DOI:
10.1039/D4RA00034J
(Review Article)
RSC Adv., 2024,
14, 9137-9158
Development of electrochemical sensors for quick detection of environmental (soil and water) NPK ions
Received
2nd January 2024
, Accepted 23rd February 2024
First published on 19th March 2024
Abstract
All over the world, technology is becoming more and more prevalent in agriculture. Different types of instruments are already being used in this sector. For the time being, every farmer is trying to produce more crops on a piece of land. Eventually, soil loses its nutrients; however, to grow more crops, farmers use more fertilizers without knowing the proper conditions of the soil in real time. To overcome this issue, many scientists have recently focused on developing electrochemical sensors to detect macronutrients, i.e., nitrogen (N), phosphorus (P), and potassium (K), in soil or water rapidly. In this review, we focus mainly on the recent developments in electrochemical sensors used for the detection of nutrients (NPK) in different types of samples. As it is outlined, the use of smart and portable electrochemical sensors can be helpful for the reduction of excess fertilizer and can play a vital role in maintaining suitable conditions in soils and water. We are optimistic that this review can guide researchers in the development of a portable and suitable NPK detection system for soil nutrients.
1. Introduction
Nowadays, advanced technologies are becoming more and more popular, and covering various aspects to improve our daily lives. In agriculture, a variety of technological tools and engineering nanoparticle-based fertilizers1 have already been developed to facilitate agricultural work and to increase crop yield. Various macronutrients and micronutrients in soil are considered the most important factors for good harvesting. Micronutrients, especially nitrogen (N), phosphorus (P), and potassium (K), play an important roles in the cultivation and production of crops.2 An adequate amount of NPK in the soil is required for optimal crop production. When NPK deficiency occurs in the root zone, it can lead to different types of syndromes such as yellowing of leaves, spots on leaves, and reduction of flowers and fruits, and details of deficiency are described in the previously reported studies.3–5
To reduce this deficiency in plants, farmers use various fertilizers to supply these nutrients without knowing the present condition, i.e., the need for nutrients by the plants. As a result, sometimes they use excess amounts of fertilizers for the fertilization of the plants, results in various negative impacts such as water pollution, eutrophication, soil contamination, and reduction in the population of soil microorganisms.6,7 To optimize crop production, it is important to know how much NPK is present in the soil and how much NPK should be added at that time.8,9 Various analytical techniques such as gas chromatography-mass spectroscopy, ion-chromatography, atomic absorption spectroscopy, UV-vis spectroscopy, and field effect transistor have been used for the determination and quantification of different types of chemical elements and compounds; however, these methods are very expensive, need a well-arranged set up, time consuming, require skilled person for data acquisition and interpretation, and the vigilant way for sample preparation though these methods are provided with high accuracy and precision.10–12
For these reasons, the development of a simple, economically feasible, and rapid technique for the detection of NPK is crucial. In the last decade, various types of sensors have been developed for the detection of NPK in soil, such as electrochemical sensors, optical sensors, and mass-sensitive biosensors. Among these biosensors, an electrochemical sensor is one of the most sensitive and rapid tools for NPK detection. It is a promising strategy for developing an innovative, cost-effective, and portable NPK testing method for soil. The use of this electrochemical sensor has many advantages; for example, this type of sensor is more sensitive, reproducible, and repeatable than other sensors2 and is sometimes comparable with conventional techniques.
The sensitivity and selectivity of an electrochemical sensor mainly depend on the surface modification of the transducer. Nowadays, researchers are interested in using different types of nanomaterials to modify the transducer. Metal and metal oxide nanoparticles are mainly used to develop transducers for electrochemical sensors for soil testing, environmental analysis, food safety,13 health management, and clinical diagnostics due to their low cost, easy modification, and availability. Nanomaterials of different sizes and shapes have been used for the development of electrochemical sensors.
In this review article, we will discuss recent developments of different kinds of sensors used for the detection of NPK in different environmental samples with the current state of research and their mechanism of operation. We review several nanomaterial-based electrochemical sensors and summarize various parameters of different NPK sensors that have been previously reported. We also discuss some prospects for electrochemical sensors based on nanomaterials as alternative soil tests.
2. Conventional technique of NPK testing
In the traditional technique of NPK investigation of agricultural fields, soil samples were taken randomly from different locations in the field. The samples were mixed and packed and levelled well. Then, the samples were transported to a laboratory where the samples were chemically processed for various analyses. Then, the processed samples were analyzed in a state-of-the-art laboratory using various expensive instruments. Analytical instruments include atomic mass spectroscopy (AAS), UV-visible spectroscopy, gas chromatography-mass spectroscopy (GCMS), and field effect transistors. These types of test methods sometimes require more than one day for sample processing and analysis and trained personnel for instrument operation and chemical processing.
Actually, nowadays, electrochemical sensing is getting popular as conventional methods are very expensive, need a well-arranged setup, are time-consuming, and require a skilled person for data acquisition and interpretation, a vigilant way for sample preparation though these methods are provided with high accuracy and precision,10–12 we have mentioned earlier, here the detection range and limit of conventional methods vary from sample to sample; for example, for the atomic adsorption spectroscopy (AAS) the detection limits for most elements (ppm) and absolute detection limits (μg) is 0.01–5, and the details are discussed in these ref. 14–21. Actually, this part should be focused more carefully as there are hardly any review papers providing a summary of the conventional methods. Here, we have mentioned a few of the conventional techniques without providing any detailed explanations.
Smart electrochemical sensors, based on microcontroller potentiostat, i.e. very cheap and versatile platform in electrochemistry and instrumentation35 and wireless communication technology36,37 have also been widely used for human health monitoring such as blood glucose, blood pressure, heart rate, wrist pulse, and other health-related conditions.38–43 If we design electrochemical sensors for sensing NPK in soil or soil water samples, a quick, accurate and easy determination is possible, thus introducing a smart sensing system in agriculture. In the schematic figure, we show that by using this kind of electrochemical sensor, designed with a smartphone-based application, it may be possible to determine the desired ion present in the soil sample dispersed in water. Already these kinds of electrochemical sensors have been developed for specific ion detection in urine or in blood samples as described elsewhere in the ref. 16–21. Therefore, we should try to improve this kind of system to detect different macronutrients in soil samples/water solutions.
2.1 Advanced electrochemical sensors for the detection of nitrogen (N)
Nitrogen is one of the most important elements in the environment and occurs in several chemical forms, including nitrate (NO3−), nitrite (NO2−), ammonium (NH4+), nitrous oxide (N2O), nitrogen oxide (NO), and nitrogen gas (N2). Recently, farmers have been using fertilizers in an inefficient and uncontrolled manner to meet the demand for surplus food production for the gradually growing population.44 These increasing amounts of N species have been shown to have a range of negative impacts on terrestrial and aquatic ecosystems.45,46 To avoid excessive use of fertilizers and to use the fertilizers properly, farmers need to monitor the nitrogen species consumed by crops in a short time. An advanced electrochemical sensor is a highly sensitive, selective, low-cost, and easily measurement in situ device that enables fertilizer selection for cultivation in real-time.
2.1.1. Nitrate ion detection. Nitrates are the most oxidized form of nitrogen found in soil and water in the form of highly soluble and mobile mineral salts.47 Previous studies indicate that the nitrate ion can serve as the basis for the nitrogenous nutrition of plants and soil microorganisms.48,49 The easily detectable nitrate ions can be detected and quantified by an electrochemical sensor, as summarized in Table 1.
Table 1 A comparison of electrochemical sensors for the detection of nitrate ions
Analytical method |
Linear range |
Low level of detection |
Sample |
Analyte |
References |
UV-visible spectrometry |
1–100 μg mL−1 |
0.33 μg mL−1 |
Drinking water |
Nitrate |
22 |
UV-visible spectrometry |
0.5–13.7 mg L−1 |
— |
Municipal waste water |
Nitrate |
23 |
Ultraviolet (UV) light |
0.08–4.0 mg L−1 |
0.04 mg L−1 |
Natural waters |
Nitrate |
24 |
UV-visible spectrometry |
0.5–3.0 mg L−1 |
— |
Agricultural soil samples and water samples |
Phosphates |
25 |
UV-visible spectrometry |
0.5–5.0 μg mL−1 |
— |
Soil/water |
Phosphates |
26 |
Ion chromatography |
4.30–4.36 mg L−1 |
0.03 mg L−1 |
Soil extract |
Nitrate |
27 |
Ion chromatography |
0.90–0.92 mg L−1 |
0.02 mg L−1 |
Soil extract |
Phosphates |
27 |
Ion chromatography |
0.30 to 5.6 mg L−1 |
0.10 mg L−1 |
Natural waters |
Potassium |
28 |
Gas chromatography-mass spectrometry |
0.02–10 mg L−1 |
3 μg L−1 |
Natural waters |
Nitrate |
29 |
Gas chromatography-mass spectrometry |
0.5–208.5 mg L−1 |
0.176 mg L−1 |
Water |
Potassium formate |
30 |
Headspace gas chromatography (HS-GC) |
— |
2.5 mg kg−1 |
Soil sample |
Phosphorus |
31 |
High-performance liquid chromatography with UV detection |
0.2–200 ppm |
9.5 mg L−1 |
Environmental water samples |
Nitrate |
32 |
0.2–200 ppm |
1.33 mg L−1 |
Environmental water samples |
Phosphate |
Graphene-based field-effect transistor |
0.001–100 mg L−1 |
1.1 μg L−1 |
Water |
Nitrates |
33 |
ZnO nanorods field-effect transistors (FETs) |
1–5000 μM |
1.0 μM |
Nutrient solution or water |
Phosphate |
34 |
2–25 000 μM |
0.1 μM |
Nitrates |
2–15 000 μM |
0.4 μM |
Potassium |
Liang et al. reported a method to determine the concentration of soluble nitrate ions in water. In their study, copper nanowires were annealed at 600 °C to obtain maximum reactive surface area for the reduction of nitrate ions.50 The schematic diagram of the nanowire-based copper electrode modification is shown in Fig. 1. However, the electrode was also prepared at an annealing temperature of 600 °C. The modified copper electrode showed a dynamic range of 8 to 5860 μM with a detection limit of 1.35 μM for nitrate ions in water. In another research work, a glassy carbon electrode was modified with copper, MWCNT, and RGO to detect nitrite ions by linear voltammetry (LSV).51 The detection of nitrate ions in tap and mineral water, sausage, salami, and cheese samples showed linearity from 0.1 to 75 μM with a detection limit of 30 nM at pH 3. Moreover, branched Ag nanoparticle electrodeposition was performed in an ammonium sulfate medium and the modified surface was well suited for electro catalytic reduction of nitrate.52 The ultra-microelectrode was able to detect nitrate ion concentration in a wide linear range (4–1000) μM. The modified electrode could be used for up to 100 interrogation cycles and was much more selective for the direct detection of nitrate ions in synthetic aquifer samples without pretreatment and pH adjustment of the solution used in the experiments. In addition, the detection of nitrate ions in agricultural soils has been performed using graphene oxide (GO) nanosheets and poly(3,4-ethylenedioxythiophene) nanofibers.53 These could detect nitrate ions from soil extracts. The modified surface GO allowed for increasing the charge transfer resistance of the modified electrode and could detect a wide concentration range of 0.44–442 mg L−1 in the soil medium. Moreover, graphene foam (GF) was added with titanium dioxide nanofibers and nitrate reductase enzyme molecules.54 In another work, nitrate reductase was entrapped in the growing PPy and the carboxyl group of CNT was immobilized. The optimal reactions were found in 0.1 M PBS at pH 7.5.55 This nitrate detection exhibits a linear range of 0.44–1.45 mM. Faisal and Abu have also shown that nitrate reductase can be immobilized on PPy surfaces to detect nitrate ions in wastewater.56
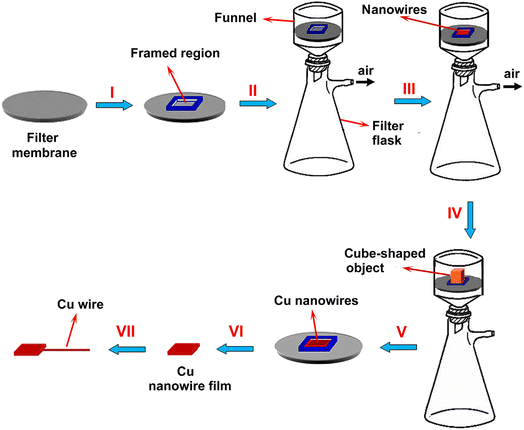 |
| Fig. 1 Thermal annealing preparation of nanowire-based Cu electrode, this figure has been adapted from ref. 50 with permission from Elsevier, copyright September 2016. | |
Wang et al. reported research on self-assembled graphene oxide (GO)-three-dimensional copper oxide nanoparticles. The coupling increased the sensitivity and catalytic activity of copper for the detection of nitrate ions, which was the highest priority.57 The electrochemical detection was carried out in seawater with a low detection limit of 7.89 μM. Moreover, the Ti nanocomposite electrode modified with graphene oxide nanosheets showed excellent durability for the detection of nitrate ions compared to Ti–Cu and Cu–Zn electrodes.58 In addition, a carbon fiber micro-disc electrode was coupled with square-wave voltammetry to investigate the effects of aerosols on human health. In this study, the nitrate concentration followed a linear response of 0.003–2 mM with a detection limit of 1.10 μM.59 Inam and others reported research work on the modification of Ag-working electrodes with electrodeposited copper nanoclusters in 2021. The modified electrode for nitrate ion detection showed a good linear concentration range (0.05 to 5) mM, with a detection limit of 0.207 nM. Another research study reported the detection of nitrate ions by an electrode modified with copper nanoclusters in freshwater.60 In addition, trace-etched polycarbamate membranes were electrochemically modified with copper nanowires. Detection of nitrate was performed in water samples containing chloride and nitrites.61 Moreover, the electrode modified with Pt–Cu showed a linear response between 0.12 and 4.75 mM concentration of nitrate ions neutral medium by the differential pulse voltammetry method. They found that Cu(I) and Cu(II) oxides reduced the reduction of nitrate, but this electrode showed excellent sensitivity (2.3782 μA μM−1 cm−2).
