DOI:
10.1039/D4QI01874E
(Research Article)
Inorg. Chem. Front., 2024,
11, 7872-7885
Al-alkyl borate salt cocatalysts for olefin polymerization: exploration of N-donor ligand variations†
Received
26th July 2024
, Accepted 14th September 2024
First published on 18th September 2024
Abstract
The well-defined Al-alkyl borate (AAB) salt {[iBu2(PhNMe2)Al]2(μ-H)}+[B(C6F5)4]− (AlHAl) has been recently identified as a promising “complete” cocatalyst for olefin polymerization. Herein, we explore structural variations of AlHAl obtained by replacing the PhNMe2 (DMA) donor with a variety of anilines, amines, and N-heterocycles. Of the 18 investigated N-donors, twelve provided stable AAB salts; these were tested as cocatalysts in ethylene/1-hexene copolymerization with an archetypical metallocene catalyst. In the other six cases, the side reactions were thoroughly analyzed by NMR spectroscopy. For instance, addition of an o-Me substituent on the DMA ligand triggers C–H activation leading to five-membered cyclometalated species; increasing the steric bulk directly at the N-donor atom leads to tricoordinate mononuclear Al-alkyl cations, which could be isolated, fully characterized and tested in polymerization when using PhNEt2 (DEA). The cocatalytic performance of aniline- and amine-based systems varies only marginally with respect to the benchmark AlHAl_DMA. N-heterocyclic AAB salts perform worse; only the two least electron donating donors, namely difluoropyridine (Py-3,5-F) and quinoline (QUI), provide noticeable productivity. A simple quantitative structure–activity relationship, correlating the steric bulk and stabilizing ability of the N-donor with productivity (R2 = 0.88), has been identified.
Introduction
Molecular olefin polymerization catalysis has tremendously progressed over the last few decades. Initially considered of mere academic interest, nowadays it is of great industrial relevance for the production of several, added-value polyolefin grades.1 The key to success has been the identification of suitable precatalyst/cocatalyst combinations providing highly productive, selective and thermally stable catalytic systems.2,3 Despite the comparable importance of a precatalyst and cocatalyst, these two components have evolved at very different paces. The design of ancillary ligand frameworks has been prolific and continues to provide novel high-performing group 4 metallocene and “post-metallocene” catalysts.4,5 In contrast, diversification among cocatalysts is far more limited; the currently most widely used systems are still based on methylaluminoxane (MAO), discovered in the late 1970s,6,7 or binary systems comprising of an aluminum trialkyl (e.g. tri-iso-butylaluminum, TIBAL) and a borate salt of organic Lewis or Brønsted acids (e.g. [Ph3C]+ [B(C6F5)4]−, trityl borate, TTB; [PhMe2NH]+ [B(C6F5)4]−, anilinium borate, AB), discovered in the 1990s.8,9
This discrepancy is due to the complexity of innovating cocatalysts to overcome their main drawbacks.10–19 In the case of MAO, some of the most widely explored optimization strategies include replacing methyl with larger alkyl groups to improve stability and solubility,20–22 and/or using additives like phenols,23–25 AlMe2F26,27 or Lewis bases28–30 to modulate reactivity. However, despite recent advancement,31 the complex and poorly understood structure of this alumoxane hampered rational design and limited optimization, for instance, with respect to minimizing the high Al/M ratios necessary for optimal performance (typically on the order of 103–104; M = group 4 metal).7,15 The well-defined structure of borate salts allows fine tuning of both cation and anion properties,3,8–19 but, also in this case, some main limitations remain like their ability to serve only as alkyl abstractors. This is the reason why an alkylating and scavenging agent like TIBAL is required with these borate activators, while MAO functions as a stand-alone and “complete” cocatalyst.
Recently, our group identified a borate salt containing an unusual homodinuclear Al-alkyl cation stabilized by N,N-dimethylaniline (DMA) ligands and a bridging hydride, namely {[iBu2(PhNMe2)Al]2(μ-H)}+[B(C6F5)4]− (AlHAl, Fig. 1a).32 The coordinately saturated nature of the metal centers makes this Al-alkyl cation relatively easy to synthesize and stable at room temperature under an inert atmosphere, even though it still manifests a marked “latent”33 Lewis acidity. In fact, propene polymerization experiments revealed that this species is a competent cocatalyst for typical group 4 metal based metallocenes and “post-metallocenes”, exhibiting performance comparable to that of established cocatalysts like MAO and borate salts/TIBAL.32,34
 |
| Fig. 1 Structure of AlHAl-type cocatalysts: (a) prototype,32 (b) hydrocarbon soluble analogue,35 and (c) structural variations explored herein (L = neutral N-donor, see Fig. 2). | |
The cocatalytic properties of this novel system are intriguing in several respects. First, like MAO, AlHAl is a “complete” cocatalyst, effectively activating both dimethyl and dichloride precatalysts and serving as an impurity scavenger. Notably, productivities comparable to those obtained with established cocatalysts, at the same catalyst concentration, can be achieved at Al/M ratios of a few tens, which is orders of magnitude lower than those typically needed with organic borate salts/TIBAL (102–103) or MAO (103–104).
Another important feature of AlHAl is its well-defined molecular structure. Recently, we reported a first proof-of-concept regarding the possibility to tune the properties of AlHAl: introduction of long alkyl chains on the DMA ligands allows overcoming one of the main limitations of ionic cocatalysts, that is their very low solubility in the saturated hydrocarbon diluents typically used in industrial solution processes.35 This modified, soluble cocatalyst (s-AlHAl in Fig. 1b) could be employed successfully in combination with a representative zirconocene in ethylene/1-hexene copolymerization at 100 °C in an alkane diluent, providing a catalytic performance comparable to those of established cocatalysts adapted for the same purpose.
Herein, we report a broader study on the structural modification of AlHAl, aiming at expanding this family of well-defined Al-alkyl borate (AAB) salt cocatalysts. In particular, we explored the possibility of replacing the DMA ligands with a variety of other N-donors (AlHAl_L, Fig. 1c) and evaluated the effect of structural modifications on the properties of these systems by spectroscopic, polymerization and computational studies.
Results and discussion
Synthesis and characterization
The 18 N-donors (L; Fig. 2) studied were selected from commercially available compounds to explore steric and electronic properties over three classes of molecules, namely anilines, amines, and N-heterocycles. Initially, the synthetic approach previously optimized for AlHAl_DMA was utilized (Scheme 1).32,35 The reaction of equimolar amounts of TIBAL, N-donor and the borate salt of the protonated N-donor provides the mononuclear intermediate Al_L; iso-butane is the only (gaseous) byproduct. Al_L is subsequently converted into dinuclear AlHAl_L by addition of 1 eq. of di-iso-butylaluminum hydride (DIBAL-H; Scheme 1a); the mono- and dinuclear species are in dynamic equilibrium,32 as further discussed in the following sections. Alternatively, the same Al_L intermediate can be obtained by reaction of TIBAL with two equivalents of N-donor and one equivalent of TTB (Scheme 1b). Although this approach is less atom-efficient, producing two byproducts, iso-butene and triphenylmethane, it can be employed for N-donors where the borate salt is not commercially available or easily synthesizable. Pure Al_L is then obtained by removing iso-butene in vacuo and triphenylmethane by washing with pentane. Both these approaches were employed depending on the specific properties of each N-donor.
 |
| Fig. 2 N-donor ligands studied (L). | |
 |
| Scheme 1 Synthetic procedures explored for AlHAl_L, inspired by those previously optimized for AlHAl_DMA.32 DIBAL-H is shown as a monomer for simplicity. | |
Anilines and amines: successful attempts.
Along with previously reported DMA32 and DMA-C16,35 the synthetic protocols depicted in Scheme 1 were successfully applied to DMA-Ph, DMA-Cl, DMCA and DMHA. Since the anilinium/ammonium salts of these latter species are not commercially available, the synthetic protocol using TTB (Scheme 1b) was used in most cases. Only for DMHA, synthesizing the ammonium salt36 and following the route of Scheme 1a is indicated; the long hexadecyl chain of this amine renders Al_DMHA highly soluble in pentane, precluding efficient removal of the Ph3CH byproduct (route b).
Characterization of reaction products by NMR spectroscopy indicates that the structural properties of AlHAl_L are only marginally affected by donor variation (see the ESI for details†). The NMR spectra of the representative AlHAl_DMA-Ph are very similar to those reported previously for AlHAl_DMA,32 the main difference being the additional signals due to the p-phenyl substituents (Fig. S37†).
