DOI:
10.1039/D4RA03833A
(Paper)
RSC Adv., 2024,
14, 18716-18729
Realization of a green-emitting pyrosilicate-structured Er3+-activated Y2Si2O7 phosphor: a systematic study of opto-electronic characteristics and thermal stability for lighting applications†
Received
24th May 2024
, Accepted 26th May 2024
First published on 11th June 2024
Abstract
A series of green-emitting Y2−xSi2O7:xEr3+ phosphors (x = 1–7 mol%) have been successfully synthesized using a straightforward gel-combustion method facilitated by urea. X-ray diffraction analysis provided specific patterns for samples, confirming a consistent triclinic phase across erbium-doped structures compared to undoped structures. Studies using TEM and EDX were conducted to identify the surface-related characteristics and chemical composition of the synthesized nanophosphor, respectively. The band gap was determined to be 5.55 eV and 5.80 eV for the host material and optimal sample, respectively. The primary peak of excitation, observed at 379 nm, represents the highly sensitive electric dipole transition from the 4I15/2 state to the 4G11/2 level, suggesting that the prepared phosphors could effectively absorb NUV light for activation. The PL profiles of Y2−xSi2O7:xEr3+ (x = 1–7 mol%) phosphors demonstrate characteristic emissions at 409 nm (2H9/2 → 4I15/2), 522 nm (2H11/2 → 4I15/2), 553 nm (4S3/2 → 4I15/2) and 662 nm (4F9/2 → 4I15/2). In accordance with Dexter's theory, luminescence quenching observed at a concentration of 4 mol% Er3+ is attributed to dipole-quadrupole interactions. The optimal sample demonstrates excellent thermal stability, indicated by its luminescence at different temperatures and activation energy of 0.2641 eV. Additionally, the CIE, color purity and CCT values of the fabricated nanomaterials make it ideal for use in lighting applications.
1 Introduction
Today, the rapid growth of the population and the swift pace of economic development have spurred a notable surge in energy demand, contributing significantly to the recent uptick in global carbon emissions.1,2 Consequently, there has been a growing global interest in renewable energy sources and environmental preservation. Approximately 20% of the world's electricity consumption is attributed to lighting.3 Presently, white light-emitting diodes (WLEDs) have garnered considerable attention as next-generation solid-state lighting to substitute conventional lamps4,5 owing to their notable advantages, including high energy efficiency, extended operational lifespan, compactness and eco-friendliness.6,7 The current mainstream commercial white LEDs utilize a combination of blue InGaN-LED-chips and YAG:Ce3+ yellow phosphors to produce white light.8 This pairing generates white light through a blend of two-color emission bands. However, the resulting white light suffers from drawbacks such as a poor color-rendering-index (CRI <80) and high correlated color temperature (CCT >4500 K) because it lacks a red-emitting component.9–11 This limitation restricts its suitability for indoor lighting applications. To address this issue and achieve white light with improved CRI and lower CCT values, an alternative approach has emerged. This method involves employing near-ultraviolet LED-chips to stimulate tricolor (RGB) phosphors.12,13 At present, the wide color gamut WLED backlight is mainly composed of a blue InGaN chip, K2SiF6:Mn4+ red phosphor and green b-SiALON:Eu2+ phosphor.14 However, the long decay time (∼8 ms) of the K2SiF6:Mn4+ (KSF:Mn4+) red phosphor easily affects the image-retention performance of fast-response backlight displays.15 Apparently, the phosphor for LEDs should not only possess a broad excitation band appropriate to LED chip emission and narrow band emission with high color purity, but also have an appropriate decay time. For solid-state lighting, the commercial WLED is a phosphor-converted light-emitting diode (LED) fabricated by a combination of an InGaN chip and YAG:Ce3+ phosphor. However, due to the lack of a red component, this type of WLED exhibits a cold white light emission with low color-rendering index (CRI <80) and high correlated color temperature (CCT >4500 K). It is thus clear that red phosphor is an important part of assembling high-CRI light sources. Presently, non-rare-earth Mn4+ red phosphors have been used in the packaging of WLEDs due to their high luminous efficacy and low cost.16 These phosphors possess unique properties such as exceptional thermal and chemical stability, cost-effectiveness, straightforward synthesis techniques and outstanding optical characteristics, resulting in significantly enhanced luminescence emission.17–19 Within the lanthanide series, erbium (Er3+) stands out for its ability to generate intense green emissions through down-conversion photoluminescence. This phenomenon arises from transitions such as 2H9/2 → 4I15/2, 2H11/2 → 4I15/2, 4S3/2 → 4I15/2, and 4F9/2 → 4I15/2.20,21 In addition to the activators, the choice of the host material holds significant importance as it can impact the photoluminescence (PL) properties of the dopant ions. Factors such as the crystal field strength, covalent nature, and potential energy transfer between the host and dopant ions all play roles in influencing these properties.22,23 The host lattice Y2Si2O7 is known for its exceptional thermal and chemical stability. It exhibits complex high-temperature polymorphism, existing in various structural phases denoted as α, β, γ, δ, γ, and η forms.24 Among these, α-Y2Si2O7 is characterized as a low-temperature phase and crystallizes into a triclinic structure with a P
space group.25
However, a significant challenge lies in preparing this system as a single-phase material. Achieving a homogeneous, single-phase material is essential for many applications, and overcoming this challenge often requires precise control over the synthesis conditions and processing techniques. It is essential to establish direct synthetic routes for fabricating Er3+-doped Y2Si2O7 luminescent nanoparticles, allowing precise manipulation of their crystalline phase, morphology and dimensions. This control enables customization of their optical characteristics, opening avenues for applications in multiple domains. As a result, several chemical techniques, such as sol–gel, chemical vapor deposition and microwave-assisted methods, have been developed to address this requirement.26–28 Among these techniques, this study focuses on the gel-combustion method due to its distinct advantages. These methods offer several advantages, including simplicity in synthesis, capability to produce nano-sized crystalline powders with high purity at low temperatures, and improved homogeneity.29,30 These characteristics make the gel-combustion method particularly well-suited for the production of Er3+-doped Y2Si2O7 nanoparticles with tailored properties for a range of applications. In this study, Y2Si2O7:Er3+ phosphors containing different amounts of Er3+ were produced using an auto gel-combustion approach. To the author's knowledge, there are no existing reports on the synthesis of Y2Si2O7 doped with Er3+ ions using this combustion method. The research thoroughly examined various properties, including diffraction, morphology, chemical composition, band gap, photoluminescence, thermal luminescence quenching and photometric characteristics.
2 Experimental
2.1 Synthesis and instrumentations
The gel combustion approach was utilized to produce Er3+-activated Y2Si2O7 nanophosphors. The initial ingredients are greatly purified A.R. grade compounds with a purity of 99.99% of the metal nitrates groups, i.e., yttrium nitrate, erbium nitrate, silica, nitric acid and urea were procured and used without any further purification. Metal nitrate precursors were utilized due to their low disintegration temperature. Combustion was facilitated by low-temperature deterioration and the solubility of metallic precursors in water. Oxygen was generated as nitrates disintegrated, while urea acted as a fuel. Various compositions of Er3+ (1–7 mol%) were incorporated into the YPS lattice. A stoichiometric mixture of metal nitrites was heated to form a gel solution, which was then heated further with urea and water. Combustion occurred at 600 °C, producing gases (N2, CO2 etc) and porous flaky nanophosphors. The process released significant energy, resulting in a crystalline structure and reduced particle size.31 The synthesized materials were then calcined at 1100 °C for three hours to achieve the desired phase. Fig. 1 depicts the illustrated explanation of the combustion synthesis method. Powder X-ray diffraction analysis was conducted using a Rigaku Ultima X-ray diffractometer, operating at 40 kV and 40 mA with Cu-Kα radiation, to confirm the crystal structure and phase purity of the prepared phosphors. Diffuse reflection spectra of the samples were obtained using an integrated sphere-mounted UV-vis-NIR spectrophotometer (UV-3600, SHIMADZU). The morphology of the doped nanopowders was examined using a transmission electron microscope (JEOL JEM-1400 Plus). Energy dispersive X-ray (EDX) analysis was performed using a Hitachi SU-8010 SERIES. Moreover, the photoluminescence (PL) and photoluminescence excitation (PLE) spectra of the samples were examined using a spectrophotometer (Fluorolog-3-Horiba) outfitted with a 150 W xenon lamp.
