DOI:
10.1039/D4RA03951C
(Paper)
RSC Adv., 2024,
14, 27241-27251
Synthesis and characterization of macrodiols and non-segmented poly(ester-urethanes) (PEUs) derived from α,ω-hydroxy telechelic poly(ε-caprolactone) (HOPCLOH): effect of initiator, degree of polymerization, and diisocyanate†
Received
29th May 2024
, Accepted 19th August 2024
First published on 27th August 2024
Abstract
Nine different macrodiols derived from α,ω-hydroxy telechelic poly(ε-caprolactone) (HOPCLOH) were prepared by ring-opening polymerization of ε-caprolactone (CL) using three linear aliphatic diols (HO–(CH2)n–OH, where n = 4, 8, and 12) as initiators and catalyzed by ammonium decamolybdate (NH4)8[Mo10O34]. The crystallization temperature (Tc) and crystallinity (xi) were relatively high for HOPCLOH species with a long aliphatic chain [–(CH2)12–] in the oligoester. Also, HOPCLOH was the precursor of twenty-seven different poly(ester-urethanes) (PEUs) with various degrees of polymerization (DP) of HOPCLOH and three types of diisocyanates such as 1,6-hexamethylene diisocyanate (HDI), methylene diphenyl diisocyanate (MDI), and 4,4′-methylenebis (cyclohexyl isocyanate) (HMDI). HOPCLOH exhibited the melting temperature (Tm) and crystallinity (xi) with a proportional dependency to the degree of polymerization (DP). PEUs showed significant thermal and mechanical properties, which had a direct correlation in terms of the type of DP and diisocyanate. PEUs derived from HDI versus MDI or HMDI exhibited an apparent effect where aliphatic diisocyanate (HDI) induced a significant xi with respect to aromatic and cyclic diisocyanate (MDI or HMDI). The profile of PEUs films according to mechanical properties is mainly a plastic behavior. The chemical nature and properties of HOPCLOH and PEUs were characterized by NMR, FT-IR, GPC, MALDI-TOF, DSC, and mechanical properties.
Introduction
Poly(ε-caprolactone) (PCL) is a biodegradable aliphatic polyester [–CO–(CH2)5–O–]n generally synthesized by ring-opening polymerization (ROP) of ε-caprolactone (CL).1,2 PCL is a hydrophobic and semi-crystalline polyester whose crystallinity tends to decrease as its molecular weight increases. The characteristics of PCL, such as solubility, low melting point (59–64 °C), biodegradability, and mixture compatibility, have stimulated extensive research into its potential application in the biomedical field3 as a controlled drug delivery vehicle,4 porous and fibrous scaffolds,5 or implants.6
Ring-opening polymerization (ROP) of CL is the common route to synthesize PCL, where a metallic or non-metallic catalyst is used, and an alcohol or amine is employed as an initiator.7,8 The main limitation to defining the type of architecture of the PCL produced is the initiator; for example, primary alcohols (R–OH) can result in monofunctional PCLs with an α-hydroxyl-ω-alkyl terminal group (R–PCL–OH)9,10 and diols (HO–R–OH) are suitable initiators to obtain α, ω-hydroxyl telechelic PCLs (HO–PCL–R–PCL–OH) (HOPCLOH),11,12 and these α, ω-hydroxyl telechelic PCLs can be chemically modified into analogues.13
α,ω-Telechelic macrodiols such as HOPCLOH can react with a diisocyanate group (OCN–R–NCO) to produce segmented amphiphilic polyurethanes,14 polyurethanes acrylates,15 poly(ester-urethane-amide),16 poly(ester-urethane) with shape-memory application,17 and poly(ester-urethane) (PEU) from miktoarm star copolymer,18 to mention a few. Thermoplastic polyurethanes are characterized by their elasticity, high abrasion, wear, and oxygen resistance, including resistance to oxidizing agents that can cause material breakdown or loss of mechanical properties over time. Another distinctive feature of these thermoplastic polyurethanes is the preservation of mechanical properties at different temperatures, which is why this type of polymer has been incorporated into the area of biomaterials, where PEUs as biodegradable materials derived from PCL,19 PDLLA,20 PLA,21 and some copolymers have been previously reported.22
The most common aliphatic diisocyanates used to obtain PEUs derived from HOPCLOH are 1,6-hexamethylene diisocyanate (HDI),23,24 and 4,4′-methylene dicyclohexyl diisocyanate (HMDI).25,26 On the other hand, in the case of aromatic diisocyanates, 2,4-toluene diisocyanate (TDI)27 and 4,4′-diphenylmethane diisocyanate (MDI) are the most commonly used.28 Diisocyanates play an important role in affecting the mechanical properties of polyurethane materials.29,30 The geometry of diisocyanates (aliphatic, cycloaliphatic, and aromatic) greatly influences the tensile strength and hardness of polyurethane materials.31–33
Polyurethanes synthesized from biodegradable diols have a potential application in biomaterials,34 tissue engineering,35–37 drug transport,38 regeneration membranes,39 and sustainable materials.40 Polyurethanes based on PCL used as memory foam have been prepared so that they have shown improvement in their properties, such as biodegradability and biocompatibility.41–43
In this work, we explored three different factors (Table 1) in the synthesis of PEUs, such as: (1) the type of diol [HO–R–OH, where R = (CH2)m and m = 4, 8, and 12] used as an initiator in the ROP of CL to obtain the HOPCLOH (Scheme 1a); (2) the degree of polymerization (DP) of HOPCLOH, where DP = 5, 10, and 15; and (3) the variation of diisocyanates (HDI, MDI, and HMDI) (Scheme 1b). HOPCLOH and PEUs were characterized by different analytical techniques to visualize their chemical nature and understand the three factors previously described.
Table 1 Chemical species involved in this work: the linear aliphatic diols [HO(CH2)mOH, where m = 4, 8, and 12] used as initiators in the ROP of CL, the poly(ε-caprolactone) diols (HOPCLOH) obtained from this initiators (where n = 5,10, and 15; relating to de degree of polymerization), and the three diisocyanates employed for the preparation of poly(ester-urethanes) (PEUs) derived from poly(ε-caprolactone) diols (HOPCLOH)
Initiator |
Macrodiol |
Diisocyanate |
1,4-Butanediol 1,4-BD |
HOPCL4OH |
HDI |
 |
 |
 |
1,8-Octanediol 1,8-OD |
HOPCL8OH |
MDI |
 |
 |
 |
1,12-Dodecanediol 1,12-DD |
HOPCL12OH |
HMDI |
 |
 |
 |
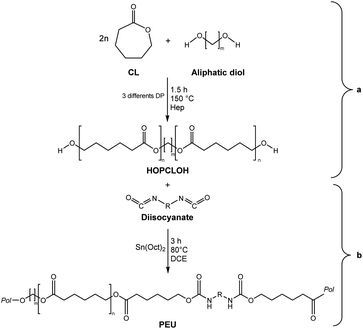 |
| Scheme 1 Synthesis of (a) α,ω-hydroxy telechelic poly(ε-caprolactone) (HOPCLOH), where m = 4, 8 and 12, with three different degrees of polymerization (DP) of 5,10 and 15. Followed by the synthesis of (b) poly(ester-urethane) (PEU) derived from HOPCLOH, where R corresponds to the central segment of the diisocyanate: 1,6-hexamethylene diisocyanate (HDI), methylene diphenyl diisocyanate (MDI), and 4,4′-methylenebis (cyclohexyl isocyanate) (HMDI). | |
Experimental
Materials
ε-Caprolactone (CL) was supplied by Aldrich Chemical Co., dried over calcium hydride (CaH2) for 24 h and distilled under reduced pressure before use. 1,4-Butanediol, 1,8-octanediol, 1,12-dodecanediol, tin(II) 2-ethylhexanoate Sn(Oct)2, 1,6-diisocyanatehexane (HDI), 4,4′-methylene dicyclohexyl diisocyanate (HMDI), methylene diphenyl diisocyanate (MDI), 1,2-dichloroethane (DCE), and ammonium heptamolybdate (NH4)8[Mo10O34]·4H2O (Hep) were purchased from Aldrich Chemical Co. and used without further purification.