Quantitative 1H NMR analysis of equilibrium constants (Keq) and corresponding Gibbs free energies (ΔG) for the reaction:
| Al\_L + [AliBu2H]3 ⇄ AlHAl\_L + [AliBu2H]2 | (1) |
also indicates only a small effect of N-donor variation on the relative stability of the mono and dinuclear species at 298 K for
L = anilines (entries 1–5,
Table 1). As previously reported for the prototypical
DMA system,
32 the dinuclear species is more stable, and solutions containing up to 90 mol%
AlHAl_L can be prepared by properly dosing DIBAL-H (see the ESI
†). For
L = amines, a similar
Keq is observed with
DMHA (entry 7,
Table 1), even though the reaction between
Al_DMHA and DIBAL-H is not instantaneous and requires approximately 12 h to equilibrate even in the presence of excess DIBAL-H (Fig. S12
†); at these long reaction times, some decomposition products become detectable in the NMR spectra. In the case of
DMCA, no reagents could be detected by NMR spectroscopy, suggesting nearly quantitative conversion to the dinuclear product (
i.e. Keq > 23; entry 6,
Table 1).
Table 1 Equilibrium constants (Keq) and corresponding Gibbs free energy differences (ΔG) for the reaction reported in eqn (1)
a
Entry |
L
|
Solvent |
K
eq
|
ΔG (kcal mol−1) |
Ref. |
By quantitative 1H NMR spectroscopy at 298 K.
Limited stability (see main text).
No accurate absolute values could be determined as the concentration of some relevant species is below detection limits of NMR. C7D8 = toluene-d8, C6D5Cl = chlorobenzene-d5.
This work.
|
1 |
DMA
|
C7D8 |
12 |
−1.5 |
32
|
2 |
DMA
|
C6D5Cl |
12 |
−1.5 |
|
3 |
DMA-C16
|
C7D8 |
23 |
−1.9 |
35
|
4 |
DMA-Ph
|
C7D8 |
11 |
−1.4 |
|
5 |
DMA-Cl
|
C6D5Cl |
11 |
−1.4 |
|
6 |
DMCA
|
C6D5Cl |
>23c |
<−1.9 |
|
7 |
DMHA
|
C7D8 |
13 |
−1.5 |
|
8 |
N-Heterocycles |
C7D8 |
≪1c |
≫0 |
|
Despite considerable efforts, no single crystals suitable for X-ray diffraction could be obtained for AlHAl_L as they precipitate as oils in all cases. The crystal structure could be instead analyzed for mononuclear Al_L with L = DMA and DMA-Cl (Fig. 3a and b). Both species exhibit the expected pseudo-tetrahedral geometry for tetracoordinated Al-complexes, with similar Al–C and Al–N bond lengths (entries 1 and 2, Table 2). The N–Al–N bond angles of ∼106° for Al_DMA and ∼104° for Al_DMA-Cl are close to the 109° angle of ideal tetrahedral geometry, while C–Al–C angles are appreciably higher (>120° in both cases; entries 1 and 2, Table 2) likely due to the steric constrain imparted by the bulky iso-butyl groups. Consistently, the C–Al–C angle reported for the methyl analogue of Al_DMA, i.e. [AlMe2(PhNMe2)]+[B(C6F5)4]−, is appreciably smaller and is approximately 115°.37
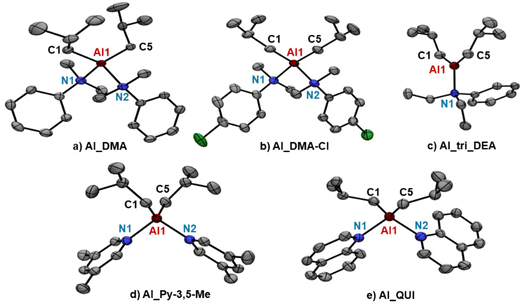 |
| Fig. 3 Ortep drawings of (a) Al_DMA, (b) Al_DMC-Cl, (c) Al_tri_DEA, (d) Al_Py-3,5-Me and (e) Al_QUI crystal structures obtained by single crystal X-ray diffraction. Ellipsoids are drawn at 50% probability level. Hydrogen atoms, [B(C6F5)4]− anion and solvent molecules are omitted for clarity. For further details, including relevant distances (Å) and angles (°), see Table 2 and the ESI.† | |
Table 2 Relevant distances (Å) and angles (°) for the crystal structure of Al_L salts reported in Fig. 3
Entry |
Al_L
|
Al–C1 |
Al–C5 |
Al–N1 |
Al–N2 |
N–Al–N |
C–Al–C |
1 |
Al_DMA
|
1.9760(16) |
1.9733(16) |
2.0660(13) |
2.0877(14) |
106.22(5) |
124.39(7) |
2 |
Al_DMA-Cl
|
1.974(3) |
1.982(4) |
2.075(2) |
2.080(3) |
104.31(11) |
122.39(15) |
3 |
Al_tri_DEA
|
1.9393(16) |
1.9421(16) |
1.9766(14) |
— |
— |
131.21(8) |
4 |
Al_Py-3,5-Me
|
1.962(2) |
1.968(2) |
1.9758(19) |
1.9703(19) |
96.42(8) |
121.91(11) |
5 |
Al_QUI
|
1.958(3) |
1.966(3) |
2.001(3) |
2.000(3) |
99.53(11) |
126.24(15) |
Anilines and amines: side reactions.
Side reactions hampered isolation or even detection of Al_L and AlHAl_L with the other anilines and amines. For instance, the para-substituted aniline DMA_CF3 undergoes C–F bond activation (Scheme 2a), which is known to potentially occur in the presence of Al-based Lewis acids.38,39
 |
| Scheme 2 Side reactions observed with some aniline and amine donors. Exploration of the reactivity of Al_Cyclo1 is reported in the dashed rectangle. DIBAL-H is shown as a monomer for simplicity. | |
Conversely, with the o-Me substituted aniline, Al_DMA-2-Me could be obtained and characterized, even though it is unstable at 298 K and converted quantitatively within a few hours into the neutral cyclometalated species Al_Cyclo1 and the DMA-2-Me anilinium borate salt (Scheme 2b). The identity of Al_Cyclo1 was further confirmed by its independent synthesis via the sequential reaction of DMA-2-Me with BuLi and di-iso-butylaluminum chloride (DIBAL-Cl; Fig. S27†).40,41 The decomposition of Al_DMA-2-Me is likely triggered by the steric hindrance imparted by the o-Me groups of the two aniline ligands. The reaction is reminiscent of directing group enabled C–H activation42 with the hydrogen being transferred to the released base. It is worth noting that, even though Al–alkyl bonds are generally more reactive than Al–aniline ones, C–H activation leading to the cyclometalated species involves only the latter (Scheme 2b); somewhat related observations have been reported for (BHT)2AlMe (BHT = 2,6-di-tert-butyl-4-methylphenolate), in which the first Al–O bond is hydrolyzed more easily than the Al–Me one, likely due to the steric strain imparted by the bulky phenolate ligands.43
The reactivity of isolated Al_Cyclo1 was preliminarily explored by reaction with TTB in the absence and presence of 1 equiv. of DMA-2-Me. In the former case, ionization and rapid (C6F5)-group abstraction from the borate anion are observed (Scheme S1†). In the latter, the reaction provides stable ionic Al_Cyclo1_DMA-2-Me (Scheme 2c), in which the Al center binds two aniline-type fragments and two alkyl groups analogously to Al_DMA-2-Me. Addition of DIBAL-H resulted in a complex and dynamic mixture of unknown products; evidence for complete conversion of o-Me groups into formally anionic methylene moieties was obtained after >24 h (Fig. S30†). Given the complexity of this reactivity and the rather low isolated yields, we decided not to further investigate these species.
With the even bulkier DMA-2,4,6-Me, having two o-Me and one p-Me groups, Al_DMA-2,4,6-Me could not be detected by NMR spectroscopy. Decomposition to the cyclometalated Al_Cyclo2, the borate salt of the donor, and a tricoordinate Al-alkyl cation having a single aniline coordinated to aluminum (Al_tri_DMA-2,4,6-Me, Scheme 2d) was observed. The latter species decomposes slowly to the cyclometalated complex, anilinium borate and a variety of other unknown products.
The clean formation of a tricoordinate species is instead observed with DEA (Scheme 2e), in which steric bulk is increased directly on the aniline N-donor atom and no easy routes to stable 5-membered cyclometalated species are accessible. Al_tri_DEA is stable in the solid form for weeks and decomposes only very slowly in solution over several days via (C6F5)-abstraction (Scheme S2†); it could be therefore thoroughly characterized by NMR spectroscopy and single-crystal X-ray diffraction, showing a nearly planar coordination geometry (Fig. 3c). Al_tri_DEA is one of the very few examples of stable and fully characterized tricoordinate Al-alkyl cations.44–50 No reaction is observed upon adding DIBAL-H to Al_tri_DEA neither in the absence nor presence of an additional DEA equivalent.
