DOI:
10.1039/D4RA04916K
(Paper)
RSC Adv., 2024,
14, 26913-26919
Stereocontrolled and time-honored access to piperidine- and pyrrolidine-fused 3-methylenetetrahydropyrans using lactam-tethered alkenols†
Received
8th July 2024
, Accepted 19th August 2024
First published on 27th August 2024
Abstract
Polycyclic oxygen-heterocycles bearing the 3-methylenetetrahydropyran (i.e., 3-MeTHP) motif are resident in bioactive molecules such as hodgsonox and iridoid. Meanwhile, the δ- and γ-lactam topologies as well as their reduced variants (i.e., piperidines and pyrrolidines) are at the core of several pharmaceuticals and fragrances. A stereocontrolled, time-honored, and cost-effective strategy that merges a 3-MeTHP motif with the aforementioned azaheterocyclic scaffolds could exponentially expand the 3D-structural space for the discovery of new small molecules with medicinal value. In these studies, readily affordable lactam-tethered alkenols have been interrogated in two complementary cascade approaches, leading to the regioselective and stereocontrolled synthesis of lactam-fused 3-MeTHPs. The first approach hinges on regioselective 6-endo-trig bromoetherification of the alkenols and concomitant elimination to arrive at the desired 3-MeTHPs. The methylene portion of the 3-MeTHP is unveiled at a late stage, which is noteworthy since all existing approaches to 3-MeTHPs rely on early-stage introduction of the methylene group. The second strategy involves transition metal-catalyzed alkoxylation of the tethered alkenol followed by base-induced double bond isomerization. The lactam-fused 3-MeTHPs are obtained in high site- and diastereo-selectivities. Post-modification of the bicycles has led to the construction of 3-MeTHP-fused saturated piperidines and pyrrolidines as well as 3-MeTHPs bearing four contiguous stereocenters.
Introduction
The ever-increasing need for the development of expedient and efficient strategies for the construction and functionalization of privileged motifs (from the standpoint of drug discovery) such as the 3-methylenetetrahydropyran (3-MeTHP) motif is supported by their prevalence in several natural products and pharmaceuticals (see, antitumor agent A, hodgsonox, and iridoid; Fig. 1).1 Hippospongic acid A, which is a known inhibitor of gastrulation in starfish embryos, also harbors the 3-alkylidenetetrahydropyran topology.1e,f Accordingly, several methodologies have emerged for the construction of diversely substituted 3-MeTHPs,2 including Claisen rearrangement,2e Ni-catalyzed reductive coupling,2f palladium-catalyzed Umpolung cycloaddition,2g and intramolecular radical cycloaddition.2h Efforts to increase step/atom economy,3 reduce harmful environmental effects, and increase the overall efficiency of experimental sequences have led to the popularity of cascade4 or domino reactions5 in the organic synthesis community.6,7 We therefore surmised that a modular, stereocontrolled, and cascade strategy that merges a 3-MeTHP motif with the ever so important lactam topology would likely expand the 3D-structural space for the discovery of new small molecules with medicinal value.
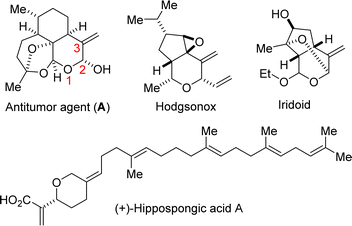 |
| Fig. 1 Examples of bioactive 3-methylenetetrahydropyrans. | |