Synthesis of α,ω-hydroxy telechelic poly(ε-caprolactone) (HOPCL4a10OH or macrodiol) by (NH4)8[Mo10O34] as a catalyst and HO(CH2)4OH as an initiator
In a dried 25 mL round-bottom flask, ε-caprolactone (CL) (65 mmol, 7.419 g), ammonium heptamolybdate tetrahydrate (NH4)6[Mo7O24]·4H2O (Hep, 2.42 × 10−3 mmol, 3 mg), and 1,4-butanediol (6.5 mmol, 580 mg) were added and heated to reflux by stirring them in an oil bath at 150 °C for 1.5 h (molar ratio CL/Hep = 26
800 and CL/1,4-butanediol = 10). By thermal decomposition in situ of ammonium heptamolybdate (NH4)6[Mo7O24], ammonium decamolybdate (NH4)8[Mo10O34] was obtained in the solid-state [8]. Conversion and number-average molecular weight (Mn) were monitored by 1H NMR. Once the reaction time was over, an aliquot of the crude reaction was dissolved in CDCl3 and analyzed by 1H NMR without purification. The peaks at 2.29 [–CH2–O–, Ipol, repetitive unit of CL] and 3.63 [(–CH2–OH)2, Iter, terminal group or end group] in the 1H NMR spectrum were used to calculate the Mn in two steps: (1) degree of polymerization (DP). DP(NMR) = (Ipol ÷ Iter) × 2. Ipol and Iter corresponded to the integrals of the methylenes obtained by 1H NMR from the polyester [CH2–O] and α,ω-hydroxy [(–CH2–OH)2] terminal group peaks, respectively, and the ×2 was due to the bifunctionality of the polymer, α,ω-hydroxyl telechelic PCL. (2) Number-average molecular weight (Mn). Mn (NMR) = (MW(CL)) (DP(NMR)) + MW (diol), where MW was the molecular weight of the repetitive unit (CL), and diol (1,4-butanediol, 1,8-octanediol or 1,12-dodecanediol), respectively; DP (NMR) was earlier calculated in step (1). Table 2 shows the number-average molecular weight (Mn) obtained by 1H NMR, MALDI-TOF, and GPC, where the Mn calculated was similar to the values of Mn obtained by 1H NMR and MALDI-TOF.
Table 2 Poly(ε-caprolactone) diols (HOPCLOH) prepared using linear aliphatic diols [HO(CH2)mOH, where m = 4, 8, and 12] as initiators in the ROP of CLa,b
Sample |
Initiator |
Alkyl c,d(%) |
PCL c(%) |
DP (calcd) |
DP (NMR)c |
Mn (calcd)e |
Mn (NMR)c,f |
Mn (MALDI)g |
Mn (GPC)h |
Conv. c(%) |
HOPCLOH, α,ω-hydroxy telechelic poly(ε-caprolactone); ROP, ring-opening polymerization; CL, ε-caprolactone; DP, degree of polymerization; PCL, poly(ε-caprolactone). Polymerization at 150° for 90 min with 65 mmol of CL, three CL/initiator molar ratios of 5,10, and 15 were used in the experiments (DP (calcd) = 5, 10, or 15) and ammonium decamolybdate as a catalyst. HOPCLOH = HO–PCL–OH or HO–(CH2)m–PCL–OH, where m = 4, 8, and 12. Determined by 1H NMR in CDCl3. Obtained from the equation alkyl (%) = (MWinitiator/Mn (NMR)) × 100, where MWinitiator is the molecular weight of initiator or alkyl diol (HOROH). Obtained from the equation Mn (calcd) = (MW (CL))(mmol CL/mmol ROH) + MW (HOROH), where MW is the molecular weight of ε-caprolactone monomer or aliphatic diol (HOROH). Obtained from the equation Mn (NMR) = (DP (PCL) × MW (CL)) + MW (ROH), where MW is the molecular weight of ε-caprolactone monomer or aliphatic diol (HOROH). Calculated by MALDI-TOF. Determined by GPC results. |
HOPCL4a5OH |
HO(CH2)4OH |
16 |
84 |
5 |
4.9 |
650 |
570 |
— |
1868 |
98 |
HOPCL4a10OH |
HO(CH2)4OH |
8 |
92 |
10 |
10.0 |
1230 |
1120 |
1254 |
2575 |
98 |
HOPCL4a15OH |
HO(CH2)4OH |
5 |
95 |
15 |
14.7 |
1760 |
1660 |
— |
3300 |
99 |
HOPCL8a5OH |
HO(CH2)8OH |
23 |
77 |
5 |
5.0 |
710 |
640 |
— |
1819 |
99 |
HOPCL8a10OH |
HO(CH2)8OH |
12 |
88 |
10 |
10.1 |
1300 |
1210 |
1314 |
2585 |
99 |
HOPCL8a15OH |
HO(CH2)8OH |
9 |
91 |
15 |
14.0 |
1750 |
1630 |
— |
3184 |
99 |
HOPCL12a5OH |
HO(CH2)12OH |
29 |
71 |
5 |
5.0 |
770 |
690 |
— |
1849 |
99 |
HOPCL12a10OH |
HO(CH2)12OH |
16 |
84 |
10 |
10.1 |
1350 |
1250 |
1366 |
2651 |
99 |
HOPCL12a15OH |
HO(CH2)12OH |
11 |
89 |
15 |
15.0 |
1910 |
1780 |
— |
3716 |
99 |
Mn (calcd) = 1,230, Mn (NMR) = 1120 (conv. = 98%), Mn (GPC) = 2,575, Mw/Mn = 1.33, Mn (MALDI) = 1254. IR (cm−1) 3434 (ν, OH, PCL), 2944 (νas, CH2, PCL), 2865 (νs, CH2, PCL), 1723 (ν, C
O, PCL), 1471 (δs, CH2, PCL), 1164 (νas, C–(C
O)–O, PCL), 1044 (νas, O–C–C, PCL), 732 (ρ, CH2, PCL). NMR data for HOPCL4a10OH. 1H NMR (400 MHz, CDCl3, ppm): δ 4.05 (t, 2H, [CH2O], CL and 1,4-But), 3.63 (t, 4H, [(CH2OH)2], CL and But), 2.29 (t, 2H, (CH2–CO–O–), CL), 1.64 (m, 4H, [(CH2)2], CL), 1.55 (t, 4H, [(CH2)2], But), 1.37 (q, 2H, [CH2], CL). 13C NMR (100 MHz, CDCl3, ppm): …—O–CHf2–CHh2–CHh2–CHg2–O–[C(
O)f–CHa2–CHb2–CHc2–CHd2–CHe2–O]n−1–C(
O)f′–
–OH: δ 173.74 (f′), 173.58 (f), 64.14 (g), 63.79 (e), 62.58 (e′), 34.22 (a′), 34.11 (a), 32.32 (d′), 29.15 (h), 28.34 (d), 25.52 (b), 25.30 (c′), 24.68 (b′), 24.57 (c).
Synthesis of poly(ester-urethane) (PEU4a10A) derived of poly(ε-caprolactone) diol (HOPCL4a10OH) and 1,6-hexamethylene diisocyanate (HDI)
The reaction was carried out in a 25 mL round-bottom flask previously dried, 2.0 g (1.787 mmol) of HOPCL4a10OH [Mn (NMR) = 1120] was added [it was assumed that 8% of unreacted diol (HO–R–OH) was present in polymer samples with DP = 10 and DP = 15, and in the case of DP = 5 a 15% of unreacted diol (HO–R–OH) was presumed, so this fraction was contemplated in the calculation to obtain the molecular weight of the macrodiol]. In a previous contribution,12 unreacted diol (diethylene glycol) (DEG) was detected, according to previous results the macrodiol or polyol called HOPCLOH had a significant amount of unreacted initiator DEG when the degree of polymerization (DP) is lower, and this percentage is decreasing to relatively higher DP. In this sense, in this contribution the value of unreacted diol was 15% for lower DP (5) and 8% for medium and high DP (10 and 15). Subsequently, 333 mg (1.985 mmol) of HDI [HOPCL4a10OH:HDI molar ratio = 1
:
1.1] was added and dissolved in 8 mL of 1,2-dichloroethane (DCE) [0.21 M with respect to HOPCL4a10OH], and tin(II) 2-ethylhexanoate [Sn(Oct)2] was added as catalyst [1 wt%, 32 mg ∼ 3 drops]. Then, the flask was placed in an oil bath at 80 °C for 3 hours. After the reaction time, a PEU4a10A film was obtained by casting at room temperature on a leveled Teflon surface within a fume cupboard covered with a conical funnel to protect it from dust and allow a slow solvent evaporation for 12 h. After, the PEU film was released and dried under a vacuum. Following the same methodology, 27 different PEUs were synthesized.