A new chemical sensor was developed on an ion-printed polymer matrix using copper nanoparticles and a polyaniline nanocomposite to detect nitrate ions. A monomer functionalized with aniline adheres to the copper nanoparticles and the electrode was used to detect nitrate in real water samples.62 Fu demonstrated the modification of a micro band electrode with a palladium-tin bimetallic composite using a microelectromechanical system. The double layer was formed by an electrochemical deposition method, which has improved electro catalytic activity.63 In this observation, they found repeatability and stability for 60 days, and a linear range from 1 mg L−1 to 20 mg L−1. In addition, poly(3-octyl-thiophene) and molybdenum disulfide (POT-MoS2) were applied to a gold electrode. The fabricated POT-MoS2 layer acted as an ion-to-electron conversion layer. The nanocomposites showed high accuracy over a period of 27 days.64 The detection of nitrate showed a dynamic linear range of 1–1500 ppm. The working principle of this soil sensor is shown in Fig. 2. Another research work dealt with the reduction of nitrate ions on AuNP surfaces on the surface of carbon paper electrodes functionalized with selenium particles.65 The modified electrode was successfully used in seawater samples with a detection limit of 8.6 μM.
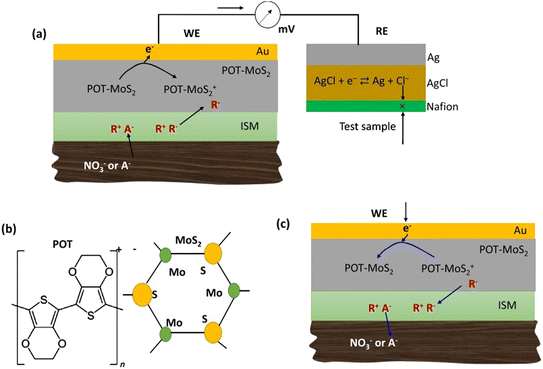 |
| Fig. 2 Graphical representation of the working principle of the soil sensor. (a) Oxidation process for the detection of nitrite ions, (b) molecular structure of POT and MoS2, and (c) mechanism of the reduction process of the working electrode, this figure has been adapted from ref. 64 with permission from American Chemical Society, copyright Aug 1, 2019. | |
Nitrate contamination of food and mineral water was also studied using the modified electrode with copper nanostructure. In this study, a hydrodynamic amperometric electrode was used to detect the concentration of nitrate ions, which had a linear dynamic range from 1 to 35 μM. The repeatability was evaluated as 2.3% and the detection limit was 0.59 μM. In addition, a two-layer bimetallic electrode modification was performed using two different salt solutions of copper and silver.66 The electrodeposited silver film behaves like a porous network-like structure and the average particle size was 200 nm. The linearity of the catalytic current increases from 0.08 to 6.52 mM.
2.1.2. Nitrite ion detection. Nitrite is an essential intermediate in the biological nitrogen cycle. The main source of nitrite in the water environment is the nitrogen fertilizer from agriculture.48 However, it has a bad effect on the human body (lethal dose 1.1 g), if it is present in drinking water and aquatic life as well.48,99 A comparison of electrochemical techniques for the detection of nitrite ions is shown in Table 2.
Table 2 A comparison of the electrochemical sensors for the detection of nitrate ions
Sensing material |
Detection range |
Detection limit |
Method of detection |
Detection medium |
Types of sample |
Ref. |
AuNPs/CS/MXene/GCE |
0.5–3300 μM |
0.069 μM |
Amperometry |
0.1 M PBS (pH 7) |
Water |
67 |
Cu@TiO2-Nf/PAR/GCE |
5–7500 μM |
2.1 μM |
DPV |
PBS (pH 1.7) |
Water |
68 |
Cu/SPE (Ag) |
0.05–5 mM |
0.21 nM |
LSV |
0.1 M KCl |
Water |
69 |
Cu nanowire electrode |
8–5860 μM |
1.35 μM |
DPV |
0.1 M Na2SO4 |
Tap water/river water |
50 |
Cu/MWCNT/RGO/GCE |
0.1–75 μM |
20 nM/30 nM |
SWV |
Na2SO4/H2SO4 |
Mineral/tap water |
51 |
Ppy-NW electrodes |
10 μM to 1 mM |
4.5 ± 1 μM |
Pulsed voltammograms |
N/A |
N/A |
70 |
AgNS on carbon and Ag UMEs |
4–1000 μM |
3.2–5.1 μM |
Chronoamperometry |
0.1 M Na2SO4 |
Synthetic aquifer |
52 |
Ag-doped zeolite expanded graphite epoxy electrode |
1–10 mM |
0.08 mM/0.004 mM |
Multiple pulsed amperometry |
0.1 M Na2SO4 |
N/A |
71 |
Cu electrode |
0.1–2.5 mM |
4.2 μM |
Amperometry |
0.1 M Na2SO4 |
Mineral water/soft drinks sample |
72 |
Chitosan/bentonite nanocomposite-based ISE |
20 mM to 0.8 M |
N/A |
Potentiometry |
N/A |
N/A |
73 |
Self-assembly nanobeads-packed (nBP) hetero columns ion-selective microelectrode |
0.1 μM to 0.1 M |
N/A |
Potentiometry |
N/A |
N/A |
74 |
Ppy-NS ISE |
0–200 mg L−1 |
N/A |
Potentiometry |
N/A |
N/A |
75 |
NiR/PEDOT NWA/AuE |
3.22–17.74 μM |
N/A |
Amperometry |
0.1 M KCl |
Water |
54 |
NiR/rGO/Ppy/GCE |
5 × 103–104 μM |
275 μM |
CV |
0.1 M KCl |
Freshwater |
56 |
NiR/CNTs/Ppy/GCE |
440–1450 μM |
170 μM |
Amperometry |
0.1 M PBS |
Freshwater |
55 |
NiR/Gr foam/Ti NF |
0.16–7128 μM |
0.16 μM |
Amperometry |
N/A |
Soil extract |
76 |
NiR/ZnO NRs/AgE |
1–3400 μM |
1 μM |
Amperometry |
N/A |
Freshwater |
77 |
NiR/GO/PEDOT NF/AuE |
7.09–7128 μM |
2.17 μM |
EIS |
N/A |
Soil extract |
53 |
Gr/CuE |
9–940 μM |
10 μM |
Amperometry |
0.1 M NaOH |
Freshwater |
78 |
Gr/Cu NPs/AuE |
10–90 μM |
7.89 μM |
DPV |
0.01 M HCl + 0.1 M Na2SO4 |
Freshwater |
57 |
CuNPs/MWCNT-PEI/PPy-PSS/GCE |
100–5000 μM |
30 μM |
Amperometry |
0.1 M PBS |
Freshwater |
79 |
CuOx/CNTs/GCE |
10–700 μM |
N/A |
DPV |
0.1 M Na2SO4 |
Freshwater |
80 |
Cu-modified CF MDE |
3–2000 μM |
1.1 μM |
SWV |
0.1 M KCl |
PM2.5 particle extraction in water |
59 |
Cu NCs/PtmE |
6.25–300, 300–3500 μM |
5 μM |
LSV |
N/A |
Freshwater |
60 |
CuNCs/PGE |
1–35 μM |
0.59 μM |
Amperometry |
0.01 M H2SO4+ 0.05 M K2SO4 |
Food extract/freshwater |
81 |
Cu NWA/TEPM |
10–400 μM |
3.0 μM |
LSV |
1 mM H2SO4 + 0.1 M K2SO4 |
Freshwater |
61 |
Cu NW/CuE |
8–5860 μM |
1.35 μM |
DPV |
Acidic (pH 2) |
Freshwater |
50 |
Cu NPs/PtE |
6.25–1000 μM |
N/A |
LSV |
0.1 M Na2SO4 |
Freshwater |
82 |
Macroporous Ag film/ITO |
20–5000 μM |
N/A |
SWV |
1 M NaOH |
Freshwater |
83 |
Ag networks like film/GCE |
80–6520 μM |
3.5 μM |
Amperometry |
0. M PBS |
Freshwater |
66 |
3D dendrite Ag NSt/Au mEA |
2–1000 μM |
2 μM |
SWV |
0.5 M NaCl |
Freshwater |
84 |
AgNPs/AuE |
0.39–50 |
0.39 |
SWV |
NaCl (34.5 g L−1) |
Seawater |
85 |
AgNPs/AuE |
1 × 10−3 to 0.01 μM |
9 × 10−4 |
CV |
NaCl (34.5 g L−1) |
Seawater |
86 |
Oxide-deficient Cu–Pt |
120–990 μM |
0.159 μM |
DPV |
0.1 M KCl |
Freshwater |
87 |
Pd NPs/epoxy-Cu |
32–560 μM |
N/A |
Amperometry |
0.1 M PBS |
Freshwater |
88 |
Pd–Sn composite/MEA |
16–322.6 μM |
3.06 μM |
LSV |
0.01 M KClO4 |
Freshwater |
63 |
Porous Cu–Ni alloy |
20–1000 μM |
2 μM |
Amperometry |
0.5 M K2SO4 |
Freshwater |
89 |
Pd–Au NPs composite |
16–242 μM |
1.19 μM |
LSV |
N/A |
Freshwater |
90 |
PEG-SH/SePs/Au NPs |
16–500 μM |
8.6 μM |
DPV |
0.1 M KCl |
Freshwater |
65 |
PNA-SA-modified SiO2@Fe3O4/CPE |
101–1453 μM |
87 μM |
SWV |
BR-buffer |
Freshwater |
91 |
CuxO-GCS/BPPGE |
10–100 μM |
1.032 μM |
CV |
1 mM HClO4 + 1 M NaClO4 |
Soil extract/food extract |
92 |
PPy/Ag NPs/GCE |
1–104 μM |
5 μM |
CV |
0.1 M K2SO4 |
Freshwater |
93 |
PPy/Pd NCs/GCE |
1 × 10−4 to 8 × 10−4 |
0.74 |
DPV |
0.1 M PBS |
Freshwater |
94 |
PANI/WO3/Cu Nsh/GCE |
40–246 μM |
1.2 μM |
LSV |
0.1 M H2SO4 |
Freshwater |
95 |
PANI/Cu NPs/GCE |
1–105 μM |
5 μM |
LSV |
0.1 M KCl |
Freshwater |
62 |
PPy/GCE |
0.001–0.006 mM |
N/A |
SWSV |
pH 9 |
Water |
96 |
PANI/Cu/MWCNT/Au electrode |
0.8–30 μM |
0.09 μM |
DPV |
0.1 M PBS (pH 5) |
Waste water |
97 |
Chit/ZnO/Pt |
0.1–2 μM |
0.01 μM |
CV |
0.1 M Na2SO4 (pH 5.5) |
Drinking water |
98 |
Stepwise modification of the glassy carbon electrode by chitosan (CS), polypyrrole (PPy), and carboxyl graph (CG) showed excellent differential pulse voltammetry (DPV) for the detection of nitrite ions in real water samples.100 The obtained electrochemical current showed a linear relationship with a wide range from 0.2 to 1000 μM with a detection limit of 0.02 μM. Moreover, the GCE modified with manganese porphyrin and niobium tungstate nano sheets showed an excellent detection limit of 0.380 μM.101
A micro-structuring system was developed to fabricate the working electrode. In this study, the reported linearity range was between 10-800 μM and the detection limit was 0.5 μM.103 In another study, ZnONRs (NR = Nano Rods) were grown on a seeded silver electrode and then dip-coated with Fe2O3 NPs. ZnONRs with Fe2O3 NPs significantly increase the electro-catalytic activity.104 The modified electrode showed a linear response from 1 to 1250 μM. Moreover, copper sulfide (CuS) nanoparticles and multi-walled carbon nanotube (MWCNT) nanocomposites were synthesized to prepare a GCE for the detection of nitrite ions. The composite showed excellent electro catalytic activity in the oxidation of nitrite ions in PBS (pH 7.0). The detection of nitrite ions showed linearity between 1 μM and 8.1 mM with a detection limit of 0.33 μM.102 The electrode modification process is shown in Fig. 3. In addition, Pt nanoparticles were loaded with Ni(OH)2/MWCNTs by a simple reduction process, which showed remarkable electro catalytic performance in nitrite oxidation.105 It has a sensitivity of 145 μA mM−1.
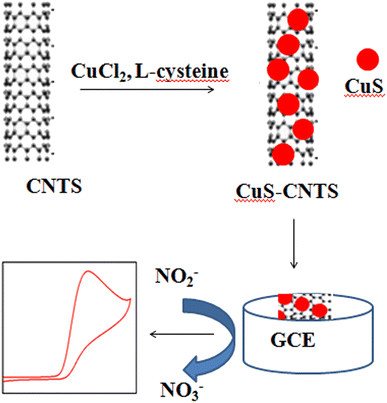 |
| Fig. 3 Schematic of the modification process for CuS-MWCNTs, this figure has been adapted from ref. 102 with permission from Elsevier, copyright 15 May 2016. | |
A novel electrochemical sensor was fabricated to determine the nitrite ion concentration on a hollo site modified with silver nano rods by a chemical process. Then, a MoS2 layer was prepared on an Ag/HNT nanocomposite by a hydrothermal process, and the catalytic activity of the finally modified carbon paste electrode was significantly enhanced. It provides good linearity from 2 to 425 μM.106 Moreover, in the research study, a GCE was fabricated using chitosan@N,S-coded MWCNTs, and gold nanoparticles (AuNPs) to obtain a nitrite sensor with a linear range of 1–5000 μM and a detection limit of 0.2 μM.107 In another study, a silver and graphene oxide-modified electrode was fabricated using ethanolamine and Ag nanoparticles. In this work, the detection limit and linear response range were reported to be 0.023 μM and 0.05 to 3000 μM, respectively.108 Wu et al. modified an electrode with Cu2O/CNTs to detect nitrite ions, with a linear concentration range from 0.1 nM to 1 mM.109 A schematic diagram of the electrode modification for nitrite ion detection is shown in Fig. 4. Huang et al. deposited gold nanoparticles on a poly(3-methylthiophene) (P3MT) modified glassy carbon electrode to form nano-Au/P3MT/GCE. Amperometric detection showed that the modified electrode exhibited excellent electrochemical responses for the detection of nitrite ions with a linear concentration range of 10–1000 μM.110 Moreover, the study on the detection of nitrite ions by amperometric detection in aqueous solution showed a satisfactory detection limit of 0.4 μM.111 Moreover, the glassy carbon electrode modified with gold nanoparticles showed excellent electro catalytic activity for the oxidation of nitrite ions. This gold-modified electrochemical sensor is comparatively better than the bare GCE and planar gold electrode, which showed a detection limit of 2.4 μM.112 Another study113 was carried out using a composite of 5-amino-1,3,4-thiadiazole-2-thiol (p-ATT) on functionalized multiwalled carbon nanotubes (FMWCNTs), and thus modified (FMWCNTs-ATT polymer (p-ATT)) glassy carbon electrode was used to detect ammonium ions amperometrically, and the oxidation peak was observed at 0.84 V. The peak of the modified electrode was larger than that of the bare electrode. This amperometric method showed a linear detection range of 10 to 1000 nM with a detection limit of 0.2 nM for the nitrite ion in the water medium.