Previously, we disclosed that the direct oxidative alkoxylation of lactam-tethered alkenols of type 1 proceeds with 6-endo selectivity under Pd- or Cu-catalysis (Fig. 2A, see 2).8 Additionally, we showed that Fe- or Ru-catalyzed dehydrative coupling of 1 furnishes the C–C cross-coupling products (see 3).9 Desiring an efficient synthesis of lactam-fused 3-MeTHPs of type 4, we surmised that alkenol 1 offered a resplendent starting point. Two convergent and potentially complementary approaches to 4 were envisioned. The first would entail regioselective 6-endo halocycloetherification of 1 to afford intermediates such as 5 (Fig. 2B1), which could then undergo concomitant anti-Zaitsev dehydrohalogenation to furnish 4. The second envisioned approach to lactam-fused 3-MeTHPs involves dehydrogenative alkoxylation of 1 to arrive at fused dihydropyrans such as 2, which could then be isomerized to 4 under suitable contra-thermodynamic conditions (Fig. 2B2). The first approach to lactam-fused 3-MeTHPs would proceed under transition metal-free conditions and would complement existing approaches to 3-MeTHPs, which are heavily reliant on transition metal catalysis (Fig. 2C).2f–j
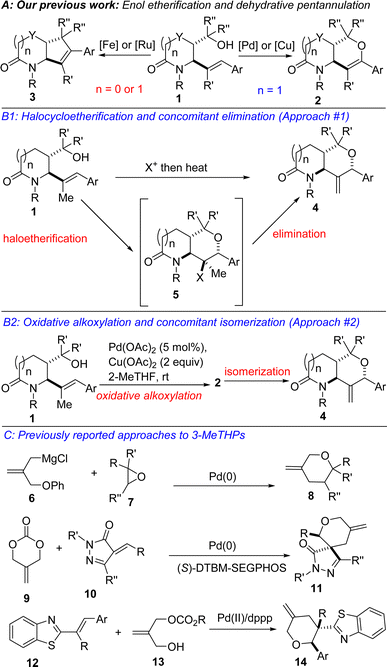 |
| Fig. 2 (A) Our prior cyclization efforts using lactam-tethered alkenol 1, (B1 and B2) our proposed plans for accessing lactam-fused 3-methylenetetrahydropyrans from 1, (C) previously reported approaches to functionalized 3-MeTHPs. | |
Another unique aspect of our design is the late-stage installation of the methylene portion of the 3-MeTHP given that all reported efforts depicted in Fig. 2C are predicated on early-stage introduction of the methylene group through one of the precursors.2f–k Central to achieving the desired outcomes in this approach to 3-MeTHPs would be to properly control the site-selectivity of the haloetherification in favor of 6-endo-trig cyclization products anti-5 or syn-5 (Fig. 3) over the undesirable 5-exo bicycles (i.e., anti-15 or syn-16). Based on our experience with halolactonization10 of such substrates, we anticipate that reaction conditions would play a crucial role. We also recognized that it would be paramount to establish epimerization-free elimination conditions that lead to the desired anti-Zaitsev product (4). This is all the more necessary since two of the three β-hydrogens resident in 5 are in innately more acidic and labile positions. One resides in the α-alkoxy and benzylic position, which could lead to the formation of enol ether 2. The other β-hydrogen is located in the α-amino position and could generate bicyclic enamide 17. Another obstacle that we would have to overcome is that of double-bond isomerization, which could instead furnish the aforementioned thermodynamic products (i.e., 2 and 17). Detailed efforts toward the manifestation of our ideals are described herein.
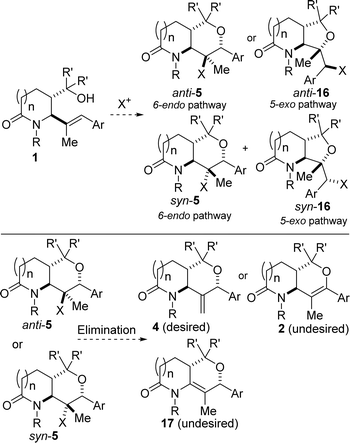 |
| Fig. 3 Potential challenges associated with the transformation of lactam-tethered alkenol 1 to lactam-fused 3-MeTHP 4. | |
Results and discussion
Studies on the regioselective and stereocontrolled synthesis of lactam-fused 3-MeTHPs of type 4 commenced with the search for appropriate conditions for efficient halocyclization of 1 and concomitant elimination (approach #1). It has previously been reported that the combination of phthaloyl peroxide (PPO) and X− can generate Brønsted base covalently tethered carbonyl hypohalites (BCTC).11 Envisioning that BCTC could act as an in situ-generated electrophilic reagent for halocyclization reactions, the authors developed an Intramolecular Chaperone-assisted Dual-anchoring Activation (ICDA) model.12,13 Four alkenols were engaged in a bromoetherification protocol and they all furnished bromotetrahydrofurans.12 Having recently found efficient but slow conditions for the conversion of 1 to 5 (X = Br),14 and being cognizant that the ICDA model is capable of delivering haloetherification products in less than an hour, we sought to interrogate 1 in this potentially sagacious mode of reactivity.