IR (cm−1): 3322 (ν, N–H, urethane), 2933 (νas, CH2, PCL), 2859 (νs, CH2, PCL), 1728 (ν, C
O, PCL), 1685 (ν, C
O, urethane), 1534 (δ, N–H, urethane), 1463 (δs, CH2, PCL), 1161 (νas, C–(C
O)–O, PCL), 1095 (νas, O–C–C, PCL), 733 (ρ, CH2, PCL). NMR data for PEU4a10A. 1H NMR (400 MHz, CDCl3, ppm): δ 4.73 (s, 1H, [NH], urethane), 4.05 (t, 2H, [CH2O], CL and 1,4-But), 3.13 (t, 4H, [(CH2OCONH)2], urethane), 2.29 (t, 2H, (–CH2–CO–O–), CL), 1.63 (m, 4H, [(CH2)2], CL), 1.63 (t, 2H, [CH2], But), 1.48 (t, 2H, [CH2], urethane), 1.37 (q, 2H, [CH2], CL and But). 13C NMR (100 MHz, CDCl3, ppm): …—O–CHf2–CHh2–CHh2–CHg2–O–[C(
O)f–CHa2–CHb2–CHc2–CHd2–CHe2–O]n−1–C(
O)f–
–O–C(
O)i–NH–CHj2–CHk2–CHl2–CHl2–CHk2–CHj2–NH: δ 173.65 (f), 156.86 (i), 64.65 (e′), 64.25 (g), 63.90 (e), 40.77 (j), 34.16 (a′), 34.11 (a), 29.91 (h), 28.75 (l), 28.34 (d), 25.52 (b), 25.31 (c′), 24.63 (b′), 24.57 (c).
All macromolecular species were named according to the next indications: macrodiols were named as follows; HOPCL4a10OH: “4” symbolizes the size of the initiator (such as 1,4-butanediol), “a” denotes aliphatic diol, and “10” represents the ε-caprolactone (CL) degree of polymerization (DP). For PEUs; PEU4a10A, B, and C: “A, B, and C” stand for the various diisocyanate types that were utilized (A for HDI, B for MDI, and C for HMDI); “4a10” refers to the matching macrodiol, HOPCL4a10OH as product (Table 2) or precursor of PEU4a10A (Table 4). To visualize the pattern of samples, see Tables S1 and S2.†
Characterization methods
Nuclear magnetic resonance (NMR). 1H and 13C NMR were recorded at room temperature on a Varian Inova or Mercury 400 MHz (400 MHz 1H and 100 MHz 13C). CDCl3 was used as a solvent, and all spectra were referenced to the residual solvent CDCl3 [δ (ppm) 7.26 (1H) and 77.0 (13C)]. Fourier Transform Infrared Spectroscopy (FT-IR). HOPCLOHs and PEUs films were recorded with an attenuated total reflectance spectroscopy (ATR) accessory in a PerkinElmer Spectrum One FT-IR spectrometer. Differential Scanning Calorimetry (DSC). Thermograms were performed in a Mettler Toledo DSC822e instrument. Three scans were obtained with two heating (25–80 °C and −90–80 °C) and one cooling (80–−90 °C) between them, at a rate of 10 °C min−1 and under a nitrogen purge. Gel permeation chromatography (GPC). The case for HOPCLOH: GPC measurements were determined using a Waters gel permeation chromatograph equipped with a Waters 1515 isocratic high-performance liquid chromatography (HPLC) pump and a Waters 2414 refractive index (RI) detector. A set of three Waters columns conditioned at 35 °C were used to elute samples at a flow rate of 1 mL min−1 of HPLC grade tetrahydrofuran (THF). Polystyrene standards (polymer laboratories) were used for calibration.
Matrix-assisted laser desorption ionization time-of-flight (MALDI-TOF). MALDI-TOF spectra were recorded in the linear mode by using a Voyager DE-PRO time-of-flight mass spectrometer (Applied Biosystems) equipped with a nitrogen laser emitting at λ = 337 nm with a 3 ns pulse width and working in positive ion mode and delayed extraction. A high acceleration voltage of 20 kV was employed. 2,5-Dihydroxybenzoic acid (DHB) at a concentration of 10 mg mL−1 in acetonitrile was used as a matrix. Samples were dissolved in acetonitrile and mixed with the matrix at a molar ratio of approximately 1
:
100. Mechanical properties. The mechanical properties were measured in an MTS testing machine equipped with a 100 N load cell. Type 3 dumbbell test pieces (according to ISO 37) were cut from the films. A crosshead speed of 200 mm min−1 was used. The strain was measured from crosshead separation and referred to a 12 mm initial length. At least four samples were evaluated for each PEU.
Results and discussion
Part 1. α,ω-hydroxy telechelic poly(ε-caprolactone) (HOPCLOH) using diols as initiators
A series of α,ω-hydroxy telechelic poly(ε-caprolactone)s (HOPCLOHs) (Scheme 1a) were synthesized by ring-opening polymerization (ROP) of ε-caprolactone (CL) via bulk polymerization with an (NH4)8[Mo10O34] as a catalyst, in the presence of three different aliphatic diols as initiators [HO–(CH2)m–OH, where m = 4, 8 and 12] and obtaining a family of HOPCLOH [HO–PCL–O–(CH2)m–O–PCL–OH, m = 4, 8 and 12, with a degree of polymerization (DP) of 5, 10 and 15] (Table S1†). The purpose in the preparation of these species is to understand the effect of the length of the substituents linear aliphatic group (AG) on the physical properties (thermal properties) of the HOPCLOH and, eventually, in their poly(ester-urethanes) (PEUs) the effect on thermal and mechanical properties. Typically, the ROP of the CL was achieved in the presence of the catalyst mentioned previously and 1,4-butanediol, and after 90 minutes at 150 °C, a high conversion (98%) was detected by 1H NMR (Table 2). The CL/HO–(CH2)m–OH feed of molar ratio allowed control of degree polymerization on the HOPCLOH. By means of three analytical techniques such as NMR, GPC, and in some cases MALDI-TOF, the experimental values of Mn were acquired, showing an expected difference between Mns values due to the different analytical techniques, as shown in Fig. 1. Mn (NMR) values are approximating to Mn (calcd), and Mn (NMR) values were preferably used in our calculations [second step, synthesis of PEUs] because the samples have a moderate polydispersity (not narrow), and all of them are oligomers (oligoesters) with relatively high contribution by their end groups (detected by 1H NMR). NMR, MALDI-TOF, and GPC; overall, these results followed the next pattern: Mn (NMR) < Mn (calcd) < Mn (MALDI) < Mn (GPC). The overestimation of Mn determined by GPC for the HOPCLOHs was attributable to the polystyrene standards used in the calibration curve. Moderate polydispersity and a unimodal distribution were observed in all GPC chromatograms (Mw/Mn = 1.18–1.39) (Table S3†).