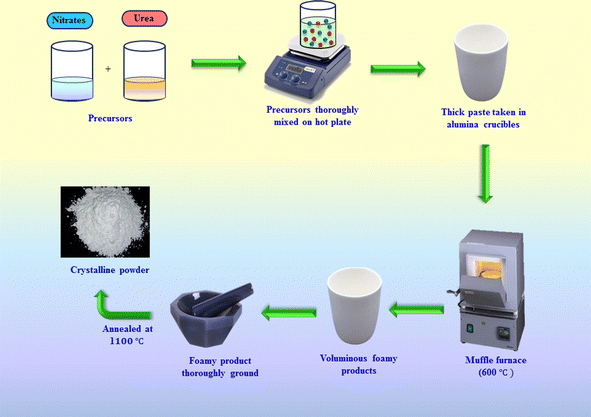 |
| Fig. 1 Synthetic diagram of the gel-combustion process. | |
3 Results and discussion
3.1 XRD evaluation
X-ray diffraction (XRD) patterns for Y2Si2O7 (YPS) and its variants doped with different concentrations (1–7 mol%) of Er3+ ions, spanning a scan range of 10° to 80°, are depicted in Fig. 2(a). These patterns correspond closely with the established disilicate structure of Y2Si2O7, as described by JCPDS card no. 38-0223, which is characterized by a triclinic phase with space group P
.32 No additional peaks appeared in the patterns after introducing Er3+ ions, indicating the maintenance of a single phase in the material. Fig. 2(b) shows that the XRD peaks shift for the samples containing Er3+; as the concentration of Er3+ in the undoped Y2Si2O7 increases, the peaks shift towards lower angles. This shift is attributed to the smaller ionic radius of Er3+ compared to Y3+ within the same coordination environment. When smaller Er3+ ions replace larger Y3+ ions in the structure, the inter-planar distances diminish. This reduction leads to an increase in the diffraction angles, which aligns with Bragg's equation, “2d
sin
θ = nλ”.33 In this equation, “n” represents an integer, d symbolizes the inter-planar distance, θ is the diffraction angle and λ is the wavelength of the X-rays. This adjustment in the inter-planar spacing and the corresponding shift in diffraction angles can be directly linked to the substitution of the ion sizes within the crystal lattice. This observation confirms that the Er3+ ions have been successfully incorporated into the Y3+ ion sites within the YPS matrix. The shift in the diffraction angles and the reduction of the inter-planar distances provide clear evidence of the substitution, indicating that the doping process was effective (Table 1). Eqn (1) assesses the percentage difference in the radius (Dr) between the doped ion and substituted cation within the host, serving to verify this substitution. This equation quantifies the alteration in ionic radii, offering a precise method to confirm the successful inclusion of Er3+ ions into Y3+ sites within YPS host.34 |
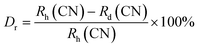 | (1) |
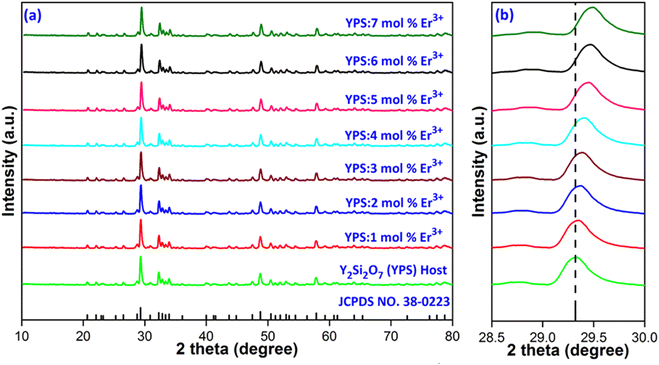 |
| Fig. 2 (a) Diffraction patterns of Y2Si2O7 and Y2−xSi2O7:xEr3+ (x = 1–7 mol%) phosphors. (b) Enlarged pattern view of all considered samples. | |
Table 1 Determined interplanar d-spacing values of the host and Er3+-doped phosphors
Sample |
2θ-Angle |
d-Spacing (Å) |
Y2Si2O7 (YPS) |
29.32 |
3.0437 |
YPS:1 mol% Er3+ |
29.33 |
3.0427 |
YPS:2 mol% Er3+ |
29.35 |
3.0406 |
YPS:3 mol% Er3+ |
29.36 |
3.0396 |
YPS:4 mol% Er3+ |
29.39 |
3.0366 |
YPS:5 mol% Er3+ |
29.43 |
3.0325 |
YPS:6 mol% Er3+ |
29.45 |
3.0305 |
YPS:7 mol% Er3+ |
29.47 |
3.0285 |
In the mentioned equation, Rh(CN) and Rd(CN) represent the ionic-radii and coordination numbers of the host and entering (doped) cation, respectively. The equation helps to determine whether the size difference between the host ion and the doped ion is within an acceptable limit, which typically should not exceed 30%. The calculated value, being within this range, supports the successful incorporation of the doped ion into the host matrix. In this case, it further confirms the effective doping of Er3+ ions into the host matrix, indicating that the radii of Er3+ and substituted ion are sufficiently close to allow for effective substitution without disrupting the crystal lattice. Scherrer's equation was employed to determine the crystallite size (D) of samples containing varying concentrations of activated ions. This eqn (2) is pivotal in the analysis of X-ray diffraction data to estimate the size of crystallites, taking into account the broadening of diffraction peaks due to particle size effects.35
|
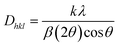 | (2) |
In this equation, λ represents the X-ray wavelength, (0.154 nm for Cu Kα radiation) and β is the full width at half maximum of the diffraction peak. The shape factor (k), referred to as the Scherrer constant with a value of 0.89, is dimensionless and accounts for the shape of the crystallite assumed in the calculation. Table 2 provides a comprehensive overview of the crystallite size, which varies across different samples with varying concentrations of activated ions. When examining X-ray line-widening in samples, both crystallite size and strain contribute to the effect. The Williamson–Hall (W–H) plot offers a method to collectively analyze these contributions. In accordance with the W–H relation, the total width at half maximum (β) is defined as a linear amalgamation of contributions originating from the size of the crystallites (D) and level of strain (ε). The pertinent equation is presented below.36
|
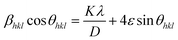 | (3) |
Table 2 Computed diffraction values of Y2Si2O7 and Y2-xSi2O7:xEr3+ (x = 1–7 mol%) phosphors
Sample (YPS) |
2 theta (2θ) |
FWHM |
Crystallite size (nm) Scherrer's W–H |
Microstrain (ε × 10−4) |
Y2Si2O7 (YPS) |
29.32 |
0.2997 |
27.40 |
35.58 |
5.1421 |
YPS:1 mol% Er3+ |
29.33 |
0.3037 |
27.30 |
34.19 |
5.3012 |
YPS:2 mol% Er3+ |
29.35 |
0.3152 |
26.07 |
33.43 |
5.6017 |
YPS:3 mol% Er3+ |
29.36 |
0.3310 |
24.83 |
32.59 |
5.9271 |
YPS:4 mol% Er3+ |
29.39 |
0.3374 |
24.35 |
31.19 |
6.1724 |
YPS:5 mol% Er3+ |
29.43 |
0.3419 |
24.31 |
29.34 |
6.4587 |
YPS:6 mol% Er3+ |
29.45 |
0.3774 |
24.05 |
27.71 |
6.7410 |
YPS:7 mol% Er3+ |
29.47 |
0.3807 |
20.86 |
26.48 |
7.0241 |
This expression allows for the determination of both crystallite size and strain from the X-ray diffraction data. In the given straight-line formula, a linear relationship between 4
sin
θ (on the x-axis) and β
cos
θ (on the y-axis) is depicted. The intercept is used to derive the values of the crystallite size, while the slope of the line is employed to compute the value of the strain. Fig. 3 presents the Williamson–Hall visualizations of the host and all doped YPS:xEr3+ (x = 1–7 mol%) nanomaterials. This figure likely offers a graphical representation of how the crystallite size and strain vary across different samples, providing valuable insights into the structural characteristics of the doped materials compared to the host material. The Rietveld refinement method assesses the intensity profile, providing a reliable model for the crystal properties. Fig. 4(a & b) shows the Rietveld profiles for YPS and YPS doped with 4 mol% Er3+ nanophosphor. All refined data align with the initial model, with reliability factors within a sufficient range. Table 3 outlines the refinement details, indicating that all samples belong to the triclinic system with space group P
. Crystallographic parameters decrease from the host material to the optimized sample, indicating effective Er3+ inclusion.