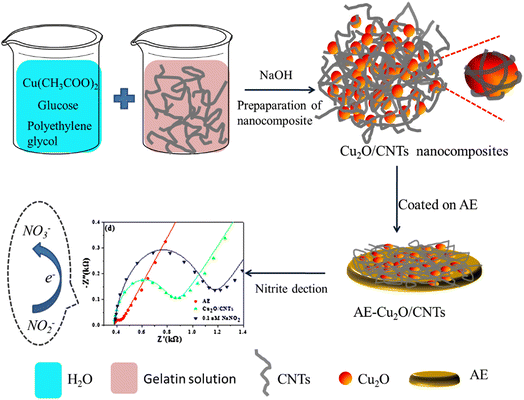 |
| Fig. 4 Schematic representation of the fabrication of Cu2O/CNTs composite-based sensor, (i) preparation of Cu2O/CNTs composite, (ii) Au electrode modification with Cu2O/CNTs composite and (iii) nitrite ion detection; this figure has been adapted from ref. 109 with permission from Elsevier, copyright November 2018. | |
For the simultaneous detection of nitrite ions with nitrate ions, Ag particle-doped zeolite with graphite epoxy electrode (AgZEGE) was used using multiple pulse amperometry (MPA). MPA improved the electrochemical response over other conventional methods.71 The studied electrochemical sensor was successfully used for the analysis of tap water samples spiked with nitrite ions and showed a linear response at 0.1–1 mM nitrite concentration. Another sensor for the simultaneous detection of superoxide anions and nitrite ions was fabricated stepwise on a Pt electrode. First, a CNT/PPy composite was deposited on a Pt electrode and then Cu and ZnSOD were deposited on it, which increased the electro catalytic response for the detection of nitrite ions.114 Micaela Badea modified the Pt electrode with a poly (1,8 diaminonapthelene) film for amperometric detection of nitrites in the water bath and flow injection analysis.115 This analysis was also applied to food, soil, vegetable, and fertilizer samples. Another research work on the determination of nitrite ions was carried out on the surface of a carbon electrode modified with cobalt-pathalocyanine.116 Also, a poly (pyrrole viologen) nitrite reductase biosensor modified with GCE was developed for the detection of nitrite ions. The linear range and detection limit of the modified electrode were 5.4–43.4 μM and 5.4 μM, respectively.117
In another research demonstration, the working electrode was modified with nano-diamond powder, which has catalytic activity for the oxidation of nitrite ions. Here, the electrochemical response increases with increasing nitrite ion concentration in the water medium.118 In addition, the detection of nitrite ions was also carried out by modifying the GCE electrode with Pt and Fe(III) nanoparticles.119 Abdollah Salimi, modified cobalt oxide (CoO) nanoparticles and flavin adenine dinucleotide (FAD) composite modified GCE using cyclic voltammetry, which showed excellent catalytic efficiency to reduce the over potential. In amperometric detection of nitrite ions, the modified electrode shows a linear dynamic range of 1–30 μM and a detection limit of 0.20 μM.120
2.1.3. Ammonium ion detection. In agricultural ecosystems, ammonium ions are important for plant growth when external inputs are low. In contrast, it causes toxicity when the amount is in the millimolar range. Root and shoot growth is affected due to the toxicity of ammonium ions. There are multiple conventional methods to determine ammonium ion concentration and electrochemical sensing research work is listed in Table 3.
Table 3 Comparison of the electrochemical sensors developed for the detection of nitrite ions
Sensing material |
Detection range |
Detection limit |
Method of detection |
Detection medium |
Types of sample |
Ref. |
Poly(1,8-DAN)/f-MWCNT |
0.3–6.5 μM |
0.075 μM |
Amperometry |
0.1 M PBS + 0.1 M KCl |
N/A |
121 |
GO-CS-AuNPs/GCE |
0.9–18.9 μM |
0.3 μM |
Amperometry |
0.1 M PBS |
N/A |
122 |
Cu/MWCNT/RGO/GCE |
0.1–75 μM |
N/A |
SWV |
Na2SO4/H2SO4, pH 3 |
Water |
51 |
MnTMPyP/NbWO6/GCE |
0.12–3.57 mM |
0.38 μM |
DPV |
PBS (pH 7) |
N/A |
101 |
CG/PPy/CS/GCE |
0.2–1000 μM |
0.02 μM |
DPV |
0.1 M NaAc-HAc buffer (pH 4) |
Tap/commercial/salt/soybean milk water |
100 |
α-MnO2- based electrode |
10–800 μM |
0.5 μM |
DPV |
0.1 M PBS |
N/A |
123 |
GNPs/graphene/MCE electrode |
0.3–720 μM |
0.1 μM |
DPV |
0.1 M acetate buffer |
Lake/river/industrial water/food |
124 |
α-Fe2O3 NPs-ZnO NRs-Ag electrode |
1–1250 μM |
0.015 μM |
LSV |
0.1 M PBS |
Tap/mineral/pond water |
104 |
Cu-MOF/rGO/GCE |
3 μM to 40 mM |
33 nM |
Chronoamperometry |
0.1 M PBS |
N/A |
125 |
CuS-MWCNT/GCE |
1 μM to 8.1 mM |
0.33 μM |
Amperometry |
0.1 M PBS |
Tap water |
102 |
Pt/Ni(OH)2/MWCNTs/GCE |
0.4 μM to 5.67 mM |
0.13 μM |
Amperometry |
0.1 M PBS |
Milk sample |
126 |
Pt NWNs/GCE |
1 μM to 24 mM/24–132 mM |
0.14 μM |
Chronoamperometry |
0.1 M PBS |
N/A |
127 |
Ag/HNTs/MoS2/CPE |
2–425 μM |
0.7 μM |
Amperometry |
0.1 M PBS |
Tap water/aqueduct water |
106 |
AuNPs/CS@N,S co-doped MWCNTs/GCE |
1–7000 μM |
0.2 μM |
Amperometry |
M PBS (pH 7.4) |
Food |
107 |
Pd/Fe3O4/poly DOPA/RGO |
2.5–6470 μM |
0.5 μM |
Amperometry |
0.1 M PBS |
River water/food |
128 |
Ag-AEfG100/GCE |
0.05–3000 μM |
0.023 μM |
Amperometry |
PBS (pH 7.4) |
Tap water |
108 |
Cu2O-CNTs/Au electrode |
0.1 nM to 1 mM |
0.0188 nM |
EIS |
5 mM K3[Fe(CN)6]/K4[Fe(CN)6} in PBS (pH 7.4) |
Tap water |
109 |
Nano-Au/P3MT/GCE |
10–1000 μM |
2.3 μM |
Amperometry |
PBS (pH 4.0) |
Iodized salt |
110 |
Bare GCE |
N/A |
0.4 μM |
Amperometry |
N/A |
Aqueous |
111 |
Au/GCE |
10–5000 μM |
2.4 μM |
CV |
0.2 M acetate buffer |
Water |
112 |
Cyt c/l-Cys/P3MT/MWCNT/GCE |
10–100 μM |
0.5 μM |
Amperometry |
0.1 M PBS |
Aqueous |
129 |
P-NiTAPc film-modified electrode |
0.5–8000 μM |
0.1 μM |
Amperometry |
H2SO4–Na2SO4 solution (pH 2.0) |
Water |
130 |
Ag-NEEs |
10–300 μM |
N/A |
CV |
0.1 M PBS |
N/A |
131 |
MC/GCE |
0.5–100 μM |
0.1 μM |
DPV |
BR-buffer (pH 3.5) |
Water |
132 |
Boron-doped diamond electrode |
2–1000 μM |
0.05 μM |
CV |
N/A |
Aqueous |
133 |
p-ATT/GCE |
0.05–100 |
340 pM |
Amperometry |
0.2 M PBS |
Water |
134 |
VO(SB)-modified CPE |
3.9–40 μM |
0.613 μM |
CV |
0.1 M TBAP |
N/A |
135 |
AgZEGE |
100–1000 μM |
10 μM |
Amperometry, CV |
0.1 M Na2SO4 |
Water |
71 |
Pt/poly(1,8-DAN)Pt/CA |
0.5–100 μM |
0.1 μM |
Amperometry |
Acetate buffer (pH 4) |
Water/food/soil |
115 |
CoPc modified electrode |
N/A |
0.005 μA |
CV |
PBS (pH 7.3) |
N/A |
116 |
CuNPs/CNTs/CS/GCE |
0.1–2500 μM |
0.024 μM |
Amperometry |
0.05 M PBS |
N/A |
136 |
CoL/MNSs/CPE |
0.2–30 μM |
0.015 μM |
DPV |
BR-buffer |
Water |
137 |
Cu/Ag/MWCNTs/GCE |
1 μM to 1 mM |
0.2 μM |
Amperometry |
PBS (pH 7) |
Lake/drinking/sea water |
138 |
AuNPs-PEI/CSPE |
0.01–4 μM |
0.0025 μM |
DPV |
PBS (pH 6.5) |
Tap/cannel water |
139 |
Snsubmicroparticles/GCE |
5–1000 μM |
0.5 μM |
DPV |
0.1 M PBS |
N/A |
140 |
Snsubmicroparticles/GCE |
150–900 μM |
10 μM |
Amperometry |
0.1 M PBS |
N/A |
140 |
Snsubmicroparticles/GCE |
100–500 μM |
50 μM |
Impedance |
0.1 M PBS |
N/A |
140 |
For the groundwater used as drinking water, ammonium ion concentration was measured with a new silver-decorated carbon nanotube-epoxy composite using the differential pulse voltammetry technique.141 The newly modified electrode has very good sensitivity and excellent electroactivity for the direct oxidation of nitrite ions. Another technique for the detection of ammonium ions was developed on a lab-on-a-chip (LOC), which works for in situ and real samples by potentiometric measurements. The fabricated device consists of poly(dimethylsiloxane) and an integrated sensor platform that includes four working microelectrodes, two reference microelectrodes, and one counter microelectrode.142 It can measure fast and reliable data in real time when immersed in a laminar flow of ambient water. In addition, an ion-selective electrode (ISE) with solid contact was developed by modifying it with graphite paste, polypyrrole, and ionophore-immobilized polyvinyl chloride materials.143 In this process, the ISEs possess a short reaction time. In addition, a wireless real-time ion-selective solid-state membrane was developed for the detection of ammonium ions in wastewater.144 It saves 25% of power under normal operating conditions. In another research work, a zeolite-based conductometric micro sensor was successfully used for the detection of ammonium ions in real media.145 The modified electrode exhibited high operational and storage stability and has a detection limit of 100 μM. Saiapina et al. reported a conductometric sensor based on 25,27-di-(5-thio-octyloxy) calyx [4]aren-crown-6 for the measurement of monovalent, divalent, and trivalent cations. The developed sensor has satisfactory sensitivity and high signal repeatability, as well as a linear range of 0.01 to 1.5 mM and a detection limit of 10 μM.146 The carbon fabric electrode was modified by electrodeposition of a PtCu alloy, which reduced the crystal lattice spacing of Pt to oxidize ammonium ions. The first developed electrode modified with PtCu alloy showed a linear response of 0.5–250 μM and a detection limit of 8.6 nM.147 The schematic is shown in Fig. 4.
Electrodeposition and electro polymerization techniques were used to prepare the SPEC/AuNPs/PMB electrode, and then a thin layer of glutamate dehydrogenase was applied to prepare an enzymatic membrane.148 In the study, the ammonium ion concentration was indirectly measured in the ammonium solution containing nicotinamide adenine di-nucleotide hydrogen phosphate (NADH). Another research work was carried out in the medium of water/1,6-dichlorohexane with amperometric detection.149 Here, the detection limit was 0.12 μM and the linear range was between 4.2 and 66 μM. Moreover, a group of chemicals was added to the NH4+ ion medium, namely alanine dehydrogenase enzyme, pyruvate substrate, and nicotinamide adenine di-nucleotide (NADH).150 Finally, the oxidation of NADH was carried out at 0.55 V, which is proportional to the ammonium ion concentration in the river water samples (Table 4 and Fig. 5).