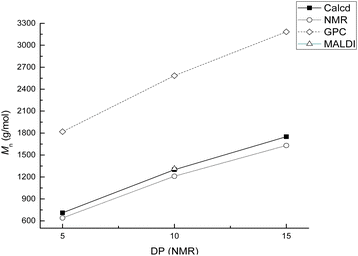 |
| Fig. 1 Molecular weight (Mn) of poly(ε-caprolactone) diol (HOPCLOH) prepared using the linear aliphatic diol HO(CH2)8OH as an initiator in the ROP of CL with degree polymerization (DP) determined by 1H NMR in CDCl3. This data was obtained by NMR, GPC, and MALDI-TOF and compared with the calculated Mn. | |
By NMR, the chemical essence of HOPCLOH samples was determined; for example, in Fig. 2, the 1H NMR spectrum of HOPCL8a10OH is shown, in which signals at 4.04 and 3.62 ppm were assigned to methylenes of the main chain of PCL [d, e, CH2–O–] and methylenes of the hydroxyl end group [d′, e′, CH2–OH][9], respectively. In the PCL, the methylene group (–CH2–CH2–) had an insertion as a monosubstitution [e′ and e, HO–CH2(e′)–(CH2)6–CH2(e)–O, δ 3.62 (e′) and 4.04 (e)] or bisubstitution [e, O–CH2(e)–(CH2)6–CH2(e)–O, δ 4.04]. In the 13C NMR spectrum for HOPCL4a10OH [Fig. 3(a and c)], a series of peaks for carbonyl (ester group) (173.66 ppm), methylenes of the main chain (64.25 ppm), and methylene attached to terminal hydroxyl groups (62.69 ppm) were detected, confirming an α,ω-hydroxy telechelic PCL.12 Previous reports corroborate the peak assignments for HOPCLOH in the 1H and 13C NMR spectra.9,12,43
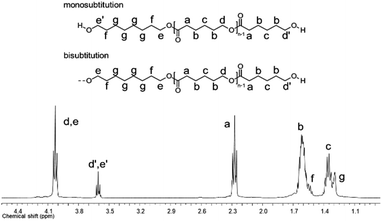 |
| Fig. 2 1H NMR (400 MHz) spectrum in CDCl3 at room temperature for HOPCL8a10OH. | |
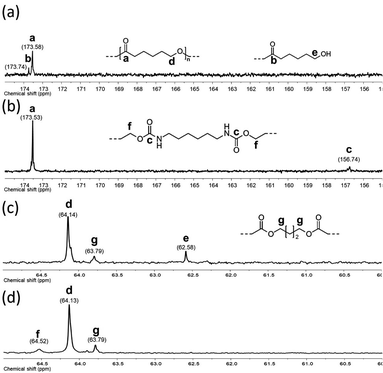 |
| Fig. 3 13C NMR (100 MHz) spectra in CDCl3 for: HOPCL4a10OH (a and c) and for PEU4a10A (b and d) at room temperature. | |
Fig. 4 shows the MALDI-TOF spectrum of HOPCLOH using diol 1,12-dodecanodiol as initiator; the zone corresponded to fragments with 6–10 CL repeat units (Na+ and K+ ions), showing a characteristic pattern of a unimodal distribution of a HOPCLOH oligoester with a systematic increase in the DP. The most intense peaks were due to HOPCLOH (linear, L) fragments doped with Na+; the K+ doped peaks were the next in intensity. Peaks with low intensity were attributable to macrocyclic species of ε-caprolactone (CL)n (cyclic, C). It is known that (CL)n is a product of intramolecular transesterification.44 The peaks with very low intensity were ascribed to species such as α-hydroxyl-ω-(carboxylic acid) poly(ε-caprolactone) and α-hydroxyl-ω-(sodium carboxylate) PCL, the carboxylic acid group (–CO2H) end groups were related to traces of water as an initiator in the ROP of CL, and the carboxylate group (–CO2–) was produced in situ during the MALDI-TOF experiment.43 The MALDI-TOF spectra for the HOPCLOH samples using 1,4-butanediol or 1,8-octanediol as initiator, showed similar patterns (Fig. S4 and S5 of the ESI File†).
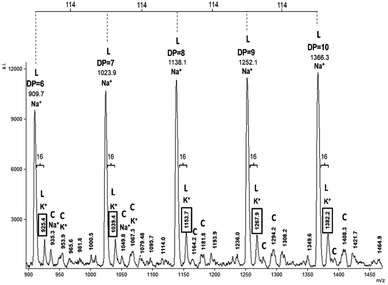 |
| Fig. 4 MALDI-TOF spectrum (linear mode) expanded view for the 890–1500 m/z fragments of HOPCL12a10OH (note: 114 and 16 are the values of the molecular weight of CL and the difference between Na+ and K+ (doping the same species of polymer), respectively). | |
Effect of the degree of polymerization (DP) on α,ω-hydroxy telechelic poly(ε-caprolactone) (HOPCLOH). The degree of polymerization (DP) of the α,ω-hydroxy telechelic poly(ε-caprolactone)s (HOPCLOHs) was a function of the CL/HO–(CH2)m–OH feed molar ratio, to observe the effect of the DP on the HOPCLOH, three DPs were studied (DP = 5, 10, and 15).Thermal properties of HOPCLOH were analyzed by Differential Scanning Calorimetry (DSC) (Table 3), where a systematic increase in the DP (DP = 5, 10, and 15) of each family of oligoesters (HOPCL4OH), (HOPCL8OH), and (HOPCL12OH) is visualized.
Table 3 Thermal properties of poly(ε-caprolactone) diols (HOPCLOH) with different types of linear aliphatic diols [HO(CH2)mOH, where m = 4, 8, and 12] as initiators in the ROP of CL.a,b The effect of the degree polymerization (DP) on the thermal properties
Sample |
Initiator |
DP (NMR)c |
Alkyl c,d(%) |
Tc e(°C) |
Tm e(°C) |
ΔHm e(J g−1) |
ΔHmPCL f(J g−1) |
xi g(%) |
Effect of the number of methylenes in HOPCLOH. HOPCLOH,α,ω-hydroxy telechelic poly(ε-caprolactone); ROP, ring-opening polymerization; CL, ε-caprolactone; PCL, poly(ε-caprolactone); DSC, differential scanning calorimetry. HOPCLOH = HO–PCL–OH or HO–(CH2)m–PCL–OH, where m = 4, 8, and 12. Determined by 1H NMR in CDCl3. Calculated from the equation alkyl (%) = (MWinitiator/Mn (NMR)) × 100, where MWinitiator is the molecular weight of initiator or alkyl diol (HOROH). Obtained by DSC analysis. Calculated from the equation ΔHmPCL = ΔHm − (ΔHm·xalkyl) where xalkyl is the weight fraction of alkyl group in the HOCLOH oligoester. Quantified from ΔHm. |
HOPCL4a5OH |
HO(CH2)4OH |
4.9 |
16 |
4 |
17 |
66 |
66 |
49 |
HOPCL4a10OH |
HO(CH2)4OH |
10.0 |
7 |
19 |
34 |
72 |
72 |
53 |
HOPCL4a15OH |
HO(CH2)4OH |
14.7 |
5 |
24 |
39 |
79 |
79 |
58 |
HOPCL8a5OH |
HO(CH2)8OH |
5.0 |
23 |
9 |
19 |
80 |
62 |
46 |
HOPCL8a10OH |
HO(CH2)8OH |
10.1 |
11 |
19 |
33 |
73 |
65 |
48 |
HOPCL8a15OH |
HO(CH2)8OH |
14.0 |
9 |
22 |
39 |
79 |
72 |
53 |
HOPCL12a5OH |
HO(CH2)12OH |
5.0 |
29 |
14 |
23 |
105 |
74 |
54 |
HOPCL12a10OH |
HO(CH2)12OH |
10.1 |
15 |
24 |
35 |
93 |
79 |
58 |
HOPCL12a15OH |
HO(CH2)12OH |
15.0 |
11 |
28 |
41 |
88 |
78 |
58 |
The weight percent of the alkyl group was decreased from HOPCL4a5OH (16%) to HOPCL4a15OH (5%), and it had an inversely proportional effect on the DP; this effect was similarly repeated in the rest of HOPCLOHs. The values of melting temperatures (Tm) and crystallinity (xi) increased proportionally to the DP (Fig. 5); this was because the crystalline microdomains of the PCL increased when the main chain of the oligoester was longer, favoring the lamellar thickness. The melting temperature (Tm) exhibited a pronounced double peak for HOPCLOH samples with DP = 5 (Fig. 6), this profile could be understood as two different sizes of crystallites in two different environments, some found in a more amorphous region (which will have a slightly lower Tm) and others in more crystalline areas (which will have a higher Tm). Additionally, this effect of the double peak in the Tm of HOPCLOH samples with DP = 5 could be attributed to a disruption of the crystal domains of PCL at low DP and the effect of the monosubstitution fraction visualized by NMR.