Optimization studies were conducted using alkenol 1a and phthaloyl peroxide (PPO) out-competed other oxidants such as malonoyl peroxide (MPO), benzoyl peroxide (BPO), and di-tert-butyl peroxide (DTBP) (Table 1, entries 1–3). Other bromine sources did not perform as well as tetrabutylammonium bromide TBAB (entries 4–11). After screening several reaction media, DCE emerged as the optimum reaction medium with respect to the yield, regioselectivity and stereoselectivity (entries 12–17). Thus, after stirring a mixture of 1a (1 mmol) and TBAB (1.1 equiv.) in dichloroethane (10 mL, 0.1 M) for 30 minutes at room temperature, 1H and 13C NMR analyses of the crude mixture prior to extractive workup revealed the presence of 5a. The intramolecular bromoetherification performed well in 1,4-dioxane. Desiring to develop a one-pot approach for the conversion of 1 to 4, we elected to go with 1,4-dioxane (entry 15) as the optimum reaction medium. Thus, a mixture of 1a (1 mmol) and TBAB (1.1 equiv.) in 1,4-dioxane (10 mL, 0.1 M) was stirred for 30 minutes at room temperature prior to heating to 100 °C for the desired length of time (Scheme 1). In the event, the structurally diverse lactam-fused 3-MeTHPs depicted in Scheme 1 were obtained. Differentially N-substituted lactams tend to display diverse reactivity and biological activity profiles.15 We were pleased to see that γ-lactam-tethered alkenols harboring electronically diverse N-benzyl substituents underwent cyclization and concomitant site-selective dehydrobromination (see 4a–d). N-Alkyl lactam-alkenols are also competent substrates for the domino process (see 4e–i). These studies have revealed that γ-lactams bearing halogenated arenes are well tolerated (see 4b–d, 4h, 4i, 4k–n, and 4p), which bodes well for late-stage diversification since the halogen group may be utilized as a requisite group for cross-coupling purposes. The results indicate that dehalogenation of the aryl halides does not occur during the second step of the one-pot process that is tailored toward dehydrobromination of the tertiary alkyl bromides. Innately more reactive γ-lactam-tethered primary alkenols afford the lactam-fused 3-MeTHPs in synthetically attractive yields and selectivities when subjected to this bromoetherification-elimination protocol (see 4o/p).
Table 1 Optimization of the bromoetherification of lactam-tethered alkenol 1a
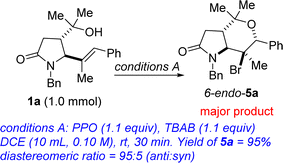
|
Entry |
Deviation from conditions A |
% Yield of 5a |
1 |
MPO in place of PPO |
88 |
2 |
BPO in place of PPO |
12 |
3 |
DTBP in place of PPO |
7 |
4 |
NBP in place of TBAB |
11 |
5 |
NBS in place of TBAB |
16 |