The bulkier donor DIPA leads to a detectable but less stable tricoordinate complex Al_tri_DIPA (Scheme 2f), which decomposes over a few hours to a very complex mixture of species: the protonated DIPA borate salt is the most abundant and the only one that could be identified. Similarly, three ethyl groups of TEA appear to be too bulky to allow the formation of a stable Al-alkyl cation (Scheme 2g).
N-heterocycles.
The synthetic route of Scheme 1b was found to be not feasible for N-heterocycles since these strong Lewis bases compete with TIBAL for the reaction with the Lewis acidic TTB (Scheme 3a). To avoid the synthesis of each protonated N-donor borate salt (Scheme 1a), a simple solution consists in replacing TTB with a Brønsted acidic ionizing agent like AB (Scheme 3b), which is less prone to reaction with N-heterocycles. The DMA byproduct of these reactions (Scheme 3b) remains “free” because it is unable to compete with N-heterocycles for coordination at Al, and it can be easily removed by washing with pentane. This approach was successfully applied to the synthesis of Al_L with nearly all N-heterocycles, including Al_Py-4-NMe2 that coordinates to aluminum selectively via the more electron-rich pyridinic nitrogen atom (Fig. S20 and 21†).
 |
| Scheme 3 Synthetic procedures adapted for N-heterocycles. DIBAL-H is shown as a monomer for simplicity. R = Me or F. | |
Major decomposition is observed only with Py-F5, which undergoes C–F bond activation. Hydrodefluorination of Py-F5 with Al/Ga reagents catalyzed by Lewis bases has been previously reported;39,51 interestingly, herein, it is only observed with perfluorinated pyridine and not with Py-3,5-F, containing less activated C–F bonds. In the case of Py-2,6-Me, formation of small amounts (1–2 mol%) of pyridinium borate byproduct is observed, indicative of proton transfer from AB. The resulting N–H+ bond does not react with TIBAL, likely because it is sterically shielded by the two o-Me groups. The pyridinium salt fraction increases upon storing solid Al_Py-2,6-Me over several weeks, suggesting that it is a product of its self-decomposition. It is worth mentioning here that all the other Al_L species prepared here can be stored under an inert atmosphere for at least 1 year without major signs of decomposition.
The crystal structure could be analyzed for Al_L with L = Py-3,5-Me and QUI (Fig. 3d and e). The two species exhibit a similar tetrahedral structure with nearly identical Al–C bond lengths of ∼1.96 Å (entries 4 and 5, Table 2). The Al–N bonds of Al_QUI (∼2.00 Å) are slightly longer than those of Al_Py-3,5-Me (∼1.97 Å), likely reflecting the lower electron donating ability and higher steric bulk of QUI. With respect to the solid state structure of the aniline-based AAB, the main difference emerges in the N–Al–N angle, which is significantly smaller for the N-heterocycles (entries 1 and 2 vs. 4 and 5, Table 2), following the trend Al_Py-3,5-Me (96.42(8)°) < Al_QUI (99.53(11)°) ≪ Al_DMA-Cl (104.31(11)°) < Al_DMA (106.22(5)°). This is likely due to the flat nature of N-heterocycle donors, having sp2 hybridized nitrogen, rendering them less sterically demanding than anilines.
Notably, addition of DIBAL-H to Al_L with N-heterocycles did not provide detectable amounts of AlHAl_L, even when an excess of hydride up to 10 eq. is added (i.e. Keq ≪ 1 for the reaction in eqn (1); entry 8, Table 1; see also the ESI†).52
Polymerization experiments
The performance of the twelve successfully synthesized AAB salts was explored in ethylene/1-hexene copolymerization at 60 °C with a representative ansa-zirconocene catalyst (Cat-Zr, Fig. 4; see the Experimental part for details).53–57 For the donors allowing isolation of both mono- and dinuclear species (i.e. anilines and amines), AlHAl_L was tested since Al_L cannot function as stand-alone cocatalyst.32 Conversely, for N-heterocycles and DEA that only form mononuclear species, an equimolar mixture of Al_L and DIBAL-H was used, since the Al-hydride is an integral part of the cocatalyst.
 |
| Fig. 4
C
2-Symmetric ansa-zirconocene precatalyst used for cocatalyst screening. | |
Based on preliminary experiments with the prototypical AlHAl_DMA (Table S7†), a rather low catalyst concentration of 2.5 × 10−7 M and a [B]/[Zr] ratio of 100 were selected as screening conditions for all cocatalysts. Even lower [B]/[Zr] ratios would suffice to obtain comparable productivity (Table S7†) but, to assure comparability also with less efficient AlHAl_L variations, we used higher cocatalyst loadings. Results are summarized in Table 3.
Table 3 Summary of ethene/1-hexene copolymerization resultsa
Entry |
L
|
R
p
|
Relative Rp c (%) |
M
n d (kDa) |
PDId |
Experimental conditions: ethylene/1-hexene copolymerization in toluene (6 mL), T = 60 °C, pC2 = 4.5 bar (65 psi), t = 10 min, [H]feed = 1.8 v/v%, [H]incorporated ≈ 7 mol%, nCat-Zr = 1.5 nmol, nAlHAl = 150 nmol (i.e. [B]/[Zr] = 100).
Productivity in kgPE mmolCat−1 h−1.
Relative productivity with respect to AlHAl_DMA.
Determined by GPC (∼20% experimental uncertainty). n.a. = not available. Results are averages of at least triplicate experiments.
|
1 |
DMA
|
462 |
100 |
80 |
2.3 |
2 |
DMA-C16
|
401 |
87 |
75 |
2.5 |
3 |
DMA-Ph
|
367 |
79 |
82 |
2.3 |
4 |
DMA-Cl
|
466 |
101 |
86 |
2.5 |
5 |
DEA
|
422 |
91 |
75 |
2.4 |
6 |
DMCA
|
533 |
116 |
75 |
2.5 |
7 |
DMHA
|
267 |
58 |
82 |
2.4 |
8 |
Py-2,6-Me
|
3 |
1 |
n.a. |
n.a. |
9 |
Py-3,5-Me
|
12 |
3 |
82 |
2.3 |
10 |
Py-3,5-F
|
224 |
48 |
83 |
2.3 |
11 |
Py-4-NMe2
|
∼0 |
∼0 |
n.a. |
n.a. |
12 |
QUI
|
95 |
21 |
77 |
2.4 |
The vast majority of AABs yield good productivities in olefin polymerization, showing that AlHAl_DMA is not a unique case of a well-defined AAB cocatalyst. Polymer properties in terms of average molecular weight and comonomer incorporation (∼7 mol% for all samples, as determined by 13C NMR spectroscopy) are not affected by the choice of AAB within experimental uncertainty. This suggests that the same active species is obtained in all cases, albeit with varying efficiency.
Fig. 5 shows a comparison of relative productivities for all AAB with respect to the benchmark AlHAl_DMA. Within the 15% experimental uncertainty estimated for these tests, all the para-substituted N,N-dimethylanilines based cocatalyst provided similarly active systems. Interestingly, Al_tri_DEA/DIBAL-H behaves similar to the other aniline cocatalysts, suggesting that the presence of two aniline ligands around the Al and, more generally, isolation of AlHAl_L are not strictly necessary for effective cocatalytic performance.
 |
| Fig. 5 Relative productivities with respect to that with AlHAl_DMA obtained with Cat-Zr at B/Zr = 100 in combination with various AAB cocatalysts (see Table 3). 15% experimental uncertainty is assumed on Rp. | |
Concerning amine-based systems, the productivity obtained with AlHAl_DMCA is comparable to that with AlHAl_DMA, while AlHAl_DMHA is appreciably worse. In contrast, the N-heterocycle based AABs produce acceptable activator systems only with the least electron donating donors, i.e.Py-3,5-F and QUI. Increasing the [B]/[Zr] ratio to ∼170 provides similar trends (Fig. S38†).
To put results in perspective, we also tested a representative established cocatalyst, namely AB/TIBAL, under the same reaction conditions (Table S7†). At the same catalyst concentration used for AlHAl_L, no detectable activity is observed with the benchmark cocatalyst, signifying the effectiveness of AlHAl_L as both an activator and scavenger. Increasing [Zr] with AB/TIBAL by a factor of ∼7 eventually leads to a productivity comparable to that observed with Py-3,5-F and QUI, indicating that even these AAB salts are moderately good cocatalysts.