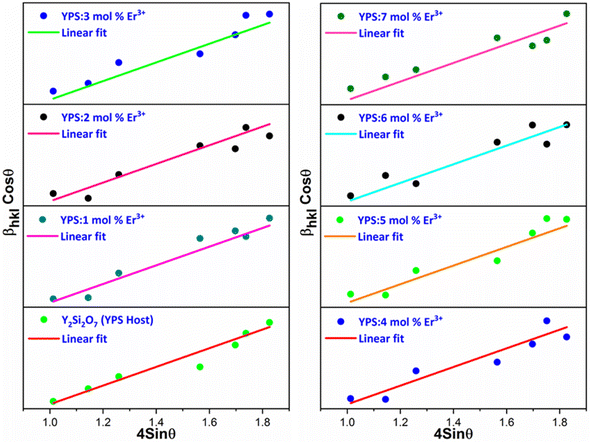 |
| Fig. 3 W–H plot of Y2Si2O7 and various doped Y2−xSi2O7:xEr3+ (x = 1–7 mol%) phosphors. | |
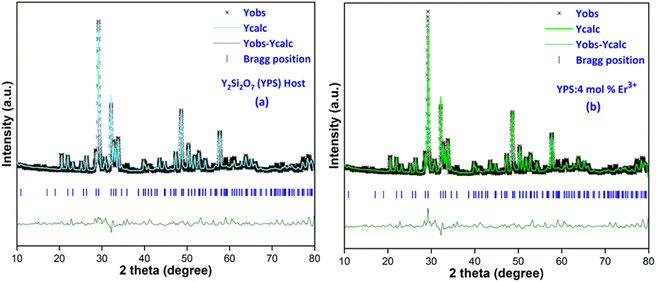 |
| Fig. 4 Rietveld profiles of the (a) Y2Si2O7 and (b) Y1.96Si2O7:4 mol% Er3+ phosphor. | |
Table 3 Refinement outcomes of Y2Si2O7 (YPS) and YPS:4 mol% Er3+ phosphors
Sample |
Y2Si2O7 (YPS) |
YPS:4 mol% Er3+ |
2θ range; step (deg.) |
10–80; 0.02 |
10–80; 0.02 |
System |
Triclinic |
Triclinic |
Lattice-type |
P |
P |
Wavelength (Å) |
1.541 |
1.541 |
Space group |
P![[1 with combining macron]](https://www.rsc.org/images/entities/char_0031_0304.gif) |
P![[1 with combining macron]](https://www.rsc.org/images/entities/char_0031_0304.gif) |
Space group number |
2 |
2 |
Formula unit (N) |
4 |
4 |
α |
97.23 |
97.20 |
β |
89.80 |
89.76 |
γ |
87.50 |
87.43 |
a (Å) |
6.556 |
6.507 |
b (Å) |
6.759 |
6.578 |
c (Å) |
12.274 |
11.7721 |
Volume (Å3) |
539.03 |
499.381 |
χ2 |
3.14 |
3.72 |
Rp (%) |
5.77 |
6.11 |
Rwp (%) |
7.59 |
8.41 |
3.2 TEM and EDX analyses
The TEM image is used to analyze the sample structure, framework and crystal size. Fig. 5 shows the TEM picture of the optimal sample, revealing the uniformly spread and roughly spherical particles ranging from 25–45 nm. Minor discrepancies in crystallite size were due to uneven heat and mass flow during combustion.37 In Fig. 6(a and b), EDX plots of the Y2Si2O7 (YPS) host and 4 mol% Er3+-doped YPS nanophosphor are shown. Fig. 6(a) displays the peaks for Y, Si and O, while Fig. 6(b) shows the peaks for Y, Si, O and Er, confirming their chemical compositions. The presence of Er peaks confirms uniform doping. Table of insets listed the elemental compositions of both samples. No additional emissions indicate the formation of pure Y2Si2O7 and Y2Si2O7:Er3+ phosphors, supporting the PL and XRD results.
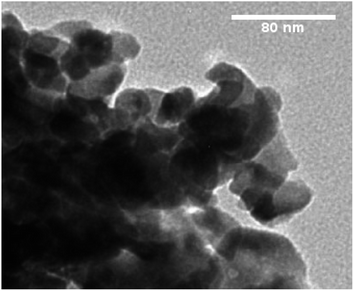 |
| Fig. 5 TEM image of the YPS:4 mol% Er3+ nanophosphor. | |
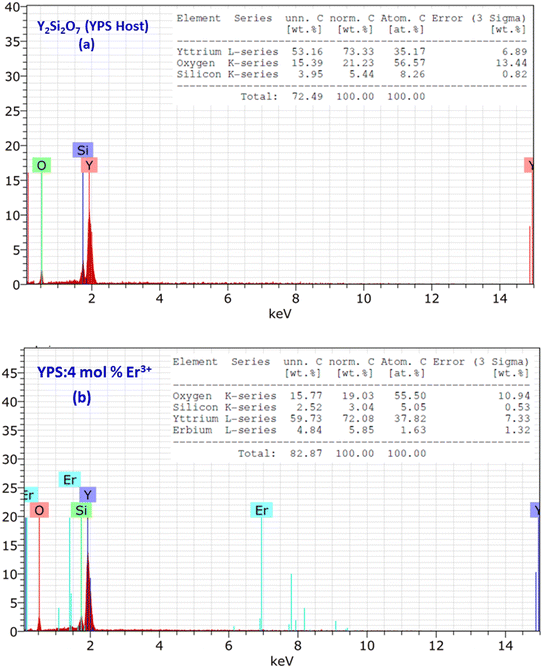 |
| Fig. 6 EDX profiles of (a) the Y2Si2O7 and (b) Y1.96Si2O7:4 mol% Er3+ phosphor. The inset represents the chemical composition of the respective samples. | |
3.3 Photoluminescence study
3.3.1 Excitation and emission spectra. To explore the light emission performance of the fabricated phosphors, their excitation (PLE) spectra were examined within the 200–550 nm wavelength range, at an emission of 550 nm for Er3+. Fig. 7 illustrates the PLE spectra of the YPS phosphors with 4 mol% doping concentration of Er3+, showcasing prominent peaks at ∼350 nm (4I15/2 → 4G7/2), ∼366 nm (4I15/2 → 4G9/2), ∼378 nm (4I15/2 → 4G11/2), ∼408 nm (4I15/2 → 2G9/2), ∼450 nm (4I15/2 → 4F5/2), ∼489 nm (4I15/2 → 4F7/2), and ∼523 nm (4I15/2 → 2H11/2).38,39 Additionally, a broad-band spanning 230–330 nm is observed, indicating the charge transfer band (CTB) from O2− to Er3+. The highest peak, occurring at 378 nm, resembles the 4I15/2 → 4G11/2 transition of erbium ions. This finding suggests that the phosphor under study could potentially be used in the production of near-ultraviolet light emitting diodes (NUV-LEDs). The photoluminescence (PL) emission spectra (Fig. 8) of YPS:xEr3+ (x = 1–7 mol%) phosphors display prominent peaks at ∼409 nm, ∼523 nm, ∼549 nm and ∼673 nm, indicating the 2H9/2 → 4I15/2, 2H11/2 → 4I15/2, 4S3/2 → 4I15/2 and 4F9/2 → 4I15/2 transitions, respectively.40,41 Among these, the strong green emission at 553 nm, resulting from the 4S3/2 → 4I15/2 transition, stands out because of the electric dipole (ED) nature (ΔJ = 3).