6 |
DBDMH in place of TBAB |
14 |
7 |
NBA in place of TBAB |
36 |
8 |
LiBr in place of TBAB |
46 |
9 |
NaBr in place of TBAB |
39 |
10 |
KBr in place of TBAB |
18 |
11 |
CsBr in place of TBAB |
11 |
12 |
DCM in place of DCE |
90 |
13 |
THF in place of DCE |
83 |
14 |
2-MeTHF in place of DCE |
86 |
15 |
1,4-Dioxane in place of DCE |
92 |
16 |
DMF in place of DCE |
9 |
17 |
Diethylether in place of DCE |
26 |
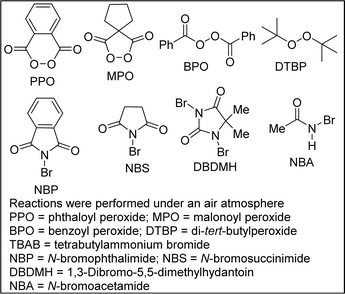 |
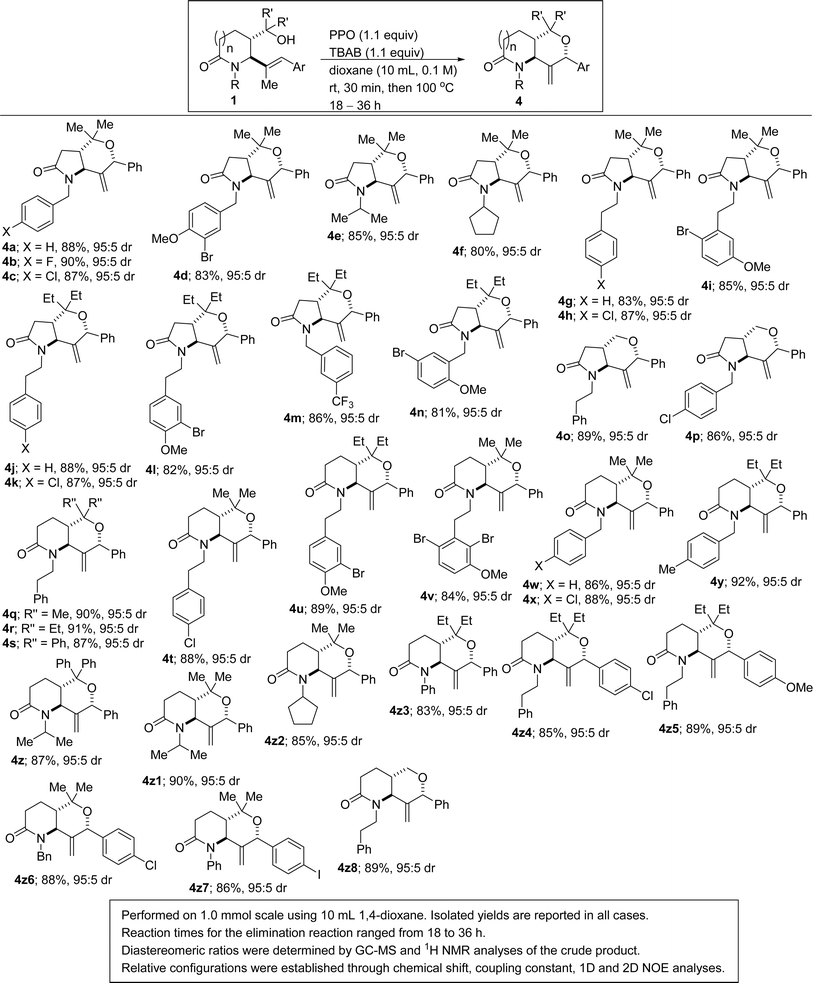 |
| Scheme 1 Scope of bromocycloetherification and concomitant dehydrobromination of lactam-tethered tertiary trisubstituted alkenols. | |
Polysubstituted and polycyclic 2-oxopiperidines (i.e., δ-valerolactams) are increasingly attractive fragments for potential drug discovery since the strategic placement of substituents about this three-dimensional scaffold is ideally suited for structure–activity relationship studies.16 It was therefore gratifying to find that δ-valerolactam-tethered alkenols also react regioselectively and diastereoselectively under the developed cascade protocol to afford the 2-piperidinone-fused 3-MeTHPs depicted in Scheme 1 (see 4q–4z8). A significant reactivity difference was observed with this one-carbon larger lactams. For example, whereas the second step of the one-pot, two-step sequence took about 36 h in the case of the γ-lactam-tethered alkenols, the homologous δ-lactam-tethered alkenols showed complete conversion in about 18 h. While these differences might not be surprising to practitioners of N-heterocyclic chemistry, they further highlight why extending reactivity trends from one class of a nitrogen heterocycle to another requires special consideration. Presumably, this pronounced disparity in reactivity is due to conformational differences. As a testament to the generality of the transformation, when the phenyl group on the styrenyl unit resident in alkenol 1 is replaced by an electron-deficient aryl group (see 4z4) or an electron-rich aryl substituent (see 4z5), the efficacy of the transformation is not compromised. Since the methyl-bearing styrenyl motif resident in alkenol 1 is derived from alpha-methyl cinnamaldehydes,17 a current limitation of these studies is our inability to install π-excessive or π-deficient heteroaromatic groups (e.g., thiophenes and pyridines, respectively) at site of cyclization.
These studies have revealed that lactam-fused 3-MeTHPs can also be assembled using the second postulated approach, which features catalytic intramolecular dehydrogenative enol etherification of 1 and concomitant base-assisted contra-thermodynamic isomerization (Scheme 2).