Structure–property correlations
By analyzing the results discussed in the previous sections, some considerations can be made regarding structure–property correlations. In terms of stability of Al-L, electronic effects appear marginal within each class of N-donors: for instance, no appreciable difference is observed comparing DMA with other para-substituted anilines, or Py-3,5-Me with Py-3,5-F. Steric effects are instead more relevant: for instance, going from the N-methyl groups of DMA to the N-ethyl groups of DEA destabilizes Al_L dramatically (Scheme 2e). The self-decomposition observed with Py-2,6-Me, but not with its homologue Py-3,5-Me, might also be ascribed to the increased steric hindrance around the N-atom of the former donor.
Regarding the relative stability of Al_L and AlHAl_L (eqn (1) and Table 1), donors belonging to the same class behave similarly, while a pronounced difference is observed across the different classes: anilines and amines have negative ΔG, while N-heterocycles have strongly positive ΔG. The reason for such marked discontinuity is likely rooted in simultaneous electronic and steric changes going from the former two classes to the latter one. The sp2 hybridized nitrogen in N-heterocycles has a much smaller steric footprint than sp3-hybridized anilines and amines (vide supra); concomitantly, N-heterocycles are stronger Lewis bases. Therefore, coordination of two N-heterocycles to Al_L leads to a less crowded, highly stabilized Al cation compared to anilines and amines.
Comparing the results presented in Tables 1 and 3, it becomes evident that the relative stability of Al_L and AlHAl_L does not correlate directly with polymerization performance. For instance, all N-heterocycles exhibit a similar preference for formation of Al_L, while only Py-3,5-F and QUI yield efficient catalytic systems in combination with Cat-Zr.
Trends in Rp are slightly more varied than those in ΔG for eqn (1). Although the variety of the dataset does not suffice to allow thorough statistical analysis of the quantitative structure–activity relationship (QSAR), we embarked on a computational study to analyze the AAB cocatalysts aiming to identify at least a preliminary model.
Al_L species were considered as they are the ones that could be observed experimentally for all the N-donors tested in polymerization. The hexadecyl chains of DMA-C16 and DMHA were modelled with shorter propyl fragments. Given the high flexibility of all these molecules, rotamer/conformer sampling was done with the CREST software suite58,59 and lowest energy structures were then fully optimized at the M06-2X(PCM)/TZ//TPSSh/DZ level (see the Computational part).
A simple quantitative relationship could be readily identified relying on two established molecular descriptors (Fig. 6 and the ESI†):
(1) The buried volume (VBur) imparted by the two N-donor fragments, which is a purely steric descriptor;60
(2) The Wiberg bond index for the weaker Al–CiBu bond, which is a mixed steric and electronic descriptor reflecting the stabilization imparted by the N-donors.61,62
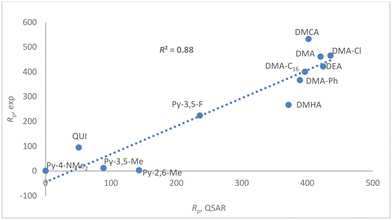 |
| Fig. 6 Plot of experimental vs. calculated Rp based on the proposed QSAR model. | |
The resulting R2 of 0.88 is satisfactory, considering that modelling productivity in olefin polymerization is problematic due to the many subtleties in determining intrinsic activity and fraction of active sites.3,63–66 In the present case, the problem is exacerbated by the fact that N-donors, especially the least sterically hindered and more basic ones, are potential poisons for the strongly electrophilic Zr-active sites.67,68
The proposed model suggests that the bulkier the N-donor and the more covalent the Al–C bond (i.e. the less the Al-alkyl cation is stabilized by the N-donor; see the ESI†), the higher the productivity. This trend likely reflects the “latent” Lewis acidity of the Al-centers. The activating (i.e. ionizing) ability of Al-cocatalysts is generally ascribed to their ability to release unsaturated Al-alkyl cations,32,69–73 which should correspond to a [AliBu2(L)]+ fragment in the present case (Scheme 4).32 It is reasonable to assume that the ease of formation of this unsaturated fragment increases for less stable AAB salts, explaining the trend in productivity.
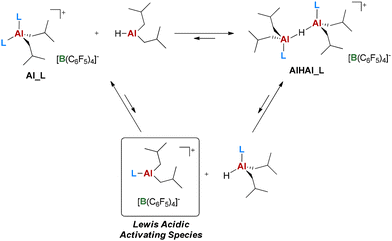 |
| Scheme 4 Proposed mechanism for Al_L and AlHAl_L manifesting their “latent” Lewis acidity by the release of an unsaturated Al-alkyl cation. DIBAL-H is shown as a monomer for simplicity. | |
Conclusion
This work explored the possibility of modifying the structure and reactivity of the recently revealed AlHAl_DMA cocatalyst for olefin polymerization,32,34,35 focusing on variation of its N-donor ligands. Reasonably stable AAB salts could be obtained with twelve of the 18 donors screened, including molecules with polar –F, –Cl and –NMe2 functionalities. Side reactions were observed with the other six donors, including the most sterically hindering ones and those featuring activated C–F bonds. Dinuclear AlHAl_L species are obtained with anilines and amines, while N-heterocycles only form mononuclear Al_L. Polymerization experiments revealed that aniline and amine donors lead to cocatalysts with similar performances to the benchmark AlHAl_DMA, except for DMHA providing lower productivity. Among N-heterocycles, only the two least electron donating donors Py-3,5-F and QUI provided a detectable, moderate productivity. A simple two-descriptors QSAR model suggests that the observed trends in Rp are related to the ease of formation of unsaturated Al-alkyl cations, capable of precatalyst activation, from the formally coordinatively saturated AAB cocatalysts.
These results demonstrate that it is possible to obtain a variety of effective AlHAl-type cocatalysts for olefin polymerization. Despite the relatively limited variability observed, especially among anilines, analysis of degradation routes and a preliminary QSAR model provided important information that might be exploited to design better performing systems. It is worth emphasizing here that Al-alkyl cations are proposed to be essential transient species for the activation of molecular olefin polymerization catalysts:32,69–73 herein, we show how these highly reactive species can be trapped in donor stabilized forms that preserve their activation capability while being amenable to reactivity tuning. In this respect, even toning down activation abilities of molecular activators can be of interest, for instance to prevent overheating and/or extend the lifetime of superactive catalysts74 in high temperature industrial processes; systematic exploration of the thermal stability of AAB salts, so far explored preliminarily only for DMA-C16,35 will provide further insights in this respect. Milder activators could also be exploited to obtain well-defined species for structural and mechanistic studies. We are currently trying to expand the family of well-defined AAB beyond N-containing Lewis bases and to explore the scope of these species in polymerization catalysis and in the broader context of aluminum organometallic chemistry.
Experimental part
Materials and methods
All manipulations were performed under rigorous exclusion of oxygen and moisture in flame-dried Schlenk-type glassware interfaced to a high-vacuum line (<10−5 Torr), or in a nitrogen-filled MBraun glovebox (<0.5 ppm O2). Molecular sieves (4 Å, MS) were activated for 24 h at ca. 200–230 °C under dynamic vacuum. Benzene-d6 and toluene-d8 were freeze–pump–thaw degassed on a high-vacuum line, dried over Na/K alloy, vacuum-transferred to a dry storage-tube with a PTFE valve and stored over activated MS in the glovebox. Chlorobenzene-d5 was freeze–pump–thaw degassed on the high-vacuum line, dried over CaH2, vacuum-transferred to a dry storage-tube with a PTFE valve and stored over activated MS, protected from light, in the glovebox. TTB and AB were obtained from Boulder Scientific Company and used as received. DMA, DMA-2-Me, DMA-2,4,6-Me, DEA, DIPA, DMCA, DMHA, TEA, Py-2,6-Me, Py-3,5-Me, Py-3,5-F, Py-4-NMe2, QUI, 4-aminobiphenyl, potassium carbonate, methyliodide, DIBAL-H, TIBAL, DIBAL-Cl, and BuLi (1.6 M in hexane) were purchased from Merck; DMA-Cl and DMA-CF3 were purchased from ABCR; Py-F5 was purchased from TCI Europe. AlHAl_DMA,32AlHAl_DMA-C1635 and [DMHA-H] +[B(C6F5)4]−36 were synthesized as reported in the literature. Cat-Zr was kindly donated by SABIC. Ethene (Linde, 99.95%) and propene (Rivoira, 99.6%) were purified by flowing them through a column containing activated 4 Å molecular sieves and an activated Cu catalyst (BASF R0-11G). 1-Hexene (Sigma-Aldrich, 99%) was purified by passing it through a mixed-bed column of activated Cu catalyst and 4 Å molecular sieves. Toluene (Romil) was dried using an MBraun SPS-5 solvent purification unit. 1,2-Dichlorobenzene (Romil, >99.8% isomeric purity) was used as received for GPC analysis or purified by passing it through a mixed-bed column of activated Cu catalyst and 4 Å molecular sieves for synthesis purposes.