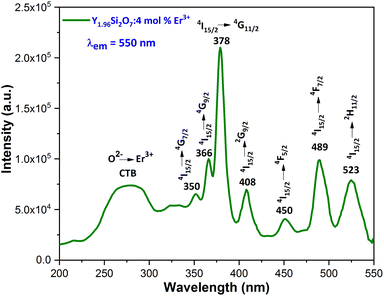 |
| Fig. 7 Excitation spectrum of the Y1.96Si2O7:4 mol% Er3+ nanophosphor. | |
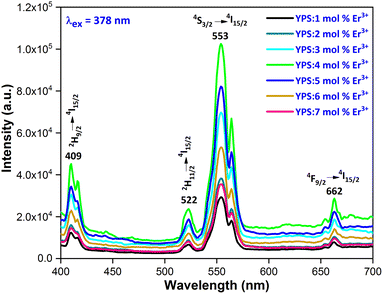 |
| Fig. 8 Emission spectrum of the Y2−xSi2O7:xEr3+ (x = 1–7 mol%) nanophosphors. | |
3.3.2 Concentration quenching (CQ). The emission intensities increased gradually until they peaked with a concentration of Er3+ ions ranging from 1 to 4 mol%. Subsequently, the luminous intensity decreased due to CQ, as illustrated in Fig. 9. The process of concentration quenching may involve non-radiative energy transfer among the Er3+ ions. Non-radiative energy transfer among erbium ions (Er3+), which is often attributed to exchange interaction, radiation re-absorption and multipolar interaction, can lead to concentration quenching. Initially, the PL intensity rises with the Er3+ doping concentration, but decreases beyond a critical concentration (xc). At this point, the critical distance (Rc) between ions is reduced enough to enable energy transfer. Blasse's eqn (4) is used to approximate the critical distance between activators.42 |
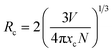 | (4) |
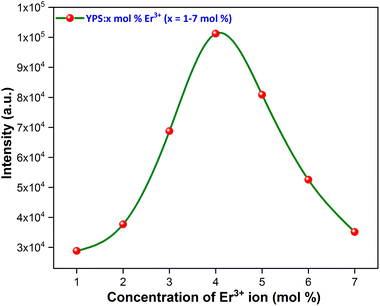 |
| Fig. 9 Concentration quenching profile of the considered nanophosphors. | |
In this context, “xc” represents the ideal concentration, “V” stands for volume and “N” denotes the cation count within the unit cell. In the current investigation, these variables are determined as xc = 0.04, V = 499.381 Å3 and N = 4. The critical distance for energy transfer is estimated at about 18.162 Å. Typically, when the value of “Rc” is around 5 Å, the exchange-interaction becomes the driving force behind energy transfer. The energy transfer among Er3+ ions in the YPS:Er3+ phosphor, with Rc exceeding 5 Å, likely occurs through multipolar interactions, such as dipole–dipole, dipole–quadrupole, and quadrupole–quadrupole mechanisms. Dexter's theory states that when the same activator ions interact multipolarly, eqn (5) can determine the specific interaction causing concentration quenching in the phosphor.
|
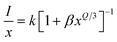 | (5) |
In this equation, Q represents the constant of the multipolar-interaction, taking values of 6, 8 and 10 for dipole–dipole (d–d), dipole–quadrupole (d–q) and quadrupole–quadrupole (q–q) interactions, respectively.43 Meanwhile, k and β denote constants specific to the host material under the same excitation conditions. Eqn (6) can also be simplified as:
|
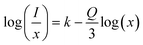 | (6) |
Eqn (6) was employed to generate a plot illustrating the correlation between log(x) and log(I/x), as depicted in Fig. 10. The data points on the graph were subjected to linear fitting, resulting in a slope of −2.90. Consequently, the calculated value of Q is approximately 8.7, which is assumed to be 8. This suggests that the dipole–quadrupole (d–q) interaction is probably accountable for the energy transfer among the dopant ions, thereby causing concentration quenching.
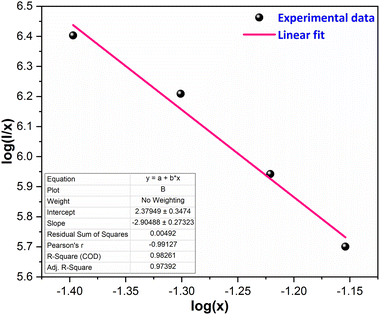 |
| Fig. 10 Straight line-fitted graph between log(x) and log(I/x). | |
3.4 Luminescence lifetime
Fig. 11 illustrates the decay behavior of the YPS nanophosphor containing 4 mol% of erbium ions. The lifetime curve, obtained under excitation at 349 nm and emission at 578 nm, was analyzed using a bi-exponential decay function.44 |
It = I0 + A1 exp(−t/τ1) + A2 exp(−t/τ2)
| (7) |
here, I and I0 denote the luminescence intensities at time t and 0, respectively. τ1 and τ2 correspond to lifetime values for the fast and slow exponential components, respectively. Additionally, A1 and A2 are constants associated with the parameters for curve fitting. The formula used to determine the average lifetime for the fabricated phosphor is: |
τavg = (A1t12 + A2t22)/(A1t1 + A2t2)
| (8) |
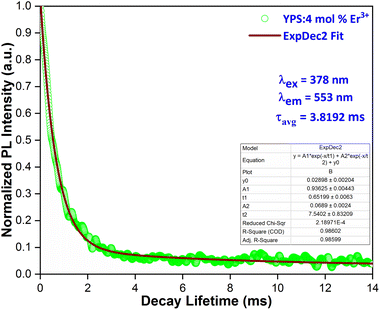 |
| Fig. 11 Lifetime curve of the Y1.96Si2O7:4 mol% Er3+ nanophosphor. | |
The average lifetime achieved from the fitted results for YPS doped with various concentrations of Er3+ is as follows: 4.3492 ms for 1 mol%, 4.2024 ms for 2 mol%, 4.1021 ms for 3 mol%, 3.8192 ms for 4 mol%, 3.5034 ms for 5 mol%, 3.2110 ms for 6 mol%, and 2.9420 ms for 7 mol%. Fig. 12 shows Auzel's model, described by eqn (9), which was used to analyze the gradual decrease in lifetime values with increasing dopant concentration.
|
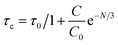 | (9) |
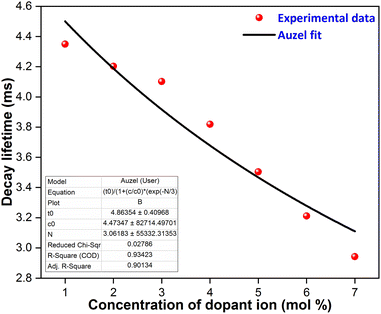 |
| Fig. 12 Auzel's fitting curve of Y2−xSi2O7:xEr3+ (x = 1–7 mol%) phosphors. | |
In this context, “N” represents the phonon number involved in quenching via the cascade multi-phonon process, “Co” is a constant, “τc” is the lifetime at concentration “C” and “τo” signifies the intrinsic lifetime. The intrinsic lifetime was determined to be 4.86 ms. The non-radiative relaxation rate (AnR) is also calculated using the radiative-lifetime (τo) and average lifetime (τavg) in the following eqn (10).45
|
 | (10) |
Quantum efficiency (η) is one of the significant parameters to describe the efficiency of the rare earth (RE)-doped material, and it was defined as the ratio between the light intensity emitted to the light intensity absorbed, and also described as a ratio of the experimental lifetime (τavg) and radiative lifetime (τo), as shown in the following eqn (11).
|
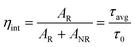 | (11) |
Quinine bisulphate was used as a reference to estimate the value of the extrinsic quantum efficiency via eqn (12).46
|
 | (12) |
here,
ηs is the quantum efficiency of the sample;
ηr represents the quantum efficiency of the reference,
A is the absorbance at the excitation wavelength, and
I is used for the integrated emission intensity. Also, s, r and n are used for the refractive index of the sample, reference and solvent, respectively.