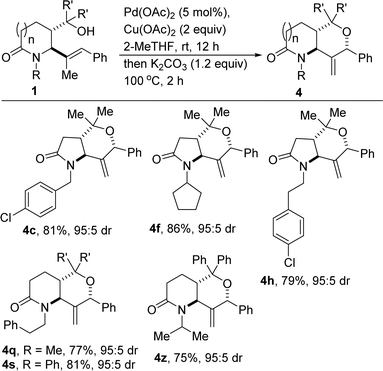 |
| Scheme 2 Construction of lactam-fused 3-MeTHPs by transition metal-catalyzed dehydrogenative coupling and base-assisted isomerization. | |
A potentially beneficial aspect of these studies is the scalable nature of the reactions given that several of the products (e.g., 4a/h/l) have been prepared in over 5 mmol scale, with little to no compromise in efficiency. This has set the stage for post-diversification studies.
There are high incentives for the construction of saturated azaheterocyclic scaffolds, especially the piperidine and pyrrolidine motifs, which are common structural motifs in agrochemicals, fragrances, and pharmaceuticals.18,19 Recognizing that some of the most potent 2-oxopyrrolidines and 2-oxopiperidines have the lactam portion reduced to the corresponding saturated cyclic amine, and seeking to demonstrate the versatility of our synthetic methodology, we decided to subject some of the lactam-fused 3-MeTHPs to complete reduction. In the event, we found that the lithium aluminium hydride-assisted reduction of selected examples of 4 affords the bicyclic amines depicted in Scheme 3 (see 18a–d). No erosion in the diastereoselectivity was observed during the reduction process. The bicyclic tertiary amines are unsurprisingly less stable than their lactam counterparts and require special handling.
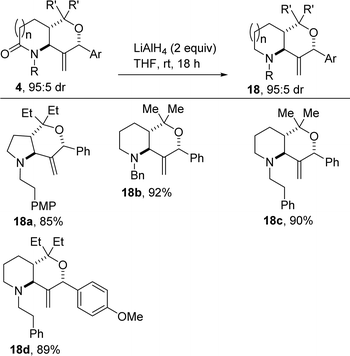 |
| Scheme 3 Synthesis of piperidine- and pyrrolidine-fused 3-MeTHPs. | |
We have found that catalytic hydrogenation of the exocyclic alkene present in 4 proceeds diastereoselectively and furnishes lactam-fused tetrahydropyrans of type 19, which bear four contiguous stereocenters (Scheme 4). The diastereomeric ratios were determined using GC-MS analysis of the crude products. In the case of 19c, 19F NMR analysis was also employed. The ascribed relative configuration was established based on 1D and 2D NOE analyses.
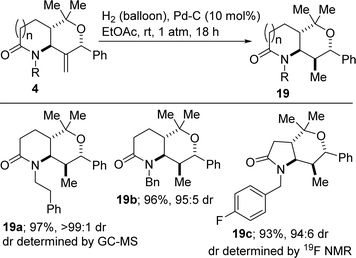 |
| Scheme 4 Catalytic hydrogenation of lactam-fused 3-MeTHPs. | |
Conclusions
In summary, we have leveraged the synthetic versatility of lactam-tethered alkenols, which are readily obtainable through the 1,3-azadiene-anhydride annulation reaction, to develop two step-economical approaches to lactam-fused 3-MeTHPs. The first strategy takes advantage of the spatially confined non-covalent orientation and proximity effect for the direct generation of heteroatom-centered radicals from O–H bonds, leading to rapid regioselective bromocycloetherification and concomitant elimination. This approach to bicyclic 3-MeTHPs proceeds under transition metal-free conditions and nicely complements existing approaches to 3-MeTHPs, which utilize transition metal catalysis. The late-stage introduction of the methylene portion of the 3-MeTHP through a thermally induced elimination or base-induced isomerization event is a unique feature of these studies. These novel cascade approaches to highly customized bicyclic 3-MeTHPs have set the stage for future post-diversification and structure–activity relationship studies. We anticipate that the scalable as well as operationally simple nature of these protocols would endear them to the organic and medicinal chemistry communities.
Data availability
The data supporting this article have been included as part of the ESI.†
Author contributions
T. K. B. – conceptualization, project administration, supervision, investigation, data curation, methodology, writing – original draft, internal funding acquisition; I. S. A. – investigation, data curation, methodology; J. K. – investigation, data curation, methodology.
Conflicts of interest
There are no conflicts of interest to declare.
Acknowledgements
We are grateful to Central Washington University for financial support through startup funds to T. K. B. and for the continuous maintenance of major research instrumentation. The School of Graduate Studies and Research at CWU is thanked for partial support of this work through a Faculty Research Award to T. K. B. and for a graduate summer fellowship to I. S. A. The Office of the Provost is acknowledged for research fellowships to J. K. and I. S. A. We thank the Office of University Research (OUR) for supporting this project through funding awarded to J. K.