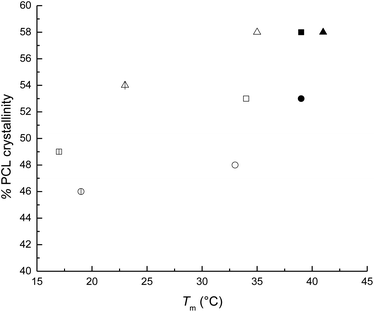 |
| Fig. 5 Dependence of melting point (Tm) and crystallinity (xi) in macrodiols (HOPCLOH). The macrodiols with HO(CH2)4OH as initiator are indicated with squares ( DP = 5; □ DP = 10, and ■ DP = 15); macrodiols with HO(CH2)8OH as initiator are indicated with circles ( DP = 5, ○ DP = 10, and ● DP = 15) and macrodiols with HO(CH2)12OH as initiator are indicated with triangles ( DP = 5, △ DP = 10, and ▲ DP = 15). | |
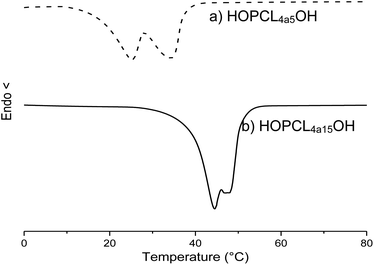 |
| Fig. 6 DSC thermograms of macrodiols with HO(CH2)4OH as initiator and (a) DP = 5 (HOPCL4a5OH), and (b) DP = 15 (HOPCL4a15OH), showing a double peak of melting temperature (Tm) Table 3. | |
Impact of the number of methylenes of the initiator [(CH2)m] on α,ω-hydroxy telechelic poly(ε-caprolactone) (HOPCLOH). All samples were synthesized with similar number-average molecular weight (Mn) taking in consideration the degree of polymerization (Mn) (Mn(calcd) = 600–800 Da, DP = 5; Mn (calcd) = 1200–1400 Da, DP = 10; Mn (calcd) = 1600–1800 Da, DP = 15). This allowed us to compare the effect of methylene (CH2)m groups of three different types of linear aliphatic diols with a systematic increase in the number of methylenes [HO(CH2)mOH, where m = 4, 8, and 12] used as initiators to synthesize the HOPCLOHs. For example, in the case of a DP = 5, the weight percent of the alkyl group increased when the number of methylenes [(CH2)m] changed from 4 to 12, from HOPCL4a5OH (16%) to HOPCL12a5OH (29%), evidencing the effect of the length of the weight percentage of the alkyl group in the oligoester, since when the methylene chain increased, the crystallization temperature (Tc) and melting temperature (Tm) increased proportionally, from HOPCL4a5OH (Tc = 4 °C; Tm = 17 °C) to HOPCL12a5OH (Tc = 14 °C; Tm = 23 °C). The number of methylenes in the initiator (CH2)m influenced the Tc, where –(CH2)12– induced a relative high value in the series in the HOPCLOH oligoester species, which can be appreciated in Fig. 7. This effect was attributed to the crystalline domain of the 12-methylene segment inducing a nucleation effect. Complementarily, the number of methylenes (initiator) inserted in the main chain of the polyester also had a significant effect on the crystallinity (xi) of HOPCLOH, in the case of DP = 5 from 49 [(CH2)4] to 54% [(CH2)12], this is consistent with the profile of Tm. So, a long aliphatic chain of methylenes can induce an increase in the crystallinity of PCL.
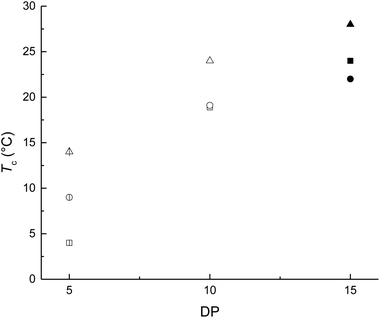 |
| Fig. 7 Dependence of crystallization temperature (Tc) and degree of polymerization (DP) in macrodiols (HOPCLOH). The macrodiols with HO(CH2)4OH as initiator are indicated with squares ( DP = 5; □ DP = 10, and ■ DP = 15); macrodiols with HO(CH2)8OH as initiator are indicated with circles ( DP = 5, ○ DP = 10, and ● DP = 15) and macrodiols with HO(CH2)12OH as initiator are indicated with triangles ( DP = 5, △ DP = 10, and ▲ DP = 15). | |
Part 2. Poly(ester-urethanes) (PEUs)
PEUs were synthesized using the HOPCLOH samples and three different diisocyanates (1,6-hexamethylene diisocyanate (HDI), 4,4′-methylene dicyclohexyl diisocyanate (HMDI), and methylene diphenyl diisocyanate (MDI)) (Scheme 1b). In a typical reaction, HOPCL4a10OH and diisocyanate (HDI, HMDI, or MDI) reacted with a molar ratio of 1
:
1.1, respectively, in the presence of tin(II) 2-ethylhexanoate [Sn(Oct)2] as a catalyst dissolved in 1,2-dichloroethane (DCE) solvent at 80 °C for 3 h. Afterwards, a film was obtained by casting at room temperature to allow slow solvent evaporation for 12 h. This methodology was used to prepare all twenty-seven PEUs samples, varying the number of methylenes [(–CH2–)m, where m = 4, 8, and 12] of the aliphatic group in the HOPCLOH, the degree of polymerization, and the type of diisocyanate used (Table S3†). Fig. 8 shows the 1H NMR spectrum of PEU4a10A, signals at 3.13 and 1.48 ppm were assigned to methylenes of the urethane chain [y, –O–(C
O)–HN–CH2(x)–CH2(y)–], respectively. Also, signals at 4.05 and 2.29 ppm were assigned to methylenes of the PCL main chain [d, CH2–O–], and methylenes next to the carbonyl [a, CH2–C
O], respectively. To corroborate the chemical essence of PEUs, 13C NMR spectra for PEU4a10A, PEU4a10B, and PEU4a10C were obtained (Fig. S1–S3†), confirming the functionality of the PEUs. Comparing the 13C NMR spectrum of the oligomer HOPCL4a10OH (Fig. 3(a)) with one of their PEU with HDI as diisocyanate, PEU4a10A [Fig. 3(b)], carbonyl peaks at 173.65 (a) and 156.86 (c) ppm attributed to ester and urethane groups, respectively, confirmed the functionality of the PEUs. In the PEU4a10A precursor [Fig. 3(c)] there was the typical peak of methylene attached to a hydroxyl group (e, –CH2–OH), which was absent in the PEU4a10A [Fig. 3(d)] due to its reaction with the diisocyanate to produce urethane groups (f, –CH2–O–CO–NH–).
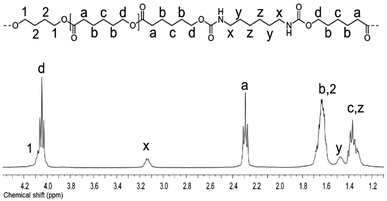 |
| Fig. 8 1H NMR (400 MHz) spectrum in CDCl3 at room temperature for PEU4a10A. | |
The FTIR spectrum for PEU8a10A [Fig. 9(b)] presented distinctive bands at 3322, 1683, and 1536 cm−1 ascribed to vibrations of the urethane group (–O–C
O–NH) with N–H (ν, stretching) C
O (ν, stretching) and N–H (δ, bending), respectively. Also, a band assigned [Fig. 9(a)] to the carbonyl of the ester group in PCL (1721–1723 cm−1, ν, stretching) was identified. In the twenty-seven PEU samples, signals of unreacted diisocyanate (HDI, MDI or HMDI) (∼2270–2250 cm−1) were not observed, confirming the formation of urethane groups.
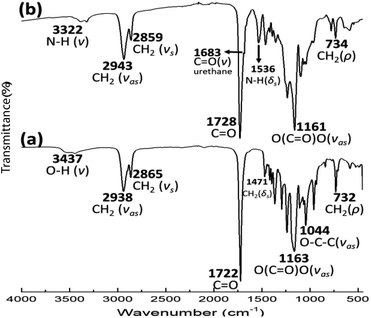 |
| Fig. 9 FT-IR spectra of (a) HOPCL8a10OH and (b) PEU8a10A. | |
Influence of the degree of polymerization (DP) and the number of methylenes of the initiator of HOPCLOH on PEUs. The effects of different degrees of polymerization (DP) in the HOPCLOH used for the synthesis of PEU were analyzed regarding their thermal properties (physical properties) using scanning differential calorimetry (DSC). DSC analysis for all PEU samples with HDI and PEUs derived from HOPCL4OH with MDI and HMDI is presented in Table 4. The comparison of HOPCLOH with respect to PEUs demonstrated a decrease in the enthalpy of fusion (ΔHm) and crystallinity (xi) of PCL microdomains, following the same pattern as the HOPCLOH samples, that is, by increasing the DP in each HOPCLOH with different aliphatic diols, ΔHm and xi increased. The decrease in xi of PCL was largely attributed to the presence of physical cross-links of the urethane groups in the main chain of PEU.35 PEU samples synthesized with HDI presented two different transitions: (1) glass transition temperature (Tg) and (2) melting temperature (Tm). Fig. 10 shows the influence of the DP of HOPCLOH macrodiols on the Tg of PEUs, showing that the DP had a significant effect, where the Tg decreased inversely proportional to the DP, according to the repetitive units in PCL increase. This involves: (1) more flexibility attributed to the methylenes [–CO(CH2)5O–] in the main chain of PCL, which produced a PEU with a lower Tg from DP = 5 to 15; and (2) the hydrogen bonding interactions induced by urethane groups were reduced by the steric hindrance of long PCL chains (DP 15). Usually, the classic value of Tg for the PCL is −60 °C; therefore, all PEUs in Fig. 10 have high Tg values with respect to the regular PCL homopolymer, indicating that PEUs are in principle a more rigid material than PCL. The pattern of Tg of the PEUs with respect to the diisocyanate used as a precursor is MDI (aromatic) > HMDI (cyclic aliphatic) > HDI (linear aliphatic). So, MDI produced a PEU that was more rigid due to the aryl groups; on the other hand, HDI produced a PEU that was more flexible due to the six linear methylenes.