Synthetic procedures
The synthetic procedures were adapted from those previously reported for Al_DMA and AlHAL_DMA,32,35 by replacing DMA with the other N-donors. The representative synthetic procedures for Al_DMA and AlHAL_DMA are reported below, while details of their characterization are reported in the ESI.† The details on the synthesis and characterization of other relevant species (DMA-Ph,75Al_Cyclo1, Al_Cyclo1_DMA-2-Me, Al_tri_TEA) are reported in the ESI,† as well.
Al_L with L = aniline or amine.
Representative general procedure: Solid TTB (150 mg, 0.16 mmol) was added in small aliquots to a preformed and stirred solution of TIBAL (45 μL, 0.18 mmol) and L donor (0.36 mmol) in toluene (1.5 mL). The resulting mixture was vigorously stirred for 16 h at room temperature. Upon stopping the stirring, a colorless oil precipitated. Pentane (6 mL) was added to facilitate the precipitation of ionic products. The supernatant was then removed, and the oil further washed with pentane (2 × 6 mL). After the last washing, the residue was dried under vacuum to obtain Al_L as a white solid (yield: 91% for DMA; 80% for DMA-C16; 93% for DMA-Ph; 91 for DMA-Cl; 87% for DMCA; 87% for DMHA). For Al_DMA-Cl, dissolving the solid in ortho-difluorobenzene and reprecipitating with pentane might be necessary for effective purification. This is because this species is poorly soluble in toluene and therefore precipitates rapidly as a solid in the reaction medium, trapping byproducts and/or excess reagents in the solid fraction. See the ESI for characterization details.†
AlHAl_L with L = aniline or amine.
Representative general procedure: Al_L (0.14 mmol) was suspended in toluene (1 mL) or dissolved in ortho-difluorobenzene (for Al_DMA-Cl), and DIBAL-H (25 μL, 0.14 mmol) was added. After stirring the resulting mixture for 15 min, pentane (6 mL) was added to facilitate the precipitation of ionic products. The supernatant was then removed, and the oil further washed with pentane (2 × 6 mL). After the last washing, the residue was dried under vacuum to obtain AlHAl_L as a highly viscous, colorless oil (>90% isolated yield in all cases; cf.Table 1). See the ESI for characterization details.†
Al_L with L = N-heterocycles.
Representative general procedure: Solid AB (130 mg, 0.16 mmol) was added in small aliquots to a preformed and stirred solution of TIBAL (45 μL, 0.18 mmol) and L donor (0.36 mmol) in toluene (1.5 mL). The resulting mixture was vigorously stirred for 16 h at room temperature. Upon stopping the stirring, a colorless oil precipitated. Pentane (6 mL) was added to facilitate the precipitation of ionic products. The supernatant was then removed, and the oil further washed with pentane (2 × 6 mL). After the last washing, the residue was dried under vacuum to obtain Al_L as a white solid (yield: 93% for Py-2,6-Me; 95% for Py-3,5-Me and Py-3,5-F; 94% for Py-4-NMe2; 97% for QUI). See the ESI for characterization details.†
NMR spectroscopy experiments
NMR experiments were performed using a Bruker Avance III HD 400 instrument equipped with a smart probe (400 MHz for 1H). 1H NMR spectra were referenced to the residual protons of the deuterated solvent used; 13C NMR spectra were referenced internally to the D-coupled 13C resonances of the NMR solvent. To describe the multiplicity of the signals, the following abbreviations are used: s, singlet; bs, broad singlet; d, doublet; bd, broad doublet; dd, doublet of doublets; t, triplet; m, multiplet.
X-ray diffraction experiments
The X-ray diffraction patterns of crystals were recorded using a Bruker D8 Venture diffractometer equipped with an Incoatec ImuS3.0 microfocus sealed-tube MoKα (λ = 0.71073 Å) source and a CCD Photon II detector. The analysis were carried out at a low temperature (120–150 K range) using an Oxford Cryosystems Cryostream 800 cooler. The data collected through generic φ and ω scans were integrated and reduced using the Bruker AXS V8 Saint Software. The structures were solved and all the thermal parameters were anisotropically refined using the SHELXT and SHELXL packages of the Bruker APEX3 software.
Polymerization experiments
Ethene/1-hexene polymerization experiments were performed in a Freeslate parallel pressure reactor setup with 48 reaction cells (PPR48), fully contained in a triple MBraun glovebox operating under nitrogen. The cells, each with a liquid working volume of 6.0 mL, featured an 800 rpm magnetically coupled stirring, and individual online reading/control of temperature, pressure, monomer uptake, and monomer uptake rate. Experiments were carried out according to established experimental protocols,53–56 under the following experimental conditions: toluene solvent; Tp = 60 °C; pethene = 65 psi (monomer fed on demand); 1-hexene 1.8 v/v%; AlHAl_L activator (nAlHAl = 150 or 250 nmol); Cat-Zr precatalyst (nCatZr = 1.5 nmol); t = 10 min. It is worth mentioning that although AlHAl_L are stable in the form of isolated oils over weeks under an inert atmosphere, freshly assembling them by reaction between equimolar amounts of Al_L and DIBAL-H may be convenient for polymerization experiments: Al_L are solids that can be stored under nitrogen for at least 1 year without noticeable decomposition (unless otherwise stated) and react with DIBAL-H instantaneously. Conversion of 1-hexene was kept below 15% to ensure a constant comonomer concentration. All experiments were performed at least in duplicate. Polymer samples were characterized by gel permeation chromatography (GPC) to determine average molecular weights and molecular weight distributions, and by 13C NMR spectroscopy to determine comonomer incorporation. A detailed experimental procedure is reported in the the ESI.†
Computational details
The chemical space of possible conformers was preliminarily explored using the CREST program.58,59 The (at least) three lowest energy conformers derived from CREST analysis for each molecule were then fully optimized with the Gaussian 16 software package,76 following the protocol described in ref. 77 and 78. All relevant structures were fully optimized in the gas phase at the TPSSh79 level of theory employing correlation-consistent polarized valence double-ζ Dunning(DZ) basis sets (cc-pVDZ quality)80,81 from the EMSL basis set exchange library.82 All calculations were performed using standard Gaussian 16 SCF convergence criteria. Final single-point energies were calculated at the M06-2X level of theory83 employing triple-ζ Dunning (TZ) basis sets (cc-pVTZ quality).84 Solvent corrections were included at this stage by the polarized continuum model (PCM; solvent = toluene).85 The density fitting approximation (resolution of identity, RI) was used at the optimization stage, as well as for final energy calculations.84,86–88 Enthalpies and Gibbs free energies were then obtained from TZ single-point energies and thermal corrections from the TPSSh/DZ vibrational analyses; entropy corrections were scaled by a factor of 0.67 to account for decreased entropy in the condensed phase.89,90 Steric and electronic descriptors were then collected from the lowest energy conformer. IBO iboview61,62 was utilized to extract relevant electronic molecular descriptors from MN15L/SVP calculations employing the IBOviews internal scf routine. SambVca 2.160 was used to determine Vbur and produce topographic steric maps. The default sphere size was chosen (3.5 Å). The aluminum atom was chosen as the center of the sphere, the z-axis was defined using the center between the two Al–C bonds, and the plane was defined using one of the two Al–C atoms.
Data availability
The data supporting this article have been included as part of the ESI. CCDC 2372888–2372892 contain the supplementary crystallographic data for this paper.†
Conflicts of interest
There are no conflicts to declare.
Acknowledgements
This research forms part of the research program of DPI (project 857). Part of this work has been funded by the European Union – NextGenerationEU under the Italian Ministry of University and Research (MUR) National Innovation Ecosystem grant ECS00000041 – VITALITY. We acknowledge Università degli Studi di Perugia and MUR for support within the project Vitality. A. M. and C. Z. thank the “FASTTO0” project (CUP J93C22000330006), funded through the Italian Ministry of Environment and Energy Security. The authors thank Dr Martina Landrini for support with crystallographic determinations. F. Z. thanks the Federico II University of Naples and PON – Ricerca e Innovazione (DM 1062) for a research fellowship.
References
- M. Stürzel, S. Mihan and R. Mülhaupt, From Multisite Polymerization Catalysis to Sustainable Materials and All-Polyolefin Composites, Chem. Rev., 2016, 116, 1398–1433 CrossRef.
- W. Kaminsky, The discovery of metallocene catalysts and their present state of the art, J. Polym. Sci., Part A: Polym. Chem., 2004, 42, 3911–3921 CrossRef CAS.
-
F. Zaccaria, L. Sian, C. Zuccaccia and A. Macchioni, Ion pairing in transition metal catalyzed olefin polymerization, in Advasnces in Organometallic Chemistry, ed. P. J. Perez, Elsevier, Amsterdam, The Netherlands, 2020, vol. 73, pp. 1–78 Search PubMed.
