. Aldawood, M. J. Akhtar and M. Ahamed, Environ. Sci. Pollut. Res., 2023, 30, 6055–6067 CrossRef CAS PubMed.
- Z. M. Alaizeri, H. A. Alhadlaq, S. Aldawood, J. Akhtar and M. Ahamed, Polymers, 2022, 14, 2036 CrossRef CAS PubMed.
- E. Abdolmajid, H. Kharazi, M. M. Chalaki, M. Khojasteh, S. Haghighat, F. Attar, F. Nemati and M. Falahati, J. Biomol. Struct. Dyn., 2018, 37, 3007–3017 CrossRef PubMed.
- M. Baithy, D. Mukherjee, A. Rangaswamy and B. M. Reddy, Catal. Lett., 2022, 152, 1428–1440 CrossRef CAS.
- M. Kikugawa, K. Yamazaki and H. Shinjoh, Appl. Catal., A, 2017, 547, 199–204 CrossRef CAS.
- M. T. Ulhakim, S. Sukarman, K. Khoirudin, N. Fazrin, T. Irfani and A. Hakim, Indones. J. Appl. Phys., 2024, 14, 141–150 CrossRef.
- H. Koohestani and S. K. Sadrnezhaad, Desalin. Water Treat., 2016, 57, 28450 CrossRef CAS.
- T. H. Nguyen, T. L. Nguyen, T. D. T. Ung and Q. L. Nguyen, Adv. Nat. Sci.:Nanosci. Nanotechnol., 2013, 4, 025002 CAS.
- R. R. Muthuchudarkodi and C. Vedhi, Adv. Mater. Res., 2013, 678, 50–55 CAS.
- N. Yaacob, A. F. Ismail, G. P. Sean and N. A. M. Nazri, SN Appl. Sci., 2019, 1, 1–14 CrossRef.
- S. Bhattacharjya, T. Adhikari, A. Sahu and A. K. Patra, Ecotoxicology, 2021, 30, 719–732 CrossRef CAS PubMed.
- A. Fall, I. Ngom, M. Bakayoko, N. F. Sylla, H. E. A. Mohamed, K. Jadvi, K. Kaviyarasu and B. D. Ngom, Mater. Today: Proc., 2021, 36, 349–356 CAS.
- D. Guerrero-Areque, R. Gómez and H. A. Calderon, Microsc. Microanal., 2017, 23, 2036–2037 CrossRef.
- D. Guerrero-Areque, R. Gómez and H. A. Calderon, Microsc. Microanal., 2017, 23, 2036–2037 CrossRef.
- Y. N. Sudhakar, H. Hemant, S. Nitinkumar, P. Poornesh and M. Selvakumar, Ionics, 2017, 23, 1267–1276 CrossRef CAS.
- M. R. Shaik, M. Alam, S. F. Adil, M. Kuniyil, A. A. Al-Warthan, M. R. H. Siddiqui, M. N. Tahir, J. P. Labis and M. Khan, Materials, 2019, 12, 711 CrossRef CAS PubMed.
- K. V. Bineesh, D.-K. Kim and D.-W. Park, Nanoscale, 2010, 2(7), 1222–1228 RSC.
- J. Tasiu, M. Y. Onimisi, A. S. Yusuf, E. Danladi and N. N. Tasie, East Eur. J. Phys., 2024, 1, 315–321 CrossRef.
- L. J. Tomar and B. S. Chakrabarty, Adv. Mater. Lett., 2013, 4, 64–67 CrossRef.
- R. R. Muthuchudarkodi and C. Vedhi, Adv. Mater. Res., 2013, 678, 50–55 CAS.
- L. Jayasinghe, V. Jayaweera, N. de Silva and A. M. Mubarak, Mater. Adv., 2022, 3, 7904–7917 RSC.
- A. G. Bekru, L. T. Tufa, O. A. Zelekew, J. Gwak, J. Lee and F. K. Sabir, Crystals, 2023, 13, 133 CrossRef CAS.
- I. M. Sharaf, J. Laifi, S. W. Alraddadi, M. Saad, M. S. I. Koubesy, N. N. Elewa, H. Almohiy, Y. A. M. Ismail, A. Soldatov and A. M. Aboraia, Heliyon, 2024, 10, 1 Search PubMed.
- A. Thakur, S. Kumar, G. Singh and V. S. Rangra, Mater. Today: Proc., 2022, 9, 129 Search PubMed.
- S. G. Babu, R. Vinoth, D. Praveen Kumar, M. V. Shankar, H. L. Chou, K. Vinodgopal and B. Neppolian, Nanoscale, 2015, 7, 7849–7857 RSC.
- D. Cosma, A. Urda, T. Radu, M.-C. Roşu, M. Miheţ and C. Socaci, Molecules, 2022, 27, 5803 CrossRef CAS PubMed.
- L. Martin, H. Martínez, D. Poinot, B. Pecquenard and F. Le Cras, J. Phys. Chem. C, 2013, 117, 4421–4430 CrossRef CAS.
- M. C. Biesinger, Surf. Interface Anal., 2017, 49, 1325–1334 CrossRef CAS.