Table 4 Comparison of the electrochemical sensors for the detection of ammonium ions
Sensing material |
Detection range |
Detection limit |
Method of detection |
Detection medium |
Types of sample |
Ref. |
PMB@GLDH/AuNPs/SPEC |
0.65–300 μM |
N/A |
Amperometry |
PBS (pH 9.5) |
Water |
148 |
Ag-CNT |
0.2–1 mM |
1 μM |
DPV |
0.1 M Na2SO4 |
Groundwater |
141 |
Ppy COSANE-Au microelectrode |
1 μM to 40 mM |
0.04 mM |
Potentiometric |
Buffer Tris-HCl/Tris |
Tap/sewage water |
142 |
NH4+ selective ASS electrode |
1 μM to 0.1 M |
<1 μM |
Potentiometric |
N/A |
Tap/well water |
143 |
Solid state ISM |
1–64 mg N/L |
1 × 10−6.5 M |
Potentiometric |
N/A |
Wastewater |
144 |
Natural zeolite clinoptilolite-Au interdigitated microelectrode (IDμE) |
0.01 μM to 1 mM |
0.01 μM |
Conductometric |
5 mM KH2PO4/Na2HPO4 (pH 6.2) |
N/A |
145 |
25,27-Di-(5-thio-octyloxy)calix[4] arene-crown-6-Au interdigitated electrode (IDE) |
0.01–1 mM |
0.01 mM |
Conductometric |
5 mM KH2PO4/Na2HPO4 (pH 6.2) |
River water |
146 |
Alanine dehydrogenase/SPE |
10–100 mM |
0.18 mM |
Amperometric |
PBS (pH 7) |
N/A |
150 |
PET microporous membrane |
4.2–66 μM |
0.12 μM |
CV, DPV, SWV |
Water/1,6-dichlorohexane |
Water |
149 |
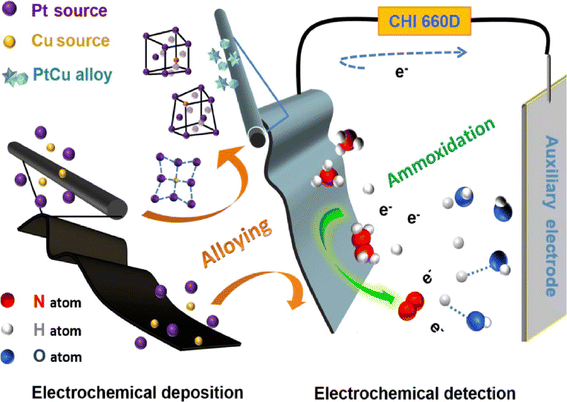 |
| Fig. 5 Schematic representation for the preparation of Pt7Cu1-CC electrode and its application for ammonia detection; this figure has been adapted from ref. 147 with permission from Elsevier, copyright 1 October 2022. | |
3. Potassium (K) detection
As a macronutrient, potassium (K) is regularly required by plants during their life cycle for the movement of water, nutrients and carbohydrates. It is related to all kinds of metabolic activities of plant growth and fruiting. Therefore, the role of potassium is essential for efficient plant production.
The detection of potassium ions is very important due to its significance in agriculture and proper fertilization of the cropland. A versatile electrochemical detection of potassium was performed using various modified electrode surfaces, which are summarized in Table 5. There are several types of potassium ion receptor compounds and probes whose main performance is also listed.
Table 5 Comparison of the electrochemical sensors for the detection of potassium ions
Sensing material |
Detection range |
LOD |
Method of detection |
Detection medium |
Types of sample |
Ref. |
AuE/11-MUA/4-AB-18-C-6 |
1 μM to 10 mM |
N/A |
DPV |
0.1 TBAB |
N/A |
151 |
GCE/GO-crown |
0.1–10−7 μM |
1 pM |
EIS |
1.5 mM MB |
N/A |
152 |
AuE/G-quadruplex/hemin |
0.1 nM to 0.1 μM |
0.1 nM |
DPV |
100 mM Tris–HCl buffer (pH 6.2) |
N/A |
153 |
Al–Cu/Si-p/SiO2/Si3N4/PVC-membrane |
1 μM to 10−1 M |
N/A |
EIS |
0.1 M Tris–HCl buffer (pH 7) |
N/A |
154 |
AuE/K+ ssDNA |
10−8 to 10−5 M |
2.31 × 10−9 M |
CV |
MB, AQMS, Na4Fe(CN)6 |
N/A |
153 |
AuE/p-ATP/AuNPs/DNA |
0.1–1 mM |
0.1 mM |
SWV |
0.1 M Tris–HCl buffer (pH 7.41) |
N/A |
157 |
1–30 mM |
GCE/P(Py-co-PAA)/G-aptamer |
20 fM to 1 mM |
14.7 fM |
EIS |
20 mM Tris–HCl buffer (pH 7.4) |
N/A |
158 |
SPC/Nafion/4-AB-18-C-6 |
1–500 ppm |
N/A |
DPV |
3 mMRu(NH3)6+/0.1 TBAB |
N/A |
159 |
SCE/CPE/hollandite-MnO2 |
4.97 × 10−5 to 9.05 × 10−4 M |
N/A |
Amperometry |
Tris–buffer (pH 8) |
N/A |
160 |
GCE/MnO2 nanorods |
2–90 μM |
0.05 μM |
CV |
Tris–buffer (pH 7.4) |
Tap and DI water |
161 |
CPEM/KSr2Nb2O15 |
1.26 × 10−5 to 1.62 × 10−3 M |
7.27 × 10–5 M |
Amperometry |
0.1 M Tris–buffer (pH 8.3) |
N/A |
168 |
AuE/ssDNS-G rich |
0.1 nM to 50 nM |
0.1 nM |
SWV |
20 mM Tris–HCl buffer (pH 7.41) |
Human serum |
164 |
Carbon SPE/PANI/valinomycin |
10−5 to 1 M |
10−5.8 M |
Potentiometric |
N/A |
Artificial serum |
75 |
Al/F-MWCNTs/ZnO/valinomycin |
5–25 mM |
N/A |
EIS |
N/A |
Soil |
166 |
PET/graphene- valinomycuin |
1–20 000 μM |
N/A |
FET |
Tris–HCl buffer |
N/A |
169 |
PET/Gel/valinomycin |
5–100 μM |
N/A |
N/A |
N/A |
Serum |
170 |
3DPE sensors |
1–10 000 ppm |
1.35 ppm |
CV |
pH 1.44 |
Soil pore water samples |
167 |
Valinomycin supported ZnO/rGO nanocomposites on GCE |
— |
0.956 mM |
CV |
N/A |
Soil sample |
171 |
F-MWCNT/ZnO/valinomycin composites |
5–25 mM |
— |
Impedance |
N/A |
Soil sample |
172 |
Prussian blue nanotube sensor |
5.0 × 10−8 to 7.0 × 10−4 M |
2.0 × 10−8 M |
CV |
N/A |
Water |
173 |
4-Aminobenzo-18-crown-6-ether (crown ether) and the K+ cation can form a complex on the electrochemical surface, which produces an electrochemically measurable signal. This type of work was reported to detect K+ ions by the DPV technique in the 0.1 M tetra-butyl ammonium bromide (TBAB) medium.151 In this study, the detection range is 1 μM to 10 mM. Another research work was conducted on ether-modified graphene surfaces in the methylene blue (MB) medium.152 In addition, K+ ions form G-quadruplexes with aptamer-modified electrodes. Here, hemin also helps to form this complex on the modified surface.153 In addition, a dibromaza[7]helicene ionophore was developed and doped with polyvinyl chloride (PVC) on the surface of an Al–Cu/Si-p/SiO2/Si3N4 transducer. The modified electrode also showed a linear response of 1 μM to 10−1 M and also has excellent sensitivity in other cationic media.154 A gold electrode modified with DNA aptamers was also developed for the quantification of K+ ions in MB, AQMS, and Na4Fe(CN)6 medium. In this work, a linear response of 10−8 to 10−5 M and a detection limit of 2.31 × 10−9 M were observed.155 The aptamers-based biosensor is shown in Fig. 6.
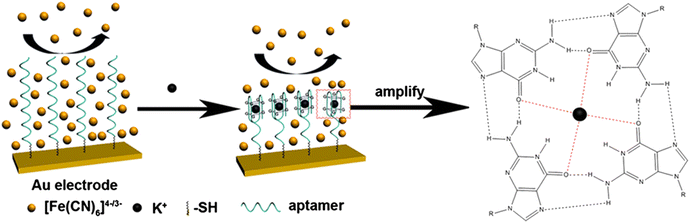 |
| Fig. 6 ssDNA and K+ reaction mechanism on the modified electrode surface, this figure has been adapted from ref. 156 with permission from Elsevier, copyright 15 October 2013. | |
Another research was conducted using AuNPs and G-rich ssDNA-modified electrodes to detect potassium ions in the linear range of 0.1–1 mM and 1–30 mM.157 To improve the conductivity of the electrode modified with G-rich ssDNA, a poly-pyrrole modification was also used as an anchor for the probe and transducer.158 In addition, a carbon-based sensor was modified with crown ether and Nafion to detect potassium ions using the DPV technique.159
Lima et al. demonstrated a research work on the modification of working electrodes using hollandite-type manganese oxide to detect potassium ions. In this study, K+ ion detection was performed by amperometric technique in Tris buffer pH 8.160 In addition, zinc oxide nanotubes (ZnONRs) were synthesized by a hydrothermal sol–gel method to produce GCE. The synthesized GCEs modified with ZnONRs were used to detect potassium ions in drinking water samples.161 Furthermore, a single-layer ssDNA electrode modified with graphene and guanine was used to detect K+ ions, which is suitable for health monitoring and other applications.162 In addition, the ssDNA probe was attached to AuE by thiol binding and the guanine-rich part captured the K+ ions. The K+ ion detection is included in this post128,163 the electrode modification process and K+ detection are shown in Fig. 6.
Valinomycin surface coating with an active layer containing the modified electrode for detection of K+ ions on the polyaniline layer of GCE has been reported.165 In this study, they reported, a detection range of 10−5 to 1 M with a detection limit of 10−5.8 M. Another research group reported an F-MWCNTs/ZnO/valinomycin electrode surface for the detection of potassium ions in soil extracts. This showed a linear detection range of 5–25 mM.166 In a recent report, a low-cost on-site detection method but accurate method has been proposed by Denis McCrudden and his group167 for monitoring soil nutrients. This sensing system consists of a microcontroller with novel 3D printed ion selective electrodes, which can be used for the simultaneous determination of potassium and pH. This electrode showed linearity for potassium ions in the range of 1–10
000 ppm electrodes with LOD of 1.10 ppm and 1.35 ppm at pH values of 1.44 and 3.05, respectively (details in Table 5).
4. Phosphorus (P) ion detection
As we have already discussed, nitrogen, phosphorus and potassium, commonly known as NPK, are the main macronutrients of soil and each of these nutrients has its own role in plant nutrition.174,175 In this part, we will discuss the existing sensors for the detection of phosphorus, as it is a component of nucleic acids and membrane lipids in plant formation. Including phosphorus, all three mentioned nutrients should be available in the soil. On the other hand, soil erosion and agricultural fertilization may increase toxic substances in water. Phosphate (PO43−) can cause eutrophication of water bodies due to its excessive presence.176 Not only the vital biomolecules of our human body, but also the growth of plants is highly dependent on the presence of an adequate amount of these phosphate ions in the soil, and therefore it plays a crucial role in both the agricultural sector and biomedical applications.
Since most farmers apply fertilizers blindly into the soil and it is necessary to reduce the negative impact of the fertilizer on the environment without reducing the crop yield, therefore, the proper management of this fertilizer is very important. Maria Khaydukova and her group have developed a potentiometric multisensory system for the simultaneous detection of N, P, and K in the aqueous soil extracts of the soils of the desired fields.177 The sensors they developed can be used to analyze pH and conductivity as well as all three macronutrients in soil samples, and good correlation coefficients of 0.69 to 0.96 were obtained between the target parameters and sensor responses. Quantification of P was possible with a root mean square error (RMSE) of 79 mg kg−1 in the range of 35–574 mg kg−1.
Talarico et al.178 reported a screen-printed electrode modified with carbon black nanoparticles (CB) using an amperometric method via electrochemical reduction of a molybdophosphate complex. Here, the CB nanoparticles help quantify the molybdophosphate complex at a lower applied potential. This CB-SPE shows a linear range of 0.5–100 μM with a detection limit of 0.1 μM. The system was tested in various water samples such as drinking, river, aquarium and wastewater and showed satisfactory recoveries.
Kabir and his group reported a novel screen-printed electrode (SPE) modified with ammonium molybdate tetrahydrate (AMT) and silver nanowires (AgNWs) for phosphate detection.179 Since AgNWs are highly conductive, they were added to AMT, which contributes to faster electron transport between AMT and SPE. As a result, the sensor they developed showed a wide detection range of 5 μM to 1 mM and LOD of 3 μM with a high sensitivity of 0.71 μA μM−1 and they suggested that this AMT/AgNWs/SPE is promising for simple, low-cost, portable phosphate ion detection and for monitoring the health of water systems and field soils.
Catalytic hydrogen waves (CHW) occur due to a classical reaction in which certain substances in solution catalyze the hydrogen evolution reaction and thus can reduce the overpotential for the hydrogen ion reduction,180 are based on this idea, and a novel sensor was developed by Zhang et al.181 In this method, a wave corresponding to catalytic hydrogen evolution was observed on the electrode surface modified with molybdenum phosphide (MoP) in the presence of phosphate. The interaction between molybdenum oxides on the surface of MoP microparticles and phosphate results in a structure (change in catalytic properties) similar to phosphomolybdic acid (MoPO), allowing quantitative detection of phosphate in the human blood. Differential pulse voltammetry (DPV) shows a linear range from 0.10 to 20.0 mmol L−1 with LOD of 0.030 mmol L−1.
Since natural enzymes lead to denaturation under environmental conditions, they are sometimes not used in measuring instruments. Recently, free-standing cobalt oxide in the form of nano-needle arrays on flexible carbon fabric (CC) serving as a substrate has been developed to detect phosphate ions in human urine,182 and according to their reports, it is inexpensive and shows scalability, good stability, and high sensitivity. The sensors they developed show a wide linear range of 0.1–1.0 mM and 1.0–30.0 mM with a detection limit of about 10 μM for the phosphate anion.
It is well known that the use of large amounts of chemical fertilizers causes contamination of agricultural wastewater, although they are treated like macronutrients NPK in soil. To determine their presence in watercourses, Jagannathan and co-workers183 developed a method based on electrochemical impedance spectroscopy measurements involving the detection of urea (N), orthophosphate (PO43−) (P), and potassium K+ (K) in agricultural watercourses, using stainless steel as working and counter electrodes and Ag/AgCl as a reference (details in Table 6).
