DOI:
10.1039/D3RA07697K
(Paper)
RSC Adv., 2024,
14, 13200-13208
Formation of H-bonding networks in the solid state structure of a trinuclear cobalt(III/II/III) complex with N2O2 donor Schiff base ligand and glutaric acid as bridging co-ligand: synthesis, structure and DFT study†
Received
14th November 2023
, Accepted 9th April 2024
First published on 23rd April 2024
Abstract
A trinuclear linear mixed-valence centrosymmetric cobalt(III)-cobalt(II)-cobalt(III) complex, [CoII{(μ-L)(μ-Hglu)CoIII(OH2)}2](ClO4)2·6H2O has been synthesized during tetradentate N2O2 donor ‘Schiff base’ ligand, H2L {N,N′-bis(salicylidene)-1,3-diaminopropane} and glutaric acid (H2glu) as anionic co-ligand. The complex has been characterized by spectroscopic measurements and its solid state structure has been determined by single crystal X-ray diffraction analysis. The supra-molecular assembly formed by the hydrogen bonding interactions in the solid state of the complex has been analysed using DFT calculations.
Introduction
The use of cobalt complexes in several biomimetic catalysis is well known.1–21 Cobalt complexes have also been used to fabricate different opto-electronic devices.22,23 The magnetic properties of cobalt complexes are also interesting.24–27 On the other hand, the attractive bridging capacity of the phenolate oxygen atoms of salen type ligands has led synthetic inorganic chemists and material scientist to synthesize and characterize high nuclearity cobalt complexes with these salen type ligands.28–30 The complexes have been used as important magnetic materials.31,32 Cobalt-salen related systems may catalyze the oxygenation of organic molecules and may be used as antiviral agents.1,33–45 They have also been used to mimic several enzymes.38–45 These types of promising applications of salen type complexes have attracted our attention for the synthesis of transition metal compounds of salen type N2O2 donor Schiff base ligands.
Water is essential for many biological and chemical reactions in any living body.46–49 Chemists, micro-biologists and theoretical chemists are trying to understand the behavior of solid and liquid water at the molecular level since long.50 To achieve a more precise narrative of the properties of bulk water at the molecular level, determination of the accurate structural data of various hydrogen-bonded water aggregations is necessary, where water clusters may assist the movement of protons along the H-bonded chain.51 To investigate the stabilization of hydrogen-bonded water clusters or chains in the solid state structure of a transition metal complex is a very attracting area of research to the structural and theoretical chemists, as it may help to propose different models for the aggregations of water molecules in numerous in vivo pathways.52–56 Different water clusters, e.g. tetramers, hexamers, octamers, decamers etc. found in a number of crystal hosts, have been extensively investigated.57–63 A useful approach to the stabilization of water molecule aggregations is their capture within transition metal complex lattices formed by crystallization from aqueous solution.57,64–77 To study the supramolecular architecture of transition metal complexes in its solid state structure is receiving immense attention now-a-days.78 In the case of metal complexes the supramolecular components are most often held together by hydrogen bonding, π⋯π, C–H⋯π interactions, cation-π, anion-π etc.79–82 Obviously, the most commonly used approach for engineering the supramolecular structure of these complexes is to employ hydrogen bonding.83–89 Carboxylate bridged trinuclear mixed valence cobalt(III/II/III) complexes with salen type ligands constitute a special class for their interesting structures and each of synthesis.28,30,84,90 The complexes could easily be prepared by adding cobalt(II) salt to a salen type ligand in presence of carboxylate co-ligands maintaining either 3
:
2
:
2 or 3
:
2
:
4 molar ratio. In the present work, we have isolated a linear, trinuclear, mixed valence cobalt(III/II/III) complex using a salen type quadridentate Schiff base ligand and di-carboxylic acid (glutaric acid) as the bridging co-ligand. Interestingly, the complex comes cationic in nature with counter perchlorate anions. Herein, we report the synthesis, characterization and supra-molecular assemblies of the cobalt(III/II/III) Schiff base complex, [CoII{(μ-L)(μ-Hglu)CoIII(OH2)}2](ClO4)2·6H2O [H2L = N,N′-bis(salicylidene)-1,3-diaminopropane, H2glu = glutaric acid]. We have performed the theoretical analysis of H-bonding networks involving the lattice water molecules, the perchlorate anions and the trinuclear cobalt(III/II/III) Schiff base complex and compared the strength of the H-bonds.
Experimental section
Materials
All chemicals were of reagent grade and used as purchased from Sigma-Aldrich without further purification.
Synthesis of [CoII{(μ-L)(μ-Hglu)CoIII(OH2)}2](ClO4)2·6H2O, {H2glu = glutaric acid; H2L = N,N′-bis(salicylidene)-1,3-diaminopropane}
H2L was synthesized as a yellow liquid by refluxing a methanol solution of 1,3-propanediamine (0.2 mL, ∼2 mmol) and salicylaldehyde (0.4 mL, ∼4 mmol) for ca. 1 h. It was not isolated and purified; but was used directly for the synthesis of the complex. A methanol (10 mL) solution of cobalt(II) perchlorate hexahydrate (1.10 g, ∼3 mmol) was added into the methanol solution of the Schiff base, H2L and the mixture was stirred for 1 h. Then a methanol (5 mL) solution of glutaric acid (C5H8O4; 0.264 g, ∼2 mmol) was added to the reaction mixture with constant stirring. Few drops of CH3CN have been added to the solution and the stirring was continued for about 2 h. Diffraction quality dark brown single crystals were obtained after few days on slow evaporation of the solution in open atmosphere.
Yield. 0.80 g (∼60%, based on cobalt), Anal. Calc. for C44H62Co3N4Cl2O28 (FW = 1342.67): C, 39.36; H, 4.65; N, 4.17%; found: C, 39.39; H, 4.63; N, 4.18%. FT-IR (KBr, cm−1): 2960–2926 (υC–H); 1630–1552 (υCOO−); UV-Vis, λmax 590 nm [εmax = 5.0 × 102 L mol−1 cm−1], 356 nm [εmax = 9.0 × 103 L mol−1 cm−1], 239 nm [εmax = 6.7 × 104 L mol−1 cm−1] (CH3CN). Magnetic moment, μ = 5.1 B. M.; crystal system: triclinic.