- J. Qian, Q. Hu, X. Hou, F. Qian, L. Dong and B. Li, Ind. Eng. Chem. Res., 2018, 57(38), 12792–12800 CrossRef CAS ..
- K. V. R. Chary, G. V. Sagar, D. Naresh, K. K. Seela and B. Sridhar, J. Phys. Chem. B, 2005, 109(19), 9437–9444 CrossRef CAS PubMed.
- Q. T. Trinh, K. Bhola, P. N. Amaniampong, F. Jérôme and S. H. Mushrif, J. Phys. Chem. C, 2018, 122(39), 22397–22406 CrossRef CAS.
- K. Dong, J. He, J. Liu, F. Li, L. Yu, Y. Zhang, X. Y. Zhou and H. Ma, J. Mater. Sci., 2017, 52, 6754–6766 CrossRef CAS PubMed.
- N. J. Ananth, R. G. S. Rao, V. S. Vinita, J. Samuel, S. Shabna, P. M. S. Shinu, S. Suresh, Y. Samson and C. S. Biju, Phosphorus, Sulfur Silicon Relat. Elem., 2021, 197, 164–168 CrossRef.
- M. Baithy, D. Mukherjee, A. Rangaswamy and B. M. Reddy, Catal. Lett., 2022, 152, 1428–1440 CrossRef CAS.
- Ma. Manríquez, M. Picquart, X. Bokhimi, T. López, P. Quintana and J. M. Coronado, J. Nanosci. Nanotechnol., 2008, 8(12), 6623–6629 CrossRef.
- M. Z. U. Shah, M. Sajjad, H. Hou, S. ur Rahman, A. Mahmood, U. Aziz and A. Shah, J. Energy Storage, 2022, 55, 105492 CrossRef.
- E. Hajialilou, H. Asgharzadeh and S. Khameneh Asl, Appl. Surf. Sci., 2021, 544, 148832 CrossRef CAS.
- S. Sagadevan, J. Podder and I. Das, J. Mater. Sci.: Mater. Electron., 2016, 27, 5622–5627 CrossRef CAS.
- M. Ikram, J. Hassan, A. Raza, A. Haider, S. Naz, A. Ul-Hamid, J. Haider, I. Shahzadi, U. Qamar and S. Ali, RSC Adv., 2020, 10, 30007–30024 RSC.
- M. Chen, T. P. Straatsma and D. A. Dixon, J. Phys. Chem. A, 2015, 119(46), 11406–11421 CrossRef CAS PubMed.
- A. K. Singh and U. T. Nakate, Sci. World J., 2014, 1, 349457 Search PubMed.
- L. Li, X. Qu, G. Pan and J. H. Jeong, Chemosensors, 2024, 12, 62 CrossRef CAS.
- D. Bala, I. Matei, G. Ioniţă, D. Cosma, M.-C. Roşu, M. Stanca, C. Gaidău, M. Baleanu, M. Virgolici and I. R. Stanculescu, Int. J. Mol. Sci., 2022, 23, 14703 CrossRef CAS PubMed.
- E. Hajialilou, H. Asgharzadeh and S. Khameneh Asl, Appl. Surf. Sci., 2021, 544, 148832 CrossRef CAS.
- N. Subha, M. Mahalakshmi, M. Myilsamy, N. Lakshmana Reddy, M. V Shankar, B. Neppolian and V. Murugesan, Int. J. Hydrogen Energy, 2018, 43, 3905–3919 CrossRef CAS.
- S. Saukani, UIN Sunan Gunung Djati Bandung, 2018 Search PubMed.
- Y. V Larichev, Inorganics, 2022, 10, 248 CrossRef.
- J. M. Berg, A. A. Romoser, N. Banerjee, R. Zebda and C. M. Sayes, Nanotoxicology, 2009, 3, 276–283 CrossRef CAS.
- A. H. Shah and M. A. Rather, Mater. Today: Proc., 2021, 44, 482–488 CAS.
- A. K. Nayak, S. Alkahtani and M. Saquib Hasnain, Biomed. Compos., 2021, 35–69 Search PubMed.
- S. U. R. Qamar, Colloid Interface Sci. Commun., 2021, 43, 100463 CrossRef CAS.
- M. J. Basante-Romo, Ó. Gutiérrez and R. J. Camargo-Amado, Inf. Tecnol., 2016, 27, 63–68 CrossRef CAS ..
- R. Vigneshwaran, D. Ezhilarasan and S. Rajeshkumar, Inorg. Chem. Commun., 2021, 133, 108920 CrossRef CAS.
- M. Ahamed, M. J. Akhtar, M. A. M. Khan and H. A. Alhadlaq, Int. J. Nanomed., 2021, 16, 89–104 CrossRef PubMed.
- C. S. Chakra, V. Rajendar, K. V. Rao and M. Kumar, 3 Biotech, 2017, 7, 1–8 CrossRef PubMed.
|
This journal is © The Royal Society of Chemistry 2024 |
Click here to see how this site uses Cookies. View our privacy policy here.