Table 6 Comparison of electrochemical sensors for the detection of phosphate ionsa
Electrode material |
Method |
LOD |
Range |
Sample |
Ref. |
Pyruvate oxidase (PyO), poly-5,2′:5′,2′′-terthiophene-3′-carboxylic acid, glassy carbon electrode (GCE), poly-TTCA (nano-CP), carbon nanotube-CNT, cellulose nano crystal – CNC, polyaniline – pANI, ammonium molybdenum tetrahydrate – AMT, molybdenum phosphide (MoP). |
PyO/nano-CP/GCE |
Amperometric |
0.3 μM |
1.0–100 μM |
Human serum |
184 |
GLA/PyO/CoPC-SPCE |
Amperometric |
2 μM |
2.5–130 μM |
Pond water |
185 |
Rotating gold disk electrode |
Amperometric |
0.11 μM |
0.59–3.49 μM |
Seawater |
186 |
CB-SPE |
Amperometric |
0.1 μM |
0.5–100 μM |
Water samples |
178 |
Screen-printed graphite macroelectrodes |
Cyclic voltammetry |
0.3 μg L−1 |
0.5 to 20 μg L−1 |
Canal water |
187 |
AMT/AgNWs/SPE |
Cyclic voltammetry |
3 μM |
5 μM to 1 mM |
Field soil |
179 |
MoP/GCE |
DPV |
0.030 mmol L−1 |
0.10 to 20.0 mmol L−1 |
Human blood |
181 |
Co3O4/CC |
Amperometric |
10.0 μM |
0.1–30.0 mM |
Human urine |
182 |
Stainless steel |
Electrochemical impedance spectroscopy |
— |
7 to 50 ppm |
Simulated agricultural run of water |
183 |
CNT/CNC/Ag@ pANI@AMT microneedle |
Voltammetry |
0.007 mM |
0–0.6 mM |
Standard sample and coffee |
188 |
GQDs-Ce4+ probe |
Fluorescent probe |
0.06 μM |
0.1 to 20.0 μM |
Sodium phosphate |
176 |
Although electrochemical sensors might be a good replacement for the conventional existing technique, still there are a few problems, such as sample preparation, and interference of different kinds of ions present in the soil sample solution. We need to focus on these issues. Different kinds of ionophores have already been reported for the selective determination of ion-like, 4-AB-18-C-6 ether that can bind potassium ions in its structure. We need to search more ionophores for different kinds of soil constituents so that we perform an analysis of soil or water samples accurately. When we are able to analyze the soil/water samples accurately, it will be easier for any farmer to measure how much fertilizer is required by the plant at that time, even which types of fertilizer should be applied might be estimated as they are able to send their data to the local agricultural specialist. It is assumed that, may be initially it will be difficult but gradually it will bring revolution when more farmers in an area are brought under these facilities.
5. Conclusions
The conventional techniques are well-established methods and by using them it is possible to measure specific ions with high accuracy, while the electrochemical sensor is less expensive, easy to use, can perform measurements in a short time, sometimes not requiring much more pretreatment of the samples, and has a low detection limit and accuracy also comparable to the conventional technique. Although all of the work is very impressive, considering linearity regarding the concentration of different analytes, such as for nitrate ions, Cu/MWCNT/RGO/GCE (20 nM-30 nM)51 and NiR/Gr foam/TiNF (0.16–7128 μM),76 for nitrite ions, CG/PPy/CS/GCE (0.2–1000 μM)100 and Cu2O-CNTs/Au electrode (0.1 nM to 1 mM),109 for ammonium ions, PMB@GLDH/AuNPs/SPEC (0.65–300 μM)148 and natural zeolite clinoptilolite-Au interdigitated microelectrode (IDμE) (0.01 μM to 1 mM),145 for potassium ions, GCE/P(Py-co-PAA)/G-aptamer (20 fM to 1 mM),158 AuE/ssDNS-G rich (0.1 nM to 50 nM),164 and AuE/G-quadruplex/Hemin,153 for phosphate analysis, GQDs-Ce4+ probe (0.1 to 20.0 μM)176 and CB-SPE (0.5–100 μM)178 are very impressive works. However, there are still many opportunities, such as the development of single short measurements, that is, a single measurement will measure different soil/water nutrients with high accuracy, and the development of smartphone-based apps (including artificial intelligence) is in high demand for real-time monitoring of agricultural systems that suggest fertilizer management to farmers. Finally, it will be easier for farmers to optimize production costs and minimize fertilizer use, which will help control pollution. If we assume that a large number of people will use this kind of smartphone-based app, it will be easier to support them (as they will be able to send their soil reports to the specialist) from a local agricultural research center or from any other agricultural specialist; thus, electrochemical sensors might be able to boost smart agriculture and bring a revolution.
Conflicts of interest
The authors declare that they have no known competing interests or personal relationships that could have appeared to influence the work reported in this paper.
Acknowledgements
This work was performed with the financial support from the Ministry of Information and Communication Technology, Government of Bangladesh (Innovation Fund).
References
- M. R. Al-Mamun, M. R. Hasan, M. S. Ahommed, M. S. Bacchu, M. R. Ali and M. Z. H. Khan, Nanofertilizers towards sustainable agriculture and environment, Environ. Technol. Innovation, 2021, 23, 101658, DOI:10.1016/j.eti.2021.101658.
- R. P. Potdar, M. M. Shirolkar, A. J. Verma, P. S. More and A. Kulkarni, Determination of soil nutrients (NPK) using optical methods: a mini review, J. Plant Nutr., 2021, 44(12), 1826–1839, DOI:10.1080/01904167.2021.1884702.
- C. Hafsi, A. Debez and C. Abdelly, Potassium deficiency in plants: effects and signaling cascades, Acta Physiol. Plant., 2014, 36(5), 1055–1070, DOI:10.1007/s11738-014-1491-2.
- D. Zhao, K. R. Reddy, V. G. Kakani and V. R. Reddy, Nitrogen deficiency effects on plant growth, leaf photosynthesis, and hyperspectral reflectance properties of sorghum, Eur. J. Agron., 2005, 22(4), 391–403, DOI:10.1016/j.eja.2004.06.005.
- S. J. Leghari, N. A. Wahocho and G. M. Laghari, et al., Role of nitrogen for plant growth and development: a review, Adv. Environ. Biol., 2016, 10, 209+ CAS . https://link.gale.com/apps/doc/A472372583/AONE?u=anon%7E70bdc11%26sid=googleScholar%26xid=0cbaab12.
- L. Liu, X. Zheng, X. Wei, Z. Kai and Y. Xu, Excessive application of chemical fertilizer and organophosphorus pesticides induced total phosphorus loss from planting causing surface water eutrophication, Sci. Rep., 2021, 11(1), 1–8, DOI:10.1038/s41598-021-02521-7.
- A. G. Good and P. H. Beatty, Fertilizing nature: a tragedy of excess in the commons, PLoS Biol., 2011, 9(8), 1–9, DOI:10.1371/journal.pbio.1001124.
- Z. Zhang, D. Liu, M. Wu, Y. Xia, F. Zhang and X. Fan, Long-term straw returning improve soil K balance and potassium supplying ability under rice and wheat cultivation, Sci. Rep., 2021, 11(1), 1–15, DOI:10.1038/s41598-021-01594-8.
- W. Hua, P. Luo and N. An, et al., Manure application increased crop yields by promoting nitrogen use efficiency in the soils of 40-year soybean-maize rotation, Sci. Rep., 2020, 10(1), 1–10, DOI:10.1038/s41598-020-71932-9.
- S. G. Lemos, A. R. A. Nogueira, A. Torre-Neto, A. Parra, J. Artigas and J. Alonso, In-soil potassium sensor system, J. Agric. Food Chem., 2004, 52(19), 5810–5815, DOI:10.1021/jf0492924.
- M. A. Ali, L. Dong, J. Dhau, A. Khosla and A. Kaushik, Perspective—Electrochemical Sensors for Soil Quality Assessment, J. Electrochem. Soc., 2020, 167(3), 037550, DOI:10.1149/1945-7111/ab69fe.
- Y. Li, H. Huang and R. Cui, et al., Electrochemical sensor based on graphdiyne is effectively used to determine Cd2+ and Pb2+ in water, Sens. Actuators, B, 2021, 332, 129519, DOI:10.1016/j.snb.2021.129519.
- M. R. Ali, M. S. Bacchu, M. R. Al-Mamun, M. S. Ahommed, M. A. Saad Aly and M. Z. H. Khan, N -Hydroxysuccinimide crosslinked graphene oxide-gold nanoflower modified SPE electrode for sensitive detection of chloramphenicol antibiotic, RSC Adv., 2021, 11(26), 15565–15572, 10.1039/d1ra02450g.
- W. Slavin, Atomic-Absorption Spectroscopy—A Critical Review, Appl. Spectrosc., 1966, 20(5), 281–288, DOI:10.1366/000370266774385787.
- S. L. C. Ferreira, M. A. Bezerra and A. S. Santos, et al., Atomic absorption spectrometry – A multi element technique, TrAC, Trends Anal. Chem., 2018, 100, 1–6, DOI:10.1016/j.trac.2017.12.012.
- D. A. Skoog, D. M. West, F. J. Holler, S. R. Crouch, Fundamentos de Química Analítica, Cengage Learning, 2014 Search PubMed, https://books.google.com.bd/books?id=q2CfzgEACAAJ.
- N. M. S. Vasconcelos, Fundamentos de Química Analítica Quantitativa, 2019, pp. 1–196 Search PubMed.
- D. Wu, Y. Hu, H. Cheng and X. Ye, Detection Techniques for Lead Ions in Water: A Review, Molecules, 2023, 28(8), 3601, DOI:10.3390/molecules28083601.
- S. S. Nielsen, Sodium and potassium determinations by atomic absorption spectroscopy and inductively coupled plasma-atomic emission spectroscopy, Food Analysis Laboratory Manual, 2003, pp. 67–73, DOI:10.1007/978-1-4757-5250-2_10.
- S. Paudel, S. Kumar and A. Mallik, Atomic Absorption Spectroscopy: a Short Review, EPRA Int. J. Res. Dev., 2021, 6(9), 322–327, DOI:10.36713/epra2016.
- A. F. Lagalante, Atomic absorption spectroscopy: a tutorial review, Appl. Spectrosc. Rev., 2004, 34(3), 173–189, DOI:10.1081/asr-100100844.
- A. Farahani, C. Tashakori and A. Shadman, Rapid determination of nitrate ions in drinking water based on image processing techniques using a smartphone platform, Ssrn, 2023, 20, DOI:10.2139/ssrn.4459063.
- M. Karlsson, B. Karlberg and R. J. O. Olsson, Determination of nitrate in municipal waste water by UV spectroscopy, Anal. Chim. Acta, 1995, 312(1), 107–113, DOI:10.1016/0003-2670(95)00179-4.
- A. C. Edwards, P. S. Hooda and Y. Cook, Determination of Nitrate in Water Containing Dissolved Organic Carbon by Ultraviolet Spectroscopy, Int. J. Environ. Anal. Chem., 2001, 80(1), 49–59, DOI:10.1080/03067310108044385.
- F. E. Adelowo and S. O. Agele, Spectrophotometric analysis of phosphate concentration in agricultural soil samples and water samples using molybdenum blue method, Braz. J. Biol. Sci., 2016, 3(6), 407–412, DOI:10.21472/bjbs.030616.
- S. Ganesh, F. Khan, M. K. Ahmed, P. Velavendan, N. K. Pandey and U. Kamachi Mudali, Spectrophotometric determination of trace amounts of phosphate in water and soil, Water Sci. Technol., 2012, 66(12), 2653–2658, DOI:10.2166/wst.2012.468.
- M. A. Tabatabai and W. A. Dick, Simultaneous Determination of Nitrate, Chloride, Sulfate, and Phosphate in Natural Waters by Ion Chromatography, J. Environ. Qual., 1983, 12(2), 209–213, DOI:10.2134/jeq1983.00472425001200020012x.
- N. T. Basta and M. A. Tabatabai, Determination of Potassium, Sodium, Calcium, and Magnesium in Natural Waters by Ion Chromatography, J. Environ. Qual., 1985, 14(3), 450–455, DOI:10.2134/jeq1985.00472425001400030028x.
- N. Lohumi, S. Gosain, A. Jain, V. K. Gupta and K. K. Verma, Determination of nitrate in environmental water samples by conversion into nitrophenols and solid phase extraction−spectrophotometry, liquid chromatography or gas chromatography–mass spectrometry, Anal. Chim. Acta, 2004, 505(2), 231–237, DOI:10.1016/j.aca.2003.10.060.
- E. Fries and J. Klasmeier, Analysis of potassium formate in airport storm water runoff by headspace solid-phase microextraction and gas chromatography–mass spectrometry, J. Chromatogr. A, 2009, 1216(5), 879–881, DOI:10.1016/j.chroma.2008.11.052.
- Y. Zheng, W. Fu, R. Zhu, Z. Hu, G. Chen and X. S. Chai, Determination of total phosphorus in soil and sludge by an effective headspace gas chromatographic method, RSC Adv., 2019, 9(70), 40961–40965, 10.1039/c9ra07228d.
- M. N. Moshoeshoe and V. Obuseng, Simultaneous determination of nitrate, nitrite and phosphate in environmental samples by high performance liquid chromatography with UV detection, S. Afr. J. Chem., 2018, 71, 79–85, DOI:10.17159/0379-4350/2018/v71a10.
- X. Chen, H. Pu and Z. Fu, et al., Real-time and selective detection of nitrates in water using graphene-based field-effect transistor sensors, Environ. Sci.: Nano, 2018, 5(8), 1990–1999, 10.1039/C8EN00588E.
- K. S. Bhat, R. Ahmad, T. Mahmoudi and Y. B. Hahn, High performance chemical sensor with field-effect transistors array for selective detection of multiple ions, Chem. Eng. J., 2021, 417(August), 128064, DOI:10.1016/j.cej.2020.128064.
- G. N. Meloni, Building a microcontroller based potentiostat: a inexpensive and versatile platform for teaching electrochemistry and instrumentation, J. Chem. Educ., 2016, 93(7), 1320–1322, DOI:10.1021/acs.jchemed.5b00961.
- A. Ainla, M. P. S. Mousavi and M. N. Tsaloglou, et al., Open-Source Potentiostat for Wireless Electrochemical Detection with Smartphones, Anal. Chem., 2018, 90(10), 6240–6246, DOI:10.1021/acs.analchem.8b00850.
- X. Shen, F. Ju, G. Li and L. Ma, Smartphone-based electrochemical potentiostat detection system using pedot: Pss/chitosan/graphene modified screen-printed electrodes for dopamine detection, Sensors, 2020, 20(10), 2781, DOI:10.3390/s20102781.
- K. Promsuwan, A. Soleh and K. Samoson, et al., Novel biosensor platform for glucose monitoring via smartphone based on battery-less NFC potentiostat, Talanta, 2023, 256, 124266, DOI:10.1016/J.TALANTA.2023.124266.
- P. Bonato, Wearable sensors/systems and their impact on biomedical engineering, IEEE Eng Med Biol Mag, 2003, 22(3), 18–20, DOI:10.1109/memb.2003.1213622.