Table 4 Thermal properties of poly(ester-urethanes) (PEUs) derived from poly(ε-caprolactone) diols (HOPCLOH) and three different types diisocyanates (1,6-hexamethylene diisocyanate (HDI), methylene diphenyl diisocyanate (MDI), and 4,4′-methylenebis (cyclohexyl isocyanate) (HMDI)). Effect of the number of methylenes in HOPCLOH on the PEUa,b
Sample |
Precursor |
HS c,e(%) |
SS d,e(%) |
Tg f(°C) |
Tc f(°C) |
Tm f(°C) |
ΔHm f(J g−1) |
ΔHmPCL g(J g−1) |
χi h(%) |
Alkyl i(%) |
HOPCLOH,α,ω-hydroxy telechelic poly(ε-caprolactone); PCL, poly(ε-caprolactone); PEU, poly(ester-urethane); HS, hard segment; SS, soft segment; A, 1,6-hexamethylene diisocyaanate (HDI); B, methylene diphenyl diisocyanate (MDI); C, 4,4′-methylenebis (cyclohexyl isocyanate) (HMDI). DSC, differential scanning calorimetry. HOPCLOH = HO–PCL–OH or HO–(CH2)m–PCL–OH, where m = 4, 8, and 12. Hard segment. Soft segment. Weight percent. Obtained by DSC analysis. Calculated from the equation ΔHmPCL = ΔHm·xss where xss is the weight fraction of soft segment in the HOCLOH oligoester. Quantified from ΔHm. Calculated with respect to the HOPCLOH precursor. Percent determined by 1H NMR in CDCl3. Calculated from the equation alkyl (%) = (MWinitiator/Mn(NMR)) × 100, where MWinitiator is the molecular weight of initiator or alkyl diol (HOROH). |
PEU4a5A |
HOPCL4a5OH |
24 |
76 |
−49 |
— |
51 |
10 |
10 |
7 |
16 |
PEU4a10A |
HOPCL4a10OH |
14 |
86 |
−57 |
— |
31 |
15 |
15 |
11 |
8 |
PEU4a15A |
HOPCL4a15OH |
10 |
90 |
−57 |
— |
43 |
29 |
29 |
22 |
5 |
PEU8a5A |
HOPCL8a5OH |
23 |
77 |
−49 |
— |
51 |
13 |
10 |
7 |
23 |
PEU8a10A |
HOPCL8a10OH |
13 |
87 |
−58 |
— |
30 |
19 |
16 |
12.5 |
12 |
PEU8a15A |
HOPCL8a15OH |
10 |
90 |
−61 |
— |
41 |
31 |
28 |
20 |
9 |
PEU12a5A |
HOPCL12a5OH |
22 |
78 |
−47 |
— |
58 |
15 |
12 |
9 |
29 |
PEU12a10A |
HOPCL12a10OH |
13 |
87 |
−58 |
— |
36 |
19 |
16 |
12 |
16 |
PEU12a15A |
HOPCL12a15OH |
10 |
90 |
−58 |
— |
46 |
29 |
26 |
19 |
11 |
PEU4a5B |
HOPCL4a5OH |
33 |
67 |
−17 |
— |
— |
— |
— |
— |
16 |
PEU4a10B |
HOPCL4a10OH |
20 |
80 |
−40 |
— |
— |
— |
— |
— |
8 |
PEU4a15B |
HOPCL4a15OH |
14 |
86 |
−48 |
−3 |
35 |
25 |
25 |
18 |
5 |
PEU4a5C |
HOPCL4a5OH |
33 |
67 |
−22 |
— |
— |
— |
— |
— |
16 |
PEU4a10C |
HOPCL4a10OH |
21 |
79 |
−49 |
— |
— |
— |
— |
— |
8 |
PEU4a15C |
HOPCL4a15OH |
15 |
85 |
−54 |
3 |
37 |
20 |
20 |
15 |
5 |
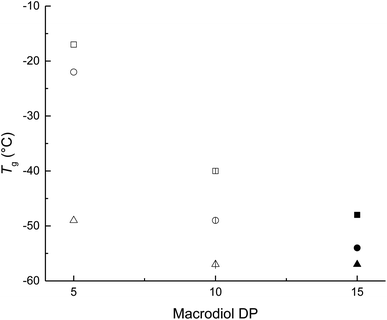 |
| Fig. 10 Effect of the degree of polymerization (DP) of the macrodiol on the glass transition temperature (Tg) in poly(ester-urethanes) (PEUs) derived from poly(ε-caprolactone) diol (HOPCLOH) with HO(CH2)4OH as initiator and three different diisocyanates (1,6-hexamethylene diisocyanate (HDI) indicated with triangles, methylene diphenyl diisocyanate (MDI) indicated with squares, and 4,4′-methylenebis (cyclohexyl isocyanate) (HMDI) indicated with circles). The PEUs derived from the macrodiol HOPCL4aOH with DP = 5 are indicated with open figures □, △, and ○ ; PEUs derived from macrodiols with DP = 10 are indicated with lined figures , , and ; and PEUs derived from macrodiols with DP = 15 are indicated with filled figures ■, ▲, and ●. | |
The Tm in PEUs is mainly attributed to the crystal domain of PCL soft segments, in the case of PEU10A derived from HDI and HOPCLOH exhibited a decrease of Tm respect to PEU5A and PEU15A. This phenome can be explained in terms of DP, where DP = 10 of HOPCLOH after its reaction induces a dispersion of the lamella crystalline domains of PCL which is embedded into the amorphous domain of PCL. In contrast, the shorter chains in PEU5A and the longer chains in PEU15A induce bigger crystallites of lamella crystalline domains favoring a relative high Tm respect to PEU10A. This explanation is according to the values of enthalpy and crystallinity of all PEU series, where the crystallinity of PEU is proportional to the DP of HOPCLOH as precursor.
On half of the PEUs synthetized with MDI and HMDI, a fusion was not observed, indicating that those samples were amorphous. So, a diisocyanate with a bulky substituent (MDI and HMDI) produced a urethane group that induced a disorder on the PCL chains, generating an amorphous domain. In the case of PEUs with DP = 15, except on PEUs with HDI, a third transition is observed: (3) crystallization temperature (Tc) (Tc = −31 to 3 °C); the existence of these three transitions is similar to the reported for the PLLA oligomers of moderate molecular weight (2100–3700 Da).45–47 Therefore, the DP in oligomers from HOPCLOH was a crucial factor in inducing transitions such as Tg, Tc, and Tm. Mostly amorphous PEUs were obtained at low DP of PCL in the case of non-linear diisocyanates.
For the mechanical properties of PEUs, for example, PEU derived from HOPCLOH with HO(CH2)4OH as an initiator, with HDI and DP = 5 showed a high modulus value (54.5 MPa), which was attributed to the high content of hard segments and low crystallinity (xi = 7%) of PCL. Additionally, the crystallinity of the three PEUs (from DP 5 to 15) contributed to the strain at break in a proportional manner (Fig. 11). Complementarily, for PEUs, prepared using HOPCLOHs with HO(CH2)4OH as an initiator and with DP = 15 (using MDI or HMDI), the value of modulus increased, which is consistent with a significant value of xi (15–18%). In general, a plastic behavior was observed for the majority of the PEUs samples.