Notes and references
-
(a) G. D. Ainge, P. J. Gerard, S. F. R. Hinkley, S. D. Lorimer and R. T. Weavers, J. Org. Chem., 2001, 66, 2818–2821 CrossRef PubMed;
(b) C. Chollet, B. Crousse, M. Ourévitch and D. Bonnet Delpon, J. Org. Chem., 2006, 71, 3082–3085 CrossRef PubMed;
(c) A. M. Galal, S. A. Ross, M. A. ElSohly, H. N. ElSohly, F. S. El Feraly, M. S. Ahmed and A. T. McPhail, J. Nat. Prod., 2002, 65, 184–188 CrossRef PubMed;
(d) F.-W. Dong, Z.-K. Wu, L. Yang, C.-T. Zi, D. Yang, R.-J. Ma, Z. H. Liu, H.-R. Luo, J. Zhou and J.-M. Hu, Phytochemistry, 2015, 118, 51–60 CrossRef PubMed;
(e) S. Ohta, M. Uno, M. Yoshimura, Y. Hiraga and S. Ikegami, Tetrahedron Lett., 1996, 37, 2265 CrossRef CAS;
(f) S. Ohta, M. Uno, M. Yoshimura, Y. Hiraga and S. Ikegami, Tetrahedron Lett., 1996, 37, 7765 CrossRef CAS.
-
(a) A. J. Barlow, B. J. Compton and R. T. Weavers, J. Org. Chem., 2005, 70, 2470–2475 CrossRef CAS PubMed;
(b) M. Kim and D. Lee, Org. Lett., 2005, 7, 1865–1868 CrossRef CAS PubMed;
(c) J. C. R. Brioche, K. M. Goodenough, D. J. Whatrup and J. P. A. Harrity, Org. Lett., 2007, 9, 3941–3943 CrossRef CAS PubMed;
(d) M. G. Beaver, S. B. Billings and K. A. Woerpel, J. Am. Chem. Soc., 2008, 130, 2082–2086 CrossRef CAS PubMed;
(e) C. Uyeda and E. N. Jacobsen, J. Am. Chem. Soc., 2008, 130, 9228–9229 CrossRef;
(f) M. G. Beaver and T. F. Jamison, Org. Lett., 2011, 13, 4140–4143 CrossRef PubMed;
(g) H. Tsukamoto, A. Kawase and T. Doi, Adv. Synth. Catal., 2019, 361, 3733–3738 CrossRef;
(h) S. Gowrisankar, K. Y. Lee, T. H. Kim and J. N. Kim, Tetrahedron Lett., 2006, 47, 5785–5788 CrossRef;
(i) X. Song, L. Xu and Q. Ni, Org. Biomol. Chem., 2020, 18, 6617–6621 RSC;
(j) J. Xu, W. Shi, M. Liu, J. Liao, W. Wang, Y. Wu and H. Guo, Adv. Synth. Catal., 2022, 364, 2060 CrossRef CAS.
-
(a) R. A. Sheldon, Chem. Soc. Rev., 2012, 41, 1437–1451 RSC;
(b) B. M. Trost, Handbook of Green Chemistry, 2012, vol. 7, pp. 1–33 Search PubMed;
(c) B. M. Trost, Angew. Chem., Int. Ed. Engl., 1995, 34, 259–281 CrossRef CAS.
-
(a) A. S. Kleinke, D. Webb and T. F. Jamison, Tetrahedron, 2012, 68, 6999–7018 CrossRef CAS;
(b) K. C. Nicolaou and J. S. Chen, Chem. Soc. Rev., 2009, 38, 2993–3009 RSC.
- L.-F. Tietze, Domino Reactions in Organic Synthesis, Wiley-VCH, Weinheim, 2006 Search PubMed.
- For reactions described in the literature as cascade reactions, see:
(a) B. D. Horning and D. W. C. MacMillan, J. Am. Chem. Soc., 2013, 135, 6442–6445 CrossRef;
(b) H. Ohno, Asian J. Org. Chem., 2013, 2, 18–28 CrossRef;
(c) M. Pintado-Sierra, A. M. Rasero-Almansa, A. Corma, M. Iglesias and F. Sanchez, J. Catal., 2013, 299, 137–145 CrossRef;
(d) K. Surendra, W. Qiu and E. J. Corey, J. Am. Chem. Soc., 2011, 133, 9724–9726 CrossRef;
(e) H. Li and T.-P. Loh, Org. Lett., 2010, 12, 2679–2681 CrossRef CAS PubMed;
(f) J. E. Dalgard and S. D. Rychnovsky, Org. Lett., 2005, 7, 1589–1591 CrossRef CAS PubMed.