Table 4 lists the lifetime, non-radiative transition rate, intrinsic quantum efficiency and extrinsic quantum efficiency values of the synthesized nanophosphors.
Table 4 Decay time and quantum efficiency of Y2-xSi2O7:xEr3+ (x = 1–7 mol%) phosphors
Sample |
τavg (ms) |
AnR (S−1) |
(ηint%) |
(ηext%) |
YPS:1 mol% Er3+ |
4.3492 |
24.22 |
89.48 |
19 |
YPS:2 mol% Er3+ |
4.2124 |
31.69 |
86.67 |
17 |
YPS:3 mol% Er3+ |
4.1021 |
38.08 |
84.40 |
14 |
YPS:4 mol% Er3+ |
3.8192 |
56.13 |
78.58 |
11 |
YPS:5 mol% Er3+ |
3.5034 |
79.73 |
72.08 |
10 |
YPS:6 mol% Er3+ |
3.2110 |
105.72 |
66.07 |
8 |
YPS:7 mol% Er3+ |
2.9420 |
134.20 |
60.53 |
6 |
3.5 Optical band gap analysis
The optical band-gap of a material is pivotal in optoelectronic applications. In the case of the prepared phosphors, this parameter has been determined through diffuse reflectance spectroscopy (DRS). This method deals with the decline in incident light after it is absorbed or reflected by the surface of the matrix. For powder samples, dispersion occurs extensively, making it challenging to measure the scattered intensity ideally. Therefore, the reflectance mode is optimal for accurately measuring this parameter. Fig. 13 depicts the DRS of Y2Si2O7 (YPS) and YPS:4 mol% Er3+ in the 200–800 nm range. Charge transfer between O2− and Er3+ is represented by the band at 245–275 nm in the doped sample. Additional peaks at 378 nm, 489 nm, and 523 nm are ascribed to the 4I15/2 → 4G11/2, 4I15/2 → 4F7/2, and 4I15/2 → 4H11/2 transitions of Er3+, respectively. The obtained DRS data were transformed into absorption using the Kubelka–Munk (KM) function, as described below.47 |
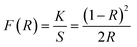 | (13) |
here, R defines the ratio of the focused phosphor's reflectance to that of the reference, and F(R) defines the KM function. The absorptions and scattering coefficients are denoted by K and S, respectively. Using Tauc's eqn (14), the band-gap was computed and is shown below.here, C, hν and Eg stand for the constant, energy of the incident light, and the optical band-gap, respectively. Here, n equals ½, indicative of the direct band-gap for the focused nanomaterials. When the material is subjected to a 60-degree angle of exposure, the interplay of the aforementioned equations, under this specific condition, leads to K = 2α and S functions as a constant, giving rise to an additional expression.48 |
[F(R)hν]2 = C(hν − Eg)
| (15) |
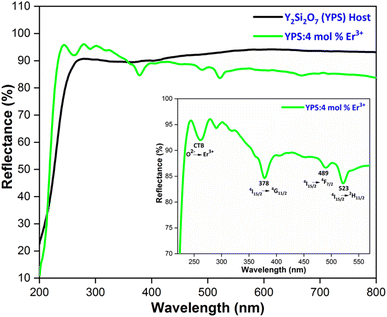 |
| Fig. 13 DRS spectra of the host and Y1.96Si2O7:4 mol% Er3+ nanophosphor. | |
The band-gap value was determined by projecting the curve onto [F(R)hν]2 = 0 in the graphical representation of [F(R)hν]2 versus hν. The calculated band-gap values are 5.55 eV for the host and 5.80 eV for the phosphor doped with 4 mol% Er3+, as illustrated in Fig. 14. The fluctuations in the optical band gap observed in both pure and doped samples could be attributed to the level of structural disorder present in the host matrix.
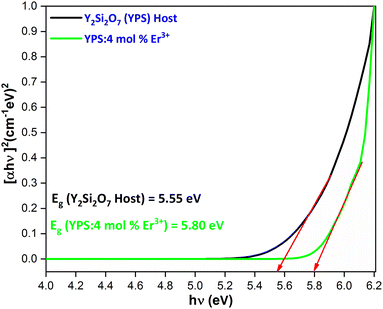 |
| Fig. 14 for the Y2Si2O7 and Y1.96Si2O7:4 mol% Er3+ phosphor. | |
3.6 Temperature-dependent luminescence and activation energy
The thermal stability of materials is a crucial aspect for their application in phosphor-converted LEDs, especially since high-power LED chips are typically used in environments with elevated temperatures. Due to the effect known as thermal quenching, a phosphor's luminescence tends to diminish when its temperature is increased beyond ambient levels. However, for a phosphor material to be considered viable for real-world white LED (WLED) applications, it must maintain its conversion efficiency even at temperatures up to 423 K. This requirement is because the temperature of a nanophosphor layer can rise to match the LED's junction temperature, which generally occurs in the 373–423 K range.49 Fig. 15(a) shows how the luminescence spectra of Y2Si2O7 doped with 4 mol% Er3+ changes with temperature, ranging from 298 K to 498 K, under a 378 nm excitation. The data indicate a steady decrease in the luminescence intensity as the temperature increases. Fig. 15(b) details this reduction in emission intensity as the temperature rises, demonstrating a consistent decline attributed to the thermal quenching effect. Interestingly, the luminous intensity at 423 K remains at 84.2% of its original intensity at 298 K, suggesting that this synthesized sample is promising for use in white LED (wLED) applications. To gain deeper insight into the thermal-quenching behavior observed in the prepared material, the activation energy was calculated employing a specific formula (16). This calculation is essential for quantifying the energy barrier that electrons must overcome during thermal quenching, providing insights into the material's thermal stability and its performance in a high-temperature environment. |
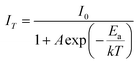 | (16) |
here, k is the Boltzmann's constant (8.617105 × 10−5 eV K−1), A is a constant; and IT and I0 stand for the luminous intensity at the target temperature and room temperature, separately. Ea is the activation energy needed for thermal quenching. In general, greater thermal stability exists when the Ea value is higher. Thus, the dependency of ln[(I0/IT) − 1)] on 1/kT may be expressed as.50 |
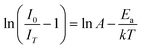 | (17) |
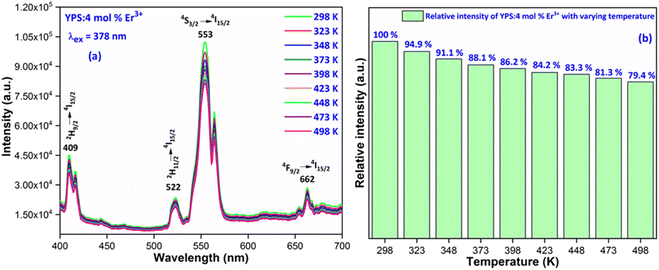 |
| Fig. 15 (a) Temperature-dependent photoluminescence and (b) relative emission intensity with varying temperature of the Y1.96Si2O7:4 mol% Er3+ phosphor. | |
This relationship is illustrated in Fig. 16, where the activation energy for the phosphor is calculated to be approximately 0.2641 eV, derived from the slope of the linear fit to the data plotted on the graph. In this case, the relatively high activation energy suggests that the phosphor possesses good thermal stability, enabling it to maintain performance at higher temperatures.