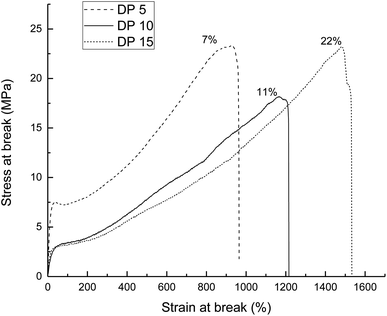 |
| Fig. 11 Stress–strain curves for PEUs derived from poly(ε-caprolactone) diol (HOPCLOH) with HO(CH2)4OH as an initiator using HDI and with different DP. The numbers next to each graph indicate the % PCL crystallinity. | |
Effect of the type of diisocyanate used for synthesis of PEUs. The main effect of the increase in the content of diisocyanate was the decrease in the enthalpy of fusion and crystallinity of PCL microdomains in PEUs with respect to HOPCLOH because the urethane groups caused a disruption in the order of the main chains of PCL and favored the hard segment interactions (Table S5†). As the percentage of hard segments increased, the Tg increased (Fig. 12). The rise in the hard segment contributed to greater stiffness in the polyurethane due to physical crosslinking (hydrogen bonding) between the urethane and carbonyl groups. Comparing the types of diisocyanate, it was evident that the PEU derived from MDI (PEU4a5B, Tg = −17 °C) exhibited the highest and the HDI (PEU4a5A, Tg = −49 °C) the lowest values of Tg (Table 4); this phenomenon was explained in terms of the aromatic (MDI) vs. aliphatic (HDI) hard segment, where the flexibility of aliphatic (methylene groups) substituents induced the lowest values of Tg. On the contrary, the rigidity of aromatic (aryl group) substituents produced high values of Tg.
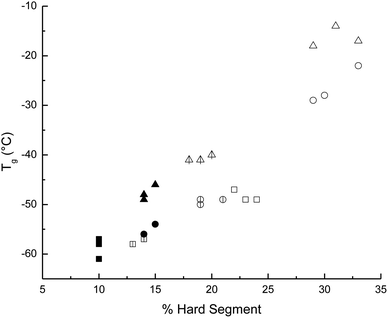 |
| Fig. 12 Effect of hard segment content on the glass transition temperature (Tg) in poly(ester-urethanes) (PEUs) derived from poly(ε-caprolactone) diols (HOPCLOH) and three different diisocyanates (1,6-hexamethylene diisocyanate (HDI) indicated with squares, methylene diphenyl diisocyanate (MDI) indicated with triangles, and 4,4′-methylenebis (cyclohexyl isocyanate) (HMDI) indicated with circles). The PEUs derived from the macrodiols with DP = 5 are indicated with open figures □, △, and ○ PEUs derived from macrodiols with DP = 10 are indicated with lined figures , , and ; and PEUs derived from macrodiols with DP = 15 are indicated with filled figures ■, ▲, and ●. | |
The Young modulus, tensile strength, and elongation at break derived from stress–strain curves are summarized in Table S5.† The modulus was affected by the type of diisocyanate; when using linear diisocyanate (HDI) with DP = 5, the modulus was higher than when using bulkier diisocyanates such as MDI and HMDI, the latter having slightly lower modulus values than PEUs with MDI, as shown in Fig. 13. So, the volume of the substituents (aromatic and cyclic) in the hard segment (urethane group) induced a disorder on the crystallinity domain of PCL, generating an amorphous domain and decreasing the modulus, which was consistent with comparisons made between PEUs with HDI and PEUs with MDI.24 Complementarily, the PEUs derived from HDI (DP = 5, Table S5,† PEU4a5A, PEU8a5A, and PEU12a5A) formed urethane groups where the intermolecular hydrogen bonding was favorable because the six methylenes (in HDI) were not bulky groups; this was the reason for the increase in the modulus. In general, the results of stress at break do not have a pattern. However, the profile of PEUs showed mainly plastic behavior.
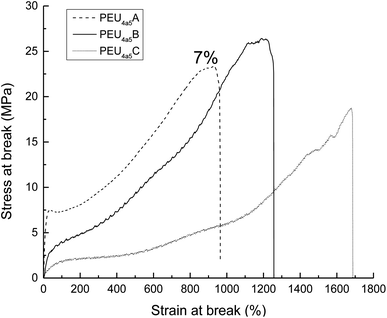 |
| Fig. 13 Stress–strain curves for PEUs derived from poly(ε-caprolactone) diol (HOPCLOH) with HO(CH2)4OH as initiator and DP of 5. The number next to PEU4a5A graph indicate the % PCL crystallinity, the other two PEUs does not present crystallinity. Where the end letter of the name of the sample corresponds to; (A) 1,6-hexamethylene diisocyanate (HDI); (B) methylene diphenyl diisocyanate (MDI); (C) 4,4′-methylenebis (cyclohexyl isocyanate) (HMDI). | |
This work contributes to the synthesis and characterization of poly(ester-urethanes) (PEUs) derived from PCL. Other studies, such as types of solvents in the synthesis of PEUs and degradation behavior, are currently underway in our laboratory.
Conclusions
Nine different macrodiols derived from α,ω-hydroxy telechelic poly(ε-caprolactone) (HOPCLOH) were synthesized by ROP of ε-caprolactone using a series of linear aliphatic diols [HO(CH2)mOH, where m = 4, 8, and 12] as initiators and three different degrees of polymerization (DP) of 5, 10, and 15, to explore the effect of initiator and DP, resulting in a controlled polymerization. In HOPCLOH, the values of crystallinity (xi) increased proportionally to the degree of polymerization (DP). Also, the melting temperature (Tm) clearly exhibited a proportional dependency on the DP. Complementarily, a longer alkyl group such as twelve methylenes (CH2)12 inserted in the main chain of HOPCLOH favored a relatively high xi, and crystallization temperature (Tc) was attributed to the nucleation effect. Twenty-seven poly(ester-urethanes) (PEUs) derived from HOPCLOH and three different diisocyanates [1,6-hexamethylene diisocyanate (HDI), 4,4′-methylene dicyclohexyl diisocyanate (HMDI), and methylene diphenyl diisocyanate (MDI)] were prepared. The thermal properties of PEUs showed a dependency on the type of diisocyanate, where linear HDI induced high values of xi with respect to MDI and HMDI. This effect was attributed to the disruption of the PCL crystalline domains due to the bulky substituents (MDI and HMDI). The glass transition temperature (Tg) of PEUs depended mainly on the percent of the hard segment (HS%) (or content of diisocyanates), where a high value of HS% produced a high Tg for all series. Complementarily, the DP in HOPCLOH oligomers was a significant factor in inducing the Tm of PEUs. Mostly amorphous PEUs were obtained at low DP (DP = 5 and 10) in the case of diisocyanates such as MDI and HMDI, which were not linear. In terms of the effects that affect the crystallinity for (1) HOPCLOH and (2) PEUs, these were: (1) DP > initiator, and (2) diisocyanate > DP > initiator, respectively. The mechanical properties indicated that factors such as HS% and xi had a significant contribution to the modulus of PEU. Most of the PEU samples exhibited plastic behavior.
Data availability
The data supporting this article have been included as part of the ESI.†
Author contributions
Miriam Paola Barrera-Nava: investigation, validation, formal analysis, writing – original draft. Rodrigo Navarro: investigation, supervision. Ángel Marcos-Fernández: investigation, supervision. José E. Báez: conceptualization, supervision, writing – original draft, writing – review, funding acquisition.
Conflicts of interest
There are no conflicts to declare.
Acknowledgements
José E. Báez would like to thank the Consejo Nacional de Ciencia y Tecnología (CONACYT) Ciencia Básica for the grant “Proyecto SEP/284893”, “CF-2023-I-2233”, and “CONACYT-SENER 177007”, the Sistema Nacional de Investigadores (SNI), the Dirección de Apoyo a la Investigación y al Posgrado (DAIP), and the University of Guanajuato (UG) Mexico, for financial support. Miriam Paola Barrera-Nava would thank the University of Guanajuato (UG) Mexico, for financial support. This research was also funded by the Spanish Ministry of Science and Innovation (MINECO) (Projects PID2020-119047RB-I00 and PLEC2021-00779). Authors Rodrigo Navarro and Ángel Marcos-Fernández are members of the SusPlast platform from the Spanish National Research Council (CSIC).
Notes and references
- A. Scribanti, M. Bortoluzzi and M. Gatto, Chem. Pap., 2016, 70, 53–60 Search PubMed.