Instrumentation
The details of different instruments are given in the ESI.† Crystallographic data and refinement details of the complex are given in Table 1. Important bond lengths and angles are listed in Tables 2 and 3, respectively.
Table 1 Crystal data and refinement details of the complex
R1 = Σ‖Fo‖Fc‖/Σ|Fo|. wR2 = Σw((|Fo|2|Fc|2)2/Σw|Fo|2)1/2. |
Formula |
C44H60Co3N4Cl2O27 |
Formula weight |
1324.65 |
Temperature (K) |
293 |
Crystal system |
Triclinic |
Space group |
P![[1 with combining macron]](https://www.rsc.org/images/entities/char_0031_0304.gif) |
a (Å) |
10.4218(4) |
b (Å) |
10.9812(4) |
c (Å) |
12.6764(4) |
α (°) |
104.684(1) |
β (°) |
97.921(1) |
γ (°) |
100.105(1) |
Z |
1 |
dcal (g cm−3) |
1.62244 |
μ (mm−1) |
1.098 |
F(000) |
683 |
Total reflection |
39 408 |
Unique reflections |
4814 |
Observed data [I > 2σ(I)] |
4359 |
R(int) |
0.036 |
aR1, bwR2 (all data) |
0.0471, 0.1101 |
aR1,bwR2 [I > 2σ(I)] |
0.0424, 0.1071 |
Table 2 Selected bond lengths (Å) of the complex
Co(1)–O(1) |
2.085(2) |
Co(1)–O(2) |
2.103(2) |
Co(1)–O(4) |
2.085(2) |
Co(2)–O(1) |
1.924(2) |
Co(2)–O(2) |
1.911(2) |
Co(2)–O(3) |
1.889(2) |
Co(2)–O(5) |
1.920(2) |
Co(2)–N(1) |
1.928(2) |
Co(2)–N(2) |
1.927(3) |
Table 3 Selected bond angles (°) of the complexa
Symmetry transformation, * = −x, 1 − y, −z. |
O(1)–Co(1)–O(2) |
73.34(8) |
O(1)–Co(1)–O(4) |
85.69(8) |
O(2)–Co(1)–O(4) |
85.26(8) |
O(1)–Co(2)–O(2) |
81.43(9) |
O(1)–Co(2)–O(3) |
92.68(9) |
O(1)–Co(2)–O(5) |
92.14(11) |
O(1)–Co(2)–N(1) |
91.39(11) |
O(1)–Co(2)–N(2) |
172.89(10) |
O(2)–Co(2)–O(3) |
92.94(9) |
O(2)–Co(2)–O(5) |
90.16(10) |
O(2)–Co(2)–N(1) |
172.82(11) |
O(2)–Co(2)–N(2) |
91.53(11) |
O(3)–Co(2)–O(5) |
174.61(10) |
O(3)–Co(2)–N(1) |
87.57(11) |
O(3)–Co(2)–N(2) |
86.68(11) |
O(5)–Co(2)–N(1) |
89.91(12) |
O(5)–Co(2)–N(2) |
88.83(12) |
N(1)–Co(2)–N(2) |
95.65(12) |
Computational details
The calculations reported herein were performed using the Turbomole 7.2 program.91 The crystallographic coordinates were used for the calculations of the supramolecular assemblies. We used the crystallographic coordinates for the assemblies because we are interested in evaluating the interactions as they stand in the solid state. The level of theory used for the calculations was RI-BP86 (ref. 92 and 93)-D3 (ref. 94)/def2-TZVP.95,96 The topological analysis of the electron density was carried out according to the quantum theory of atoms in molecules (QTAIM) method proposed by Bader97 and represented using the VMD program.98 They were computed using the MultiWFNprogram99 at the PB86-D3/def2-TZVP level of theory.
Results and discussion
Synthesis
Facile condensation of 1,3-propanediamine with salicylaldehyde in 1
:
2 molar ratio in methanol afforded a Schiff base ligand, H2L (where H indicates dissociable phenolic hydrogen atoms). The Schiff base was, however, not isolated and purified, but it was employed directly as a tetradentate chelating ligand in the present work for the synthesis of the complex. The structure of the ligand used is shown in Scheme 1.
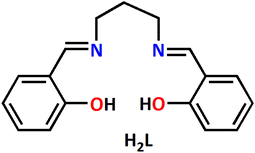 |
| Scheme 1 Schematic representation of the ligand used in the work. | |
The Schiff base, H2L, upon reaction with cobalt(II) perchlorate hexahydrate and glutaric acid in 2
:
3
:
2 ratio in methanol yielded the complex. Slow evaporation of mother liquor in open atmosphere gave brown crystals of the trinuclear mixed-valence cobalt(III/II/III) complex, as authenticated by X-ray structure determinations (vide infra). The synthetic procedure of the complex is shown in Scheme 2.
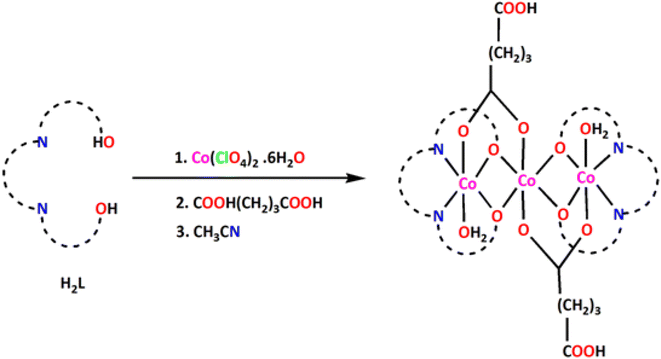 |
| Scheme 2 The synthetic route to the complex. | |
The precursor cobalt(II) perchlorate is partially oxidized by atmospheric oxygen during the course of the reaction. In order to explore the importance of aerial oxidation, the reactions were carried out under nitrogen atmosphere. In this case, we did not get any brown compound, but red coloured compound were isolated. Suitable single crystals of the compound could not be produced, but IR and mass spectral analysis and also elemental analysis indicates the formation of mononuclear cobalt(II) complex.