- J. Klosin, P. P. Fontaine and R. Figueroa, Development of group IV molecular catalysts for high temperature ethylene-α-olefin copolymerization reactions, Acc. Chem. Res., 2015, 48, 2004–2016 CrossRef CAS PubMed.
- M. C. Baier, M. A. Zuideveld and S. Mecking, Post-metallocenes in the industrial production of polyolefins, Angew. Chem., Int. Ed., 2014, 53, 9722–9744 CrossRef CAS.
- A. Andresen, H.-G. Cordes, J. Herwig, W. Kaminsky, A. Merck, R. Mottweiler, J. Pein, H. Sinn and H.-J. Vollmer, Halogen–Free Soluble Ziegler Catalysts for the Polymerization of Ethylene. Control of Molecular Weight by Choice of Temperature, Angew. Chem., Int. Ed. Engl., 1976, 15, 630–632 CrossRef.
- H. S. Zijlstra and S. Harder, Methylalumoxane - History, production, properties, and applications, Eur. J. Inorg. Chem., 2015, 2015, 19–43 CrossRef CAS.
- E. Y. X. Chen and T. J. Marks, Cocatalysts for metal-catalyzed olefin polymerization: Activators, activation processes, and structure-activity relationships, Chem. Rev., 2000, 100, 1391–1434 CrossRef CAS.
- M. Bochmann, The chemistry of catalyst activation: The case of group 4 polymerization catalysts, Organometallics, 2010, 29, 4711–4740 CrossRef CAS.
- Y. Gao, M. D. Christianson, Y. Wang, M. P. Coons, J. Chen, J. Zhang, S. Marshall, T. L. Lohr, J. Klosin and T. J. Marks, Alkane-Soluble Bis[tris(alkylphenyl)carbenium] Diborate Cocatalyst for Olefin Polymerizations, ACS Catal., 2022, 12, 7589–7597 CrossRef CAS.
- Y. Gao, J. Chen, Y. Wang, D. B. Pickens, A. Motta, Q. J. Wang, Y. W. Chung, T. L. Lohr and T. J. Marks, Highly branched polyethylene oligomers via group IV-catalysed polymerization in very nonpolar media, Nat. Catal., 2019, 2, 236–242 CrossRef CAS.
- S. Kitphaitun, T. Fujimoto, Y. Ochi and K. Nomura, Effect of Borate Cocatalysts toward Activity and Comonomer Incorporation in Ethylene Copolymerization by Half-Titanocene Catalysts in Methylcyclohexane, ACS Org. Inorg. Au, 2022, 2, 386–391 CrossRef CAS PubMed.
- S. O. Gunther, Q. Lai, T. Senecal, R. Huacuja, S. Bremer, D. M. Pearson, J. C. DeMott, N. Bhuvanesh, O. V. Ozerov and J. Klosin, Highly Efficient Carborane-Based Activators for Molecular Olefin Polymerization Catalysts, ACS Catal., 2021, 11, 3335–3342 CrossRef CAS.
- S. J. Lancaster, A. Rodriguez, A. Lara-Sanchez, M. D. Hannant, D. A. Walker, D. H. Hughes and M. Bochmann, [H2N{B(C6F5)3}2]−: A new, remarkably stable diborate anion for metallocene polymerization catalysts, Organometallics, 2002, 21, 451–453 CrossRef CAS.
- R. Tanaka, Precise control of coordination polymerization via the modification of methylaluminoxane, Polym. J., 2020, 52, 661–670 CrossRef CAS.
-
W. E. Piers, The Chemistry of Perfluoroaryl Boranes, in Advances in Organometallic Chemistry, Academic Press, 2004, vol. 52, pp. 1–76 Search PubMed.
- D. Langford, I. Göttker-Schnetmann, F. P. Wimmer, L. A. Casper, P. Kenyon, R. F. Winter and S. Mecking, Tetrakis[3,5-bis(pentafluorosulfanyl)phenyl]borate: A Weakly Coordinating Anion Probed in Polymerization Catalysis, Organometallics, 2019, 38, 2710–2713 CrossRef CAS.
- V. C. Williams, W. E. Piers, W. Clegg, M. R. J. Elsegood, S. Collins and T. B. Mardell, New bifunctional perfluoroaryl boranes. Synthesis and reactivity of the ortho-phenylene-bridged diboranes 1,2-[B(C6F5)2]2C6X4 (X = H, F), J. Am. Chem. Soc., 1999, 121, 3244–3245 CrossRef CAS.
- Y. X. Chen, C. L. Stern and T. J. Marks, Very large counteranion modulation of cationic metallocene polymerization activity and stereoregulation by a sterically congested (perfluoroaryl) fluoroaluminate, J. Am. Chem. Soc., 1997, 119, 2582–2583 CrossRef CAS.
- H. S. Zijlstra, A. Joshi, M. Linnolahti, S. Collins and J. S. McIndoe, Modifying methylalumoxane via alkyl exchange, Dalton Trans., 2018, 47, 17291–17298 RSC.
- R. Kleinschmidt, Y. Van Der Leek, M. Reffke and G. Fink, Kinetics and mechanistic insight into propylene polymerization with different metallocenes and various aluminium alkyls as cocatalysts, J. Mol. Catal. A: Chem., 1999, 148, 29–41 CrossRef CAS.
- M. Galimberti, M. Destro, O. Fusco, F. Piemontesi and I. Camurati, Ethene/propene copolymerization from metallocene-based catalytic systems: Role of the alumoxane, Macromolecules, 1999, 32, 258–263 CrossRef CAS.
- V. Busico, R. Cipullo, F. Cutillo, N. Friederichs, S. Ronca and B. Wangt, Improving the performance of methylalumoxane: A facile and efficient method to trap ‘free’ trimethylaluminum, J. Am. Chem. Soc., 2003, 125, 12402–12403 CrossRef CAS.
- F. Zaccaria, C. Zuccaccia, R. Cipullo and A. Macchioni, Extraction of Reliable Molecular Information from Diffusion NMR Spectroscopy: Hydrodynamic Volume or Molecular Mass?, Chem. – Eur. J., 2019, 25, 9930–9937 CrossRef CAS.
- F. Zaccaria, C. Zuccaccia, R. Cipullo, P. H. M. Budzelaar, A. Macchioni, V. Busico and C. Ehm, On the Nature of the Lewis Acidic Sites in “TMA-Free” Phenol-Modified Methylaluminoxane, Eur. J. Inorg. Chem., 2020, 2020, 1088–1095 CrossRef CAS.
-
L. Luo, S. A. Sangokoya, W. Xiao, S. P. Diefenbach and B. Kneale, Aluminoxane catalyst activators derived from dialkylaluminum cation precursor agents, processes for making same, and use thereof in catalysts and polymerization of olefins, 2009, Patent Appl, US8575284 Search PubMed.
- L. Oliva, P. Oliva, N. Galdi, C. Pellecchia, L. Sian, A. Macchioni and C. Zuccaccia, Solution Structure and Reactivity with Metallocenes of AlMe2F: Mimicking Cation–Anion Interactions in Metallocenium–Methylalumoxane Inner-Sphere Ion Pairs, Angew. Chem., Int. Ed., 2017, 56, 14227–14231 CrossRef CAS PubMed.
- J. C. W. Chien and D. He, Olefin copolymerization with metallocene catalysts. II. Kinetics, cocatalyst, and additives, J. Polym. Sci., Part A: Polym. Chem., 1991, 29, 1595–1601 CrossRef CAS.
- G. Zanchin, A. Piovano, A. Amodio, F. De Stefano, R. Di Girolamo, E. Groppo and G. Leone, NEt3-Triggered Synthesis of UHMWPE Using Chromium Complexes Bearing Non-innocent Iminopyridine Ligands, Macromolecules, 2021, 54, 1243–1253 CrossRef CAS.
- P. G. Belelli, M. L. Ferreira and D. E. Damiani, Addition of Lewis bases and acids. Effect on α-olefins polymerization with soluble metallocenes, 1 Ethylene, Macromol. Chem. Phys., 2000, 201, 1458–1465 CrossRef CAS.
- L. Luo, J. M. Younker and A. V. Zabula, Structure of methylaluminoxane (MAO): Extractable [Al(CH3)2]+ for precatalyst activation, Science, 2024, 384, 1424–1428 CrossRef PubMed.
- F. Zaccaria, C. Zuccaccia, R. Cipullo, P. H. M. Budzelaar, A. Vittoria, A. Macchioni, V. Busico and C. Ehm, Methylaluminoxane's Molecular Cousin: A Well-defined and “Complete” Al-Activator for Molecular Olefin Polymerization Catalysts, ACS Catal., 2021, 11, 4464–4475 CrossRef CAS.