- For reactions described as domino sequences, see:
(a) X.-L. Liu, W.-Y. Han, X.-M. Zhang and W.-C. Yuan, Org. Lett., 2013, 15, 1246–1249 CrossRef CAS;
(b) Y. Long, Z. She, X. Liu and Y. Chen, J. Org. Chem., 2013, 78, 2579–2588 CrossRef CAS;
(c) B. Z. Lu, H.-X. Wei, Y. Zhang, W. Zhao, M. Dufour, G. Li, V. Farina and C. H. Senanayake, J. Org. Chem., 2013, 78, 4558–4562 CrossRef CAS.
- K. Hovenkotter, H. Braunstein, S. Langevin and T. K. Beng, Org. Biomol. Chem., 2017, 15, 1217–1221 RSC.
- T. K. Beng, M. Bauder, M. J. Rodriguez and A. Moreno, New J. Chem., 2018, 42, 16451–16455 RSC.
- T. K. Beng, M. Rodriguez and C. Borg, RSC Adv., 2022, 12, 17617–17620 RSC.
-
(a) R. Zhao, K. Fu, Y. Fang, J. Zhou and L. Shi, Angew. Chem., Int. Ed., 2020, 59, 20682–20690 CrossRef PubMed;
(b) R. Zhao, Y. Yao, D. Zhu, D. Chang, Y. Liu and L. Shi, Org. Lett., 2018, 20, 1228–1231 CrossRef PubMed.
- X. Yang, H. Gao, J. Yan, J. Zhou and L. Shi, Chem. Sci., 2024, 15, 6130–6140 RSC.
- N. London, D. Movshovitz-Attias and O. Schueler-Furman, Structure, 2010, 18, 188–199 CrossRef PubMed.
- I. S. Anosike and T. K. Beng, RSC Adv., 2024, 14, 18501–18507 RSC.
- T. K. Beng and R. E. Gawley, J. Am. Chem. Soc., 2010, 132, 12216 CrossRef PubMed.
-
(a) For examples of piperidines in fragment libraries, see: G. Wang, L. Chen, T. Xian, Y. Liang, X. Zhang, Z. Yang and M. Luo, Org. Biomol. Chem., 2014, 12, 8048–8060 RSC;
(b) J. A. Johnson, C. A. Nicolaou, S. E. Kirberger, A. K. Pandey, H. Hu and W. C. K. Pomerantz, ACS Med. Chem. Lett., 2019, 10, 1648–1654 CrossRef CAS PubMed;
(c) T. D. Downes, S. P. Jones, H. F. Klein, M. C. Wheldon, M. Atobe, P. S. Bond, J. D. Firth, N. S. Chan, L. Waddelove, R. E. Hubbard, D. C. Blakemore, C. De Fusco, S. D. Roughley, L. R. Vidler, M. A. Whatton, A. J.-A. Woolford, G. L. Wrigley and P. O'Brien, Chem.–Eur. J., 2020, 26, 8969–8975 CrossRef CAS PubMed;
(d) F. Lovering, J. Bikker and C. Humblet, J. Med. Chem., 2009, 52, 6752–6756 CrossRef CAS PubMed.
-
(a) H. Braunstein, S. Langevin, M. Khim, J. Adamson, K. Hovenkotter, L. Kotlarz, B. Mansker and T. K. Beng, Org. Biomol. Chem., 2016, 14, 8864–8872 RSC;
(b) J. Garcia, J. Eichwald, J. Zesiger and T. K. Beng, RSC Adv., 2022, 12, 309–318 RSC;
(c) T. K. Beng, J. Kaur, I. S. Anosike, B. Rentfro and S. Newgard, RSC Adv., 2024, 14, 16678–16684 RSC.