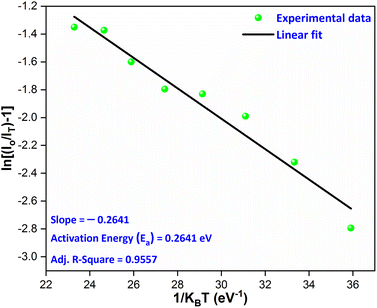 |
| Fig. 16 Linear fitted graph for the calculation of the activation energy. | |
3.7 Photometric investigation
The color emission characteristics of the YPS:xEr3+ samples (x = 1–7 mol%) were analyzed, resulting in color points plotted on CIE charts (Figs. S1–S7†). These coordinates (Table 5) consistently fall within the green region, confirming the uniform color output. The green emission coordinates adhere to the criteria set by both the European Broadcasting Union and the National Television System Committee, making these materials suitable for incorporation into the green segment of RGB-based white LEDs. Table 5 elaborates the color purity, which can be computed via the following formula.51 |
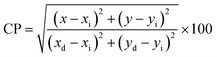 | (18) |
Table 5 Chromaticity parameters of Y2−xSi2O7:xEr3+ (x = 1–7 mol%) phosphors
Sample |
(x, y) |
CP (%) |
(u′, v′) |
CCT (K) |
YPS:1 mol% Er3+ |
0.3504, 0.4518 |
83.56 |
0.1815, 0.5267 |
5085 |
YPS:2 mol% Er3+ |
0.3496, 0.4507 |
85.04 |
0.1814, 0.5262 |
5102 |
YPS:3 mol% Er3+ |
0.3505, 0.4500 |
82.55 |
0.1821, 0.5261 |
5081 |
YPS:4 mol% Er3+ |
0.3528, 0.4544 |
87.23 |
0.1822, 0.5279 |
5034 |
YPS:5 mol% Er3+ |
0.3491, 0.4509 |
80.19 |
0.1811, 0.5262 |
5114 |
YPS:6 mol% Er3+ |
0.3472, 0.4476 |
78.08 |
0.1809, 0.5248 |
5156 |
YPS:7 mol% Er3+ |
0.3504, 0.4508 |
79.45 |
0.1818, 0.5263 |
5083 |
The equation calculates the color purity using the coordinates (x, y) of the current synthesized nanomaterials, ideal-white-light (xi, yi), and dominant wavelength (xd, yd). The highest achieved purity is 87.23% for the optimum sample. The analysis characterizes green emission by determining the correlated color temperature (CCT) using the McCamy formula. This calculation offers a comprehensive insight into the nature (warm or cool) of emitted light.52
|
CCT = −437n3 + 3601n2 − 6861n + 5514.31
| (19) |
The formula n = (x − xc)/(y − yc) is used, where (xc, yc) represents (0.332, 0.186). Calculating the (u′, v′) coordinates using the given equations is a crucial step, enabling a thorough exploration of the chromaticity characteristics of luminous nanomaterials.53
|
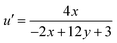 | (20) |
|
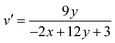 | (21) |
Table 5 lists the CCT values and (u′, v′) coordinates for the YPS:Er3+ (x = 1–7 mol%) phosphors. Each sample demonstrates the correlated color temperature (CCT) values exceeding 5000 K, suggesting their suitability as light sources for producing cool white illumination. This is illustrated in the CCT chart in Fig. S8–S14.†
4 Conclusions
In summary, we utilized a urea-assisted gel-combustion method to produce progressively green glowing Y2Si2O7:Er3+ phosphors. These phosphors endured comprehensive characterization, including structural, morphological, optical, photoluminescence, temperature-dependent luminescence and colorimetric analyses. The synthesized phosphors exhibited a triclinic phase with space group P
, and TEM revealed nearly spherical particles ranging from 25–45 nm. EDX spectra confirmed the effective assimilation of Er3+ ions into the YPS host matrix. The phosphors displayed a strong emission peak at 548 nm, corresponding to the characteristic 4S3/2 → 4I15/2 transition, when excited by a wavelength of 379 nm. This results in a vibrant green luminescence. Notably, the optimal luminescence occurred at 4 mol% Er3+ content, with concentration quenching observed beyond this level due to dipole–dipole interactions. Decay lifetimes decreased from 4.3492 to 2.9420 ms with increasing dopant ion concentration. Optical band gap analysis yielded a value of 5.80 eV for the YPS:4 mol% Er3+ phosphor, indicating a broad band gap material. Moreover, the phosphors emitted green light with high color purity within the suitable CIE zone. Overall, these phosphors exhibit promising characteristics for applications requiring thermally stable light-emitting diodes.
Data availability
Data will be made available on request.
Author contributions
Pawan Kumar: data curation, writing – original draft, investigation, methodology; Devender Singh: writing – review & editing, resources, supervision; Sitender Singh: validation; Harish Kumar: software; Ramesh Kumar: visualization.
Conflicts of interest
The authors declare that they have no known competing financial interests or personal relationships that could have appeared to influence the work reported in this paper.
Acknowledgements
Pawan Kumar is thankful to UGC-New Delhi for providing SRF [117/(CSIRNETJUNE2019)]. Devender Singh is grateful to MDU, Rohtak, for the Post Seed Grant under the research promotion scheme.
References
- H. Chander, Development of nanophosphors—A review, Mater. Sci. Eng., R, 2005, 49, 113–155, DOI:10.1016/j.mser.2005.06.001.
- I. Gupta, S. Singh, S. Bhagwan and D. Singh, Rare earth (RE) doped phosphors and their emerging applications: A review, Ceram. Int., 2021, 47, 19282–19303, DOI:10.1016/j.ceramint.2021.03.308.
- P. Kumar, S. Singh, I. Gupta, V. Kumar and D. Singh, Structural refinement and optical characteristics of single-phase Gd3Al5O12:Er3+ nanophosphors for luminescent applications, J. Lumin., 2022, 252, 119338, DOI:10.1016/j.jlumin.2022.119338.
- V. V. Shanbhag, S. C. Prashantha, T. S. Shekhar, H. Nagabhushana, R. Naik, K. M. Girish and D. S. Prasanna, Enhanced photoluminescence of SiO2 coated CaTiO3:Dy3+, Li+ nanophosphors for white light emitting diodes, Ceram. Int., 2021, 47, 10346–10354, DOI:10.1016/j.ceramint.2020.11.077.
- I. Gupta, D. Singh, S. Singh, P. Kumar, S. Bhagwan and V. Kumar, Structural and luminescent features of warm reddish-orange light-emitting Sm(III) doped Gd2Si2O7 nanophosphors for near UV-energized LEDs, J. Lumin., 2023, 263, 20007, DOI:10.1016/j.jlumin.2023.120007.
- W. Li, N. Ma, B. Devakumar and X. Huang, Blue-light-excitable broadband yellow-emitting CaGd2HfSc(AlO4)3: Ce3+ garnet phosphors for white light-emitting diode devices with improved color rendering index, Mater. Today Chem., 2022, 23, 100638, DOI:10.1016/j.mtchem.2021.100638.
- B. Marí, K. C. Singh, P. Cembrero-Coca, I. Singh, D. Singh and S. Chand, Red emitting MTiO3 (M = Ca or Sr) phosphors doped with Eu3+ or Pr3+ with some cations as codopants, Displays, 2013, 34, 346–351, DOI:10.1016/j.molstruc.2021.131802.