- B. F. Spencer, M. E. Ruiz-Sandoval and N. Kurata, Smart sensing technology: opportunities and challenges, Struct. Control Health Monit., 2004, 11(4), 349–368, DOI:10.1002/stc.48.
- N. T. Brannelly and A. J. Killard, An electrochemical sensor device for measuring blood ammonia at the point of care, Talanta, 2017, 167, 296–301, DOI:10.1016/j.talanta.2017.02.025.
- J. C. Chen, H. H. Chung, C. T. Hsu, D. M. Tsai, A. S. Kumar and J. M. Zen, A disposable single-use electrochemical sensor for the detection of uric acid in human whole blood, Sens. Actuators, B, 2005, 110(2), 364–369, DOI:10.1016/j.snb.2005.02.026.
- K. Nishiyama, R. Mizukami and S. Kuki, et al., Electrochemical enzyme-based blood ATP and lactate sensor for a rapid and straightforward evaluation of illness severity, Biosens. Bioelectron., 2022, 198, 113832, DOI:10.1016/j.bios.2021.113832.
- W. Zhang, Global pesticide use: profile, trend, cost/benefit and more, Proc. Int. Acad. Ecol. Environ. Sci., 2018, 8(1), 1–27 Search PubMed . https://www.iaees.org.
- D. Aune, R. Lau and D. S. M. Chan, et al., Dairy products and colorectal cancer risk: a systematic review and meta-analysis of cohort studies, Ann. Oncol., 2012, 23(1), 37–45, DOI:10.1093/annonc/mdr269.
- X. Liu, L. Duan and J. Mo, et al., Nitrogen deposition and its ecological impact in China : an overview, Environ. Pollut., 2011, 159(10), 2251–2264, DOI:10.1016/j.envpol.2010.08.002.
- P. Verma and J. K. Ratan, Assessment of the negative effects of the biosphere — an overview, Inorganic Pollutants in Water, INC, 2020, DOI:10.1016/B978-0-12-818965-8.00005-6.
- P. K. Bhattacharyya and N. Basak, Biological Nitrogen Fixation for Sustainable Agriculture, 2017, DOI:10.1007/978-981-10-5343-6.
- J. Mart and J. Berbel, Nitrogen fertilization. a review of the risks associated with the inefficiency of its use and policy responses, Sustainability, 2021,(3), 1–15 Search PubMed.
- J. Liang, Y. Zheng and Z. Liu, Nanowire-based Cu electrode as electrochemical sensor for detection of nitrate in water, Sens. Actuators, B, 2016, 232, 336–344, DOI:10.1016/j.snb.2016.03.145.
- H. Bagheri, A. Hajian, M. Rezaei and A. Shirzadmehr, Composite of Cu metal nanoparticles-multiwall carbon nanotubes-reduced graphene oxide as a novel and high performance platform of the electrochemical sensor for simultaneous determination of nitrite and nitrate, J. Hazard. Mater., 2017, 324(Part B), 762–772, DOI:10.1016/j.jhazmat.2016.11.055.
- H. R. Lot and R. Y. Lai, Comparison of nanostructured silver-modi fi ed silver and carbon ultramicroelectrodes for electrochemical detection of nitrate, Anal. Chim. Acta, 2015, 892, 153–159, DOI:10.1016/j.aca.2015.08.022.
- A. Ali, H. Jiang and N. K. Mahal, et al., Microfluidic impedimetric sensor for soil nitrate detection using graphene oxide and conductive nanofibers enabled sensing interface, Sens. Actuators, B, 2017, 239, 1289–1299, DOI:10.1016/j.snb.2016.09.101.
- A. A. Gokhale, J. Lu, R. R. Weerasiri, J. Yu and I. Lee, Amperometric detection and quantification of nitrate ions using a highly sensitive nanostructured membrane electrocodeposited biosensor array, Electroanalysis, 2015, 1127–1137, DOI:10.1002/elan.201400547.
- F. Can, S. K. Ozoner, P. Ergenekon and E. Erhan, Amperometric nitrate biosensor based on Carbon nanotube/Polypyrrole/Nitrate reductase bio fi lm electrode, Mater. Sci. Eng., C, 2012, 32(1), 18–23, DOI:10.1016/j.msec.2011.09.004.
- M. Faisal and U. Abu, Reduced graphene oxide/polypyrrole/nitrate reductase deposited glassy carbon electrode ( GCE/RGO/PPy/NR ): biosensor for the detection of nitrate in wastewater, Appl. Water Sci., 2018, 1–10, DOI:10.1007/s13201-018-0860-1.
- L. Wang, J. Kim and T. Cui, Self-assembled graphene and copper nanoparticles composite sensor for nitrate determination, Microsyst. Technol., 2018, 24, 3623–3630, DOI:10.1007/s00542-018-3792-7.
- X. Ma, M. Li and X. Liu, et al., A graphene oxide nanosheet-modi fi ed Ti nanocomposite electrode with enhanced electrochemical property and stability for nitrate reduction, Chem. Eng. J., 2018, 348(April), 171–179, DOI:10.1016/j.cej.2018.04.168.
- L. Yu, Q. Zhang and Q. Xu, et al., Talanta Electrochemical detection of nitrate in PM 2 . 5 with a copper-modi fi ed carbon fi ber micro-disk electrode, Talanta, 2015, 143, 245–253, DOI:10.1016/j.talanta.2015.04.049.
- Y. Li, J. Sun, C. Bian, J. Tong and S. Xia, Micro electrochemical sensor with copper nanoclusters for nitrate determination in freshwaters, Inst. Eng. Technol., 2012, 1197–1201, DOI:10.1049/mnl.2012.0533.
- A. M. Stortini, L. M. Moretto, A. Mardegan, M. Ongaro and P. Ugo, Arrays of copper nanowire electrodes : preparation , characterization and application as nitrate sensor, Sens. Actuators, B, 2015, 207, 186–192, DOI:10.1016/j.snb.2014.09.109.
- H. Essousi, H. Barhoumi and M. Bibani, et al., Ion-imprinted electrochemical sensor based on copper nanoparticles-polyaniline matrix for nitrate detection, J. Sens., 2019, 2020 Search PubMed.
- Y. Fu, C. Bian, J. Kuang, J. Wang, J. Tong and S. Xia, A palladium-tin modified microband electrode array for nitrate determination, Sensors, 2015, 23249–23261, DOI:10.3390/s150923249.
- M. A. Ali, X. Wang and Y. Chen, et al., Continuous Monitoring of Soil Nitrate Using a Miniature Sensor with Poly(3-octyl-thiophene) and Molybdenum Disulfide Nanocomposite, ACS Appl. Mater. Interfaces, 2019, 11(32), 29195–29206, DOI:10.1021/acsami.9b07120.
- A. Abbas, Dual Detection of Nitrate and Mercury in Water using Disposable Electrochemical Sensors, Biosens. Bioelectron., 2016, 85, 280–286, DOI:10.1016/j.bios.2016.05.017.
- D. L. Zhou, Q. L. Zhang and Z. Y. Lv, et al., Facile synthesis of a porous network-like silver film for electrocatalytic detection of nitrate, Microchim. Acta, 2013, 1495–1500, DOI:10.1007/s00604-013-1089-1.
- T. Wang, X. Xu, C. Wang, Z. Li and D. Li, A Novel Highly Sensitive Electrochemical Nitrite Sensor Based on a AuNPs/CS/Ti3C2 Nanocomposite, Nanomaterials, 2022, 12(3), 397, DOI:10.3390/nano12030397.
- N. Amini, A. Maleki and P. Maleki, Electrochemical detection of nitrate ions via reduction of NO2−and oxidation of NO reactions based on Cu@TiO2 coreshell/nafion/polyalizarin immobilized electrode, Mater. Chem. Phys., 2021, 264(September 2020), 3–10, DOI:10.1016/j.matchemphys.2021.124384.
- A. K. M. S. Inam, M. A. C. Angeli and B. Shkodra, et al., Flexible screen-printed electrochemical sensors functionalized with electrodeposited copper for nitrate detection in water, ACS Omega, 2021, 6(49), 33523–33532, DOI:10.1021/acsomega.1c04296.
- S. Aravamudhan and S. Bhansali, Development of micro-fluidic nitrate-selective sensor based on doped-polypyrrole nanowires, Chem. Pap., 2008, 132, 623–630, DOI:10.1016/j.snb.2008.01.046.
- F. Manea, A. Remes, C. Radovan, R. Pode, S. Picken and J. Schoonman, Talanta Simultaneous electrochemical determination of nitrate and nitrite in aqueous solution using Ag-doped zeolite-expanded graphite-epoxy electrode, Talanta, 2010, 83(1), 66–71, DOI:10.1016/j.talanta.2010.08.042.
- J. C. M. Gamboa and R. C. Pe, A renewable copper electrode as an amperometric flow detector for nitrate determination in mineral water and soft drink samples, Talanta, 2009, 80, 581–585, DOI:10.1016/j.talanta.2009.07.028.
- M. O. Mendoza, E. P. Ortega, O. A. De Fuentes, Y. Prokhorov and J. G. Luna, Chitosan/bentonite nanocomposite : preliminary studies of its potentiometric response to nitrate ions in water, 2014 IEEE 9th IberoAmerican Congress on sensors, IBERSENSOR 2014—conference proceedings, 2014, pp. 7–10 Search PubMed.
- A. Jang, Z. Zou, K. Kug, C. H. Ahn and P. L. Bishop, Potentiometric and voltammetric polymer lab chip sensors for determination of nitrate , pH and Cd ( II ) in water, Talanta, 2010, 83(1), 1–8, DOI:10.1016/j.talanta.2010.07.061.
- L. Zhang, M. Zhang, H. Ren, P. Pu, P. Kong and H. Zhao, Comparative investigation on soil nitrate-nitrogen and available potassium measurement capability by using solid-state and PVC ISE, Comput. Electron. Agric., 2015, 112, 83–91, DOI:10.1016/j.compag.2014.11.027.
- M. A. Ali, K. Mondal, Y. Wang, N. K. Mahal, M. J. Castellano and L. Dong, Microfluidic detection of soil nitrate ions using novel electrochemical foam electrode, in 2017 IEEE 30th International Conference on Micro Electro Mechanical Systems (MEMS), IEEE, 2017, pp. 482–485, DOI:10.1109/MEMSYS.2017.7863448.
- R. Ahmad, K. S. Bhat, M. S. Ahn and Y. B. Hahn, Fabrication of a robust and highly sensitive nitrate biosensor based on directly grown zinc oxide nanorods on a silver electrode, New J. Chem., 2017, 41(19), 10992–10997, 10.1039/C7NJ02526B.
- T. Öznülüer, B. Özdurak and H. Ö. Doğan, Electrochemical Reduction of Nitrate on Graphene Modified Copper Electrodes in alkaline media, J. Electroanal. Chem., 2013, 699, 1–5, DOI:10.1016/j.jelechem.2013.04.001.
- E. Andreoli, V. Annibaldi, D. A. Rooney, K. S. Liao, N. J. Alley and S. A. Curran, Electrochemical conversion of copper-based hierarchical micro/nanostructures to copper metal nanoparticles and their testing in nitrate sensing, Electroanalysis, 2011,(9), 2164–2173, DOI:10.1002/elan.201100105.
- D. Pan, W. Lu, S. Wu, H. Zhang and W. Qin, In situ spontaneous redox synthesis of carbon nanotubes/copper oxide nanocomposites and their preliminary application in electrocatalytic reduction of nitrate, Mater. Lett., 2012, 89, 333–335, DOI:10.1016/j.matlet.2012.09.004.
- H. Medetalibeyoglu, G. Kotan, N. Atar and M. L. Yola, A novel sandwich-type SERS immunosensor for selective and sensitive carcinoembryonic antigen (CEA) detection, Anal. Chim. Acta, 2020, 1139, 100–110, DOI:10.1016/j.aca.2020.09.034.
- Y. Li, Z. Zhang, Y. Song, et al., Determination of nitrate in potable water using a miniaturized electrochemical sensor, 2018 IEEE 13th Annual International Conference on Nano/Micro Engineered and Molecular Systems, 2018, pp. , pp. 619–622 Search PubMed.
- M. C. Tsai, D. X. Zhuang and P. Y. Chen, Electrodeposition of macroporous silver films from ionic liquids and assessment of these films in the electrocatalytic reduction of nitrate, Electrochim. Acta, 2010, 55, 1019–1027, DOI:10.1016/j.electacta.2009.09.070.
- J. Hu, J. Sun, C. Bian and J. Tong, 3D dendritic nanostructure of silver-array: preparation, growth mechanism and application in nitrate sensor, Electroanalysis, 2013,(2), 546–556, DOI:10.1002/elan.201200465.
- D. C. Legrand, C. Barus and V. Garc, Square wave voltammetry measurements of low concentrations of nitrate using Au/AgNPs electrode in chloride solutions, Electroanalysis, 2017, 1–7, DOI:10.1002/elan.201700447.
- K. Fajerwerg, V. Ynam, B. Chaudret, V. Garçon, D. Thouron and M. Comtat, Electrochemistry Communications An original nitrate sensor based on silver nanoparticles electrodeposited on a gold electrode, Electrochem. Commun., 2010, 12(10), 1439–1441, DOI:10.1016/j.elecom.2010.08.003.
- Z. Mumtarin, M. M. Rahman, H. M. Marwani and M. A. Hasnat, Electro-kinetics of conversion of NO3− into NO2−and sensing of nitrate ions via reduction reactions at copper immobilized platinum surface in the neutral medium, Electrochim. Acta, 2020, 346, 135994, DOI:10.1016/j.electacta.2020.135994.
- A. Gutés, C. Carraro and R. Maboudian, Ac ce pt cr t, Electrochim. Acta, 2013, 103, 38–43, DOI:10.1016/j.electacta.2013.03.199.
- N. Comisso, S. Cattarin and P. Guerriero, Study of Cu, Cu-Ni and Rh-modified Cu porous layers as electrode materials for the electroanalysis of nitrate and nitrite ions, J. Solid State Electrochem., 2016, 20, 1139–1148, DOI:10.1007/s10008-015-2915-7.
- S. Zhao, J. Tong, Y. Li, J. Sun, C. Bian and S. Xia, Palladium-gold modified ultramicro interdigital array electrode chip for nitrate detection in neutral water, Micromachines, 2019, 10(4), 223, DOI:10.3390/mi10040223.
