Abstract
In this study, a new composite from silica coated MnFe2O4 nanoparticles, diethylenetriamine, 3-chloropropyl trimethoxysilane and Mg–Al Layered Double Hydroxide (Mg–Al LDH/DETA/CPTMS/SCNPs) composite was synthesized. The Mg–Al LDH/DETA/CPTMS/SCNPs composite was examined by Fourier transform infrared spectrometer (FT-IR), Scanning Electron Microscopy (SEM), Energy Dispersive X-ray (EDS), X-ray diffraction (XRD), Thermogravimetric Analysis (TGA) and Vibrating Sample Magnetometry (VSM). The synthesized composite exhibited magnetic property with a saturation magnetization of 0.40 emu g−1. The Mg–Al LDH/DETA/CPTMS/SCNPs composite was utilized as a successful adsorbent for removal of tetracycline from aqueous solutions. The effect of various operation factors such as initial drug concentration, adsorbent dosage, pH and contact time were investigated. The optimized variable conditions such as adsorbent dose of 60 mg L−1, drug concentration of 100 mg L−1, pH = 7 and contact time 30 min were obtained. For describing the adsorption isotherms, the Langmuir, Freundlich and Temkin adsorption models were utilized. The results indicated that the adsorption isotherm is in good agreement with Langmuir model. According to the Langmuir analysis, the maximum adsorption capacity (qm) of the Mg–Al LDH/DETA/CPTMS/SCNPs composite for tetracycline was obtained to be 40.16 mg g−1. The kinetic studies revealed that the adsorption in all cases to be a pseudo second-order process. The negative value of ΔG° and the positive value of ΔH° showed the adsorption process to be spontaneous and endothermic.
3.2.5. Adsorption isotherm. One of the important methods to investigate the relationship between the amount of adsorbed on adsorbent in the solution phase is adsorption isotherm.46 To describe the relationship between the adsorbed amount on adsorbent, several isotherm models have been developed.47 In the present study, various adsorption isotherm models like those of Langmuir, Freundlich and Temkin were applied to evaluating the adsorption of drug from solution. The Langmuir isotherm model is based on the monolayer adsorption of the adsorbate onto the adsorbent surface.48 The eqn (4) shows the linear form of the Langmuir model: |
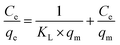 | (4) |
where, Ce is the equilibrium concentration of the dye solution (mg L−1), qe (mg g−1) is the amount of dye adsorbed, qm is the value of monolayer adsorption capacity in Langmuir model and KL: constant value of Langmuir (mg L−1). The Langmuir plot for the adsorption of tetracycline onto Mg–Al LDH/DETA/CPTMS/SCNPs composite is shown in Fig. 13. The values of qm and KL were calculated from the linear regression plot of (Ce/qe) versus Ce at 25 °C.
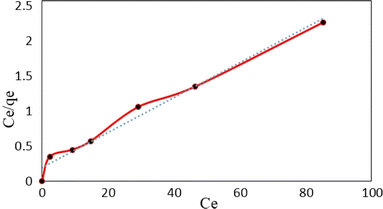 |
| Fig. 13 Langmuir plot for the adsorption of tetracycline (200 rpm, 25 °C and pH = 7). | |
The Freundlich isotherm model demonstrates a heterogeneous adsorption of adsorbate onto the adsorbent surface. Eqn (5) shows the linear form of the Freundlich isotherm model:
|
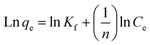 | (5) |
KF and
n are experimental constants where
KF is adsorption capacity at unit concentration (L mg
−1) and
n shows the intensity of adsorption. The 1/
n values can be classified as irreversible (1/
n = 0), favorable (0 < 1/
n < 1) and unfavorable (1/
n > 1). The values of
KF and
n were determined from the intercept and slope of the plot of ln
qe versus ln
Ce at 25 °C (
Fig. 14). Furthermore, the dimensionless separation factor (
RL) was calculated by the following
eqn (6):
|
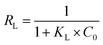 | (6) |
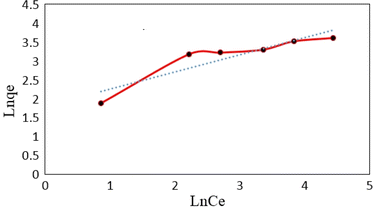 |
| Fig. 14 Freundlich plot for the adsorption of tetracycline (200 rpm, 25 °C and pH = 7). | |
The values of RL can demonstrate the shape of the isotherm to be either unfavorable (RL > 1), linear (RL = 1), favorable (0 < RL < 1) or irreversible (RL = 0).
The Temkin adsorption isotherm directly investigates account of adsorbent–adsorbate interactions. In this model, first, heat of adsorption would decrease linearly rather than logarithmic, then uniform distribution of binding energies up to some maximum binding energy.49 Eqn (7) and (8) show the Temkin isotherm model:
|
 | (8) |
where
R is gas constant 8.314 J mol
−1 K
−1.
T is absolute temperature (K),
b is the Temkin constant related to the heat of adsorption (J mol
−1) and
A is the equilibrium binding constant corresponding to the maximum binding energy (L g
−1). The values of
A and
b can be determined from the intercept and slope of the linear plot of
qe versus ln
Ce (
Fig. 15). The Langmuir, Freundlich and Temkin parameters and the regression coefficients
R2 of the adsorption of tetracycline onto Mg–Al LDH/DETA/CPTMS/SCNPs composite are given in
Table 1. As seen in
Table 1, calculated amount of
RL was found between 0 and 1, which confirmed a favorable adsorption process for tetracycline removal using Mg–Al LDH/DETA/CPTMS/SCNPs composite. Also according to
Table 1, the correlation coefficient (
R2) of the Langmuir isotherm was greater than that of the Freundlich and Temkin isotherm models for the adsorption of investigated drug. The applicability of the Langmuir model suggests homogeneous surfaces of the composite and monolayer coverage of tetracycline onto the adsorbent. On the basis of the Langmuir analysis, the maximum adsorption capacity was 40.12 mg g
−1 for tetracycline.
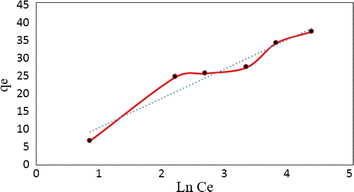 |
| Fig. 15 Temkin plot for the adsorption of tetracycline (200 rpm, 25 °C and pH = 7). | |
Table 1 Langmuir, Freundlich and Temkin isotherms parameters and correlation coefficients for the adsorption of tetracycline onto Mg–Al LDH/DETA/CPTMS/SCNPs composite
Langmuir isotherm parameters |
Freundlich isotherm parameters |
Temkin isotherm parameters |
qm (mg g−1) |
KL (L mg−1) |
RL |
R2 |
KF (L mg−1) |
n |
R2 |
A |
b (kJ mol−1) |
B |
R2 |
40.12 |
0.125 |
0.13 |
0.982 |
66.06 |
2.23 |
0.841 |
1.86 |
0.301 |
8.23 |
0.9434 |