In the complex, the central cobalt center is assigned as cobalt(II) and both the terminal cobalt centers are assigned as cobalt(III), from BVS calculation and also from bond lengths data (Table 4). The central cobalt(II) is octahedral and is placed in O6 donor environment, whereas the terminal cobalt centers are present in N2O4 donor environment. As oxygen-donor ligands are having relatively weak crystal field, this may be a reason that +2 charges are favoured on this central cobalt. As cobalt(II) is high-spin with t2g5eg2 configuration, whereas cobalt(III) is essentially low-spin with t2g6 electronic configuration, the oxidation of cobalt(II) to cobalt(III) involves the removal of one electron from eg orbital and transfer of another electron from eg to t2g. Both these processes are favoured when the energy gap between t2g and eg is high. Thus, the oxidation of cobalt(II) is preferred in presence of stronger field of N-donor ligands, producing large energy gap between t2g and eg.
Table 4 Result of BVS calculations of the complex
Metal centre |
i–j reported |
r0 |
i–j of this complex |
rij |
sij |
zj |
Co1 |
O–Co2+ |
1.68 |
O(1)–Co(1) |
2.086 |
0.336 |
1.976 |
O–Co2+ |
1.68 |
O(2)–Co(1) |
2.104 |
0.316 |
O–Co2+ |
1.68 |
O(4)–Co(1) |
2.084 |
0.336 |
O–Co2+ |
1.68 |
O(1a)–Co(1) |
2.086 |
0.336 |
O–Co2+ |
1.68 |
O(2a)–Co(1) |
2.104 |
0.316 |
O–Co2+ |
1.68 |
O(4a)–Co(1) |
2.084 |
0.336 |
Co2 |
O–Co2+ |
1.68 |
O(1)–Co(2) |
1.923 |
0.522 |
3.51 |
O–Co2+ |
1.68 |
O(2)–Co(2) |
1.912 |
0.532 |
O–Co2+ |
1.68 |
O(3)–Co(2) |
1.888 |
0.517 |
O–Co2+ |
1.68 |
O(5)–Co(2) |
1.924 |
0.522 |
N–Co2+ |
1.80 |
N(1)–Co(2) |
1.929 |
0.705 |
N–Co2+ |
1.80 |
N(2)–Co(2) |
1.927 |
0.712 |
It may be noted here, the Schiff bases may be reduced with NaBH4 to form the corresponding ‘reduced Schiff base’ ligands. These reduced Schiff bases may also be used to form similar mixed valence cobalt complexes. Recently we have reported the synthesis, structure and application of trinuclear mixed valence cobalt complexes of reduced Schiff bases containing different di-carboxylic acids as bridging co-ligands.100
Description of structure of [CoII{(μ-L)(μ-Hglu)CoIII(OH2)}2](ClO4)2·6H2O
The complex consists of a linear, trinuclear, centrosymmetric, mixed valence cobalt(III)–cobalt(II)–cobalt(III) unit and crystallizes in triclinic space group, P
. The central cobalt centre, Co(1), is in +II oxidation state, and the terminal cobalt centres, Co(2) and its symmetry related counterpart, Co(2)*, are in +III oxidation states [symmetry transformation * = −x, 1 − y, −z]. The perspective view of the complex including the selective atom labeling scheme is shown in Fig. 1. The oxidation states of cobalt(III) and cobalt(II) may be confirmed by charge balance considerations, inter-atomic cobalt-nitrogen and cobalt oxygen distances.In the complex, the central cobalt(II) centre, Co(1), is connected with the terminal cobalt(III) center, Co(2) and its centrosymmetric counterpart, Co(2)* by two carboxylate and four phenolate oxygen bridges forming bis{μ-carboxylato-μ-phenonate} bridged cobalt(III)–cobalt(II)–cobalt(III) motifs, similar to the complexes reported in the literature.89,101 It is interesting to note that two carboxylate bridges are trans in the centrosymmetric trimer, an arrangement observed in similar systems.89,101
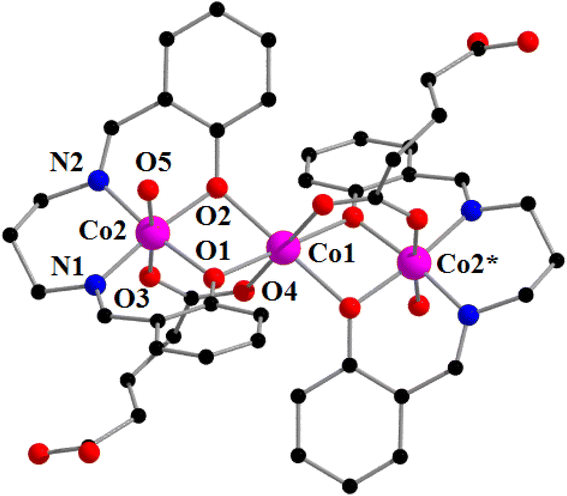 |
| Fig. 1 Perspective view of the complex with selective atom-numbering Scheme. H atoms, perchlorate anions and lattice water molecules have been omitted for clarity. Symmetry transformation, * = −x, 1 − y, −z. Only one set of positions for the disordered –(CH2)3COOH group is shown. | |
The terminal cobalt center, Co(2), has a six-coordinate octahedral environment in which the equatorial plane is occupied by two phenolate oxygen atoms O(1) and O(2), and two imine nitrogen atoms, N(1) and N(2), of the di-Schiff base H2L. The axial positions are occupied by an oxygen atom, O(3), of a bridging glutarate and a water molecule, O(5). Central Co(1), on the other hand, is coordinated by four phenolate oxygen atoms {O(1), O(2), and their symmetry related counter parts, O(1)* and O(2)*} [symmetry transformation * = −x, 1−y, −z] and two oxygen atoms {O(4) and its symmetry related counterpart, O(4)*} of a di-glutarate. Cobalt–cobalt bonding is not expected from the value of the Co(1)⋯Co(2) distance 3.076(3) Å.84,102,103 The bridging angles, ∠Co(1)–O(1)–Co(2) = 100.1(1)0 and ∠Co(1)–O(2)–Co(2) = 99.9(1)0 indicate the non-collinear arrangement of the cobalt(III)–oxygen–cobalt(II) fragments.84,102,103 The cobalt(II)–oxygen, cobalt(III)–oxygen and cobalt(III)–nitrogen distances (Table 2) are in the range 2.085(2)–2.103(2) Å, 1.889(2)–1.924(2) Å and 1.927(2)–1.928(2) Å, respectively, which are equivalent to those found in similar complexes reported in the literature.28,30,89,101 There are four water molecules in the asymmetric unit, one coordinated O(5W) and three uncoordinated. All are involved in strong hydrogen bonds with details given in Table 5. These four water molecules form a network of H-bonds, as highlighted in Fig. 2, where the coordinated water molecule (O5) establishes two OH⋯O contacts with two different lattice water molecules (O1W and O5W). One bridges O5 with the anion and the other bridges O5 with O4 (from the μ-HGlu ligand).