- L.-H. Yao, S.-X. Shao, L. Jiang, N. Tang and J.-C. Wu, Chem. Pap., 2014, 68, 1381–1389 CAS.
- L. McKeen L, The Effect of Long Term Thermal Exposure on Plastics and Elastomers, Elsevier, 2nd edn, 2021, pp. 313–332 Search PubMed.
- A. Rai, S. Senapati, S. K. Saraf and P. Maiti, J. Mater. Chem. B, 2013, 4, 5151–5160 RSC.
- R. Dwivedi, S. Kumar, R. Pandey, A. Mahajan, D. Nandana, D. S. Katti, D. Mehrotra and J. Oral, J. Oral Biol. Craniofac. Res., 2020, 10, 381–388 CrossRef.
- R. Boia, P. A. N. Dias, J. M. Martins, C. Galindo-Romero, I. D. Aires, M. Vidal-Sanz, M. Agudo-Barriuso, H. C. de Sousa, A. F. Ambrósio, M. E. Braga and A. R. Santiago, J. Controlled Release, 2019, 316, 331–348 CrossRef CAS.
- M. Zikode, S. O. Ojwach and M. P. Akerman, J. Mol. Catal. A: Chem., 2016, 413, 24–31 CrossRef CAS.
- J. E. Báez, M. Martínez-Rosales and A. Martínez-Richa, Polymer, 2003, 44, 6767–6772 CrossRef.
- J. E. Báez, A. Marcos-Fernández and P. Galindo-Iranzo, Polym.-Plast. Technol. Eng., 2011, 50, 839–850 CrossRef.
- J. E. Báez, M. Martínez-Rosales, A. Martínez-Richa and P. Galindo-Iranzo, Polym.-Plast. Technol. Eng., 2017, 56, 889–898 CrossRef.
- J. Deng, M. Saleem, Q. Jia, Y. Ding, Y. Liu and Y. Chen, React. Funct. Polym., 2020, 148, 104506 CrossRef.
- J. E. Báez, A. Marcos-Fernández, R. Lebón-Aguilar and A. Martínez-Richa, Polymer, 2006, 47, 8420–8429 CrossRef.
- S. M. Guillaume, Eur. Polym. J., 2013, 49, 768–779 CrossRef CAS.
- L. B. Bronzeri, C. Gauche, L. Gudimard, E.-J. Courtial, C. Marquette and M. I. Felisberti, Eur. Polym. J., 2021, 151, 110449 CrossRef CAS.
- H. Chen, S.-Y. Lee and Y.-M. Lin, Polymers, 2020, 12, 1500 CrossRef CAS PubMed.
- J. E. Báez, D. Ramírez, J. L. Valentín and A. Marcos-Fernández, Macromolecules, 2012, 45, 6966–6980 CrossRef.
- P. Ping, W. Wang, X. Chen and X. Jin, Biomacromolecules, 2005, 6, 587–592 CrossRef PubMed.
- J. E. Báez and A. Marcos-Fernández, React. Funct. Polym., 2012, 72, 349–357 CrossRef.
- X. Lin, D. Tang, S. Gu, H. Du and E. Jiang, New J. Chem., 2013, 37(8), 2433–2439 RSC.
- W. Yang, D. Guan, J. Liu, Y. Luo and Y. Wang, New J. Chem., 2020, 44(8), 3493–3503 RSC.
- S. Naderi and A. Esmaeili A, New J. Chem., 2020, 44(28), 12090–12098 RSC.
- H.-Y. Mi, X. Jing, B. N. Napiwocki, B. S. Hagerty, G. Chen and L.-S. Turng, J. Mater. Chem. B, 2017, 5(22), 4137–4151 RSC.
- X. Lui, B. Yang, Z. Hou, N. Zhang and Y. Gao, Mater. Sci. Eng., C, 2019, 104, 109952 CrossRef PubMed.
- B. Fernández-d’Arlas, A. Alonso-Varona, T. Palomares, M. A. Corcuera and A. Eceiza, Polym. Degrad. Stab., 2015, 122, 153–160 CrossRef.
- L. H. Chan-Chan, R. Solis-Correa, R. F. Vargas-Coronado, J. M. Cervantes-Uc, J. V. Cauich-Rodríguez, P. Quintana and P. Bartolo-Pérez, Acta Biomater., 2010, 6, 2035–2044 CrossRef CAS.
- B. Bogdano, V. Toncheva, E. Schacht, L. Finelli, B. Sarti and M. Scandola, Polymer, 1999, 40, 3171–3182 CrossRef.
- M. A. Javai, M. Younas, I. Zafar, R. A. Khera, K. M. Zia and S. Jabee, Int. J. Biol. Macromol., 2019, 124, 321–330 CrossRef.
- Y. Li, W. Kang, J. O. Stoffer and B. Chu, Macromolecules, 1994, 27(2), 612–614 CrossRef CAS.
- L. Hojabri, J. Jose, A. L. Leao, L. Bouzidi and S. S. Narine, Polymer, 2012, 53, 3762–3771 CrossRef CAS.
- J. V. Džunuzović, I. S. Stefanović, E. S. Džunuzović, A. Dapčević, S. I. Šešlija, B. D. Balanč and G. C. Lama, Prog. Org. Coat., 2019, 137, 105305 CrossRef.
- I. Yilgor, E. Yilgor, I. G. Guler, T. C. Ward and G. M. Wilkes, Polymer, 2006, 47, 4105–4114 CrossRef CAS.
- L. Zhang, C. Zhang, W. Zhang, H. Zhang and Z. Hou, J. Biomater. Sci., Polym. Ed., 2019, 30(13), 1212–1226 CrossRef CAS.
- C. Check, B. Imre, H. Gojzewski, R. Chartoff and G. J. Vancso, Polym. Chem., 2018, 9(15), 1983–1995 RSC.
- A. Haryńska, J. Kucinska-Lipka, A. Sulowska, I. Gubanska, M. Kostrzewa and H. Janik, Materials, 2019, 12, 887 CrossRef.
- K. Walinska, A. Iwan, K. Gorna and S. Gogolewski, J. Mater. Sci.: Mater. Med., 2008, 19, 129–135 CrossRef CAS PubMed.
- L. Meng, X. Shi, R. Zhang, L. Yan, Z. Liang, Y. Nie and T. Hao, J. Appl. Polym. Sci., 2020, 49314 CrossRef CAS.
- L. Zhou, L. Yu, M. Ding, J. Li, H. Tan, Z. Wang and Q. Fu, Macromolecules, 2011, 44(4), 857–864 CrossRef CAS.
- S. Fu, G. Yang, J. Wang, X. Wang, X. Cheng and R. Tang, Polymer, 2017, 114, 1–14 CrossRef CAS.
- S.-Y. Lee, S.-C. Wu, H. Chen, L.-L. Tsai, J.-J. Tzeng, C.-H. Lin and Y.-M. Lin, BioMed Res. Int., 2018, 3, 1–13 Search PubMed.
- E. Rodríguez-deLeón, M. Bah, J. O. C. Jiménez-Halla, J. Bonilla-Cruz, M. Estévez and J. E. Báez, Polym. Chem., 2019, 10, 6580–6587 RSC.
- Q. Luo, J. Chen, P. Gnanasekar, X. Ma, D. Qin, H. Na and N. Yan, New J. Chem., 2019, 44(3), 658–662 RSC.
- R. Wu, J. Lai, Y. Pan, Z. Zheng and X. Ding, Soft Matter, 2018, 14(22), 4558–4568 RSC.
- A. Biswas, A. P. Singh, D. Rana, V. K. Aswal and P. Maiti, Nanoscale, 2018, 10(21), 9917–9934 RSC.
- J. E. Báez, A. Martínez-Richa and A. Marcos-Fernández, Macromolecules, 2005, 38, 1599–1608 CrossRef.
- M. Bero, B. Czapla, P. Dobrzyński, H. Janeczek and J. Kasperczyk, Macromol. Chem. Phys., 1999, 200(4), 911–916 CrossRef CAS.
- J. E. Báez, P. Galindo-Iranzo and A. Marcos-Fernández, Int. J. Polym. Anal. Charact., 2016, 21(2), 149–155 CrossRef.
- J. E. Báez, A. Marcos-Fernández and P. Galindo-Iranzo, J. Polym. Res., 2011, 18, 1137–1146 CrossRef.
|
This journal is © The Royal Society of Chemistry 2024 |