- C. J. Harlan, A. R. Barron and S. G. Bott, Three-Coordinate Aluminum Is Not a Prerequisite for Catalytic Activity in the Zirconocene—Alumoxane Polymerization of Ethylene, J. Am. Chem. Soc., 1995, 117, 6465–6474 CrossRef CAS.
- G. Urciuoli, F. Zaccaria, C. Zuccaccia, R. Cipullo, P. H. M. Budzelaar, A. Vittoria, C. Ehm, A. Macchioni and V. Busico, Cocatalyst effects in Hf-catalysed olefin polymerization: taking well-defined Al-alkyl borate salts into account, Dalton Trans., 2024, 53, 2286–2293 RSC.
- G. Urciuoli, F. Zaccaria, C. Zuccaccia, R. Cipullo, P. H. M. Budzelaar, A. Vittoria, C. Ehm, A. Macchioni and V. Busico, A Hydrocarbon Soluble, Molecular and “Complete” Al-Cocatalyst for High Temperature Olefin Polymerization, Polymers, 2023, 15, 1378 CrossRef CAS PubMed.
- H.-J. Lee, J.-W. Baek, Y.-H. Seo, H.-C. Lee, S.-M. Jeong, J. Lee, C.-G. Lee and B.-Y. Lee, Preparation of High-Purity Ammonium Tetrakis(pentafluorophenyl)borate for the Activation of Olefin Polymerization Catalysts, Molecules, 2021, 26, 2827 CrossRef CAS PubMed.
- O. Wrobel, F. Schaper and H. H. Brintzinger, Bulky Siloxyaluminum Alkyls as Models for Al2Me6-Treated Silica Gel Surfaces. Characterization of a Dimethylaniline-Stabilized Dimethylaluminum Cation, Organometallics, 2004, 23, 900–905 CrossRef CAS.
- M. Klahn, C. Fischer, A. Spannenberg, U. Rosenthal and I. Krossing, Hydrodefluorination of non-activated C-F bonds by diisobutyl-aluminiumhydride via the aluminium cation [i-Bu2Al]+, Tetrahedron Lett., 2007, 48, 8900–8903 CrossRef CAS.
- A. D. Jaeger, C. Ehm and D. Lentz, Organocatalytic C−F Bond Activation with Alanes, Chem. – Eur. J., 2018, 24, 6769–6777 CrossRef CAS PubMed.
- H. T. Al-Masri, Oxidation reactions, coordination chemistry and antibacterial activities with ligand 2-{(diphenylphosphino) methyl}-N,N-dimethylaniline, J. Organomet. Chem., 2020, 905, 121021 CrossRef CAS.
-
J. T. B. H. Jastrzebski, G. Van Koten, M. F. Lappert, P. C. Blake and D. R. Hankey, Cyclometallated Organolithium Compounds, in Inorganic Syntheses, 1989, pp. 150–155 Search PubMed.
- K. Murali, L. A. Machado, R. L. Carvalho, L. F. Pedrosa, R. Mukherjee, E. N. Da Silva Júnior and D. Maiti, Decoding Directing Groups and Their Pivotal Role in C−H Activation, Chem. – Eur. J., 2021, 27, 12453–12508 CrossRef CAS PubMed.
- R. A. Stapleton, A. Al-Humydi, J. Chai, B. R. Galan and S. Collins, Sterically hindered aluminum alky is: Weakly interacting scavenging agents of use in olefin polymerization, Organometallics, 2006, 25, 5083–5092 CrossRef CAS.
- T. A. Engesser, M. R. Lichtenthaler, M. Schleep and I. Krossing, Reactive p-block cations stabilized by weakly coordinating anions, Chem. Soc. Rev., 2016, 45, 789–899 RSC.
- S. Dagorne, S. Bellemin-Laponnaz and R. Welter, Synthesis and Structure of Neutral and Cationic Aluminum Complexes Incorporating Bis(oxazolinato) Ligands, Organometallics, 2004, 23, 3053–3061 CrossRef CAS.
- K. Jakobsson, T. Chu and G. I. Nikonov, Hydrosilylation of Olefins Catalyzed by Well-Defined Cationic Aluminum Complexes: Lewis Acid versus Insertion Mechanisms, ACS Catal., 2016, 6, 7350–7356 CrossRef CAS.
- A. Friedrich, J. Eyselein, H. Elsen, J. Langer, J. Pahl, M. Wiesinger and S. Harder, Cationic Aluminium Complexes as Catalysts for Imine Hydrogenation, Chem. – Eur. J., 2021, 27, 7756–7763 CrossRef CAS.
- C.-P. Hsu, C.-A. Liu, C.-C. Wen, Y.-H. Liu, Y.-F. Lin and C.-W. Chiu, Chiral Bis(oxazoline) Ligand-Supported Alkyl Aluminum Cations, ChemCatChem, 2022, 14, e202101715 CrossRef CAS.
- L. Werner, J. Hagn, J. Walpuski and U. Radius, Aluminum(III) Cations [(NHC)·AlMes2]+: Synthesis, Characterization, and Application in FLP-Chemistry, Angew. Chem., Int. Ed., 2023, 62, e202312111 CrossRef CAS.
- S. Ju, C. Zhang, B. Tang, L. L. Liu, D. W. Stephan and Y. Wu, The Lewis superacidic aluminium cation: [(NHC)Al(C6F5)2]+, Chem. Commun., 2023, 60, 698–701 RSC.
- A. D. Jaeger, R. Walter, C. Ehm and D. Lentz, Gallium Hydrides and O/N-Donors as Tunable Systems in C−F Bond Activation, Chem. – Asian J., 2018, 13, 2908–2915 CrossRef CAS.
- Addition of DIBAL-H to Al_Py-2,6-Me does not form AlHAl_Py-2,6-Me but quantitatively scavenges the pyridinium borate salt, giving H2 as byproduct.
- V. Busico, R. Pellecchia, F. Cutillo and R. Cipullo, High-throughput screening in olefinpolymerization catalysis: from serendipitous discovery towards rational understanding, Macromol. Rapid Commun., 2009, 30, 1697–1708 CrossRef CAS PubMed.
- A. Vittoria, V. Busico, F. D. Cannavacciuolo and R. Cipullo, Molecular Kinetic Study of ‘chain Shuttling’ Olefin Copolymerization, ACS Catal., 2018, 8, 5051–5061 CrossRef CAS.
- C. Ehm, A. Vittoria, G. P. Goryunov, V. V. Izmer, D. S. Kononovich, O. V. Samsonov, P. H. M. Budzelaar, A. Z. Voskoboynikov, V. Busico, D. V. Uborsky and R. Cipullo, On the limits of tuning comonomer affinity of ‘Spaleck-type’ ansa-zirconocenes in ethene/1-hexene copolymerization: a high-throughput experimentation/QSAR approach, Dalton Trans., 2020, 49, 10162–10172 RSC.
- D. V. Uborsky, D. Y. Mladentsev, B. A. Guzeev, I. S. Borisov, A. Vittoria, C. Ehm, R. Cipullo, C. Hendriksen, N. Friederichs, V. Busico and A. Z. Voskoboynikov, C1-Symmetric Si-bridged (2-indenyl)(1-indenyl) ansa-metallocenes as efficient ethene/1-hexene copolymerization catalysts, Dalton Trans., 2020, 49, 3015–3025 RSC.
- F. D. Cannavacciuolo, R. Yadav, A. Esper, A. Vittoria, G. Antinucci, F. Zaccaria, R. Cipullo, P. H. M. Budzelaar, V. Busico, G. P. Goryunov, D. V. Uborsky, A. Z. Voskoboynikov, K. Searles, C. Ehm and A. S. Veige, A High-Throughput Approach to Repurposing Olefin Polymerization Catalysts for Polymer Upcycling, Angew. Chem., Int. Ed., 2022, 61, e202202258 CrossRef CAS.
- P. Pracht, S. Grimme, C. Bannwarth, F. Bohle, S. Ehlert, G. Feldmann, J. Gorges, M. Müller, T. Neudecker, C. Plett, S. Spicher, P. Steinbach, P. A. Wesołowski and F. Zeller, CREST—A program for the exploration of low-energy molecular chemical space, J. Chem. Phys., 2024, 160, 114110 CrossRef CAS PubMed.
- P. Pracht, F. Bohle and S. Grimme, Automated exploration of the low-energy chemical space with fast quantum chemical methods, Phys. Chem. Chem. Phys., 2020, 22, 7169–7192 RSC.