Table 5 List of hydrogen bonding interactions studied in the complex
Atoms involved (D–H⋯A) |
Distance D–H (Å) |
Distance H⋯A (Å) |
Distance D⋯A (Å) |
Angle ∠D–H⋯A (°) |
Symmetry transformation |
O(3W)–H(2W3)⋯O(24B) |
0.82(8) |
1.63(6) |
2.27(1) |
134(10) |
— |
O(5)–H(1W5)⋯O(2W) |
0.85(3) |
1.76(3) |
2.606(4) |
171(4) |
— |
O(5)–H(2W5)⋯O(1W) |
0.83(4) |
1.90(4) |
2.731(6) |
175(4) |
— |
O(1W)–H(2W1)⋯O(51) |
0.84(7) |
2.24(9) |
2.906(7) |
136(6) |
— |
O(2W)–H(2W2)⋯O(4)* |
0.85(4) |
1.94(4) |
2.780(4) |
174(4) |
* = −x, 1 − y, −z |
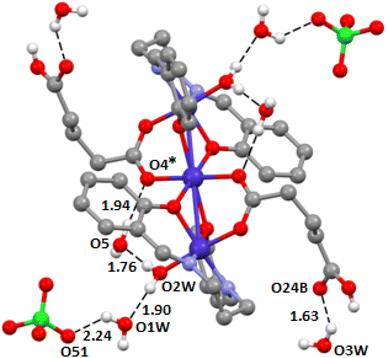 |
| Fig. 2 Perspective view of hydrogen bonding network in the complex involving the coordinated and lattice water molecules as H-bond donor and acceptors. Only the relevant hydrogen atoms have been shown for clarity. Symmetry transformation, * = −x, 1 − y, −z. | |
Theoretical study on supramolecular interaction
The DFT study is focused on one main aspect of the solid state structure of the complex, that is the H-bonding network highlighted in Fig. 2. That has been analyzed using the quantum theory of atoms in molecules. This method not only confirms the existence of the H-bonds, but also provides an estimation of the strength of the interaction via the value of the potential energy density at the bond CP that connect the H-atom to the O-atom. The QTAIM analysis is shown in Fig. 3. Each hydrogen bond is characterized by a Bond Critical Point (BCP, indicated by a small red sphere) and a bond path (depicted as an orange line) linking the hydrogen (from H2O) and oxygen atoms belonging to other water molecules, the μ-HGlu ligand of the perchlorate anion. It is interesting to note that each perchlorate anion establishes two H-bonds, one with the nearby water molecule (−2.5 kcal mol−1) and another one with one CH bond of the aromatic ring (−1.0 kcal mol−1). In general, the H-bonds between water molecules are stronger than those involving the O-atoms of the anion. The strongest H-bond (−11.8 kcal mol−1) correspond to the one that bridges the coordinated water molecule with the coordinated O-atom of the μ-HGlu ligand [O(5)–H(1W5)⋯O(2W)] through a lattice water molecule. The formation energy of the H-bonding network is −63.8 kcal mol−1, which corresponds to the hydrogen bonding interactions shown in Fig. 3. This significant formation energy emphasizes the importance of the H-bonds in the solid state of the complex and also explain the incorporation of the co-crystallized water molecules.
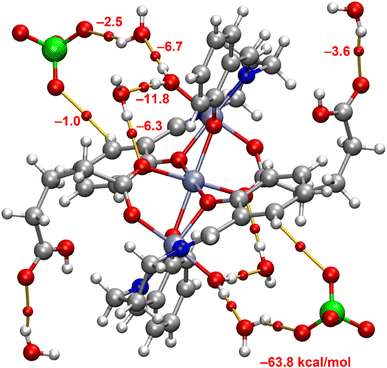 |
| Fig. 3 QTAIM analysis (bond CPs in red and paths as orange lines) for the H-bonding network in the complex. Only H-bonding interactions are represented. The individual energies of the H-bonds were estimated using the equation E = 0.5 V, where V is the potential energy density at the BCP. | |
Hirshfeld surface analysis
Hirshfeld surface analysis is used to analyze the electronic distribution around the surface of a complex which helps in interpreting and visualizing the non-covalent interactions present in the crystal framework. Hirshfeld surfaces of the complex appeared over dnorm (range −0.1 Å to 1.5 Å), shape index and curvedness have been illustrated in Fig. S1.† Red spots on the dnorm Hirshfeld surfaces reveal the positions of strong contacts and interactions. The blue and white zone on the surfaces reveals weaker and longer contacts.
2D fingerprint plot of the complex show major interaction which is present in the form of H⋯H interaction, which constitutes of 45.7% of total surfaces of the complex. The proportion of C⋯H/H⋯C interaction and O⋯H/H⋯O interaction in the complex comprise of 14.4% and 37.6% of the Hirshfeld surface, respectively. The significant O⋯H interaction supports the complex to form a supramolecular 1D polymer. The intermolecular interactions appearing as distinct spikes in the 2D fingerprint plot is given in Fig. 4 (https://www.sciencedirect.com/science/article/pii/S0277538722003916).