- A. Afkhami, T. Madrakian, H. Ghaedi and H. Khanmohammadi, Construction of a chemically modified electrode for the selective determination of nitrite and nitrate ions based on a new nanocomposite, Electrochim. Acta, 2012, 66, 255–264, DOI:10.1016/j.electacta.2012.01.089.
- T. Ramakrishnappa, K. Sureshkumar and M. Pandurangappa, Copper Oxide Impregnated Glassy Carbon Spheres Based Electrochemical Interface For Nitrite/Nitrate Sensing, Mater. Chem. Phys., 2020, 245, 122744, DOI:10.1016/j.matchemphys.2020.122744.
- M. A. B. E. Alcock-earley, A conducting polymer/Ag nanoparticle composite as a nitrate sensor, J. Appl. Electrochem., 2011, 1341–1347, DOI:10.1007/s10800-011-0354-4.
- M. R. Mahmoudian, Y. Alias and W. J. Basirun, et al., A sensitive electrochemical nitrate sensor based on polypyrrole coated palladium nanoclusters, J. Electroanal. Chem., 2015, 751, 30–36, DOI:10.1016/j.jelechem.2015.05.026.
- B. Liu and B. X. Zou, Electrocatalytic sensing of nitrate at Cu nanosheets electrodeposited on WO3/polyaniline modified electrode., Adv. Mater. Res., 2014, 883, 159–164, DOI:10.4028/www.scientific.net/AMR.881-883.159.
- D. Centre, Electrochemical determination of nitrate ion from fresh water on nanosphere polypyrrole modified electrode surface, Int. J. Res. Anal. Rev., 2018, 5(3), 1807–1813 Search PubMed.
- M. H. Motaghedifard, S. M. Pourmortazavi, M. Alibolandi and S. Mirsadeghi, Au-modified organic/inorganic MWCNT/Cu/PANI hybrid nanocomposite electrode for electrochemical determination of nitrate ions, Microchim. Acta, 2021, 99 CrossRef CAS PubMed.
- M. Bhargavi, N. Nesakumar and B. Lakshmishri, Zinc oxide nanoparticles-based electrochemical sensor for the detection of nitrate ions in water with a low detection limit — a chemometric approach 1, J. Anal. Chem., 2017, 72(3), 316–326, DOI:10.1134/S1061934817030078.
- G. Feiner, Color in cured meat products and fresh meat, in Salami, Elsevier, 2016, pp. 89–101, DOI:10.1016/B978-0-12-809598-0.00005-6.
- Q. Xiao, M. Feng, Y. Liu, S. Lu, Y. He and S. Huang, The graphene/polypyrrole/chitosan-modified glassy carbon electrode for electrochemical nitrite detection, Ionics, 2017 DOI:10.1007/s11581-017-2247-y.
- Z. Fan, L. Sun, S. Wu and C. Liu, Preparation of manganese porphyrin/niobium tungstate nanocomposites for enhanced electrochemical detection of nitrite, J. Mater. Sci., 2019, 54, 10204–10216, DOI:10.1007/s10853-019-03526-4.
- S. Zhang, B. Li, Q. Sheng and J. Zheng, Electrochemical sensor for sensitive determination of nitrite based on the CuS-MWCNTs nanocomposites, J. Electroanal. Chem., 2016, 769, 118–123, DOI:10.1016/j.jelechem.2016.03.025.
- Y. Dai, J. Huang, H. Zhang and C. C. Liu, Sens. Actuators, B, 2018, 281, 746–750, DOI:10.1016/j.snb.2018.11.014.
- R. Ahmad, M. S. Ahn and Y. B. Hahn, A highly sensitive nonenzymatic sensor based on Fe2O3 nanoparticle coated ZnO nanorods for electrochemical detection of nitrite, Adv. Mater. Interfaces, 2017, 1700691, DOI:10.1002/admi.201700691.
- S. Saif, A. Tahir, T. Asim and Y. Chen, Plant mediated green synthesis of CuO nanoparticles: comparison of toxicity of engineered and plant mediated CuO nanoparticles
towards Daphnia magna, Nanomaterials, 2016, 6(11), 1–15, DOI:10.3390/nano6110205.
- M. Ghanei-motlagh and M. A. Taher, A novel electrochemical sensor based on silver/halloysite nanotube/molybdenum disulfide nanocomposite for efficient nitrite sensing, Biosens. Bioelectron., 2018, 109, 279–285, DOI:10.1016/j.bios.2018.02.057.
- H. Rao, Y. Liu, J. Zhong, Z. Zhang, X. Zhao and X. Liu, Gold nanoparticle/chitosan@N,S Co-doped multiwalled carbon nanotubes sensor: fabrication, characterization, and electrochemical detection of catechol and nitrite, ACS Sustainable Chem. Eng., 2017, 5(11), 10926–10939, DOI:10.1021/acssuschemeng.7b02840.
- C. Ma, Y. Qian and S. Zhang, et al., Temperature-controlled ethanolamine and Ag-nanoparticle dual- functionalization of graphene oxide for enhanced electrochemical nitrite determination, Sens. Actuators, B, 2018, 274(March), 441–450, DOI:10.1016/j.snb.2018.08.012.
- L. Wu, X. Zhang, M. Wang, L. He and Z. Zhang, Preparation of Cu2O/CNTs composite and its application as sensing platform for detecting nitrite in water environment, J. Int. Meas. Confed., 2018, 128(May), 189–196, DOI:10.1016/j.measurement.2018.06.041.
- X. Huang, Y. Li, Y. Chen and L. Wang, Electrochemical determination of nitrite and iodate by use of gold nanoparticles/poly (3-methylthiophene ) composites coated glassy carbon electrode, Sens. Actuators, B, 2008, 134, 780–786, DOI:10.1016/j.snb.2008.06.028.
- B. R. Kozub, N. V. Rees and R. G. Compton, Electrochemical determination of nitrite at a bare glassy carbon electrode ; why chemically modify electrodes ?, Sens. Actuators, B, 2010, 143, 539–546, DOI:10.1016/j.snb.2009.09.065.
- Y. Cui, C. Yang, W. Zeng, M. Oyama, W. Pu and J. Zhang, Electrochemical Determination of Nitrite Using a Gold Nanoparticles-modified Glassy Carbon Electrode Prepared by the Seed-mediated Growth Technique, Anal. Sci., 2007, 23(12), 1421–1425, DOI:10.2116/analsci.23.1421.
- K. Rajalakshmi and S. A. John, Highly sensitive determination of nitrite using FMWCNTs-conducting polymer composite modified electrode, Sens. Actuators, B, 2015, 215, 119–124, DOI:10.1016/j.snb.2015.03.050.
- S. Rajesh, A. Koteswararao, K. Bhargava, G. Ilavazhagan, S. Kotamraju and C. Karunakaran, Biosensors and Bioelectronics Simultaneous electrochemical determination of superoxide anion radical and nitrite using Cu , ZnSOD immobilized on carbon nanotube in polypyrrole matrix, Biosens. Bioelectron., 2010, 26(2), 689–695, DOI:10.1016/j.bios.2010.06.063.
- M. Badea, A. Amine, G. Palleschi, D. Moscone, G. Volpe and A. Curulli, New electrochemical sensors for detection of nitrites and nitrates, J. Electroanal. Chem., 2001, 509(1), 66–72, DOI:10.1016/S0022-0728(01)00358-8.
- C. Caro, Electrocatalytic oxidation of nitrite on a vitreous carbon electrode modified with cobalt phthalocyanine, Electrochim. Acta, 2002, 47(9), 1489–1494, DOI:10.1016/S0013-4686(01)00875-1.
- S. Da, S. Cosnier, M. G. Almeida and J. G. Moura, An efficient poly ( pyrrole – viologen ) -nitrite reductase biosensor for the mediated detection of nitrite, Electrochem. Commun., 2004, 6, 404–408, DOI:10.1016/j.elecom.2004.02.007.
- L. H. Chen, J. B. Zang, Y. H. Wang and L. Y. Bian, Electrochemical oxidation of nitrite on nanodiamond powder electrode, Electrochim. Acta, 2008, 53, 3442–3445, DOI:10.1016/j.electacta.2007.12.023.
- S. Wang, Y. Yin and X. Lin, Cooperative effect of Pt nanoparticles and Fe(III) in the electrocatalytic oxidation of nitrite, Electrochem. Commun., 2004, 6, 259–262, DOI:10.1016/j.elecom.2003.12.008.
- A. Salimi, R. Hallaj, H. Mamkhezri, S. Mohamad and T. Hosaini, Electrochemical properties and electrocatalytic activity of FAD immobilized onto cobalt oxide nanoparticles:application to nitrite detection, J. Electroanal. Chem., 2008, 620, 31–38, DOI:10.1016/j.jelechem.2008.03.003.
- O. Salhi, T. Ez-zine, L. Oularbi and M. El Rhazi, Electrochemical sensing of nitrite ions using modified electrode by poly functionalized multi-walled carbon nanotubes, Front. Chem., 2022, 10, 1–12, DOI:10.3389/fchem.2022.870393.
- R. Mo, X. Wang, Q. Yuan, X. Yan, T. Su, Y. Feng, L. Lv, C. Zhou, P. Hong, S. Sun, Z. Wang and C. Li, Sensors, 2018, 18(7), 1986, DOI:10.3390/s18071986.
- K. S. Novoselov, A. K. Geim and S. V. Morozov, et al., Electric Field Effect in Atomically Thin Carbon Films, Science, 2004, 306(5696), 666–669, DOI:10.1126/science.1102896.
- S. Neampet, N. Ruecha, J. Qin, W. Wonsawat, O. Chailapakul and N. Rodthongkum, A nanocomposite prepared from platinum particles, polyaniline and a Ti3C2 MXene for amperometric sensing of hydrogen peroxide and lactate, Microchim. Acta, 2019, 186(12), 752, DOI:10.1007/s00604-019-3845-3.
- P. K. Kannan, D. J. Late, H. Morgan and C. S. Rout, Recent developments in 2D layered inorganic nanomaterials for sensing, Nanoscale, 2015, 7(32), 13293–13312, 10.1039/C5NR03633J.
- A. Abellán-Llobregat, I. Jeerapan and A. Bandodkar, et al., A stretchable and screen-printed electrochemical sensor for glucose determination in human perspiration, Biosens. Bioelectron., 2017, 91, 885–891, DOI:10.1016/j.bios.2017.01.058.
- S. S. Chen, S. Y. Cheng, A. J. Wang, L. X. Xiao and F. J. Ju, Free-standing Pt nanowire networks with clean surfaces : highly sensitive electrochemical detection of nitrite, J. Electroanal. Chem., 2017, 791, 131–137, DOI:10.1016/j.jelechem.2017.03.016.
- Z. Zhao, Z. Xia, C. Liu, H. Huang and W. Ye, Green synthesis of Pd/Fe 3 O 4 composite based on polyDOPA functionalized reduced graphene oxide for electrochemical detection of nitrite in cured food, Electrochim. Acta, 2017, 256, 146–154, DOI:10.1016/j.electacta.2017.09.185.
- M. Eguílaz, L. Agüí and J. M. Pingarrón, A biosensor based on cytochrome c immobilization on a electrode . Application to the electrochemical determination of nitrite, J. Electroanal. Chem., 2010, 644(1), 30–35, DOI:10.1016/j.jelechem.2010.03.025.
- Z. H. Wen and T. F. Kang, Determination of nitrite using sensors based on nickel phthalocyanine polymer modified electrodes, Talanta, 2004, 62, 351–355, DOI:10.1016/j.talanta.2003.08.003.
- M. Pal and V. Ganesan, Electrochemical determination of nitrite using silver nanoparticles modified electrode, Analyst, 2010,(2), 2711–2716, 10.1039/c0an00289e.
- L. Jiang, R. Wang, X. Li, L. Jiang and G. Lu, Electrochemical oxidation behavior of nitrite on a chitosan-carboxylated multiwall carbon nanotube modified electrode, Electrochem. Commun., 2005, 7, 597–601, DOI:10.1016/j.elecom.2005.04.009.
- T. N. Rao, D. A. Tryk and A. Fujishima, Determination of nitrite and nitrogen oxides by anodic voltammetry at conductive diamond electrodes determination of nitrite and nitrogen oxides by anodic voltammetry at conductive diamond electrodes, Electrochem. Soc., 2001, 148(3) DOI:10.1149/1.1346611.
- P. Kalimuthu and S. A. John, Electrochemistry Communications Highly sensitive and selective amperometric determination of nitrite using electropolymerized film of functionalized thiadiazole modified glassy carbon electrode, Electrochem. Commun., 2009, 11(5), 1065–1068, DOI:10.1016/j.elecom.2009.03.015.
- M. A. Kamyabi and F. Aghajanloo, Electrocatalytic oxidation and determination of nitrite on carbon paste electrode modified with oxovanadium (IV)-4-methyl salophen, J. Electroanal. Chem., 2008, 614, 157–165, DOI:10.1016/j.jelechem.2007.11.026.
- S. Yang, X. Liu and X. Zeng, et al., Fabrication of nano-copper/carbon nanotubes/chitosan film by one-step electrodeposition and its sensitive determination of nitrite, Sens. Actuators, B, 2010, 145(2), 762–768, DOI:10.1016/j.snb.2010.01.032.
- M. Parsaei, Z. Asadi and S. Khodadoust, A sensitive electrochemical sensor for rapid and selective determination of nitrite ion in water samples using modified carbon paste electrode with a newly synthesized
cobalt (II)-Schiff base complex and magnetite nanospheres, Sens. Actuators, B, 2015, 220, 1131–1138, DOI:10.1016/j.snb.2015.06.096.
- Y. Zhang, J. Nie and H. Wei, et al., Electrochemical detection of nitrite ions using Ag/Cu/MWNT nanoclusters electrodeposited on a glassy carbon electrode, Sens. Actuators, B, 2018, 258, 1107–1116, DOI:10.1016/j.snb.2017.12.001.
- M. Talbi, A. Al-Hamry, P. R. Teixeira, L. G. Paterno, M. B. Ali and O. Kanoun, Enhanced Nitrite Detection by a Carbon Screen Printed Electrode Modified with Photochemically-Made AuNPs, Chemosensors, 2022, 10, 40, DOI:10.3390/chemosensors10020040.