-
(a) D. O'Hagan, Nat. Prod. Rep., 2000, 17, 435–446 RSC;
(b) R. D. Taylor, M. MacCoss and A. D. G. Lawson, J. Med. Chem., 2014, 57, 5845–5859 CrossRef CAS PubMed;
(c) E. Vitaku, D. T. Smith and J. T. Njardarson, J. Med. Chem., 2014, 57, 10257–10274 CrossRef CAS PubMed;
(d) D. C. Blakemore, L. Castro and I. Churcher, et al, Nat. Chem., 2018, 10, 383–394 CrossRef CAS PubMed;
(e) P. Goel, O. Alam and M. J. Naim, et al, Eur. J. Med. Chem., 2018, 157, 480–502 CrossRef CAS PubMed;
(f) A. Trowbridge, S. M. Walton and M. J. Gaunt, Chem. Rev., 2020, 120, 2613–2692 CrossRef CAS.
-
(a) Z. Amara, J. Caron and D. Joseph, Nat. Prod. Rep., 2013, 30, 1211–1225 RSC;
(b) T. K. Beng, H. Takeuchi, M. Weber and R. Sarpong, Chem. Commun., 2015, 51, 7653–7656 RSC;
(c) K. Kubota, Y. Watanabe, K. Hayama and H. Ito, J. Am. Chem. Soc., 2016, 138, 4338–4341 CrossRef PubMed;
(d) B. Qu, H. P. R. Mangunuru, X. Wei, K. R. Fandrick, J.-N. Desrosiers, J. D. Sieber, D. Kurouski, N. Haddad, L. P. Samankumara, H. Lee, J. Savoie, S. Ma, N. Grinberg, M. Sarvestani, N. K. Yee, J. J. Song and C. H. Senanayake, Org. Lett., 2016, 18, 4920–4923 CrossRef PubMed;
(e) J. J. Topczewski, P. J. Cabrera, N. I. Saper and M. S. Sanford, Nature, 2016, 531, 220–224 CrossRef PubMed;
(f) T. Sandmeier, S. Krautwald and E. M. Carreira, Angew. Chem., 2017, 129, 11673–11677 CrossRef;
(g) W. Chen, L. Ma, A. Paul and D. Seidel, Nat. Chem., 2018, 10, 165–169 CrossRef CAS PubMed;
(h) J. B. Roque, Y. Kuroda, L. T. Göttemann and R. Sarpong, Nature, 2018, 564, 244–248 CrossRef CAS;
(i) A. Paul and D. Seidel, J. Am. Chem. Soc., 2019, 141, 8778–8782 CrossRef CAS;
(j) I. S. Hassan, A. N. Ta, M. W. Danneman, N. Semakul, M. Burns, C. H. Basch, V. N. Dippon, B. R. McNaughton and T. Rovis, J. Am. Chem. Soc., 2019, 141, 4815–4819 CrossRef CAS;
(k) A. Millet, P. Larini, E. Clot and O. Baudoin, Chem. Sci., 2013, 4, 2241–2247 RSC;
(l) C. J. Cordier, R. J. Lundgren and G. C. Fu, J. Am. Chem. Soc., 2013, 135, 10946–10949 CrossRef CAS PubMed;
(m) A. McNally, C. K. Prier and D. W. C. MacMillan, Science, 2011, 334, 1114–1117 CrossRef PubMed;
(n) T. K. Beng and D. P. Bassler, Tetrahedron Lett., 2014, 55, 6662–6664 CrossRef;
(o) D. P. Bassler, A. Alwali, L. Spence, O. Beale and T. K. Beng, J. Organomet. Chem., 2015, 780, 6–12 CrossRef;
(p) T. K. Beng, S. Langevin, H. Braunstein and M. Khim, Org. Biomol. Chem., 2016, 14, 830–834 RSC;
(q) T. K. Beng, A. W. V. Silaire, A. Alwali and D. P. Bassler, Org. Biomol. Chem., 2015, 13, 7915–7919 RSC;
(r) T. K. Beng, N. Fox, D. P. Bassler, A. Alwali, K. Sincavage and A. W. V. Silaire, Org. Biomol. Chem., 2015, 13, 8647–8651 RSC;
(s) B. Li, F. Yu, W. Chen and D. Seidel, Org. Lett., 2024, 26, 5972–5977 CrossRef CAS;
(t) N. A. Frolov and A. N. Vereshchagin, Int. J. Mol. Sci., 2023, 24, 2937 CrossRef CAS PubMed.
|
This journal is © The Royal Society of Chemistry 2024 |
Click here to see how this site uses Cookies. View our privacy policy here.