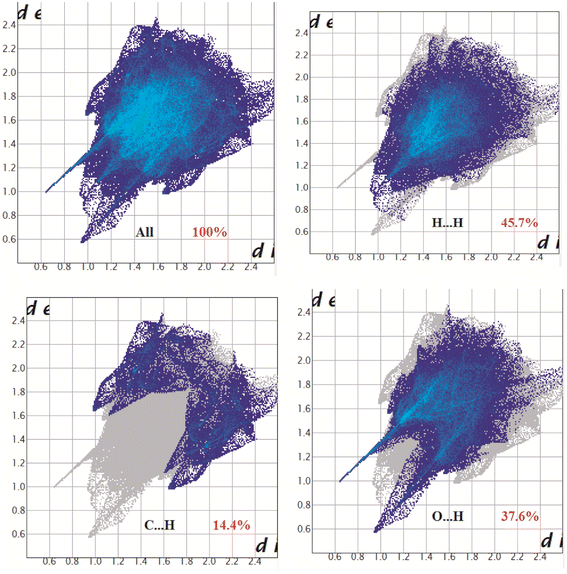 |
| Fig. 4 Fingerprint plot of the complex: full and resolved into H⋯H, C⋯H/H⋯C and O⋯H/H⋯O contacts contributed to the total Hirshfeld surface area. | |
IR and electronic spectra
The bands in the range of 1650–1550 cm−1are due to the presence of bridging carboxylate group.30,84,104 In the IR spectrum of the complex, a distinct band appears around 1600 cm−1 and may be identified as stretching vibration of azomethine (C
N) group.30,105–107 The IR spectrum also shows a weak band appearing in the range of 2930–2880 cm−1 due to stretching vibrations of alkyl C–H group.108–110 The broad band observed around 3250 cm−1 in the IR spectrum is assigned to the O–H stretching vibration.103,109 Two sharp bands around 600 cm−1 and 750 cm−1 are observed due to stretching of the Co–O and Co–N bonds, respectively.111–113 The IR spectrum of the complex is given in Fig. S2.†
Electronic absorption spectrum of the complex in acetonitrile shows an intra-ligand π–π* transitions at ∼240 nm.114–116 A strong ligand to metal charge transfer transition at ∼ 430 nm has been observed which is characteristic of transition metal complexes with Schiff base ligands.84,110 The band around 585 nm may be assigned as one of the expected d–d transitions originated from cobalt(II).30,115,116 The bands corresponding to the electronic transitions from cobalt(III) center are not observed as they are obscured by the transitions from cobalt(II) or by LMCT transitions.84,117,118 The UV-vis spectrum of the complex is given in Fig. S3.†
Magnetic moment
The effective magnetic moment at room temperature of the trinuclear complex is found to be 5.16 BM, which is indicative of the presence of only one high-spin Co(II) center in the molecule. The value is higher than the spin-only value for three unpaired electrons of high spin cobalt(II) with d7 electronic configuration. This may be due to orbital contribution of magnetic moment. The DFT study also supports that the spin density is localized on central cobalt with three unpaired electrons (2.47 e), as represented in Fig. 5, showing some spin delocalization toward the atoms directly bonded to the Co(II) atom.
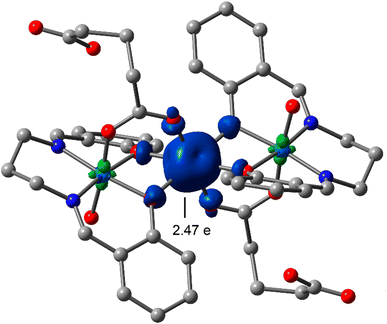 |
| Fig. 5 Spin density plot of the cobalt(III/II/III) Schiff base complex reported in this work at the BP86-D3/def2-TZVP level of theory. The spin density at the Co(II) metal center is given. | |
Conclusion
In conclusion, a new trinuclear, mixed valence, carboxylate bridged, cobalt(III)–cobalt(II)–cobalt(III) complex has been synthesized and characterized. Single crystal X-ray diffraction study has confirmed its structure. Moreover, the complex forms extended H-bonding networks using the Co(III)-coordinated water ligands, the lattice water molecules and the carboxylic acid groups of the μ-HGlu ligands. The energy associated to each H-bond has been estimated and the formation energy of the supramolecular H-bonding network calculated. It is very significant, revealing their strong influence in the crystal packing. We expect that the results reported herein may attract the interest of the crystal engineers and supramolecular chemists working with cobalt Schiff base complexes.
Conflicts of interest
The authors declare no conflict of interest.
Acknowledgements
S. M. and S. B. thanks UGC, India for providing Junior Research Fellowship and Senior Research Fellowship, respectively. A. F. thanks MICIU/AEI (project PID2020-115637GB-I00, FEDER funds) for financial support. We thank the CTI (UIB) for computational facilities.
References
- A. Nishinaga, T. Yamada, H. Fujisawa, K. Ishizaki, H. Ihara and T. Matsuura, J. Mol. Catal., 1988, 48, 249–264 CrossRef CAS
. - C. Christmas, J. B. Vincent, H. R. Chang, J. C. Huffman, G. Christou and D. N. Hendrickson, J. Am. Chem. Soc., 1988, 110, 823–830 CrossRef CAS
. - A. Harton, M. K. Nagi, M. M. Glass, P. C. Junk, J. L. Atwood and J. B. Vincent, Inorg. Chim. Acta, 1994, 217, 171–179 CrossRef CAS
. - Biomimetic Oxidations Catalyzed by Transition Metals, ed. B. Meunier, Imperial College Press, London, 2000 Search PubMed
. - R. D. Cannon and R. P. White, Prog. Inorg. Chem., 1988, 36, 195–298 CrossRef CAS