- P. Kumar, S. Singh, I. Gupta, K. Nehra, V. Kumar and D. Singh, Structural and luminescent behaviour of Dy (III) activated Gd3Al5O12 nanophosphors for white-LEDs applications, Mater. Chem. Phys., 2023, 295, 127035, DOI:10.1016/j.matchemphys.2022.127035.
- D. Liu, X. Yun, P. Dang, H. Lian, M. Shang, G. Li and J. Lin, Yellow/orange-emitting ABZn2Ga2O7: Bi3+ (A= Ca, Sr; B= Ba, Sr) phosphors: optical temperature sensing and white light-emitting diode applications, Chem. Mater., 2020, 32, 3065–3077, DOI:10.1021/acs.chemmater.0c00054.
- V. Tanwar, S. Singh, I. Gupta, P. Kumar, H. Kumar, B. Mari and D. Singh, Preparation and luminescence characterization of Eu(III)-activated Forsterite for optoelectronic applications, J. Mol. Struct., 2022, 1250, 131802, DOI:10.1016/j.molstruc.2021.131802.
- P. Kumar, D. Singh, I. Gupta, S. Singh, V. Kumar, H. Kumar and S. K. Chhikara, Perovskite GdAlO3:Dy3+ nanophosphors: A gel-combustion synthesis, phase evaluation and down conversion luminescent characteristics for lighting applications, J. Lumin., 2022, 252, 119409, DOI:10.1016/j.jlumin.2022.119409.
- P. Dang, D. Liu, Y. Wei, G. Li, H. Lian, M. Shang and J. Lin, Highly efficient cyan-green emission in self-activated Rb3RV2O8 (R= Y, Lu) vanadate phosphors for full-spectrum white light-emitting diodes (LEDs), Inorg. Chem., 2020, 59, 6026–6038, DOI:10.1021/acs.inorgchem.0c00015.
- P. Kumar, D. Singh, I. Gupta, S. Singh, S. Nehra and R. Kumar, Combustion derived single phase Y4Al2O9: Tb3+ nanophosphor: crystal chemistry and optical analysis for solid state lighting applications, RSC Adv., 2023, 13, 7752–7765, 10.1039/D3RA00735A.
- H. Lin, T. Hu, Q. Huang, Y. Cheng, B. Wang, J. Xu and Y. Wang, Non-rare-earth K2XF7: Mn4+ (X= Ta, Nb): a highly-efficient narrow-band red phosphor enabling the application in wide-color-gamut LCD, Laser Photon. Rev., 2017, 11, 1700148, DOI:10.1002/lpor.201700148.
- J. E. Murphy, F. Garcia-Santamaria, A. A. Setlur and S. Sista, PFS, K2SiF6: Mn4+: the Red-line Emitting LED Phosphor behind GE's TriGain Technology Platform, Dig. Tech. Pap., 2015, 46, 927–930, DOI:10.1002/sdtp.10406.
- E. Song, Y. Zhou, X. B. Yang, Z. Liao, W. Zhao, T. Deng and Q. Zhang, Highly efficient and stable narrow-band red phosphor Cs2SiF6:Mn4+ for high-power warm white LED applications, ACS Photonics, 2017, 4, 2556–2565, DOI:10.1021/acsphotonics.7b00852.
- G. Chen, L. Luo, W. Li, J. S. Yu and P. Du, Designing tunable luminescence in Ce3+/Eu2+-codoped Ca8Zn(SiO4)4Cl2 phosphors for white light-emitting diode and optical anti-counterfeiting applications, Mater. Today Chem., 2022, 26, 101038, DOI:10.1016/j.mtchem.2022.101038.
- P. Kumar, D. Singh and H. Kumar, Gel-combustion synthesis, structural and optoelectronic analyses of Tb3+ activated LaSr2AlO5 nanophosphors: A green emitter for displays, Mater. Sci. Semicond. Process., 2024, 174, 108162, DOI:10.1016/j.mssp.2024.108162.
- J. Du, X. Pan, Z. Liu, Y. Jing, B. Wang, L. Luo and P. Du, Highly efficient Eu3+-activated Ca2Gd8Si6O26 red-emitting phosphors: a bifunctional platform towards white light-emitting diode and ratiometric optical thermometer applications, J. Alloys Compd., 2021, 859, 157843, DOI:10.1016/j.jallcom.2020.157843.
- I. Gupta, D. Singh, P. Kumar, S. Singh, S. Bhagwan and V. Kumar, Crystallographic and luminescence studies of Gd2Si2O7: Er3+ nanomaterials for NUV energized lighting applications, J. Mol. Struct., 2023, 1287, 135595, DOI:10.1016/j.molstruc.2023.135595.
- P. Liu, F. Wang and B. Yang, Upconversion/downconversion luminescence of color-tunable Gd2O3: Er3+ phosphors under ultraviolet to near-infrared excitation, Solid State Sci., 2020, 102, 106165, DOI:10.1016/j.solidstatesciences.2020.106165.
- P. Kumar, S. Singh, I. Gupta, V. Kumar and D. Singh, Preparation and luminescence behaviour of perovskite LaAlO3: Tb3+ nanophosphors for innovative displays, Optik, 2022, 267, 169709, DOI:10.1016/j.ijleo.2022.169709.
- Y. Zhang, Z. Liang, W. Wang, Y. Lin, R. Tang, J. Wang and R. Yu, Optical properties of yellow-emitting Sr3LaNb3O12: Dy3+ phosphors with an abnormal thermal quenching for white light-emitting diode applications, J. Mater. Sci.: Mater. Electron., 2022, 33, 26619–26632, DOI:10.1007/s10854-022-09374-4.
- J. Sokolnicki, Rare earths (Ce, Eu,Tb) doped Y2Si2O7 phosphors for white LED, J. Lumin., 2013, 134, 600–606, DOI:10.1016/j.jlumin.2012.07.023.
- L. Marciniak, D. Hreniak, W. Strek, F. Piccinelli, A. Speghini, M. Bettinelli and F. Priolo, Spectroscopic and structural properties of polycrystalline Y2Si2O7 doped with Er3+, J. Lumin., 2016, 170, 614–618, DOI:10.1016/j.jlumin.2015.02.015.
- D. Singh, V. Tanwar, A. P. Simantilleke, S. Bhagwan, B. Mari, P. S. Kadyan and I. Singh, Synthesis and enhanced luminescent characterization of SrAl4O7:Eu2+, RE3+ (RE = Nd, Dy) nanophosphors for light emitting applications, J. Mater. Sci.: Mater. Electron., 2016, 27, 5303–5308, DOI:10.1007/s10854-016-4428-2.
- C. Y. Yang, S. Som, S. Das and C. H. Lu, Synthesis of Sr2Si5N8:Ce3+ phosphors for white LEDs via efficient chemical vapor deposition, Sci. Rep., 2017, 7, 45832, DOI:10.1038/srep45832.
- J. Miranda de Carvalho, C. C. S. Pedroso, M. S. D. N. Saula, M. C. F. C. Felinto and H. F. D. Brito, Microwave-assisted preparation of luminescent inorganic materials: A fast
route to light conversion and storage phosphors, Molecules, 2021, 26, 2882, DOI:10.3390/molecules26102882.
- P. Kumar, S. Singh, I. Gupta, V. Kumar and D. Singh, Structural and optical characterization of trivalent samarium-activated LaAlO3 nanocrystalline materials for solid-state lighting, J. Mol. Struct., 2022, 1265, 133362 CrossRef CAS.
- R. H. Krishna, B. M. Nagabhushana, B. N. Sherikar, N. S. Murthy, C. Shivakumara and T. Thomas, Luminescence enhancement in monoclinic CaAl2O4: Eu2+, Cr3+ nanophosphor by fuel-blend combustion synthesis, Chem. Eng., 2015, 267, 317–323, DOI:10.1016/j.cej.2014.12.102.