- L. Falivene, Z. Cao, A. Petta, L. Serra, A. Poater, R. Oliva, V. Scarano and L. Cavallo, Towards the online computer-aided design of catalytic pockets, Nat. Chem., 2019, 11, 872–879 CrossRef CAS.
- G. Knizia and J. E. M. N. Klein, Electron Flow in Reaction Mechanisms—Revealed from First Principles, Angew. Chem., Int. Ed., 2015, 54, 5518–5522 CrossRef CAS.
- G. Knizia, Intrinsic Atomic Orbitals: An Unbiased Bridge between Quantum Theory and Chemical Concepts, J. Chem. Theory Comput., 2013, 9, 4834–4843 CrossRef CAS PubMed.
- X. Desert, J. F. Carpentier and E. Kirillov, Quantification of active sites in single-site group 4 metal olefin polymerization catalysis, Coord. Chem. Rev., 2019, 386, 50–68 CrossRef CAS.
- F. Zaccaria, C. Ehm, P. H. M. Budzelaar, V. Busico and R. Cipullo, Catalyst Mileage in Olefin Polymerization: The Peculiar Role of Toluene, Organometallics, 2018, 37, 2872–2879 CrossRef CAS.
- C. H. Chen, W. C. Shih and C. Hilty, In situ determination of tacticity, deactivation, and kinetics in [rac-(C2H4(1-Indenyl)2)ZrMe][B(C6F5)4] and [Cp2ZrMe][B(C6F5)4]-Catalyzed Polymerization of 1-Hexene Using 13C hyperpolarized NMR, J. Am. Chem. Soc., 2015, 137, 6965–6971 CrossRef CAS PubMed.
- R. Cipullo, P. Melone, Y. Yu, D. Iannone and V. Busico, Olefin polymerisation catalysts: When perfection is not enough, Dalton Trans., 2015, 44, 12304–12311 RSC.
- E. Novarino, I. Guerrero Rios, S. Van Der Veer, A. Meetsma, B. Hessen and M. W. Bouwkamp, Catalyst deactivation reactions: The role of tertiary amines revisited, Organometallics, 2011, 30, 92–99 CrossRef CAS.
- F. Schaper, A. Geyer and H. H. Brintzinger, Displacement of H3CB(C6F5)3− anions from zirconocene methyl cations by neutral ligand molecules: Equilibria, kinetics, and mechanisms, Organometallics, 2002, 21, 473–483 CrossRef CAS.
- F. Ghiotto, C. Pateraki, J. Tanskanen, J. R. Severn, N. Luehmann, A. Kusmin, J. Stellbrink, M. Linnolahti and M. Bochmann, Probing the structure of methylalumoxane (MAO) by a combined chemical, spectroscopic, neutron scattering, and computational approach, Organometallics, 2013, 32, 3354–3362 CrossRef CAS.
- S. Collins, M. Linnolahti, M. G. Zamora, H. S. Zijlstra, M. T. Rodríguez Hernández and O. Perez-Camacho, Activation of Cp2ZrX2 (X = Me, Cl) by Methylaluminoxane As Studied by Electrospray Ionization Mass Spectrometry: Relationship to Polymerization Catalysis, Macromolecules, 2017, 50, 8871–8884 CrossRef CAS.
- F. Zaccaria, P. H. M. Budzelaar, R. Cipullo, C. Zuccaccia, A. Macchioni, V. Busico and C. Ehm, Reactivity Trends of Lewis Acidic Sites in Methylaluminoxane and Some of Its Modifications, Inorg. Chem., 2020, 59, 5751–5759 CrossRef CAS.
- C. Götz, A. Rau and G. Luft, Ternary metallocene catalyst systems based on metallocene dichlorides and AlBu3i/[PhNMe2H] [B(C6F5)4] NMR investigations of the influence of Al/Zr ratios on alkylation and on formation of the precursor of the active metallocene species, J. Mol. Catal. A: Chem., 2002, 184, 95–110 CrossRef.
- M. Bochmann and M. J. Sarsfield, Reaction of AlR3 with [CPh3][B(C6F5)4]: Facile degradation of [B(C6F5)4]− by transient ‘[AlR2]+’, Organometallics, 1998, 17, 5908–5912 CrossRef CAS.
- C. Ehm, A. Mingione, A. Vittoria, F. Zaccaria, R. Cipullo and V. Busico, High-Throughput Experimentation in Olefin Polymerization Catalysis: Facing the Challenges of Miniaturization, Ind. Eng. Chem. Res., 2020, 59, 13940–13947 CrossRef CAS.
- K. Kinashi, K.-P. Lee, S. Matsumoto, K. Ishida and Y. Ueda, Alkyl substituent effects on J- or H-aggregate formation of bisazomethine dyes, Dyes Pigm., 2012, 92, 783–788 CrossRef CAS.
-
M. J. Frisch, et al., Gaussian 16, rev C, Gaussian Inc., Wallingford CT, 2016 Search PubMed.
- C. Ehm, G. Antinucci, P. H. M. Budzelaar and V. Busico, Catalyst activation and the dimerization energy of alkylaluminium compounds, J. Organomet. Chem., 2014, 772, 161–171 CrossRef.
- C. Ehm, P. H. M. Budzelaar and V. Busico, Calculating accurate barriers for olefin insertion and related reactions, J. Organomet. Chem., 2015, 775, 39–49 CrossRef CAS.
- J. Tao, J. P. Perdew, V. N. Staroverov and G. E. Scuseria, Climbing the density functional ladder: Nonempirical meta–generalized gradient approximation designed for molecules and solids, Phys. Rev. Lett., 2003, 91, 146401 CrossRef PubMed.
- N. B. Balabanov and K. A. Peterson, Systematically convergent basis sets for
transition metals. I. All-electron correlation consistent basis sets for the 3d elements Sc-Zn, J. Chem. Phys., 2005, 123, 64107 CrossRef.
- N. B. Balabanov and K. A. Peterson, Basis set limit electronic excitation energies, ionization potentials, and electron affinities for the 3d transition metal atoms: Coupled cluster and multireference methods, J. Chem. Phys., 2006, 125, 74110 CrossRef.
- K. L. Schuchardt, B. T. Didier, T. Elsethagen, L. Sun, V. Gurumoorthi, J. Chase, J. Li and T. L. Windus, Basis set exchange: A community database for computational sciences, J. Chem. Inf. Model., 2007, 47, 1045–1052 CrossRef CAS PubMed.
- Y. Zhao and D. G. Truhlar, The M06 suite of density functionals for main group thermochemistry, thermochemical kinetics, noncovalent interactions, excited states, and transition elements: Two new functionals and systematic testing of four M06-class functionals and 12 other function, Theor. Chem. Acc., 2008, 120, 215–241 Search PubMed.
- J. L. Whitten, Coulombic potential energy integrals and approximations, J. Chem. Phys., 1973, 58, 4496–4501 CrossRef CAS.
- J. Tomasi, Thirty years of continuum solvation chemistry: A review, and prospects for the near future, Theor. Chem. Acc., 2004, 112, 184–203 Search PubMed.
- E. J. Baerends, D. E. Ellis and P. Ros, Self-consistent molecular Hartree-Fock-Slater calculations I. The computational procedure, Chem. Phys., 1973, 2, 41–51 CrossRef CAS.
- M. Feyereisen, G. Fitzgerald and A. Komornicki, Use of approximate integrals in ab initio theory. An application in MP2 energy calculations, Chem. Phys. Lett., 1993, 208, 359–363 CrossRef CAS.
- O. Vahtras, J. Almlöf and M. W. Feyereisen, Integral approximations for LCAO-SCF calculations, Chem. Phys. Lett., 1993, 213, 514–518 CrossRef CAS.
- S. Tobisch and T. Ziegler, Catalytic oligomerization of ethylene to higher linear α-olefins promoted by the cationic group 4, [(η5-Cp-(CMe2-bridge)-Ph)MII(ethylene)2]+ (M = Ti, Zr, Hf) active catalysts: A density functional investigation of the influence of the metal on the cataly, J. Am. Chem. Soc., 2004, 126, 9059–9071 CrossRef CAS PubMed.
- F. Zaccaria, C. Ehm, P. H. M. Budzelaar and V. Busico, Accurate prediction of copolymerization statistics in molecular olefin polymerization catalysis: The role of entropic, electronic, and steric effects in catalyst comonomer affinity, ACS Catal., 2017, 7, 1512–1519 CrossRef CAS.
Footnote |
† Electronic supplementary information (ESI) available: Additional synthetic, characterization and computational details. CCDC 2372888–2372892. For ESI and crystallographic data in CIF or other electronic format see DOI: https://doi.org/10.1039/d4qi01874e |
|
This journal is © the Partner Organisations 2024 |
Click here to see how this site uses Cookies. View our privacy policy here.