. - S. J. Lippard and J. M. Berg, Principles of Bioinorganic Chemistry, University Science Books, Mill Valley, California, 1994 Search PubMed
. - D. Gatteschi and R. Sessoli, Angew. Chem., 2003, 115, 278–309 CrossRef
. - R. H. Holm, P. Kennepohl and E. I. Solomon, Chem. Rev., 1996, 96, 2239–2314 CrossRef CAS PubMed
. - E. I. Solomon, M. J. Baldwin and M. D. Lowery, Chem. Rev., 1992, 92, 521–542 CrossRef CAS
. - R. E. P. Winpenny, Adv. Inorg. Chem., 2001, 52, 1–11 CrossRef CAS
. - C. Boskovic, E. K. Brechin, W. E. Streib, K. Folting, J. C. Bollinger, D. N. Hendrickson and G. Christou, J. Am. Chem. Soc., 2002, 124, 3725–3736 CrossRef CAS PubMed
. - H. Andres, R. Basler, H. U. G. Del, G. Arom, G. Christou, H. B. Ttner and B. Ruffl, J. Am. Chem. Soc., 2000, 122, 12469–12477 CrossRef CAS
. - R. Sessoli, H.-L. Tsai, A. R. Schake, S. Wang, J. B. Vincent, K. Folting, D. Gatteschi, G. Christou and D. N. Hendrickson, J. Am. Chem. Soc., 1993, 115, 1804–1816 CrossRef CAS
. - L. Chun and C. R. Kagan, J. Am. Chem. Soc., 2003, 125, 336–337 CrossRef PubMed
. - H. E. Toma, J. Braz. Chem. Soc., 2003, 14, 845–869 CrossRef CAS
. - A. Bousseksou, G. Molnar, P. Demont and J. Menegotto, J. Mater. Chem., 2003, 13, 2069–2071 RSC
. - E. Coronado, J. R. Galan-Mascaros, C. J. Gomez-Garcia and V. Laukhin, Nature, 2000, 408, 447–449 CrossRef CAS PubMed
. - J. S. Miller, Inorg. Chem., 2000, 39, 4392–4408 CrossRef CAS
. - S. K. Dey and A. Mukherjee, Coord. Chem. Rev., 2016, 310, 80–115 CrossRef CAS
. - S. K. Dey and A. Mukherjee, New J. Chem., 2014, 38, 4985–4995 RSC
. - A. Mukherjee, I. Rudra, S. G. Naik, S. Ramasesha, M. Nethaji and A. R. Chakravarty, Inorg. Chem., 2003, 42, 5660–5668 CrossRef CAS PubMed
. - S. Roy, S. Halder, K. Harms, P. P. Ray and S. Chattopadhyay, New J. Chem., 2020, 44, 1285–1293 RSC
. - K. Ghosh, S. Silb, P. P. Ray, J. Ortega-Castroc, A. Frontera and S. Chattopadhyay, RSC Adv., 2019, 9, 34710–34719 RSC
. - O. B. Ona, D. R. Alcoba, G. E. Massaccesi, A. Torre, L. Lain, J. I. Melo, J. M. Oliva-Enrich and J. E. Peralta, Inorg. Chem., 2019, 58(4), 2550–2557 CrossRef CAS PubMed
. - B. Ozcelik, D. Yazici and A. Ekicibil, J. Supercond. Novel Magn., 2013, 26, 1599–1605 CrossRef
. - K. Katayama, M. Hirotsu, I. Kinoshita and Y. Teki, Dalton Trans., 2014, 43, 13384–13391 RSC
. - D. Kowalkowska-Zedler, A. Dołęga, N. Nedelko, R. Łyszczek, P. Aleshkevych, I. Demchenko, J. Łuczak, A. Ślawska-Waniewska and A. Pladzyk, Dalton Trans., 2020, 49, 697–710 RSC
. - S. Chattopadhyay, M. G. B. Drew and A. Ghosh, Eur. J. Inorg. Chem., 2008, 1693–1701 CrossRef CAS
. - T. G. Dastidar and S. Chattopadhyay, Polyhedron, 2022, 211, 115511 CrossRef CAS
. - S. Chattopadhyay, G. Bocelli, A. Musatti and A. Ghosh, Inorg. Chem. Commun., 2006, 9, 1053–1057 CrossRef CAS
. - M. Valko, R. Klement, P. Pelikán, R. Boéa, L. Dlháñ, A. Bottcher, H. Elias and L. Müller, J. Phys. Chem., 1995, 99, 137–143 CrossRef CAS
. - R. M. Clarke and T. Storr, Dalton Trans., 2014, 43, 9380–9391 RSC
. - J. J. R. Frasto da Silva and R. J. P. Williams, The Biological Chemistry of the Elements, Clarendon Press, Oxford, 1997 Search PubMed
. - L. G. Marzilli, M. F. Summers, N. Bresciani-Pahor, E. Zangrando, J. P. Charland and L. Randaccio, J. Am. Chem. Soc., 1985, 107, 6880–6888 CrossRef CAS
. - M. F. Summers, L. G. Marzilli, N. Bresciani-Pahor and L. Randaccio, J. Am. Chem. Soc., 1984, 106, 4478–4485 CrossRef CAS
. - M. Calligaris, G. Nardin and L. Randaccio, Coord. Chem. Rev., 1972, 7, 385–403 CrossRef CAS
. - T. Kuroda-Sowa, M. Lamm, A. L. Rheingold, C. Frommen, W. M. Reitt, M. Nakano, J. Yoo, A. L. Maniero, L.-C. Brunel, G. Christou and D. N. Hendrickson, Inorg. Chem., 2001, 40, 6469–6480 CrossRef CAS PubMed
. - K. Ghosh, K. Harms and S. Chattopadhyay, Polyhedron, 2017, 123, 162–175 CrossRef CAS
. - K. Ghosh, S. Roy, A. Ghosh, A. Banerjee, A. Bauzá, A. Frontera and S. Chattopadhyay, Polyhedron, 2016, 112, 6–17 CrossRef CAS
. - K. Ghosh, M. G. B. Drew and S. Chattopadhyay, Inorg. Chim. Acta, 2018, 482, 23–33 CrossRef CAS
. - K. Ghosh, K. Harms, A. Franconetti, A. Frontera and S. Chattopadhyay, J. Organomet. Chem., 2019, 883, 52–64 CrossRef CAS
. - K. Ghosh and S. Chattopadshyay, Polyhedron, 2019, 170, 495–507 CrossRef CAS
. - A. Hazari, A. Das, P. Mahapatra and A. Ghosh, Polyhedron, 2017, 134, 99–106 CrossRef CAS