- R. Sivasubramanian and M. V. Sangaranarayanan, Electrochemical sensing of nitrite ions using tin- submicroparticles modified glassy carbon electrodes, Electroanalysis, 2014, 1–8, DOI:10.1002/elan.201400259.
- A. Baciu, F. Manea, A. Pop and R. Pode, Simultaneous voltammetric detection of ammonium and nitrite from groundwater at silver-electrodecorated carbon nanotube electrode, Process Saf. Environ. Prot., 2016, 1–8, DOI:10.1016/j.psep.2016.05.006.
- J. Gallardo-gonzalez, A. Baraket and S. Boudjaoui, et al., Science of the Total Environment A fully integrated passive micro fl uidic Lab-on-a-Chip for real-time electrochemical detection of ammonium : sewage applications, Sci. Total Environ., 2019, 653, 1223–1230, DOI:10.1016/j.scitotenv.2018.11.002.
- J. Schwarz, K. Trommer and M. Mertig, Solid-contact ion-selective electrodes based on graphite paste for potentiometric nitrate and ammonium determinations, Am. J. Anal. Chem., 2018, 591–601, DOI:10.4236/ajac.2018.912043.
- Y. Huang, T. Wang and Z. Xu, et al., Real-time in situ monitoring of nitrogen dynamics in wastewater treatment processes using wireless, solid- state, and ion-selective membrane (S-ISM) sensors, Environ. Sci. Technol., 2019 DOI:10.1021/acs.est.8b05928.
- O. Y. Saiapina, S. V. Dzyadevych, A. Walcarius, V. Cedex, T. Shevchenko and K. National, A novel highly sensitive zeolite-based conductometric microsensor for ammonium determination, Anal. Lett., 2012, 37–41, DOI:10.1080/00032719.2012.675487.
- O. Y. Saiapina, S. G. Kharchenko, S. G. Vishnevskii, V. M. Pyeshkova, V. I. Kalchenko and S. V. Dzyadevych, Development of Conductometric Sensor Based on 25 , 27-Di- ( 5-thio- Determination of Ammonium, Nanoscale Res. Lett., 2016, 11, 105, DOI:10.1186/s11671-016-1317-9.
- G. Wang, J. Gao, B. Sun, D. He, C. Zhao and H. Suo, Enhanced ammonia sensitivity electrochemical sensors based on PtCu alloy nanoparticles in-situ synthesized on carbon cloth electrode, J. Electroanal. Chem., 2022, 922, 116721, DOI:10.1016/j.jelechem.2022.116721.
- C. Wang, T. Wang, Z. Li, X. Xu, X. Zhang and D. Li, An Electrochemical Enzyme Biosensor for Ammonium Detection in Aquaculture Using Screen-Printed Electrode Modified by Gold Nanoparticle/Polymethylene Blue, Biosensors, 2021, 11(9), 335, DOI:10.3390/bios11090335.
- J. A. Ribeiro, F. Silva and C. M. Pereira, Electrochemical sensing of ammonium ion at the water/1,6-dichlorohexane interface, Talanta, 2012, 88, 54–60, DOI:10.1016/j.talanta.2011.09.054.
- L. L. Tan, A. Musa, Y. H. Lee, U. Sains and B. B. Nilai, Determination of ammonium ion using a reagentless amperometric biosensor based on immobilized alanine dehydrogenase, Sensors, 2011, 9344–9360, DOI:10.3390/s111009344.
- S. Kumbhat and U. Singh, A potassium-selective electrochemical sensor based on crown-ether functionalized self assembled monolayer, J. Electroanal. Chem., 2018, 809(July 2017), 31–35, DOI:10.1016/j.jelechem.2017.12.051.
- D. Iannazzo, C. Espro, A. Ferlazzo, C. Celesti, C. Branca and G. Neri, Electrochemical and Fluorescent Properties of Crown Ether Functionalized Graphene Quantum Dots for Potassium and Sodium Ions Detection, Nanomaterials, 2021, 11(11), 2897, DOI:10.3390/nano11112897.
- X. Ji, J. Li and C. Yang, A Label-Free Electrochemical Aptasensor for the Analysis of The Potassium Ion, J. Immunoassay Immunochem., 2015, 36(2), 162–169, DOI:10.1080/15321819.2014.915221.
- M. Tounsi, M. Ben Braiek, A. Baraket, M. Lee, N. Zine, M. Zabala, J. Bausells, F. Aloui, B. Ben Hassine, A. Maaref and A. Errachid, Electrochemical capacitive K+ EMIS chemical sensor based on the dibromoaza [7] helicene as an ionophore for potassium ions detection, Electroanalysis, 2016, 28, 2892, DOI:10.1002/elan.201600104.
- M. Jarczewska, Ł. Górski and E. Malinowska, Application of DNA aptamers as sensing layers for electrochemical detection of potassium ions, Sens. Actuators, B, 2016, 226, 37–43, DOI:10.1016/j.snb.2015.11.139.
- Z. Chen, L. Chen, H. Ma, T. Zhou and X. Li, Aptamer biosensor for label-free impedance spectroscopy detection of potassium ion based on DNA G-quadruplex conformation, Biosens. Bioelectron., 2013, 48, 108–112, DOI:10.1016/j.bios.2013.04.007.
- L. D. Li, H. X. Qing and L. Guo, Electrochemical potassium ion sensor based on DNA G-quadruplex conformation and gold nanoparticle amplification, Rare Met., 2013, 32, 369–374, DOI:10.1007/s12598-013-0102-5.
- B. Zhu, M. A. Booth, Y. Woo and J. M. Hodgkiss, Label-free, electrochemical quantitation of potassium ions from femtomolar levels, Chem.–Asian J., 2015, 2169–2175, DOI:10.1002/asia.201500313.
- S. Bhandari, U. Singh and S. Kumbhat, Nafion-modified carbon based sensor for soil potassium detection, Electroanalysis, 2019, 31(5), 813–819, DOI:10.1002/elan.201800583.
- A. S. Lima, N. Bocchi, H. M. Gomes and M. F. S. Teixeira, An Electrochemical Sensor Based on Nanostructured Hollandite-type Manganese Oxide for Detection of Potassium Ions, Sensors, 2009, 9, 6613–6625, DOI:10.3390/s90906613.
- M. S. Ahn, R. Ahmad, J. Y. Yoo and Y. B. Hahn, Journal of Colloid and Interface Science Synthesis of manganese oxide nanorods and its application for potassium ion sensing in water, J. Colloid Interface Sci., 2018, 516, 364–370, DOI:10.1016/j.jcis.2018.01.081.
- X. Liu, C. Ye and X. Li, Highly sensitive and selective potassium ion detection based on graphene hall effect biosensors, Materials, 2018, 1–9, DOI:10.3390/ma11030399.
- H. Chai, X. Ma, F. Meng, Q. Mei, Y. Tang and P. Miao, Electrochemical aptasensor based on a potassium ion-triggered DNA conformation transition and self-assembly on an electrode, New J. Chem., 2019, 43(21), 7928–7931, 10.1039/C9NJ00158A.
- Z. Chen, J. Guo, S. Zhang and L. Chen, Short communication A one-step electrochemical sensor for rapid detection of potassium ion based on structure-switching aptamer, Sens. Actuators, B, 2013, 188, 1155–1157, DOI:10.1016/j.snb.2013.08.039.
- T. N. T. Tran, S. Qiu and H. J. Chung, Potassium Ion Selective Electrode Using Polyaniline and Matrix-Supported Ion-Selective PVC Membrane, IEEE Sens. J., 2018, 18(22), 9081–9087, DOI:10.1109/JSEN.2018.2871001.
- A. A. Kumar, S. K. N. Kumar and R. E. Fernandez, Real Time Sensing of Soil Potassium Levels Using Zinc Oxide-Multiwall Carbon Nanotube-Based Sensors, IEEE Trans. NanoBiosci., 2021, 20(1), 50–56, DOI:10.1109/TNB.2020.302786.
- M. McCole, M. Bradley, M. McCaul and D. McCrudden, A low-cost portable system for on-site detection of soil pH and potassium levels using 3D printed sensors, Results Eng., 2023, 20(November), 101564, DOI:10.1016/j.rineng.2023.101564.
- M. F. S. Teixeira, B. H. Freitas, P. M. Seraphim, L. O. Salmazo, M. A. Nobre and S. Lanfredi, Procedia Chemistry Development of an electrochemical sensor for potassium ions based on KSr 2 Nb 5 O 15 modified electrode, Procedia Chem., 2009, 1(1), 293–296, DOI:10.1016/j.proche.2009.07.073.
- H. Li, Y. Zhu, S. Islam, A. Rahman, K. B. Walsh and G. Koley, Graphene field effect transistors for highly sensitive and selective detection of K+ ions, Sens. Actuators, B, 2017, 253, 759–765, DOI:10.1016/j.snb.2017.06.129.
- F. S. Diba and H. J. S. C. Lee, Amperometric sensing of sodium, calcium and potassium in biological fluids using a microhole supported liquid/gel interface, J. Electroanal. Chem., 2016, 769, 5–10, DOI:10.1016/j.jelechem.2016.02.045.
- C. Tharini, G. Iyappan, E. Manikandan and P. J. Sephra, Potentiometric sensing of potassium ion (K+) using valinomycin supported on ZnO/rGO nanocomposites, J. Mater. Sci.: Mater. Electron., 2023, 34(19), 1474, DOI:10.1007/s10854-023-10806-y.
- A. A. Kumar, S. K. N. Kumar and R. E. Fernandez, Real Time Sensing of Soil Potassium Levels Using Zinc Oxide-Multiwall Carbon Nanotube-Based Sensors, IEEE Trans. NanoBiosci., 2021, 20(1), 50–56, DOI:10.1109/TNB.2020.3027863.
- Y. Wang, Y. Wang and R. Zhu, et al., Woven fiber organic electrochemical transistors based on multiwalled carbon nanotube functionalized PEDOT nanowires for nondestructive detection of potassium ions, Mater. Sci. Eng., B, 2022, 278, 115657, DOI:10.1016/j.mseb.2022.115657.
- H. Marschner, Mineral Nutrition of Higher Plants, 2nd edn, 1995 Search PubMed.
- D. P. Schachtman and R. Shin, Nutrient Sensing and Signaling: NPKS, Annu. Rev. Plant Biol., 2007, 58(1), 47–69, DOI:10.1146/annurev.arplant.58.032806.103750.
- Y. Wang, Q. He and X. Zhao, et al., Journal of Environmental Chemical Engineering Synthesis of corn straw-based graphene quantum dots ( GQDs ) and their application in PO43- detection, Environ. Chem. Eng., 2022, 10(2), 4–10 Search PubMed.
- M. Khaydukova, D. Kirsanov and S. Sarkar, et al., One shot evaluation of NPK in soils by “electronic tongue”, Comput. Electron. Agric., 2021, 186(July 2020), 106208, DOI:10.1016/j.compag.2021.106208.
- D. Talarico, F. Arduini, A. Amine, D. Moscone and G. Palleschi, Screen-printed electrode modified with carbon black nanoparticles for phosphate detection by measuring the electroactive phosphomolybdate complex, Talanta, 2015, 141, 267–272, DOI:10.1016/j.talanta.2015.04.006.
- F. Kabir, T. Rahman, A. Gurung and Q. Qiao, Electrochemical Phosphate Sensors using Silver Nanowires Treated Screen Printed Electrodes, IEEE Sens. J., 2018, 1–6 Search PubMed.
- M. Xu, J. Song, N. Li and C. Zhao, Sensitive determination of platinum by the parallel catalytic hydrogen wave of Pt (IV) in the presence of persulfate, J. Electroanal. Chem., 2003, 553, 163–168, DOI:10.1016/S0022-0728(03)00319-X.
- J. Zhang, Y. Bian, D. Liu, Z. Zhu, Y. Shao and M. Li, Detection of Phosphate in Human Blood Based on a Catalytic Hydrogen Wave at a Molybdenum Phosphide Modified Electrode, Anal. Chem., 2019, 91(22), 14666–14671, DOI:10.1021/acs.analchem.9b03862.
- J. Xu, Z. Gao, X. Dou and Y. Y. Song, Needle-like Co3O4 nanoarrays as a dual-responsive amperometric sensor for enzyme-free detection of glucose and phosphate anion, J. Electroanal. Chem., 2021, 897(June), 115605, DOI:10.1016/j.jelechem.2021.115605.
- G. J. Parasuraman, R. Vishnuraj, S. Kannankuzhiyil, M. Govindaraj, S. S. Biji and M. Rangarajan, Determination of urea, phosphate, and potassium in agricultural runoff waters using electrochemical impedance spectroscopy, Environ. Sci. Pollut. Res., 2023, 30(44), 98858–98868, DOI:10.1007/s11356-022-22369-2.
- M. A. Rahman, D. S. Park, S. C. Chang, C. J. McNeil and Y. B. Shim, The biosensor based on the pyruvate oxidase modified conducting polymer for phosphate ions determinations, Biosens. Bioelectron., 2006, 21(7), 1116–1124, DOI:10.1016/j.bios.2005.04.008.
- L. Gilbert, A. T. A. Jenkins, S. Browning and J. P. Hart, Development of an amperometric, screen-printed, single-enzyme phosphate ion biosensor and its application to the analysis of biomedical and environmental samples, Sens. Actuators, B, 2011, 160(1), 1322–1327, DOI:10.1016/j.snb.2011.09.069.
- J. Jońca, W. Giraud and C. Barus, et al., Reagentless and silicate interference free electrochemical phosphate determination in seawater, Electrochim. Acta, 2013, 88, 165–169, DOI:10.1016/j.electacta.2012.10.012.
- A. V. Kolliopoulos, D. K. Kampouris and C. E. Banks, Rapid and Portable Electrochemical Quanti fi cation of Phosphorus, Anal. Chem., 2015, 87(8), 4269–4274, DOI:10.1021/ac504602a.
- S. M. Mugo, W. Lu and S. Lemieux, Stainless steel electrochemical capacitive microneedle sensors for multiplexed simultaneous measurement of pH, nitrates, and phosphates, Microchim. Acta, 2022, 189, 206, DOI:10.1007/s00604-022-05307-4.
|
This journal is © The Royal Society of Chemistry 2024 |
Click here to see how this site uses Cookies. View our privacy policy here.