- P. Kumar, D. Singh, I. Gupta, S. Singh, S. Nehra and R. Kumar, A study of phase evolution, crystallographic and down-conversion luminescent behaviour of monoclinic Y4Al2O9:Dy3+ nanophosphors for white light applications, Opt. Mater., 2023, 138, 113677, DOI:10.1016/j.optmat.2023.113677.
- V. Kahlenberg, W. Wertl, D. M. Többens, R. Kaindl, P. Schuster and H. Schottenberger, Rietveld analysis and raman spectroscopic investigations on α-Y2Si2O7, Z. Anorg. Allg. Chem., 2008, 634, 1166–1172, DOI:10.1002/zaac.200700548.
- S. K. Sharma, S. Som, R. Jain and A. K. Kunti, Spectral and CIE parameters of red emitting Gd3Ga5O12: Eu3+ phosphor, J. Lumin., 2015, 159, 317–324, DOI:10.1016/j.jlumin.2014.11.010.
- P. Kumar, D. Singh, I. Gupta and H. Kumar, Influence of Dy3+ ion concentration on structural, photoluminescence and energy transfer mechanism of promising GdSr2AlO5 nanophosphors for white light applications, Ceram. Int., 2023, 49, 29010–29024, DOI:10.1016/j.ceramint.2023.06.173.
- S. K. Pathak, A. Verma and A. Verma, Structural and photoluminescence properties of Eu3+ activated ZnAl2O4 orange-red phosphor, J. Mater. Sci.: Mater. Electron., 2020, 31, 16137–16149, DOI:10.1007/s10854-020-03715-x.
- A. Mondal and J. Manam, Structural, optical and temperature dependent photoluminescence properties of Cr3+-activated LaGaO3 persistent phosphor for optical thermometry, Ceram. Int., 2020, 46, 23972–23984, DOI:10.1016/j.ceramint.2020.06.174.
- P. Kumar, D. Singh and I. Gupta, UV excitable GdSr2AlO5:Eu3+ red emitting nanophosphors: Structure refinement, photoluminescence, Judd-Ofelt analysis and thermal stability for w-LEDs, J. Alloys Compd., 2023, 966, 171410, DOI:10.1016/j.jallcom.2023.171410.
- I. Gupta, D. Singh, S. Singh, P. Kumar, S. Bhagwan and V. Kumar, Structural and photophysical measurements of Er3+ doped Gd4Al2O9 nanophosphors for NUV excitable solid-state lighting applications, Chem. Phys. Lett., 2023, 814, 140350, DOI:10.1016/j.cplett.2023.140350.
- I. Gupta, P. Kumar, S. Singh, S. Bhagwan, S. K. Chhikara and D. Singh, Crystal configuration, spectroscopic and optical characteristics of Er3+ doped YAlO3 perovskites for advanced photonic appliances, Inorg. Chim. Acta, 2022, 543, 121183, DOI:10.1016/j.ica.2022.121183.
- R. Reddappa, L. L. Devi, P. Babu, I. R. Martín, V. Lavín, V. Venkatramu and C. K. Jayasankar, Structural, morphological and photoluminescence properties of Ca2SiO4: Er3+ phosphors synthesized from agro-food waste materials, Ceram. Int., 2022, 48, 37013–37019, DOI:10.1016/j.ceramint.2022.08.271.
- Y. Liu, Y. Liu, G. Liu, X. Dong and J. Wang, Up/down conversion, tunable photoluminescence and energy transfer properties of NaLa(WO4)2: Er3+, Eu3+ phosphors, RSC Adv., 2015, 5, 97995–98003, 10.1039/C5RA17370A.
- R. A. Talewar, S. K. Mahamuda, A. S. Rao and S. V Moharil, Intense infrared emission of Er3+ in ZnB2O4 phosphors from energy transfer of Bi3+ by broadband UV excitation, J. Lumin., 2022, 244, 118706, DOI:10.1016/j.jlumin.2021.118706.
- I. Gupta, P. Kumar, S. Singh, S. Bhagwan, V. Kumar and D. Singh, Phase recognition, structural measurements and photoluminescence studies of reddish-orange-emissive YAlO3: Sm3+ perovskite nanophosphors for NUV energized WLEDs, J. Mol. Struct., 2022, 1267, 133567, DOI:10.1016/j.molstruc.2022.133567.
- P. Kumar, D. Singh and I. Gupta, Physical insights into crystal structure and optical response of green light emitting Tb3+ activated GdSr2AlO5 nanophosphors for optical displays, Mater. Res. Bull., 2023, 167, 112413, DOI:10.1016/j.materresbull.2023.112413.
- P. Kumar, D. Singh, I. Gupta, S. Singh, S. Nehra and R. Kumar, Realization of warm reddish-orange light emitter single phase Y4Al2O9:Sm3+ nanophosphors for indoor lighting applications, J. Lumin., 2023, 257, 119703, DOI:10.1016/j.jlumin.2023.119703.
- K. Nehra, A. Dalal, A. Hooda, K. Jakhar, D. Singh and S. Kumar, Preparation, optoelectronic and spectroscopic analysis of fluorinated heteroleptic samarium complexes for display applications, Inorganica Chim. Acta, 2022, 537, 120958, DOI:10.1016/j.ica.2022.120958.
- D. Gao, Y. Li, L. Cheng, S. Liu, S. Xu, X. Li and B. Chen, Concentration effects of fluorescence quenching and optical transition properties of Dy3+ doped NaYF4 phosphor, J. Alloys Compd., 2022, 895, 162616, DOI:10.1016/j.jallcom.2021.162616.
- S. P. Ghorpade, R. H. Krishna, R. M. Melavanki, V. Dubey and N. R. Patil, Effect of Eu3+ on optical and energy bandgap of SrY2O4 nanophosphors for FED applications, Optik, 2020, 208, 164533, DOI:10.1016/j.ijleo.2020.164533.
- N. M Bhiri, M. Dammak, M. Aguilo, F. Diaz, J. J. Carvajal and M. C. Pujol, Stokes and anti-Stokes operating conditions dependent luminescence thermometric performance of Er3+-doped and Er3+, Yb3+ co-doped GdVO4 microparticles in the non-saturation regime, J. Alloys Compd., 2020, 814, 152197, DOI:10.1016/j.jallcom.2019.152197.
- P. Kumar, D. Singh, I. Gupta and H. Kumar, Synthesis, crystallographic structure, down shifting luminescence of Er(III) activated GdSr2AlO5 nanophosphors: An efficient green emitter for solid state lighting, Mater. Sci. Semicond. Process., 2023, 167, 107765, DOI:10.1016/j.mssp.2023.107765.
- P. Kumar, S. Singh, I. Gupta, A. Hooda, V. Kumar and D. Singh, Reddish-orange color tunable Sm3+ activated Gd3Al5O12 phosphors: crystallographic and photophysical investigation for lighting applications, J. Mol. Struct., 2023, 1271, 134074, DOI:10.1016/j.molstruc.2022.134074.
- P. Kumar, S. Singh, I. Gupta, A. Dalal, V. Kumar and D. Singh, Preparation, structural and photometric properties of single-phased Gd3Al5O12:Tb3+ green-emitting phosphors for solid state lighting purpose, Mater. Sci. Eng., B, 2023, 288, 166189, DOI:10.1016/j.mseb.2022.116189.
- P. Kumar, D. Singh and H. Kumar, Red-emitting Eu3+ activated LaSr2AlO5 phosphor for wLEDs: Crystal structure, photoluminescence, thermal stability, Judd-Ofelt calculation and band-gap analyses, Mater. Res. Bull., 2024, 173, 112683, DOI:10.1016/j.materresbull.2024.112683.
|
This journal is © The Royal Society of Chemistry 2024 |