. - K. Ghosh, A. Banerjee, A. Bauzá, A. Frontera and S. Chattopadhyay, RSC Adv., 2018, 8, 28216–28237 RSC
. - K. Ghosh, K. Harms and S. Chattopadhyay, ChemistrySelect, 2017, 2, 8207–8220 CrossRef CAS
. - W. D. Horrocks Jr. and D. R. Sudnick, J. Am. Chem. Soc., 1979, 101, 334–340 CrossRef
. - P. Ball, Chem. Rev., 2008, 108, 74–108 CrossRef CAS PubMed
. - L. J. Barbour, G. W. Orr and J. L. Atwood, Nature, 1998, 393, 671–673 CrossRef CAS
. - S. K. Pal and A. H. Zewail, Chem. Rev., 2004, 104, 2099–2124 CrossRef CAS PubMed
. - M. H. Mir and J. J. Vittal, Angew. Chem., Int. Ed., 2007, 46, 5925–5928 CrossRef CAS PubMed
. - Z. Sun, C.-K. Siu, O. P. Balaj, M. Gruber, V. E. Bondybey and M. K. Beyer, Angew. Chem., Int. Ed., 2006, 45, 4027–4030 CrossRef CAS PubMed
. - B. Roux and R. MacKinnon, Science, 1999, 285, 100–102 CrossRef CAS PubMed
. - K. Mitsuoka, K. Murata, T. Walz, T. Hirai, P. Agre, J. B. Heyman, A. Engel and Y. Fujiyoshi, J. Struct. Biol., 1999, 128, 34–43 CrossRef CAS PubMed
. - R. Ludwig, Angew. Chem., Int. Ed., 2001, 40, 1808–1827 CrossRef CAS PubMed
. - M. Mascal, L. Infantes and J. Chisholm, Angew. Chem., Int. Ed., 2006, 45, 32–36 CrossRef CAS PubMed
. - J. M. Ugalde, I. Alkorta and J. Elguero, Angew. Chem., Int. Ed., 2000, 39, 717–721 CrossRef CAS PubMed
. - L.-S. Long, Y.-R. Wu, R.-B. Huang and L.-S. Zheng, Inorg. Chem., 2004, 43, 3798–3800 CrossRef CAS PubMed
. - M. Zuhayra, W. U. Kampen, E. Henze, Z. Soti, L. Zsolnai, G. Huttner and F. Oberdorfer, J. Am. Chem. Soc., 2006, 128, 424–425 CrossRef CAS PubMed
. - B.-H. Ye, B.-B. Ding, Y.-Q. Weng and X.-M. Chen, Inorg. Chem., 2004, 43, 6866–6868 CrossRef CAS PubMed
. - A. Michaelides, S. Skoulika, E. G. Bakalbassis and J. Mrozinski, Cryst. Growth Des., 2003, 3, 487–492 CrossRef CAS
. - X.-Q. Song, W. Dou, W.-S. Liu and J.-X. Ma, Inorg. Chem. Commun., 2007, 10, 419–422 CrossRef CAS
. - Z. M. Wilseck and R. L. LaDuca, Inorg. Chem. Commun., 2011, 14, 706–710 CrossRef CAS
. - A. Wutkowski, C. Näther and W. Bensch, Inorg. Chim. Acta, 2011, 379, 16–22 CrossRef CAS
. - M. Tadokoro, S. Fukui, T. Kitajima, Y. Nagao, S. Ishimaru, H. Kitagawa, K. Isobe and K. Nakasuji, Chem. Commun., 2006, 1274–1276 RSC
. - W. H. Zhu, Z.-M. Wang and S. Gao, Dalton Trans., 2006, 765–768 RSC
. - J. Moorthy, R. Natarajan and P. Venugopalan, Angew. Chem., Int. Ed., 2002, 41, 3417–3420 CrossRef CAS PubMed
. - R. Custelcean, C. Afloroaei, M. Vlassa and M. Polverejan, Angew. Chem., Int. Ed., 2000, 39, 3094–3096 CrossRef CAS PubMed
. - J. Wang, L.-L. Zheng, C.-J. Zheng, Y.-Z. Li and M.-L. Tong, Cryst. Growth Des., 2006, 6, 357–359 CrossRef CAS
. - K. M. Park, R. Kuroda and T. Iwamoto, Angew. Chem., Int. Ed. Engl., 1993, 32, 884–887 CrossRef
. - S. K. Ghosh and P. K. Bharadwaj, Inorg. Chem., 2004, 43, 5180–5182 CrossRef CAS PubMed
. - S. K. Ghosh and P. K. Bharadwaj, Inorg. Chem., 2003, 42, 8250–8254 CrossRef CAS PubMed
. - X.-M. Zhang, R.-Q. Fang and H.-S. Wu, Cryst. Growth Des., 2005, 121, 1335–1337 CrossRef
. - W. Blanton, S. Gordon-Wylie, G. Clark, K. Jordon, J. Wood, U. Geiser and T. Collins, J. Am. Chem. Soc., 1999, 121, 3551–3552 CrossRef CAS
. - J. Atwood, L. Barbour, T. Ness, C. Ratson and P. Ratson, J. Am. Chem. Soc., 2001, 123, 7192–7193 CrossRef CAS PubMed
. - R. J. Doedens, E. Yohannes and M. I. Khan, Chem. Commun., 2002, 62–63 RSC
. - B. Sreenivasulu and J. Vittal, Angew. Chem., Int. Ed., 2004, 43, 5769–5772 CrossRef CAS PubMed
. - B. Zhao, P. Cheng, X. Chen, C. Cheng, W. Shi, D. Liao, S. Yan and Z. Jiang, J. Am. Chem. Soc., 2004, 126, 3012–3013 CrossRef CAS PubMed
. - R. J. Forster and T. E. Keyes, Coord. Chem. Rev., 2009, 253, 1833–1853 CrossRef CAS
. - S. Scheiner, Noncovalent Forces, Springer, 2015 Search PubMed
. - S. K. Dey and G. Das, Cryst. Growth Des., 2010, 10, 754–760 CrossRef CAS
. - C. A. Bessel, R. F. See, D. L. Jameson, M. R. Churchill and K. J. Takeuchi, J. Chem. Soc., Dalton Trans., 1992, 3223–3228 RSC
. - K. F. Bowes, I. P. Clark, J. M. Cole, M. Gourlay, A. M. E. Griffin, M. F. Mahon, L. Ooi, A. W. Parker, P. R. Raithby, H. A. Sparkes and M. Towrie, CrystEngComm, 2005, 7, 269–275 RSC
. - P. K. Bhaumik, S. Jana and S. Chattopadhyay, Inorg. Chim. Acta, 2012, 390, 167–177 CrossRef CAS
. - K. Ghosh, K. Harms, A. Bauzá, A. Frontera and S. Chattopadhyay, CrystEngComm, 2018, 20, 7281–7292 RSC
. - P. K. Bhaumik, K. Harms and S. Chattopadhyay, Polyhedron, 2014, 68, 346–356 CrossRef CAS
. - S. Thakur, M. G. B. Drew, A. Franconetti, A. Frontera and S. Chattopadhyay, RSC Adv., 2019, 9, 4789–4796 RSC
. - S. Thakur, S. Roy, A. Bauzáb, A. Frontera and S. Chattopadhyay, Inorg. Chim. Acta, 2017, 467, 212–220 CrossRef CAS
. - A. D. Burrows, C. Wing Chan, M. M. Chowdhry, J. E. McGrady, D. Michaeland and P. Mingos, Chem. Soc. Rev., 1995, 24, 329–339 RSC
. - K. Ghosh, S. Banerjee and S. Chattopadhyay, CrystEngComm, 2019, 21, 6026–6037 RSC
. - S. Bhunia and S. Chattopadhyay, Results Chem., 2023, 5, 100701 CrossRef CAS
. - R. Ahlrichs, M. Bär, M. Häser, H. Horn and C. Kölmel, Chem. Phys. Lett., 1989, 162, 165–169 CrossRef CAS
. - A. D. Becke, Phys. Rev. A, 1988, 38, 3098–3100 CrossRef CAS PubMed
. - J. P. Perdew, P. Ziesche and H. Eschrig, Electronic Structure of Solids, AkademieVerlag, Berlin, 1991, vol. 91, p. 11 Search PubMed
. - S. Grimme, J. Antony, S. Ehrlich and H. Krieg, J. Chem. Phys., 2010, 132, 154104 CrossRef PubMed
. - F. Weigend and R. Ahlrichs, Phys. Chem. Chem. Phys., 2005, 7, 3297–3305 RSC
. - F. Weigend, Phys. Chem. Chem. Phys., 2006, 8, 1057–1065 RSC
. - R. F. W. Bader, Chem. Rev., 1991, 91, 893–928 CrossRef CAS
. - W. Humphrey, A. Dalke and K. Schulten, J. Mol. Graphics, 1996, 14, 33–38 CrossRef CAS PubMed
. - T. Lu and F. Chen, J. Comput. Chem., 2012, 33, 580–592 CrossRef CAS PubMed
. -
(a) S. Bhunia, R. M. Gomila, A. Frontera and S. Chattopadhyay, Inorg. Chim. Acta, 2023, 547, 121324 CrossRef CAS
;
(b) S. Bhunia, P. Das, S. Banerjee, R. M. Gomila, M. G. B. Drew, A. Frontera, P. P. Roy and S. Chattopadhyay, New J. Chem., 2023, 47, 14202–14216 RSC
;
(c) S. Bhunia, R. M. Gomila, A. Frontera and S. Chattopadhyay, Polyhedron, 2022, 223, 115910 CrossRef CAS
. - X. He, C.-Z. Lu and C.-D. Wu, J. Coord. Chem., 2006, 59, 977–984 CrossRef CAS
. - C. Fukuhara, E. Asato, T. Shimoji and K. Katsura, J. Chem. Soc., Dalton Trans., 1987, 1305–1311 RSC
. -
(a) A. Hazari, L. K. Das, R. M. Kadam, A. Bauza, A. Frontera and A. Ghosh, Dalton Trans., 2015, 44, 3862–3876 RSC
;
(b) S. Banerjee, M. Nandy, S. Sen, S. Mandal, G. M. Rosair, A. M. Z. Slawin, C. J. G. Garcia, J. M. Clemente-Juan, E. Zangrando, N. Guidolin and S. Mitra, Dalton Trans., 2011, 40, 1652–1661 RSC
. - M. A. S. Goher and F. A. Mautner, J. Chem. Soc., Dalton Trans., 1999, 1535–1536 RSC
. - A. Bhattacharyya and S. Chattopadhyay, RSC Adv., 2015, 5, 18252–18257 RSC
. - T. Basak, A. Frontera and S. Chattopadhyay, CrystEngComm, 2020, 22, 5731–5742 RSC
. - S. Roy, S. Halder, M. G. B. Drew, P. P. Ray and andS. Chattopadhyay, New J. Chem., 2018, 42, 15295–15305 RSC
. - S. Bhunia, M. Das, S. Banerjee, M. G. B. Drew, P. P. Ray and S. Chattopadhyay, RSC Adv., 2024, 14, 11185–11196 RSC
. - S. Roy, A. Dey, M. G. B. Drew, P. P. Ray and S. Chattopadhyay, New J. Chem., 2019, 43, 5020–5031 RSC
. - A. Banerjee, D. Das, P. P. Ray, S. Banerjee and S. Chattopadhyay, Dalton Trans., 2021, 50, 1721–1732 RSC
. - E. A. A. Aboelazm, G. A. M. Ali and K. F. Chong, Chem. Adv. Mater., 2018, 3(4), 67–74 Search PubMed
. - O. A. Fouad, S. A. Makhlouf, G. A. M. Ali and A. Y. El-Sayed, Mater. Chem. Phys., 2011, 128, 70–76 CrossRef CAS
. - C.-W. Tang, C.-B. Wang and S.-H. Chien, Thermochim. Acta, 2008, 473, 68–73 CrossRef CAS
. - A. Saha, P. Majumdar and S. Goswami, J. Chem. Soc., Dalton Trans., 2000, 1703–1708 RSC
. - A. Banerjee, S. Banerjee, C. J. G. Garcia, S. Benmansour and S. Chattopadhyay, ACS Omega, 2019, 4, 20634–20643 CrossRef CAS PubMed
. - K. Ghosh, K. Harms, A. Bauzá, A. Frontera and S. Chattopadhyay, Dalton Trans., 2018, 47, 331–347 RSC
. - A. Banerjee, S. Banerjee, C. J. G. Garcia, S. Benmansour and S. Chattopadhyay, Dalton Trans., 2020, 49, 16778–16790 RSC
. - A. Banerjee, A. Frontera and S. Chattopadhyay, Dalton Trans., 2019, 48, 11433–14447 RSC
.
Footnote |
† Electronic supplementary information (ESI) available: Details of instruments used in the work and Fig. S1–S3. CCDC 2259856. For ESI and crystallographic data in CIF or other electronic format see DOI: https://doi.org/10.1039/d3ra07697k |
|
This journal is © The Royal Society of Chemistry 2024 |